Non-Invasive Electrochemical Biosensors for TNF-α Cytokines Detection in Body Fluids
- 1Department of Cardiovascular, The Second Hospital of Jilin University, Jilin University, Changchun, China
- 2State Key Laboratory on Integrated Optoelectronics, College of Electronic Science and Engineering, Jilin University, Changchun, China
The measurement of pro-inflammatory cytokine tumour necrosis factor-alpha (TNF-α), which is an important indicator of the inflammatory process, has received increasing attention recently because it is easy to extract from body fluid and serves as an early sign of a serious systemic inflammatory disease. Developing fast and simple detection methods to quantify the concentration of TNF-α is essential. Saliva, tears, and urine, which can easily be sampled in a non-invasive way, are considered to be important matrices for monitoring and assessing the physiological status of humans; importantly, they also provide an ideal window for monitoring the concentration of TNF-α. As a fast, accurate, inexpensive, portable, and scalable method, electrochemical biosensors are very promising for biomarker detection in matrices obtained in a non-invasive manner. This review summarises and compares the electrochemical biosensors for the detection of TNF-α in a non-invasive manner and highlights recent advances and future prospects in developing high-performance electrochemical platforms for noninvasive measurement of TNF-α.
Introduction
Tumour necrosis factor alpha (TNF-α), a pleiotropic cytokine produced by activating monocytes/macrophages in the inflammatory process, is considered an important biomarker of autoimmune diseases (Arya et al., 2007; Ali et al., 2011; Altintas et al., 2014; Kalliolias and Ivashkiv, 2016). It has distinct roles in modulating a series of biological processes, such as in rheumatoid arthritis, human immunodeficiency virus infection, Alzheimer’s disease, stroke, diabetic retinopathy (Boss et al., 2017), and colon and lung cancers (Sadighbayan et al., 2019). It is also related to the severity of cardiovascular lesions and is involved in suppressing myocardial contractility, elevating the content of cardiac peroxynitrite and nitric oxide, changes in intracellular calcium homeostasis, and induction of pathological changes in the failing myocardium (Berthonneche et al., 2004). Moreover, the TNF-α cytokine plays a role in preventing bone and cartilage damage and assisting wound healing (Lipsky et al., 2000; Sandborn et al., 2007). Therefore, developing an accurate and fast TNF-α measurement technique is highly desirable for predicting, preventing, assessing, and monitoring inflammation.
Over the past few decades, evaluating physiological status, detecting illness at an early stage, monitoring disease progression, and assessing therapeutic outcomes through non-invasive methods has become the most ideal and promising approach for clinical diagnosis and research (Bellagambi et al., 2020; Loo and Pui, 2020; Vozgirdaite et al., 2021). Previous studies have revealed that TNF-α can be easily extracted from non-invasively obtained body fluids, such as saliva, tears, and urine (Brennan and Galvin, 2018; Yanez-Sedeno et al., 2019). Monitoring the trace concentration of TNF-α in these body fluids provides a painless, simple, real-time means for cardiovascular disease diagnosis; in particular, it may serve as a sign of inflammation, which is important for the early diagnosis of some acute diseases, such as acute heart failure. However, the level of TNF-α in non-invasively obtained body fluids is extremely low. For example, the TNF-α concentration in the saliva of healthy humans is lower than 3 pg ml−1 and increases to about 30 pg ml−1 in the saliva of patients with severe autoimmune diseases (Liu and Duan, 2012; Bellagambi et al., 2017). The concentration of urinary TNF-α was determined to be 4 pg mg−1 in healthy individuals, normalised by the concomitant urinary creatinine level (to compensate for the TNF-α variations in urine fluid) (Bellagambi et al., 2017). Its concentration can be up to 196.9 ± 121.2 pg/ml in tear fluid, and research is beginning to focus on how immune and inflammatory diseases affect the content of TNF-α in tear fluid, which has a less complex biological composition (Comoglu et al., 2013; Costagliola et al., 2013). Therefore, methods offering low limits of detection and high sensitivity are of great interest for clinical diagnosis and research.
For this purpose, intensive research on TNF-α determination has been conducted, using techniques such as enzyme-linked immunosorbent assay (ELISA), radio immunoassays, chemiluminescence imaging, mass spectrometry, fluorescence immunoassay, and electrochemical immunosensors (Bosnjakovic et al., 2012). Among these techniques, electrochemical biosensors are considered promising platforms that take into account the advantages of easy miniaturisation, small sample volume, simple operation, and low cost (Arya and Bhansali, 2011; Baraket et al., 2016; Povedano et al., 2018). In addition, compared to the ELISA method, which is commonly used in clinical analysis, electrochemical biosensors are more flexible and time-saving, contributing to the rapid evaluation of inflammatory TNF-α (Baydemir et al., 2016). This mini-review summarises the recent progress in the development of electrochemical biosensors for TNF-α determination in a non-invasive manner, and highlights recent advances and future prospects in developing high-performance electrochemical platforms for non-invasive determination of TNF-α biomarkers.
Non-Invasive Electrochemical Biosensors for Salivary TNF-α
Immunosensors for Detection of TNF-α in Saliva
As a promising tool for non-invasive detection, the saliva matrix can comprise more than 25% of the proteins in the human serum with strong correlations. Benefiting from the characteristics of easy collection and storage as well as good stability, saliva detection can effectively decrease the demand for cost, sophisticated instruments, and professional operators. In this section, recent studies focused on the detection of salivary TNF-α are summarised, and their advantages and disadvantages are discussed.
The immunosensor technique with different sensing receptors and transducers is considered as a critical method in biomarker screening (Raluca-Ioana et al., 2000; Baraket et al., 2012) and is considered to be the most promising candidate to compete with well-established laboratory techniques. Barhoumi et al. (2018) designed an electrochemical immunosensor on a gold working electrode (Figure 1A). After modifying 4-carboxymethylaniline (CMA) via electrodeposition, the surface of the electrode was activated by ethanolamine, and then the anti-TNF-α antibody was further functionalised through the carboxylic acid groups of CMA. For TNF-α determination, a secondary antibody (Ab-TNF-α-HRP) labelled with horseradish peroxidase (HRP) was employed to utilise a sandwich-type strategy in tetramethylbenzidine solution. Since the concentration of TNF-α showed a good correlation with heart failure and its severity, the sensor was applied to detect different levels of Ag-TNF-α in artificial saliva. The as-fabricated biosensor exhibited a linear detection range of 1–30 pg ml−1 with a fast response in 5 s to the Ag-TNF-α cytokine (Figure 1B). In addition, it showed good selectivity against other cytokines, such as interleukin (IL)-10 and the hormone cortisol. The above sensing performance of the as-built biosensor enables it to serve as a potential tool for fast and accurate quantification of Ag-TNF-α biomarkers. However, the sensing performance of this biosensor was evaluated in artificial saliva, and further clinical examinations should be performed. Similarly, a bifunctional electrochemical immunosensor for the detection of TNF-α and IL-1β cytokines was reported by Sanchez-Tirado et al. (2017). In this study, they used screen-printed carbon electrodes (SPCEs) with dual working electrodes as the sensing platform and then introduced CMA as scaffolds for further modification of the corresponding antibodies (Figure 1C). Such a dual immunosensor displayed a linearity range of 1–100 pg ml−1 with a limit of detection (LOD) of 0.85 pg ml−1 and 0.5–100 pg ml−1 with an LOD of 0.38 pg ml−1 for TNF-α and IL-1β, respectively, which was sufficient for application in clinical testing. In addition, the cross-talk between the adjacent SPCEs that were modified with anti-IL or anti-TNF in the dual immunosensor was non-perceptible, and no obvious differences were detected in the amperometric responses of immunosensors when faced with the non-target antigen or without the corresponding target antigen (Figure 1D). When tested in the saliva sample, the results obtained from the dual electrochemical immunosensor showed almost consistent results with the clinical data measured from the ELISA arrays. Note that the reagent consumption was also very low (2.5 μl) compared to that of ELISA (100 μl).
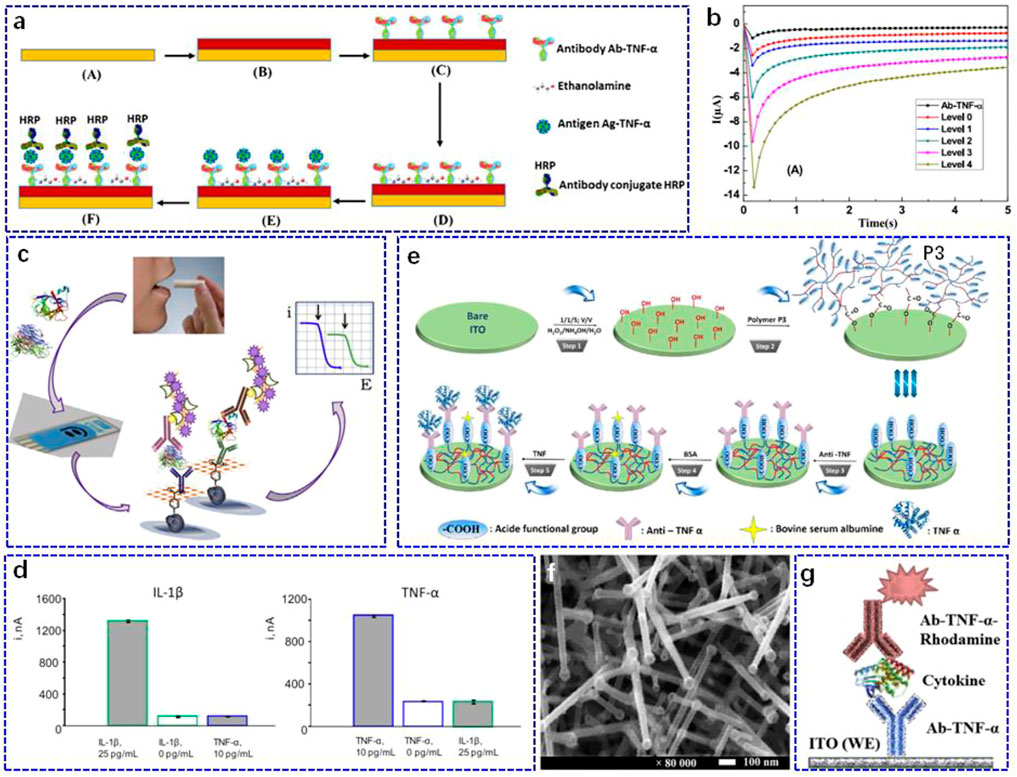
FIGURE 1. (A) Schematic illustration of the immunosensor preparation: A. bare gold, B. electro-addressing of 4-carboxymethylaniline (CMA) on gold working electrode followed by CMA activation, C. biofunctionalisation through incubation with Ab-TNF-α, D. deactivation of the remaining active carboxylic acid groups by incubation in ethanolamine solution, E. incubation of the device in Ag-TNF-α solution, F. subsequent incubation in Ab-TNF-α-HRP solution. (B) Chronoamperometric analyses for different incubation of the immunosensor in real human saliva. Reproduced with permission from Barhoumi et al.(2018). Copyright 2018, Elsevier Science SA. (C) Schematic illustration of the electrochemical immunosensor for simultaneous determination of TNF-α and IL-1β using dual SPEs. (D) Amperometric responses recorded with the dual immunosensor for right: 25 or 0 pg ml−1 IL-1β (green), or 10 pg ml−1 TNF-a (blue) (right), and left: for 10 or 0 pg ml−1 TNF-a (blue), or 25 pg ml−1 IL-1β (green). Reproduced with permission from Sanchez-Tirado et al., 2017). Copyright 2017, Elsevier. (E) Schematic illustration of the immunosensor based on ITO thin films covered by P3-conjugated polymer. Reproduced with permission from Aydin et al., 2017). Copyright 2017, Elsevier Advanced Technology. (F) Surface characterisation of the nanostructured electrode by scanning electron microscopy. (G) Schematic illustration of sandwich-type detection for nanostructured ITO electrode-based electrochemical immunosensors. Reproduced with permission from Pruna et al., 2018a). Copyright 2018, Pergamon-Elsevier Science Ltd.
Both of these immunosensors used CMA as the conjugated and blocking layer; however, the weak electron transfer capacity limits the sensing performance to some extent (Barhoumi et al., 2019). To avoid this problem, Aydin et al. (2017) developed a label-free immunosensor for salivary TNF-α determination by applying a semiconductive polymer (poly (3-thiophene acetic acid, P3)) on an indium tin oxide (ITO) electrode (Figure 1E). Polymer P3 not only showed good conductivity but was also rich in carboxylic acid groups, which was beneficial for the effective immobilisation of the anti-TNF-α antibodies. Using P3 as the conjugated matrix, the linear detection response of the developed immunosensor had an LOD of 3.7 fg ml−1, which was suitable for clinical salivary sample determination. To evaluate the practicability of the P3-based electrochemical immunosensor, it was used to quantify the level of TNF-α in clinical saliva from a local hospital, and it showed recoveries between 98.39 and 105.20%, demonstrating the good potential of the developed immunosensor to be employed as an accurate diagnostic tool for TNF-α determination. Using a similar strategy, the sensing performance of the TNF-α immunosensor could be improved by using a polymer-coated magnetic microparticles matrix for antibody immobilisation (Barhoumi et al., 2019).
Although the above polymer-coated immunosensors could realise the improvement of the sensing properties, the employed electrode-modified strategy still requires polymers or other layers to cover the whole surface (Eletxigerra et al., 2015; Arya and Estrela, 2020; Nessark et al., 2020), which may require additional nanostructures to maintain a larger surface area (Hou et al., 2013; Baraket et al., 2017; Yola and Atar, 2021). For this purpose, Pruna et al. (2018a) constructed a nanostructured ITO electrode with a high surface-to-volume ratio to build opto-electrochemical sensors (Figure 1F). Together with the good optical transparency and electrical conductivity, a nanostructured ITO electrode was fabricated to sandwich the antibody-antigen type immunosensor for TNF-α recognition (Figure 1G). Because of its good linear range of 10–100 pg ml−1, the proposed immunosensor was expected to trigger a stable response to trace TNF-α in salivary samples from healthy patients.
Other Electrochemical Biosensors
Apart from the typical amperometric method, electrochemical biosensors based on the principle of capacitance as well as biosensors with aptamers as recognition species have also been developed for TNF-α in saliva. Bahri et al. (2020) studied a capacitance electrochemical biosensor using a silicon nitride (Si3N4) transducer (Figure 2A). After activation by the piranha solution, silanol and silylamine groups were introduced on the surface of the Si3N4 transducer in conjunction with bio/chemical substances. Then, a self-assembled monolayer of aldehyde-silane (11-(triethoxysilyl) undecanal) (TEUSD) was further modified for anti-TNF-α bonding, and the bonded condition was checked using the micro-contact printing technique. The sandwich antibody-antigen bio-recognition was chosen for TNF-α determination, in which the anti-TNF-α antibody was labelled with rhodamine. Mott-Schottky analyses of this capacitance electrochemical biosensor were used for TNF-α quantification. The result showed that this biosensor had a sensitivity of 4.4 mV pM−1 with an LOD of 1 pg ml−1 in artificial saliva (Figure 2B). Furthermore, it displayed good selectivity against cortisol and IL-10.
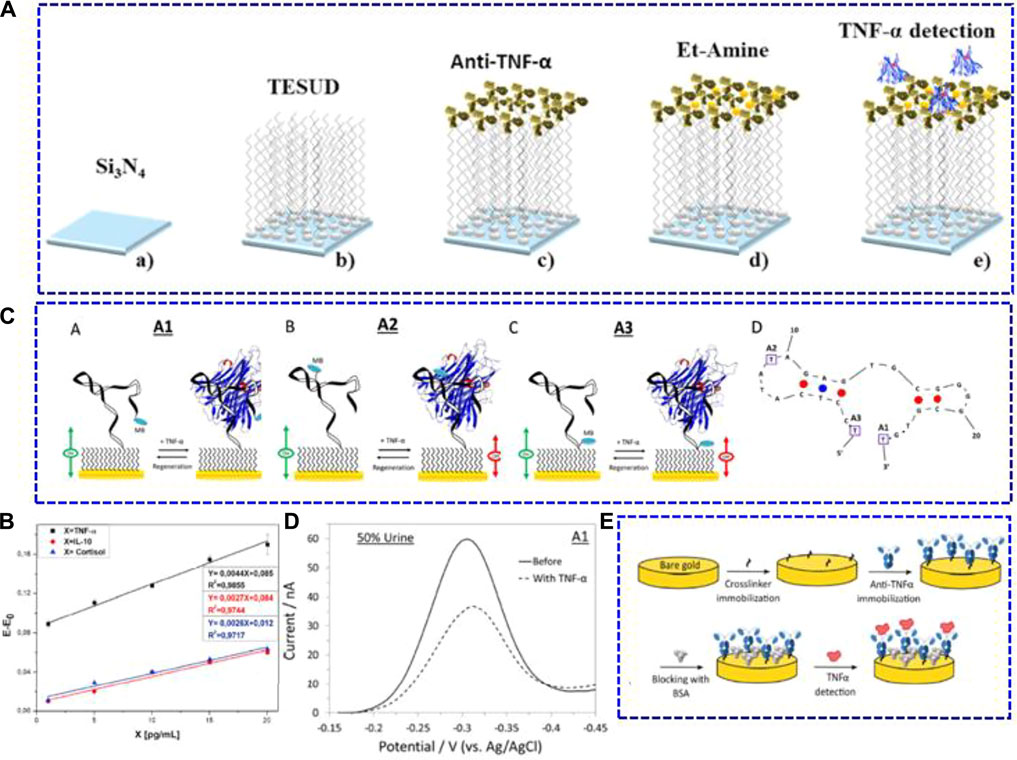
FIGURE 2. (A) Schematic illustrations of the chemical surface modification and biofunctionalisation process of the immunosensor with anti-TNF-α antibodies. a) Si3N4 surface cleaning and activation, (B) Si3N4 surface functionalisation with TESUD, (C) anti-TNF-α antibodies immobilisation step, d) Si3N4 surface blocking using 1% ethanolamine, and (E) electrochemical TNF-α detection step. (B) Detection sensitivity curves of Ag-TNF-α, IL-10 cytokine, and cortisol in artificial saliva. Reproduced with permission from Bahri et al. (2020). Copyright 2020, Elsevier.(C) Schematic illustrations of the three electrochemical aptamer-based TNF-α biosensors (A–C) and the optimal (minimum energy) structure of the adopted aptamer (D). (D) Alternating current voltammetry of the A1 sensor in the absence and presence of 100 nM TNF-α in a 50% synthetic urine sample. Reproduced with permission from Mayer and Lai (2018) Copyright 2018, Elsevier.(E) Schematic representation of the immunosensor biofunctionalisation process. Reproduced with permission from Cruz et al. (2019). Copyright 2019, American Chemical Society.
Aptamers are single-stranded DNA or RNA molecules that can bind to specific targets in terms of base pairing (Tuerk and Gold, 1990; Ellington and Szostak, 1992). Unlike antibodies utilised in immunosensors, biosensors using aptamers as biorecognition components are more stable under different experimental conditions and are much cheaper than the generation of antibodies. Mayer and Lai. (2018) studied electrochemical aptamer-based TNF-α biosensors using three aptamer probes labelled with methylene blue (Figure 2C). When exposed to TNF-α, the conformation of the chosen aptamer probe changed, which adjusted the electron transfer capacity of the labelled methylene blue. As a result, the corresponding redox current was altered. Among these biosensors, the one employing the aptamer with methylene blue modified at the distal end (A1 biosensor, as shown in Figure 2C) had the best sensing performance. This biosensor showed an LOD of 100 pM and good reproducibility. Moreover, it exhibited a good response in synthetic fluid with 50% urine and 50% saliva (Figure 2D).
In addition to saliva, electrochemical biosensors for TNF-α in other non-invasively obtained body fluids, such as tears, were also studied. Cruz et al. (2019) built a highly sensitive label-free immunosensor for TNF-α screening (Figure 2E). The SPE (screen-printed electrodes) with gold as the working part was modified with sulfo-LC-SPDP (sulfosuccinimidyl 6-(3’-(2-pyridyldithio) propionamido) hexanoate) as a crosslinker for anti-TNF-α immobilisation. After blocking with bovine serum albumin, the biosensor was further bonded with TNF-α for quantitative determination. The sensing performance of the as-built immunosensor was tested using Nyquist plots. As calculated, it exhibited an LOD of 0.085 pg ml−1 in PBS. To investigate the practical applicability in clinical samples, the immunosensor was applied to measure the trace concentration of TNF-α in tears, blood serum, and human cerebral spinal fluid. As a result, the immunosensor could detect TNF-α in tears beyond the LOD of ELISA (4 pg ml−1).
Smart Point-of-Care Biosensors
With the rapid development of technology, the need for intelligent POC devices in clinical and home self-monitoring is rapidly increasing, especially for patients with cardiovascular disease. Longo et al. (2016) constructed an integrated electrochemical bioMEMS for TNF-α cytokine detection. Because the BioMEMS had eight gold working microelectrodes (integrated reference and counter electrodes on one chip), it allowed for the simultaneous detection of multiple target molecules through the antibody-antigen bio-recognition mode (Figure 3A). Using EIS characterisation, the BioMEMS platform exhibited a linear detection range of 1–15 pg ml−1 in artificial human saliva against other cytokines, such as IL-1 and IL-8. They further applied the same system for TNF-α determination in human saliva with a linear range of 1–100 pg ml−1 and an LOD of 3.1 pg ml−1 (Figure 3B) (Bellagambi et al., 2017). Miniaturising the potentiostat in POC electrochemical biosensing systems while maintaining their effective characteristics is still a challenge. Using a similar BioMEMS electrode, Pruna et al. (2018b) integrated analogue circuitry, microcontrollers, and digital filter implementation to avoid the complex structures of the previously reported circuits, which have a total size of 12 × 7 × 2 cm3. This electronic system was further connected to BioMEMS electrodes for TNF-α determination (Figure 3C). This device had a high sensitivity in the randomly chosen range of 266 pg ml−1–666 ng ml−1.
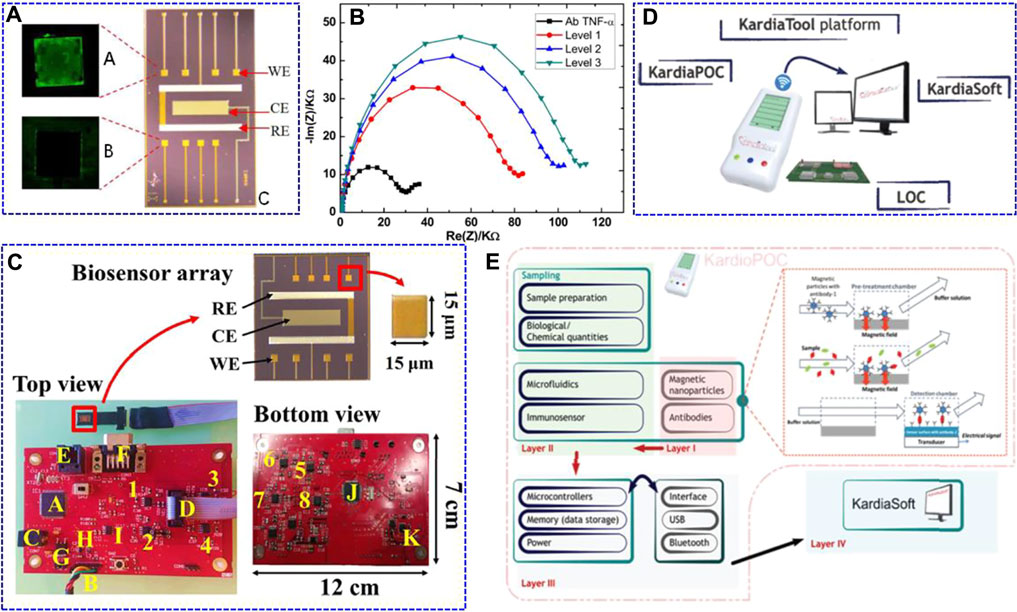
FIGURE 3. Fluorescence optical images of A: anti-TNF-α/TNF-α-modified working electrode (WE) after incubation in secondary fluorescent TNF-α, B: no functionalised WE, and C: optical image of the fully integrated BioMEMS. WE: working electrode, CE: counter electrode, RE: reference electrode. Reproduced with permission from Longo et al. (2016). Copyright 2016, Elsevier Science BV. (B) Nyquist impedance plots obtained from the standard addition method performed on a real saliva sample (black point) Ab TNF-α for Ag TNF-α (red point) Level 1 (corresponding to an addition of 0 pg ml−1); (blue point) Level 2 (addition of 3 pg ml−1); (green point) Level 3 (addition of 7 pg ml−1). Reproduced with permission from Bellagambi et al. (2017). Copyright 2017, Elsevier Science SA. (C) Manufacturing details of the printed circuit board and the biosensor array. The potentiostatic circuits for the different channels are labelled as 1–4 (top view) and 5–8 (bottom view). Regarding the rest of the elements, A) is the PIC32 microcontroller; B) is the UART connector for PC communication; C) is the external power supply; D) is the 10-pin connector for the biosensor array; E) is the RJ-11 connector to program the microcontrollers; F) is the data bus; G) is the LM1117 voltage regulator; H) is the MCP1603 B voltage regulator; I) is the ADR4525 stable voltage reference; J) is the PIC24 microcontroller, and K) is the TC962 voltage regulator. Reproduced with permission from Pruna et al. (2018b). Copyright 2018, Elsevier Advanced Technology. (D) KardiaTool concept. (E) High level architecture of the KardiaTool platform. Reproduced with permission Tripoliti et al. (2018). Copyright 2018, IEEE Engineering in Medicine and Biology Society.
Tripoliti et al. (2018) presented an integrated device with the name of Kardiatool platform as an example for the non-invasive diagnosis of heart failure (Figure 3D). This device consists of two parts: a portable POC device (KardioPOC) and the corresponding software. The KardioPOC has a first layer with magnetic nanoparticles and antibodies for target species recognition, and then an added microfluidic system, immunosensor component, and saliva samples for measurement. The third layer is composed of integrated circuit sections, such as the power supply, memory, microcontroller, and communication module (Figure 3E). In addition, it can simultaneously measure four different biomarkers, such as TNF-α, IL-10, N-terminal pro b-type natriuretic peptide (NT-pro BNP), and cortisol. This platform provides a proof of concept for the POC device for heart failure evaluation. The above work is promising for the development of low-cost, portable, and time-saving electrochemical systems for POC TNF-α determination. However, POC platforms for in situ tracking of cardiovascular diseases are not satisfactory, and the development of new types of biosensors is still needed.
Conclusion
This review summarises the progress in electrochemical biosensors for non-invasive detection of TNF-α cytokines, including immunosensors for detection of TNF-α in saliva, electrochemical biosensors based on other principles or other non-invasive fluids (such as tears), and smart POC biosensors. Among these sensors, saliva testing plays an important role in non-invasive TNF-α cytokine determination because of its ability to be easily collected, good stability, and suitable TNF-α concentration within the detection capability of electrochemical biosensors. These electrochemical biosensors for non-invasive detection of TNF-α cytokines have promising prospects for continuous, fast, real-time, and portable monitoring of cardiovascular disease. Moreover, smart POC biomarkers provide a great solution for chronic disease self-management, such as chronic heart failure. To make a clearer comparison, the sensing performance, such as sensitivity and specificity, of different non-invasive biosensors that discussed in this review are listed in Table 1. As compared, the most immunosensors can response to TNF-α concentration level of pg mL−1. Due to the different electrochemical methods, It’s hard to quantitatively compare the sensitivity. However, the selectivity is generally good, which is related to the well-designed structure and specific recognition of Ab-TNF-α. Especially, the Au WE/sulfo-LC-SPDP/Ab-TNF-α immunosensor built by Cruz et al. exhibited a high specificity to TNF-α, which is at least 6 times higher than interferon gamma (IFNγ) and IL-4. This can be attributed to the introduction of SPDP monolayer which is considered to be effective for Ab-TNF-α immobilization.
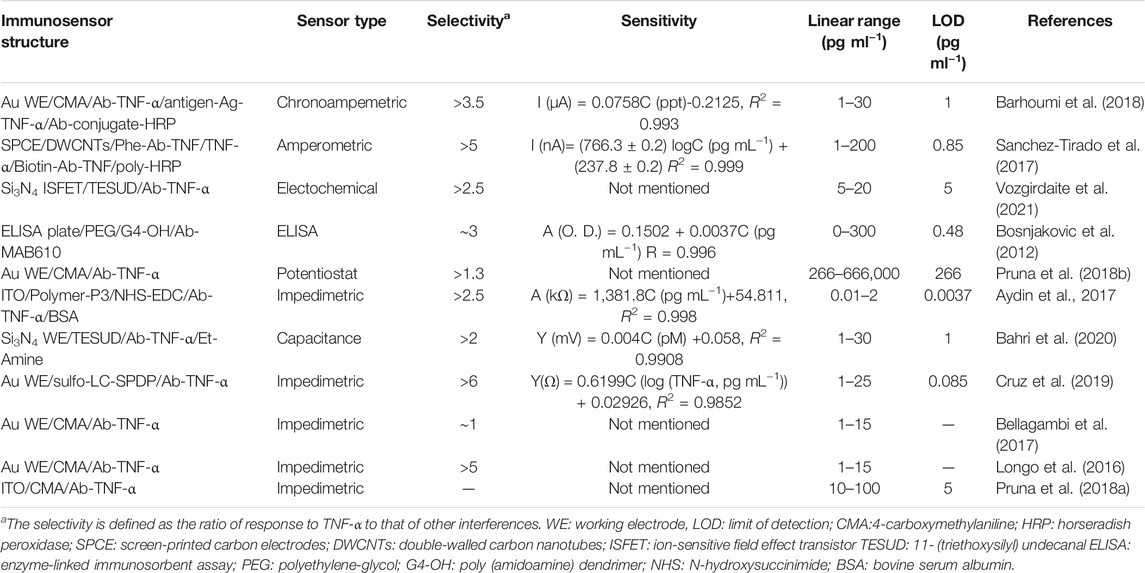
TABLE 1. A comparison of the sensing performance of non-invasive immunosensors discussed in the review.
Although research attention has been rapidly gained in the area of non-invasive TNF-α cytokine detection via electrochemical biosensors, further optimisation of sensor reliability should be critically investigated, and biosensors that can reliably and accurately determine TNF-α in clinical settings are still in their infancy. Besides the TNF-α cytokine, lactate, 8-isoprostalandin F2α, C-reactive protein, and IL-1β from saliva can also act as biomarkers for cardiovascular disease (Aydın et al., 2018; Vilian et al., 2019; Ghimenti et al., 2020). Designing biosensors that can simultaneously detect multiple biomarkers is necessary to improve accuracy and reliability. Owing to the accessibility of non-invasive body fluids, especially saliva, the design of portable and integrated devices for POC applications in remote and self-monitoring is promising, and related studies are needed.
Author Contributions
YL and LX conceived the idea. YL and QZ wrote the manuscript and equally contributed in the study with support from LX.
Funding
This work was supported by the National Natural Science Foundation of China (Grant Nos. 61874049, 61775080, 61822506), the Jilin Province Natural Science Foundation of China (No. 20200801017GH), and the Fundamental Research Funds for the Central Universities of China.
Conflict of Interest
The authors declare that the research was conducted in the absence of any commercial or financial relationships that could be construed as a potential conflict of interest.
Publisher’s Note
All claims expressed in this article are solely those of the authors and do not necessarily represent those of their affiliated organizations, or those of the publisher, the editors and the reviewers. Any product that may be evaluated in this article, or claim that may be made by its manufacturer, is not guaranteed or endorsed by the publisher.
References
Ali, M. K. M., Ibrahim, K., Hamad, O. S., Eisa, M. H., Faraj, M. G., and Azhari, F. (2011). Deposited Indium Tin Oxide (ITO) Thin Films by Dcmagnetron Sputtering on Polyethylene Terephthalate Substrate (PET). Rom. J Phys. 56, 730–741.
Altintas, Z., Fakanya, W. M., and Tothill, I. E. (2014). Cardiovascular Disease Detection Using Bio-Sensing Techniques. Talanta 128, 177–186. doi:10.1016/j.talanta.2014.04.060
Arya, S. K., and Bhansali, S. (2011). Lung Cancer and its Early Detection Using Biomarker-Based Biosensors. Chem. Rev. 111, 6783–6809. doi:10.1021/cr100420s
Arya, S. K., and Estrela, P. (2020). Electrochemical ELISA Protein Biosensing in Undiluted Serum Using a Polypyrrole-Based Platform. Sensors 20, 2857. doi:10.3390/s20102857
Arya, S. K., Prusty, A. K., Singh, S. P., Solanki, P. R., Pandey, M. K., Datta, M., et al. (2007). Cholesterol Biosensor Based on N-(2-aminoethyl)-3-aminopropyl-trimethoxysilane Self-Assembled Monolayer. Anal. Biochem. 363, 210–218. doi:10.1016/j.ab.2007.01.029
Aydın, E. B., Aydın, M., and Sezgintürk, M. K. (2017). A Highly Sensitive Immunosensor Based on ITO Thin Films Covered by a New Semi-conductive Conjugated Polymer for the Determination of TNFα in Human Saliva and Serum Samples. Biosens. Bioelectron. 97, 169–176. doi:10.1016/j.bios.2017.05.056
Aydın, E. B., Aydın, M., and Sezgintürk, M. K. (2018). Highly Sensitive Electrochemical Immunosensor Based on Polythiophene Polymer with Densely Populated Carboxyl Groups as Immobilization Matrix for Detection of Interleukin 1β in Human Serum and Saliva. Sensors Actuators B: Chem. 270, 18–27. doi:10.1016/j.snb.2018.05.014
Bahri, M., Baraket, A., Zine, N., Ben Ali, M., Bausells, J., and Errachid, A. (2020). Capacitance Electrochemical Biosensor Based on Silicon Nitride Transducer for TNF-α Cytokine Detection in Artificial Human Saliva: Heart Failure (HF). Talanta 209, 120501. doi:10.1016/j.talanta.2019.120501
Baraket, A., Lee, M., Zine, N., Sigaud, M., Bausells, J., and Errachid, A. (2017). A Fully Integrated Electrochemical Biosensor Platform Fabrication Process for Cytokines Detection. Biosens. Bioelectron. 93, 170–175. doi:10.1016/j.bios.2016.09.023
Baraket, A., Lee, M., Zine, N., Yaakoubi, N., Bausells, J., and Errachid, A. (2016). A Flexible Electrochemical Micro Lab-On-Chip: Application to the Detection of Interleukin-10. Microchim Acta 183, 2155–2162. doi:10.1007/s00604-016-1847-y
Baraket, A., Lee, M., Zine, N., Yaakoubi, N., Trivella, M. G., Zabala, M., et al. (2012). Cytokine Detection Using Diazonium Modified Gold Microelectrodes onto Polyimide Substrates with Integrated Ag/AgCl Reference Electrode. Proced. Eng. 47, 1181–1184. doi:10.1016/j.proeng.2012.09.362
Barhoumi, L., Baraket, A., Bellagambi, F. G., Karanasiou, G. S., Ali, M. B., Fotiadis, D. I., et al. (2018). A Novel Chronoamperometric Immunosensor for Rapid Detection of TNF-α in Human Saliva. Sensors Actuators B: Chem. 266, 477–484. doi:10.1016/j.snb.2018.03.135
Barhoumi, L., Bellagambi, F., Vivaldi, F., Baraket, A., Clément, Y., Zine, N., et al. (2019). Ultrasensitive Immunosensor Array for TNF-α Detection in Artificial Saliva Using Polymer-Coated Magnetic Microparticles onto Screen-Printed Gold Electrode. Sensors 19, 692. doi:10.3390/s19030692
Baydemir, G., Bettazzi, F., Palchetti, I., and Voccia, D. (2016). Strategies for the Development of an Electrochemical Bioassay for TNF-Alpha Detection by Using a Non-immunoglobulin Bioreceptor. Talanta 151, 141–147. doi:10.1016/j.talanta.2016.01.021
Bellagambi, F. G., Baraket, A., Longo, A., Vatteroni, M., Zine, N., Bausells, J., et al. (2017). Electrochemical Biosensor Platform for TNF-α Cytokines Detection in Both Artificial and Human Saliva: Heart Failure. Sensors Actuators B: Chem. 251, 1026–1033. doi:10.1016/j.snb.2017.05.169
Bellagambi, F. G., Lomonaco, T., Salvo, P., Vivaldi, F., Hangouët, M., Ghimenti, S., et al. (2020). Saliva Sampling: Methods and Devices. An Overview. Trac Trends Anal. Chem. 124, 115781. doi:10.1016/j.trac.2019.115781
Berthonneche, C., Sulpice, T., Boucher, F., Gouraud, L., De Leiris, J., O'connor, S. E., et al. (2004). New Insights into the Pathological Role of TNF-α in Early Cardiac Dysfunction and Subsequent Heart Failure after Infarction in Rats. Am. J. Physiology-Heart Circulatory Physiol. 287, H340–H350. doi:10.1152/ajpheart.01210.2003
Bosnjakovic, A., Mishra, M. K., Han, H. J., Romero, R., and Kannan, R. M. (2012). A Dendrimer-Based Immunosensor for Improved Capture and Detection of Tumor Necrosis Factor-α Cytokine. Analytica Chim. Acta 720, 118–125. doi:10.1016/j.aca.2012.01.017
Boss, J. D., Singh, P. K., Pandya, H. K., Tosi, J., Kim, C., Tewari, A., et al. (2017). Assessment of Neurotrophins and Inflammatory Mediators in Vitreous of Patients with Diabetic Retinopathy. Invest. Ophthalmol. Vis. Sci. 58, 5594–5603. doi:10.1167/iovs.17-21973
Brennan, D., and Galvin, P. (2018). Flexible Substrate Sensors for Multiplex Biomarker Monitoring. MRS Commun. 8, 627–641. doi:10.1557/mrc.2018.134
Çomoğlu, S. S., Güven, H., Acar, M., Öztürk, G., and Koçer, B. (2013). Tear Levels of Tumor Necrosis Factor-Alpha in Patients with Parkinson's Disease. Neurosci. Lett. 553, 63–67. doi:10.1016/j.neulet.2013.08.019
Costagliola, C., Romano, V., De Tollis, M., Aceto, F., dell’Omo, R., Romano, M. R., et al. (2013). TNF-alpha Levels in Tears: A Novel Biomarker to Assess the Degree of Diabetic Retinopathy. Mediators Inflamm. 2013, 1–6. doi:10.1155/2013/629529
Cruz, A., Queirós, R., Abreu, C. M., Barata, C., Fernandes, R., Silva, R., et al. (2019). Electrochemical Immunosensor for TNFα-Mediated Inflammatory Disease Screening. ACS Chem. Neurosci. 10, 2676–2682. doi:10.1021/acschemneuro.9b00036
Eletxigerra, U., Martinez-Perdiguero, J., and Merino, S. (2015). Disposable Microfluidic Immuno-Biochip for Rapid Electrochemical Detection of Tumor Necrosis Factor Alpha Biomarker. Sensors Actuators B: Chem. 221, 1406–1411. doi:10.1016/j.snb.2015.08.026
Ellington, A. D., and Szostak, J. W. (1992). Selection In Vitro of Single-Stranded DNA Molecules that Fold into Specific Ligand-Binding Structures. Nature 355, 850–852. doi:10.1038/355850a0
Ghimenti, S., Lomonaco, T., Bellagambi, F. G., Biagini, D., Salvo, P., Trivella, M. G., et al. (2020). Salivary Lactate and 8-isoprostaglandin F2α as Potential Non-invasive Biomarkers for Monitoring Heart Failure: a Pilot Study. Sci. Rep. 10, 7441. doi:10.1038/s41598-020-64112-2
Hou, Y., Li, T., Huang, H., Quan, H., Miao, X., and Yang, M. (2013). Electrochemical Immunosensor for the Detection of Tumor Necrosis Factor α Based on Hydrogel Prepared from Ferrocene Modified Amino Acid. Sensors Actuators B: Chem. 182, 605–609. doi:10.1016/j.snb.2013.03.067
Kalliolias, G. D., and Ivashkiv, L. B. (2016). TNF Biology, Pathogenic Mechanisms and Emerging Therapeutic Strategies. Nat. Rev. Rheumatol. 12, 49–62. doi:10.1038/nrrheum.2015.169
Lipsky, P. E., Van Der Heijde, D. M. F. M., St. Clair, E. W., Furst, D. E., Breedveld, F. C., Kalden, J. R., et al. (2000). Infliximab and Methotrexate in the Treatment of Rheumatoid Arthritis. N. Engl. J. Med. 343, 1594–1602. doi:10.1056/nejm200011303432202
Liu, J., and Duan, Y. (2012). Saliva: a Potential media for Disease Diagnostics and Monitoring. Oral Oncol. 48, 569–577. doi:10.1016/j.oraloncology.2012.01.021
Longo, A., Baraket, A., Vatteroni, M., Zine, N., Baussells, J., RogerFuocoDi Francesco, F., et al. (2016). Highly Sensitive Electrochemical BioMEMS for TNF-α Detection in Humansaliva: Heart Failure. Proced. Eng. 168, 97–100. doi:10.1016/j.proeng.2016.11.156
Loo, S. W., and Pui, T.-S. (2020). Cytokine and Cancer Biomarkers Detection: The Dawn of Electrochemical Paper-Based Biosensor. Sensors 20, 1854. doi:10.3390/s20071854
Mayer, M. D., and Lai, R. Y. (2018). Effects of Redox Label Location on the Performance of an Electrochemical Aptamer-Based Tumor Necrosis Factor-Alpha Sensor. Talanta 189, 585–591. doi:10.1016/j.talanta.2018.07.055
Nessark, F., Eissa, M., Baraket, A., Zine, N., Nessark, B., Zouaoui, A., et al. (2020). Capacitance Polypyrrole‐based Impedimetric Immunosensor for Interleukin‐10 Cytokine Detection. Electroanalysis 32, 1795–1806. doi:10.1002/elan.201900633
Povedano, E., Vargas, E., Montiel, V. R.-V., Torrente-Rodríguez, R. M., Pedrero, M., Barderas, R., et al. (2018). Electrochemical Affinity Biosensors for Fast Detection of Gene-specific Methylations with No Need for Bisulfite and Amplification Treatments. Sci. Rep. 8, 6418. doi:10.1038/s41598-018-24902-1
Pruna, R., Baraket, A., Bonhommé, A., Zine, N., Errachid, A., and López, M. (2018a). Novel Nanostructured Indium Tin Oxide Electrode for Electrochemical Immunosensors: Suitability for the Detection of TNF-α. Electrochimica Acta 283, 1632–1639. doi:10.1016/j.electacta.2018.07.066
Pruna, R., Palacio, F., Baraket, A., Zine, N., Streklas, A., Bausells, J., et al. (2018b). A Low-Cost and Miniaturized Potentiostat for Sensing of Biomolecular Species Such as TNF-α by Electrochemical Impedance Spectroscopy. Biosens. Bioelectron. 100, 533–540. doi:10.1016/j.bios.2017.09.049
Sadighbayan, D., Sadighbayan, K., Tohid-Kia, M. R., Khosroushahi, A. Y., and Hasanzadeh, M. (2019). Development of Electrochemical Biosensors for Tumor Marker Determination towards Cancer Diagnosis: Recent Progress. Trac Trends Anal. Chem. 118, 73–88. doi:10.1016/j.trac.2019.05.014
Sánchez-Tirado, E., Salvo, C., González-Cortés, A., Yáñez-Sedeño, P., Langa, F., and Pingarrón, J. M. (2017). Electrochemical Immunosensor for Simultaneous Determination of Interleukin-1 Beta and Tumor Necrosis Factor Alpha in Serum and Saliva Using Dual Screen Printed Electrodes Modified with Functionalized Double-Walled Carbon Nanotubes. Analytica Chim. Acta 959, 66–73. doi:10.1016/j.aca.2016.12.034
Sandborn, W. J., Feagan, B. G., Stoinov, S., Honiball, P. J., Rutgeerts, P., Mason, D., et al. (2007). Certolizumab Pegol for the Treatment of Crohn's Disease. N. Engl. J. Med. 357, 228–238. doi:10.1056/NEJMoa067594
Stefan, R. I., van Staden, J., and Aboul-Enein, H. (2000). Design and Use of Electrochemical Sensors in Enantioselective High Throughput Screening of Drugs. A Minireview. Cchts 3, 445–454. doi:10.2174/1386207003331382
Tripoliti, E. E., Karanasiou, G. S., Ioannidou, P., Toumpaniaris, P., Goletsis, Y., Baussels, J., Lomonaco, T., Pfeiffer, N., Anasthase, R., Tsopela, A., Leekens, B., Naka, K. K., Lourme, J.-C., Salvo, P., Liakopoulos, T., Jordan, J., Gallagher, J., Errachid, A., and Fotiadis, D. I. (2018). “KardiaTool: An Integrated POC Solution for Non-invasive Diagnosis and Therapy Monitoring of Heart Failure Patients,” in Annual International Conference of the IEEE Engineering in Medicine and Biology Society, 3878–3881. doi:10.1109/embc.2018.8513298
Tuerk, C., and Gold, L. (1990). Systematic Evolution of Ligands by Exponential Enrichment: RNA Ligands to Bacteriophage T4 DNA Polymerase. Science 249, 505–510. doi:10.1126/science.2200121
Vilian, A. T. E., Kim, W., Park, B., Oh, S. Y., Kim, T., Huh, Y. S., et al. (2019). Efficient Electron-Mediated Electrochemical Biosensor of Gold Wire for the Rapid Detection of C-Reactive Protein: A Predictive Strategy for Heart Failure. Biosens. Bioelectron. 142, 111549. doi:10.1016/j.bios.2019.111549
Vozgirdaite, D., Ben Halima, H., Bellagambi, F. G., Alcacer, A., Palacio, F., Jaffrezic-Renault, N., et al. (2021). Development of an ImmunoFET for Analysis of Tumour Necrosis Factor-α in Artificial Saliva: Application for Heart Failure Monitoring. Chemosensors 9, 26. doi:10.3390/chemosensors9020026
Yáñez-Sedeño, P., Campuzano, S., and Pingarrón, J. M. (2019). Pushing the Limits of Electrochemistry toward Challenging Applications in Clinical Diagnosis, Prognosis, and Therapeutic Action. Chem. Commun. 55, 2563–2592. doi:10.1039/c8cc08815b
Keywords: non-invasive, electrochemical biosensor, TNF-α cytokines, cardiovascular disease, diease biomarker
Citation: Lu Y, Zhou Q and Xu L (2021) Non-Invasive Electrochemical Biosensors for TNF-α Cytokines Detection in Body Fluids. Front. Bioeng. Biotechnol. 9:701045. doi: 10.3389/fbioe.2021.701045
Received: 27 April 2021; Accepted: 10 August 2021;
Published: 21 September 2021.
Edited by:
Masoud Mozafari, University of Toronto, CanadaReviewed by:
Suzanne Hagan, Glasgow Caledonian University, United KingdomSajjad Ashraf, University of Toronto, Canada
Copyright © 2021 Lu, Zhou and Xu. This is an open-access article distributed under the terms of the Creative Commons Attribution License (CC BY). The use, distribution or reproduction in other forums is permitted, provided the original author(s) and the copyright owner(s) are credited and that the original publication in this journal is cited, in accordance with accepted academic practice. No use, distribution or reproduction is permitted which does not comply with these terms.
*Correspondence: Lin Xu, linxu@jlu.edu.cn