- 1Department of Plastic Surgery Medical University of Gdańsk, Gdańsk, Poland
- 2Institute of Molecular and Industrial Biotechnology Lodz, University of Technology, Łódź, Poland
- 3Bowil Ltd, Władysławowo, Poland
- 4Department of Pathology, Medical University of Gdansk, Gdansk, Poland
Although new therapeutic approaches for surgery and wound healing have recently made a great progress, there is still need for application of better and use novel methods to enhance biocompatibility as well as recovery and healing process. Bacterial Cellulose (BC) is natural cellulose in the form of nanostructure which has the advantages of being used in human body. The medical application of BC in reconstructive, cardiac and vascular surgery as well as wound healing is still under development, but without proved success of repetitive results. A review of studies on Bacterial Cellulose (BC) since 2016 was performed, taking into account the latest reports on the clinical use of BC. In addition, data on the physicochemical properties of BC were used. In all the works, satisfactory results of using Bacterial Cellulose were obtained. In all presented studies various BC implants demonstrated their best performance. Additionally, the works show that BC has the capacity to reach physiological as well as mechanical properties of relevance for various tissue replacement and can be produced in surgeons as well as patient specific expectations such as ear frames, vascular tubes or heart valves as well as wound healing dressings. Results of those experiments conform to those of previous reports utilizing ADM (acellular dermal matrix) and demonstrate that the use of BC has no adverse effects such as ulceration or extrusion and possesses expected properties. Based on preliminary animal as well as the few clinical data BC fittings are promising implants for various reconstructive applications since they are biocompatible with properties allowing blood flow, attach easily to wound bed and remain in place until donor site is healed properly. Additionally, this review shows that BC can be fabricated into patient specific shapes and size, with capability to reach mechanical properties of relevance for heart valve, ear, and muscle replacement. Bacterial cellulose appears, as shown in the above review, to be one of the materials that allow extensive application in the reconstruction after soft tissue defects. Review was created to show the needs of surgeons and the possibilities of using BC through the eyes and knowledge of biotechnologists.
1 Introduction
For the last years, the main method of treating diseased or dying soft tissues was to remove them, which significantly compromised the patient’s quality of life. Reconstructive options from the patient’s own tissues are limited, and no materials were widely available to replace the removed tissues, resulting in little opportunity for reconstruction. This situation has improved significantly in recent decades, largely due to the progress of biotechnology and the possibility of using better and compatible materials to replace diseased tissue. The use of biomaterials is nowadays increasingly common both in vitro and in vivo, but there are still many areas of medicine where further research using biomaterials is needed.
Biotechnological developments are increasingly more often used in surgery. This is mainly because the patient’s own tissues do not always allow full functionality and thus do not fulfil that task. An alternative is to obtain the material from a living donor, but this means that patients need to be started on regimens reducing the risk of tissue rejection. Their use is also associated with very long and complicated procedures and thus with very high costs. That is why new materials to replace living tissues are so much sought after. Their use is possible thanks to such properties as bioinertness, adequate mechanical strength, ease of shaping and modification, nanoporosity or hydrophilicity (Pang et al., 2020). Especially natural biopolymers have gained acceptance in medical practice. They show low or no immunogenicity (particularly when based on polysaccharides), and they are favorably perceived by the public, which is also significant. Many in vitro laboratory tests, in vivo tests and clinical trials, have indicated that biomaterials can be an adequate mechanical and functional replacement for the living structures of the patient’s tissues. New bioimplants are increasingly widely used to fill in defects and foster healing and regeneration of the surrounding tissues.
This study focuses on the specific features of one biomaterial, bacterial cellulose (BC). Contrary to its plant equivalent, it is formed as a pure polymer hydrogel without lignin admixtures, which must be removed from the plant cellulose mass. Bacterial cellulose nanofibers are characterized by a high concentration of hydroxyl groups and a surface with densely packed thin fibers. Unlike plant-derived cellulose, whose fibers are much thicker and have a lower hydrogen bond density, BC is more hydrophilic and has a more remarkable ability to form a three-dimensional branched network of ultra-thin fibers. This structure determines many beneficial properties, such as high tensile strength, excellent water holding capacity (98%), which, in turn, spark an interest in bacterial cellulose as a biomedical material of natural origin (Pogorelova et al., 2020).
1.1 The Natural Diversity of BC and Its Potential Applications in Medicine
Bacterial cellulose is atypical biomaterial used in medicine due to its biotechnological origin, most of its features may be controlled by appropriate choice of a producer strain and conditions for propagation. The great majority of examples of BC applications in medicine include the polymer produced by Komagataeibacter as well as Zoogloea genus of bacteria. Cellulose synthesizing bacteria colonize rotting fruits and other parts of plants, hence their presence in the production processes of traditional fermented products (such as Nata de Coco dessert or Kombucha, Kimchi drinks) (Nugroho and Aji, 2015; Villarreal-Soto et al., 2018) in the form known as SCOBY (symbiotic culture of bacteria and yeast). The variety of environments from which BC-producing strains are isolated generates a variety of strains with different living requirements. For example, glucose is the preferred carbon source for most strains, e.g., for K. xylinus E26, K. hansenii 53582, K. hansenii 23770 (Ryngajłło et al., 2019b; Chen et al., 2019), but there are examples of strains that also efficiently grow on sucrose, for instance, K. hansenii 23770 (Chen et al., 2019) or glycerol, such as, e.g., K. xylinus E26 (Ryngajłło et al., 2019b). The presence of ethanol in the culture medium is most often desirable, as is the case for the strains K. xylinus E25 and, e.g., K. xylinus subsp. sucrofermentans BPR3001A, K. rheticus K3 or K. uvaceti FXV3, for which the addition of 2% v/v ethanol to the medium causes up to a twofold increase in the cellulose synthesis efficiency (Naritomi et al., 1998; Krystynowicz et al., 2002; Jacek et al., 2021; Nascimento et al., 2021) but in some cases, it reduces cellulose production, e.g., in the case of the strain K. hansenii 53582 (Jacek et al., 2021). The differences in breeding preferences of the Komagataeibacter strains and their sensitivity to environmental factors result from the genetic diversity of cellulose producing bacteria and are reflected in the properties of the biopolymer they produce (Ryngajłło et al., 2019a; Ryngajłło et al., 2019b). The latter aspect is vital in selecting the appropriate producer strain for the intended use of the material. Resent studies has proven that carbon source used for cellulose propagation has an influence on membrane morphology, namely medium with the highest sucrose content resulted in evident pore size reduction (from 17.832 ± 4.429 µm to 2.698 ± 1.035 µm) and tensile strength increase (from 240.75 ± 14.62 MPa to 339.04 ± 33.82 MPa) in the BC produced by K. xylinus strain TISTR 975 (Jaroennonthasit et al., 2021).
Depending on the strain of the genus Komagataeibacter used in cultivation, the obtained cellulose membranes differ in flexibility, water holding capacity (WHC), cellulose content, degree of polymerization and degree of crystallinity (Table 1). The texture of BC hydrogels is determined both by the network of cellulose fibers and by the water contained in the membrane. It can be observed that the higher the cellulose content or density, the lower the WHC value, and the higher the values of BC breaking stress (Table 1). Cellulose is biosynthesized by cellulose synthase enzyme complexes (terminal complexes, TCs) arranged along the long axis of the cell (Brown et al., 1976; Zaar, 1979; Benziman et al., 1980). Cellulose fibers that build membranes are ribbons coiled from nanofibers drawn from all TCS of a given cell (Benziman et al., 1980). Therefore, most likely, the producer cell morphology translates directly into such properties of cellulose fibers as their diameter or nanofiber packing density. The tendency (or lack thereof) of bacterial cells to move may affect the spatial arrangement of the fibers. In turn, their morphology (diameter, shape and spatial arrangement) affects the mechanical strength of the synthesized material. Machado et al. (2016) observed that the BC synthesized by K. xylinus ATCC 23760 is made of fibers with an average size of about 150 ± 50 nm, while the BC membrane synthesized by K. rhaeticus is made of fibers approx. 100 ± 25 nm in size on average (Machado et al., 2016). Young’s tensile modulus for K. rhaeticus BC was twice as high as that of K. xylinus ATCC 23760 BC (Table 1). As can be seen from the above examples, even slight differences between the cells of producer strains translate into the properties of the material they produce, such as the width and packing density of cellulose fibers (and thus the pore size), which in turn translates into the absorption capacity (water holding capacity, WHC), water retention capacity (WRC) and the mechanical properties of the formed membrane (Chen et al., 2017; Vigentini et al., 2019). Therefore, the knowledge about the diversity of bacterial strains which efficiently synthesize cellulose differing parameters as well as the multiplicity of changes and additional modifications that can be designed for this biopolymer as early as at the stage of bacterial cultivation and post-culture processing allow for extending the application possibilities of this material is evidenced by the emerging new research results in the field of in vivo tests and clinical trials of dressings and implants based on bacterial cellulose.
Native cellulose was first used as a moist dressing for burn and ulcerative wounds (Czaja et al., 2006). However, it soon transpired that its unique compatibility with human tissues opened many more possibilities for its use in biomedicine. As a non-degradable implant, indifferent to the surrounding tissues, cellulose has been used to replace the dura mater, eardrum, valve or interventricular septum. Cellulose biomaterials provide adequate tissue protection in these areas, primarily due to stability, high mechanical strength and flexibility, high hydrophilicity and excellent oxygen permeability (Rosen et al., 2011; Lang et al., 2015; Czaja, 2020; Dawidowska et al., 2020).
Bacterial cellulose reinforced in situ (during cultivation) with a polymer or titanium mesh can be used where higher mechanical strength of the biomaterial is required, in herniotherapy or bone reconstruction procedures (Ludwicka et al., 2018). The structure is also strengthened by the specific arrangement of the fibers (Wang et al., 2017). Cellulose produced by techniques involving a wet-drawing and wet-twisting process of ultralong nanofibers shows significantly greater stiffness, as compared with natural cellulose fibers, regenerated cellulose macrofibers, and novel lightweight steel materials, as well as reduced porosity, both resulting from a highly ordered orientation of fibers.
By controlling cellulose shape during cultivation or adjusting it after cleaning, we extend the scope of its applications to vascular surgery and neurosurgery, including, in particular, tubes for vascular reconstruction or peripheral nerves regeneration (neurotubes) (Kowalska-Ludwicka et al., 2013; Bao et al., 2020). The appropriate nanostructure of the material means an appropriate range of limited (selective) permeability and flexibility adjusted to the requirements applicable to vascular implants and guidance channels (Kołaczkowska et al., 2019).
Compacted (densified) cellulose BC (about 17%) is a competitive scaffolding material for the reconstruction or regeneration of ear cartilage or bone tissue. This kind of material corresponds to the elastic mechanical properties of human cartilage and can be produced in patient-specific auricular shapes, and at the same time shows excellent biocompatibility with chondrocytes and osteocytes in vivo, without interfering with the healing processes (Nimeskern et al., 2013; Martínez Ávila et al., 2015; Pang et al., 2020). Several techniques have been developed to aid cell growth into BC scaffolds, such as adjusting the pore size and pore interconnection during BC (Ashrafi et al., 2019; Zhang et al., 2019), and at the post-production stage—through laser ablation (laser patterning) (Jing et al., 2013), 3D printing (Sultan et al., 2017), or freeze-drying (Martínez Ávila et al., 2015). Particular attention is paid to the methods that increase the microporosity of cellulose without adversely affecting its mechanical and biological parameters, as they frequently constitute the exceptional value of this biopolymer.
The mentioned nanoporosity, hydrogelicity, large surface area and excellent loading capacity of cellulose are successfully used in drug delivery systems (DDS). Such carriers can be used in many fields of pharmacy, medicine and surgery, from dressings to internal or dental implants that deliver painkillers and anti-inflammatory drugs or bacteriostatics to a specific location (Badshah et al., 2017).
The aim of this review is to draw attention to the growing number of applications of bacterial cellulose in regenerative medicine, which have been validated in clinical trials or in vivo experiments. This article also discusses the specific properties of BC to pave a way to increasing its recognition on the market of implants and dressings. The presented examples of BC applications relate only to the most advanced and practically relevant research. The paper indicates the ways of selecting or modifying the properties of BC in terms of specific requirements set by specialists in reconstructive medicine. We have limited the scope of this paper to two areas: reconstructive surgery and wound treatment as the fields with the best-documented applications of BC.
2 Reconstructive Surgery
2.1 Requirements for Implants
Reconstructive surgery is a surgical field whose representatives face the challenge of closing various defects in many body regions. They include extensive, post-traumatic defects of muscles or soft tissues covering them, and minor ones, where they constitute a frame or support. Thanks to the intensive development of technology involving the production of new biomaterials, reconstructive surgery has changed a lot in recent years. New implants are more widely used to fill in defects and foster healing and cell production in the surrounding tissues.
Tissue replacement materials should have the following basic properties:
1. no signs of antigenicity,
2. lack of toxicity,
3. preventing contracture or scarring and provoking the lowest possible inflammatory response,
4. sterility and bacterial barrier or antibacterial properties,
5. appropriate water volume and appropriate mechanical properties, depending on the type of reconstructed tissue,
6. facilitating angiogenesis.
In addition, it is good if they are readily available, packaged and handled, and the production and storage costs are low. It is not easy to implement because many of these features can be mutually exclusive, e.g., mechanical strength with adequate flexibility and maximum reduction of the material thickness. The set of desired features depends on the field of surgery and the properties of the tissues at the site of intervention. The reconstruction of cartilage requires a different set of properties than bone reconstruction. However, BC offers vast possibilities and developing one advantage can be prioritized over another. A different aspect that is very important in cardiac surgery or vascular surgery is the production of long-lasting materials that do not increase the tendency to platelet aggregation and, as a result, thromboembolic complications and are resistant to long-term repetitive work.
2.2 BC as an Implant
A field where biomaterials are already used successfully is reconstructive surgery of the integumentary structures. The potential for a controlled change in the properties of bacterial cellulose depending on the method and conditions of cultivation or post-biosynthetic modification makes it especially suitable (Kołaczkowska et al., 2019) for soft tissue reconstruction. The requirements for the expected properties of each reconstructed tissue are vast and diverse. This is mainly due to the physiological functions of given tissues. The obtained BC material may have properties suitable for an implant of elastic skin and a cartilage scaffold, for instance. There are increasingly more described applications in many areas of reconstructive surgery (Table 2).
2.3 Examples of BC Implant Application in Clinical Practice and In Vivo Experiments
BC has been widely described in many surgical fields. Bacterial nanocellulose patches are excellent biological dressings serving as a temporary skin substitute when applied to exudative and bleeding tissues (Kwak et al., 2015). This is important in wounds with a significant loss of tissue, such as burns or ulcers. Moreover, BC is an excellent scaffold for type I collagen synthesis by mesenchymal stem cells (Vielreicher et al., 2018). A detailed analysis with collagen I-specific binding protein revealed a highly ordered collagen network structure at the interface between the cell and bacterial nanocellulose. This shows the reproductive capacity of soft tissues thanks to BC. The potential of bacterial nanocellulose in integumentary reconstruction was also assessed in vivo (Jankau et al., Unpublished study). The study was conducted on three pigs. The same defects were produced in the ear cartilage and the rectus abdominis muscle, and BC membranes were sutured into them. After 3 months, the morphology, physical parameters and functions of BC were assessed compared to the live body tissues. Histopathological examinations included the degree of resorption, pus exudation, fibrosis and scar formation (Figures 1–4). The implantation sites did not show clinical signs of complications such as inflammation or necrosis. Histologically, a normal scar was produced due to the material healing into the host’s body. These preliminary studies confirmed the usefulness of BC as an effective filling of cartilage and muscle tissue defects.
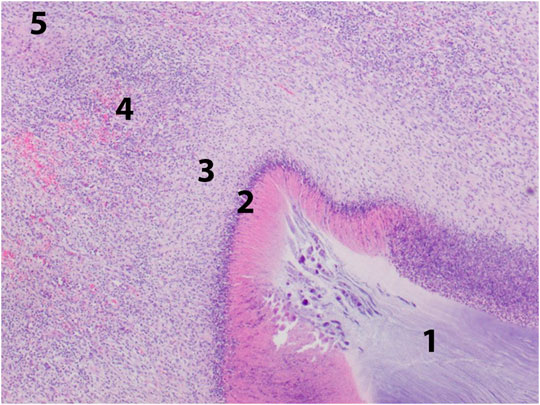
FIGURE 1. Body integument - resorptive reaction to an implant. Zone 1: implant, zone 2: purulent necrosis, zone 3: macrophagal infiltration, zone 4: granulation tissue, zone 5: fibrous scar tissue.
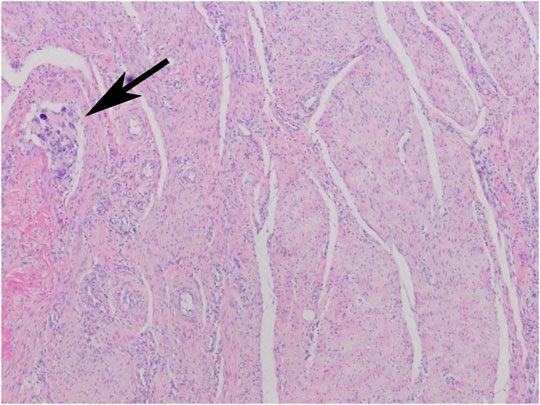
FIGURE 2. Body integument. Fibrous scar tissue formed by thick collagen-fibroblastic bundles. Focal resorptive granuloma (arrow).
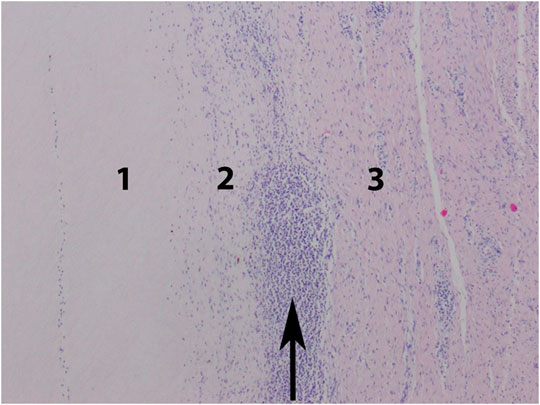
FIGURE 3. Auricle. Resorptive reaction to the implant. Zone 1: implant, zone 2: macrophagal infiltration, zone 3: fibrous scar tissue. Focal nodular cluster of lymphocytes (arrow).
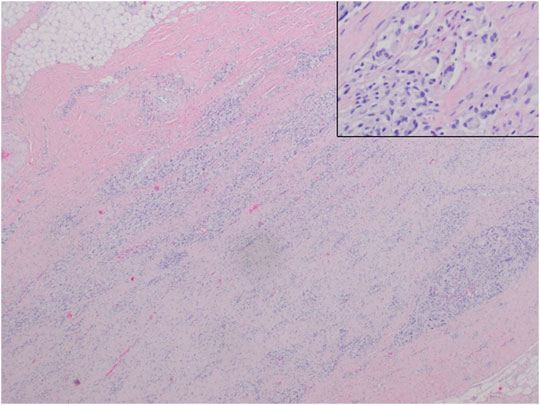
FIGURE 4. Auricle. Interstitial granules (insert) are visible between the thick bundles of fibrous scar tissue.
BC can be used as a cartilage implant. Until now, the gold standard of ear cartilage reconstruction was the use of autologous rib grafts, which significantly extended the operation time, increased the surface area of the patient’s injured tissue and was associated with a higher risk of complications. The development of tissue engineering is a promising alternative therapy for this approach. BC has also been reported as a material for the reconstruction of vestibular cartilage. Excellent biocompatibility and tissue integration were demonstrated (Martínez Ávila et al., 2015). BC scaffolds provide good mechanical stability and maintain structural integrity while providing a porous architecture that supports chondrocyte development.
Another area where bacterial nanocellulose is used as a reconstructive material is ophthalmology. Corneal infection or improper treatment can have serious consequences, such as vision deterioration, vision loss, and deteriorated quality of life. A method for treating such injuries has been developed. It involves using biomaterials as a dressing for the damaged cornea (Anton-Sales et al., 2021). The use of an amniotic membrane graft was compared to the use of bacterial nanocellulose. BC was shown to be effective in covering corneal defects. Moreover, unlike the amniotic membrane, BC is stable at room temperature, and its production is much easier—the size, shape and thickness are much easier to control. In addition, BC can be easily sterilized by heat. Its use is tissue-independent and carries no risk of disease transmission as it is a product of plant origin.
Bacterial cellulose can also be used in neurosurgery. A frequent complication of head injuries, neurosurgical procedures, congenital defects or neoplastic diseases is disruption or damage of the dura mater. The primary function of the dura mater is to protect the central nervous system and provide an appropriate environment; therefore, disrupting its integrity is a significant problem in neurosurgery. The material that can be replaced must be biocompatible and cannot cause inflammation because the potential activation of inflammatory mediators, including nitric oxide or prostaglandins, may lead to a cascade of inflammatory processes and, as a result, to inflammatory diseases of the central nervous system (CNS), which are associated with a high risk of long-term complications. Until now, silk fibroin, human amniotic membrane and other materials have been researched as substitutes for dura mater. The BC has the advantage of being cost-effective and straightforward to produce. Several papers describe the repair of dural damage with BC membranes (Goldschmidt et al., 2016). They indicate a great potential of BC for use as a new type of artificial dura mater material due to its high strength and very good biocompatibility. The commercial production of the material is already planned (Bacakova et al., 2019; Lipovka et al., 2021).
Congenital heart defects are among the most common defects and the most common cause of death in children under 1 year. Ventricular septal defect accounts for the highest percentage of congenital heart defects (20%–29%). It is usually closed with a synthetic patch (PTFE), an autologous or bovine pericardium patch, but long-term results do not give satisfactory results. Currently available materials are not sufficiently flexible, which may weaken the diastolic compliance of the heart in the long term. Therefore, a biomaterial is sought to combine biocompatibility, adequate flexibility, tensile strength and flexibility similar to heart tissue. The network of cellulose nanofibrils shows similarities to the collagen network, thanks to this biomaterial has high mechanical strength, favorable flexibility and.
Water content up to 99%. A study was conducted on 25 pigs (German landrace pigs) (Lang et al., 2015), in which the ventricular septal defect was closed with BC sheets. Immediate occlusion was observed in all cases, and no tendency for platelet aggregation was detected. The biocompatibility of BC was good in the presence of blood, and the tissue responses were comparable to the materials currently used. Wound healing was observed to be normal, with complete neoendotheliation, progression over time with cellular organization, and mild chronic inflammatory reactions. The use of BC in vascular surgery or cardiac surgery is currently being explored by several research centers worldwide. Chemically pure BC free of pyrogenic agents is produced by Bowil Biotech Sp. z o.o., Władysławowo, Poland, and used in animal models as part of the “Kardio-BC” study (Kołaczkowska et al., 2019). Large animals such as pigs are often used in preliminary biocompatibility studies of new materials developed for use in medicine, including vascular and cardiac surgery. They were used in studies involving the implantation of reconstructive pericardial patches, thoracic aortic prostheses or both to determine BC biocompatibility (Kowalik et al., 2020). Individual operations were performed sequentially, depending on the increasing surgical complexity: pericardial patches, thoracic aortic prostheses, and a combination of both. After 90 days of observation, the animals were euthanized, and tissue fragments containing BC implants were collected at necropsy and subjected to histopathological examination. The assessment of bioimplant properties included macro and microscopic evaluation, scanning electron microscopy (SEM), X-ray diffraction examination, histopathological evaluation of the inflammatory reaction of the surrounding tissues. These studies provided evidence for the usefulness of BC as a vascular prosthesis in an animal model. The results indicate adequate healing of the prostheses, low percentage of mechanical damage, no infiltration of the host cells inside bacterial cellulose bioimplant, coverage of the bioimplant surface by the native vascular endothelium. The material itself did not degenerate. In addition, it was more durable than natural tissues such as porcine pericardial sac, aortic valve cusps or aortic wall.
Similar in vascular surgery, a material imitated blood vessels (creates thin “tubes”) that will no affected blood clotting) has been searched for years. Just as the use of two dimensional (2D) biomaterials has already bee relatively thoroughly studied, three dimensional (3D) BC is still unknown topic. Some 3D BNC biomaterials have been reported in the literature, but rather there are theoretical pages about properties (Klemm et al., 2021). There is described the in vivo use of BC by Osorio et al., evaluating the effect of using BC as blood vessels and their haemolysis and thrombogenicity (contact coagulation) (Osorio et al., 2019). The presented research results, it can be concluded that bacterial cellulose can be safely and effectively used as a vascular prosthesis and heart valve prosthesis after appropriate preparation.
3. BC Application in Healing Wounds
The healing process of all kinds of injuries and wounds, both internal and external, is the result of many coordinated molecular mechanisms, and their correct regulation is crucial for the duration and results of treatment. The risk of bacterial or fungal infection and an incorrectly progressing inflammatory process may also affect the outcome. If the healing process is normal, haemostasis (bleeding) is followed by an inflammatory reaction lasting from 24 h up to 6 days. The immune system cells must release cytokines and proteolytic enzymes to cleanse the wound of damaged tissue debris and prevent secondary infection by producing reactive oxygen species (ROS) (Thomason et al., 2018). Topical application of antibiotics and anti-fungal substances are therefore essential for treatment success. In addition, natural signaling molecules involved in healing processes, such as cytokines, components of the extracellular matrix (ECM) or tissue-specific growth factors, are now used as active substances in modern dressing and regeneration materials (Catanzano et al., 2021; Farahani and Shafiee, 2021). What is particularly challenging in dressing materials is the treatment of chronic and hard-to-heal wounds in which the balance between matrix metalloproteinases (MMPs) necessary for cleaning damaged tissue and their inhibitors (tissue inhibitors of metalloproteinitors, TIMPs) is disturbed (Grzela et al., 2016; Dissemond et al., 2020; Tort et al., 2020).
3.1 Requirements for Dressings
Natural polymeric materials that have been tested so far as wound dressings include agar, sodium alginate, hyaluronic acid, chitin, chitosan, carrageenan, cellulose, pectin, starch, and collagen. The variety of forms of dressings includes bandages, hydrogels, foil, sponges, foams, nanofiber mats (Zheng et al., 2020). Conventional dry dressings such as absorbent gauze and/or absorbent cotton dressings have a limited therapeutic effect and require multiple dressing changes, adversely affecting the patient’s experience (Stoica et al., 2020).
One of the main external factors responsible for optimal wound healing is hydration. Creating a moist wound environment through the use of ointments or hydrogels results in an improvement and acceleration of healing, a reduction in pain and a significant reduction in scarring compared to conventional wound treatment (Zhang et al., 2020). Moist wound healing optimally supports and facilitates the cleansing of the wound by the body, creating a clean wound environment. In the case of fibrin and necrotic tissues, they are softened and removed. Exudate, dead tissue debris and bacteria are absorbed through the dressing.
Besides additional active substances, a good modern dressing should support the wound healing process, which an ideal matrix/carrier in the dressing material should ensure (Bielecki et al., 2012; Zheng et al., 2020):
1. appropriate humidity—(favorable WHC to WRC ratio)
2. absorption of excess exudate (favorable WHC to WRC ratio)
3. maintaining an appropriate exchange of gaseous water vapor and gases (appropriate porosity)
4. not causing allergic reactions
5. not triggering the body’s immune response (inflammation in the initial stage of healing is desirable to stimulate the mechanisms of proliferation, i.e., rebuilding damaged tissues, but its prolongation may lead to the formation of a chronic wound)
6. biocompatibility
7. protecting the wound against bacterial infections (also as a physical barrier)
8. adequate thermal insulation (thickness of the dressing)
9. easy application and removal from the wound (adequate flexibility and mechanical strength)
10. painless dressing change (proper adhesion to the cells of regenerating tissues)
11. removal of necrotic cells (proper adherence to the wound, appropriate flexibility, size, adaptability to anatomical shapes)
12. observation of the healing process (desired transparency)
3.2 BC as a Dressing
Native bacterial cellulose can absorb wound exudate while providing good moisture of the wound bed and gas exchange with the environment. Since the 1990s, when this biomaterial was first used in medicine, much evidence has been accumulated to confirm that natural hydrogel created by bacterial cellulose supports wound healing (Czaja et al., 2004). A particular advantage of this material is that it is easy to obtain large sheets to cover the whole body and to adapt to the contours of the target area. In addition, due to its mechanical properties, this material also supports the skin tissue regeneration stage due to proper adhesion and support for cell proliferation and differentiation, and protection against mechanical injuries (Vilar et al., 2016; Emre Oz et al., 2021).
Native BC may constitute a physical barrier to secondary wound infections but has no bactericidal or bacteriostatic properties. To achieve this feature of the dressing, the BC membrane can be soaked with antibiotics (or other biocides) or subjected to further modification leading to the formation of a composite, e.g., with silver nanoparticles (Wu et al., 2014; Wen et al., 2015). The following section presents examples of the applications of BC as a dressing validated in in vivo experiments or clinical trials (Table 3).
3.3 Examples of BC Dressings in Clinical Applications and In Vivo Experiments
Pioneering clinical trials of bacterial cellulose dressing were conducted at the Burn Wound Treatment Center in Siemianowice Śląskie, Poland (Czaja et all. 2020). They aimed to assess the suitability of the dressing in the treatment of burn wounds in the following areas: prevention of water loss through a burn wound, reduction of burn wound infection, preparing the wound for a skin graft. The study group consisted of patients with I, IIA, IIB and III degree skin burns covering 9%–18% of the total body surface. There were 77 study participants aged 18–70. The study results indicate that BC dressings are particularly effective for exuding wounds and wounds with elevated temperature. Due to the high water content, they cool the burn site and relieve pain. In the case of fresh and shallow burn wounds, BC dressing accelerates the epithelialization process, reducing the risk of infection and fluid loss. However, in the case of deep burns (IIB and III degree), it supports the removal of necrotic tissues from the wound. Faster healing was manifested by earlier change in gram-negative to gram-positive species abundance ratio as well. Thanks to proteolytic enzymes, gram-negative microflora causes faster wound cleansing, preparing for further treatment with a free skin graft (Czaja et al., 2004). According to clinical data, due to their flexibility, bacterial cellulose dressings are particularly recommended for the treatment of burn wounds in anatomically complex places (ears, face, joint area, hands, feet, genitals) (Oliveira et al., 2020). It has also been shown that combining the dressing with antiseptics, such as silver nitrate, hydroxyquinoline sulphate or boric acid, has a positive effect on reducing burn wound infection (Czaja et al., 2004). Clinical trials were also conducted by Jankau et al. using BC (Bowil Biotech Ltd., Władysławowo, Poland) as a dressing in a patient with thermal burns, achieving a very good clinical result (Figures 5, 6).
An example of a new application of bacterial cellulose dressings is their use in treating wounds in the urogenital area in men in the postoperative period. Phase 2 clinical trials showed that no adverse effects were observed in the case of applying a BC dressing, no disadvantages such as ischemia, infection and pain were identified, confirming the safety of the BC dressing and its effectiveness as a highly satisfactory alternative to the currently used dressings (Vilar et al., 2016).
A well-documented example of a BC composite studied in vivo in the process of wound healing is BC supplemented with vaccarin (BC-Vac), which performance has been compared to standard dressings, i.e., petrolatum gauze and a dressing with silver nanoparticles (Table 3). The most important advantages of the composite included increased flexibility, which facilitates its practical use (changing the dressing, adherence to the wound), and most importantly, a more advanced healing process (more active fibroblasts) was confirmed after 14 days of treatment (Qiu et al., 2016).
Rayon dressings are often used to treat chronic leg ulcers. When compared to rayon dressings for wound care, BC dressings have been shown (Silva et al., 2021) to be superior due to lower dressing change frequency, minimized direct contact with wounds, less risk of contamination and infection, greater patient autonomy and less dressing adhesion to bed (Silva et al., 2021).
Relieving inflammation and neuropathy in diabetic foot ulcers has been a great challenge for physicians for many years. Significant acceleration of the healing of diabetic foot ulcer wounds was achieved while using sheets of bacterial cellulose nanofibers soaked with venlafaxine (VEN) and doxycycline (DOX) as compared to the control group (Meamar et al., 2021). The patients showed no differences in their biochemical parameters before and after the intervention.
4 Prospects and Challenges for BC as a “Tailor-Made” Biomaterial for the Needs of Medicine
The native properties of BC modulated as in the discussed above in situ and ex situ modifications make it an attractive material for modern dressings and implants. However, constant progress in reconstructive medicine requires the development of more advanced, dedicated solutions. This section shows the emerging possibilities and pathways for further dedicated modifications of BC properties.
4.1 Molecular Intervention in BC Properties
Progress in functional genomics and transcriptomics of bacteria of the genus Komagataeibacter (Ryngajłło et al., 2019a, 2019b, 2020; Jang et al., 2019; La China et al., 2020) suggests that in the near future, the understanding of the relationship between the genotype of the producer strain and the properties of the polymer produced by its cells will reach a level that allows for the planned editing of the genome depending on the requirements set for materials in a specific application. The first published results of the properties of BC membranes produced by producer strain mutants confirm this thesis.
A correlation was observed between the thickness of the fibers and the mechanical properties of the membranes of the K. obediens R 37-4 and R37-9 mutants—the thinner the fibers, the higher the tensile strength (Taweecheep et al., 2019). BC synthesized by these mutants showed two times higher density and tensile strength than BC synthesized by the parent strain. The two obtained mutants, R 37-4 and R37-9, were also more efficient at synthesizing cellulose membranes (Table 3).
The data collected by our team indicate a correlation between changes in cell morphology and the diameter of cellulose fibers in the final material (Jacek et al., 2019a; 2019b, 2021). In the studies of the membrane structure using the SEM technique, thinner fibers (on average 45 nm in diameter in the lower part of the membrane) were observed in the case of shorter cells, such as those of the K. xylinus E25 strain (approx. 3.0 µm) (Jacek et al., 2021) or K. hansenii 23769 (approx. 2 µm) (Jacek et al., 2018) and widened (an average of 90 nm diameter in the lower part of the membrane) for longer cells in strains such as K. hansenii ATCC 5358 (5.2 µm) (Jacek et al., 2019a). In addition, studies have shown that by influencing the length of cells and their motility, it is possible to obtain a material with changed fiber dimensions and fiber arrangement in mutants of all the strains mentioned above within the motA and motB genes (Jacek et al., 2018; 2019a). The motA and motB genes have no proven molecular function in Komagataeibacter cells but are sequentially similar to the genes encoding the proton pumps necessary to generate the pmf (proton motive force) that drives flagella in E. coli (Hosking et al., 2006; Kim et al., 2008) and their homologues with proven function, e.g., in gliding in Myxococcus (Nan et al., 2013; Fu et al., 2018). Their presence is necessary to maintain motility in the strain K. hansenii ATCC 53582, as shown by microscopic observations and soft-agar motility assay for motAB deletion mutants (Jacek et al., 2019a). Moreover, these deletion mutants are characterized by a compact structure of thin fibers (average diameter of 40 nm compared to 90 nm on average for the wild type strain). As a consequence of the changes in the fiber architecture visible in the SEM examination, a change in mechanical properties was also noted, especially clearly in the case of Young’s Modulus for compressive tests of the wet membranes: it was ∼333 MPa for the disruptive strain, compared to ∼194 MPa for the wild type strain (Table 4) (Jacek et al., 2019a). Quite to the contrary, the motAB+ overexpression mutants obtained in the strain K. hansenii ATCC 23769 have elongated cells or occur in chains and produce cellulose material with a looser arrangement of fibers (Jacek et al., 2018). This kind of material was demonstrated to be useful in tissue engineering for the generation of scaffolds—the favorable difference in favor of BC derived from the mutant strain compared to the wild one was noticeable even at the level of the response of ATDC5 cells seeded into the scaffold (glycosaminoglycan secretion through Alcian blue staining) (Jacek et al., 2018).
4.2 BC as a Drug Delivery System—Potential and Challenges
Among the many listed medical applications of bacterial cellulose, its use in developing drug delivery systems (DDS) is the most diverse application field. Considering the different types of drug administration to the patient’s body (including dermal, transdermal and oral administration) and the different types of conditions managed with such treatments, a large number of published studies focus on an attempt to create an effective BC-based system, enabling the effective delivery of selected drugs to a wound or diseased tissue (Ciolacu et al., 2020). Such systems involve carriers based on whole fragments of membranes and its composites, cellulose beads formed in shake cultures, as well as cellulose fibers, which are used for creating hybrids for drug immobilization (Shi et al., 2014; Shao et al., 2016; Bayazidi et al., 2018; Cacicedo et al., 2018; Blanco et al., 2021). The latest trends in this area are oriented towards developing controlled systems targeting specific areas undergoing treatment and stimulating specific factors to reduce the adverse effects of the drug on healthy tissues. It is also expected that the carriers and their degradation products will be harmless to the body, able to accommodate the amount of the drug necessary to achieve the expected treatment outcomes and that the release time of such drugs will be adjustable to the duration of treatment, be it hours or even days (Ciolacu et al., 2020). In this field, bacterial cellulose has considerable potential as it is a highly hydrophilic biopolymer, which is relatively easy to modify and create various types of composites and hybrids, and above all, shows high biocompatibility. Nevertheless, published studies report on various challenges that still face scientists who explore BC-based DDS. First of all, cellulose sheets are not easy to handle in forming standard and well-known drug carriers, such as liposomes, conjugates or polyplexes, for instance. In most published studies, a membrane is used as a carrier and most often as a dressing, so the drug is delivered by soaking, which limits the possibility of controlled, delayed release (Silva et al., 2014; Cacicedo et al., 2020). Due to the compact nanostructure resulting from the dense cross-linking of the fibers, the incorporation of larger particles into the membrane is also challenging. The vast majority of research into BC carriers concerns combining cellulose (most often a dressing) with small molecules, such as analgesics and anti-inflammatory agents (e.g., diclofenac, ibuprofen, venlafaxine and doxycycline), bacteriostats such as metal ions (TiO2, ZnO2, Ag, etc.), antibiotics (amoxicillin, ciprofloxacin, gentamicin, etc.), or other chemical compounds (e.g., glycerol, glutaraldehyde, ε-poly-l-Lysine, nisin, ocetnidine, rutin) (Silva et al., 2014; Shao et al., 2016; Anton- Sales et al., 2019; Gorgieva and Trček, 2019; Jiji et al., 2020; Blanco et al., 2021; Emre Oz et al., 2021; Fonseca et al., 2021; Meamar et al., 2021). Larger molecules such as proteins (e.g., albumin, lysozyme, lipase or phospholipase) and growth factors should be adequately immobilized in the membrane after modifying the porosity of the cellulose nanostructure (Wu et al., 2017; Drozd et al., 2019; Wang et al., 2020). For such immobilization, carriers made of bacterial cellulose nanofibers (BCNF) are often used when porosity can be adjusted at the stage of carrier development or when the immobilization takes place during its development (Bayazidi et al., 2018). Such techniques find particular application in the development of cellulose dressings and implants, fostering angiogenesis and epithelialization. Promising solutions include cellulose carriers that stimulate blood vessel formation with the help of, e.g., vascular endothelial growth factor (VEGF) or interleukin 4 (IL-4), as well as dressings and implants supporting healing by inhibiting pro-inflammatory factors such as matrix metalloproteinases (Ai et al., 2020; Li et al., 2020; Wang et al., 2020). Stopping the expression of genes coding selected MMPs with the use of siRNA molecules encapsulated in bacterial cellulose and its hybrids, will allow for avoiding long-term treatment or hard-to-heal wounds and reducing inflammation in the case of surgical interventions. Moreover, this type of gene therapy will reduce the adverse effects of drugs on other tissues.
The most advanced, although still relatively few targeted therapies that use bacterial cellulose are applicable to cancer treatment, where the active substance is usually doxorubicin, (Cacicedo et al., 2018; Ando et al., 2021; Islam et al., 2021). They use cellulose nanofibers (BCNF) or hybrids of BC hydrogel with nanostructured lipid carriers for immobilization to efficiently and effectively release the drug at the target area. In this field, the challenge is in functionalizing cellulose in such a way as to make it an actual release system. Hence, new, non-aggressive methods of increasing cellulose reactivity (e.g., enzymatic oxidation) or the development of composites, e.g., with magnetic, thermo- and photosensitive properties, (Ivanova et al., 2020; Kim et al., 2020; Troncoso and Torres, 2020; Poddar and Dikshit, 2021; Sriplai and Pinitsoontorn, 2021). This direction of research may lead to developing a carrier that will be non-toxic, functional and adapted to the state-of-the-art treatment methods.
5 Conclusion
The Human body in large is composed of soft tissues. They play an important role in maintaining the human body with structure and function. Repairing or replacing them is now an important part of reconstructive surgery. For this purpose, many organic or inorganic polymers are used from which the structures that make up the human body are built or rebuilt. One of these polymers is biocellulose, produced by Komagataeibacter batteries.
Searching the bibliographic databases: Scholar, Embase or Europe PMC from the last 5 years using the tool “LinkSource” and typing the keywords: bacterial cellulose, biocellulose, bionanocellulose, BNC or nanocellulose in combination with the keyword reconstructive surgery, we get over 62, 452 results. In the PubMed online database, typing in the same keywords, only 2,843 papers appear, and narrowing the search to humans, only 27 papers appear. Even if we assume that some of them are duplicated, it shows the importance of the problem. Thus, the potential of using bacterial cellulose in soft tissue reconstruction, from reconstruction to aesthetics, is unlimited. An interesting and often misunderstood term in the context of cellulose is the acronym BNC, in medicine and surgery it stands for bladder neck contracture. We excluded this from our search. Scientific papers describing clinical use of CC in animals or humans, however, are sparde, indicating how much this topic is not researched.
Possibilities of using BC in modern and reconstructive surgery are enormous. As Table 2 shows, many surgical disciplines have the chances to benefit from the potential of this natural polymer. It is a desirable, versatile material thanks to its various shape, hydratation, flexibility, hardness . Its use requires research, especially phase III studies. Unfortunately, even the models of pigs are not a proper equivalent of the metabolism of the human body, but even these are few. This is particularly when we mention about implanted parts into animal or human body.
The purpose of this review is to highlight the challenge for surgeons and biotechnologists to produce a cellulosic material that will meet the versatile needs of soft tissue restoration as well as use as inserts in various forms (e.g., skeletons, frames or structures, tubes, and even valves) in the human body. Such material is yet to be developed, as shown in the above article, bacterial cellulose has a chance to come close to the ideal. In the above review, the authors combined the knowledge of surgeons and biotechnologists to present the expectations and possibilities of microbial cellulose, believing it will be possible to personalize it and combine it with other substances to induce the growth of regenerated tissues and be “indestructible.” Therefore, further research activities need to be conducted to fill current gaps from laboratory in vitro and in vivo research to tests in humans and put in commercial production, so that unlimited cellulose inserts will replace missing soft tissues, both in their form and function. Above all authors believe that patient directed “bacterial cellulose” will certainly improve their quality of life. The authors also hope that further research and production capabilities will allow for quick and inexpensive production, including 3D printing, of unlimited cellulose inserts to replace soft tissues, both in their function and form. This, however, requires close cooperation between the interested parties and the patients.
Author Contributions
JJ, AB-S, KK, contributed to conception and design of the study. TP, MJ-K, KL, AD organized the data based. JJ, AB-S, KK wrote the first draft of the manuscript. MJ-K, KL, TP wrote the section of tables. JJ, AD did the figures. RP read and explained pathology slides. All authors contributed to manuscript revision, read, and approved the submitted version.
Conflict of Interest
Author AD is employed by Bowil Ltd.
The remaining authors declare that the research was conducted in the absence of any commercial or financial relationships that could be construed as a potential conflict of interest.
Publisher’s Note
All claims expressed in this article are solely those of the authors and do not necessarily represent those of their affiliated organizations, or those of the publisher, the editors and the reviewers. Any product that may be evaluated in this article, or claim that may be made by its manufacturer, is not guaranteed or endorsed by the publisher.
References
Ai, F.-F., Mao, M., Zhang, Y., Kang, J., and Zhu, L. (2020). Experimental Study of a New Original Mesh Developed for Pelvic Floor Reconstructive Surgery. Int. Urogynecol. J. 31, 79–89. doi:10.1007/s00192-019-03947-4
Ando, H., Mochizuki, T., Lila, A. S. A., Akagi, S., Tajima, K., Fujita, K., et al. (2021). Doxorubicin Embedded into Nanofibrillated Bacterial Cellulose (NFBC) Produces a Promising Therapeutic Outcome for Peritoneally Metastatic Gastric Cancer in Mice Models via Intraperitoneal Direct Injection. Nanomaterials 11, 1697. doi:10.3390/nano11071697
Anton-Sales, I., Beekmann, U., Laromaine, A., Roig, A., and Kralisch, D. (2019). Opportunities of Bacterial Cellulose to Treat Epithelial Tissues. Cdt 20, 808–822. doi:10.2174/1389450120666181129092144
Anton-Sales, I., Roig-Sanchez, S., Traeger, K., Weis, C., Laromaine, A., Turon, P., et al. (2021). In Vivo soft Tissue Reinforcement with Bacterial Nanocellulose. Biomater. Sci. 9, 3040–3050. doi:10.1039/d1bm00025j
Ashrafi, Z., Lucia, L., and Krause, W. (2019). Bioengineering Tunable Porosity in Bacterial Nanocellulose Matrices. Soft Matter 15, 9359–9367. doi:10.1039/c9sm01895f
Autier, L., Clavreul, A., Cacicedo, M. L., Franconi, F., Sindji, L., Rousseau, A., et al. (2019). A New Glioblastoma Cell Trap for Implantation after Surgical Resection. Acta Biomater. 84, 268–279. doi:10.1016/j.actbio.2018.11.027
Aydinli, S., Bişkin, S., Dinç, A. E., Uçal, Y. O., Kandemir, N., and Tekinözel, I. (2017). Bacterial Cellulose as a New Graft Model for the Turkish Delight Technique in Rhinoplasty: An experiment in 20 Rats. Ear, Nose Throat J. 96. Available at: https://pubmed.ncbi.nlm.nih.gov/28931193/(Accessed October 21, 2021).
Bacakova, L., Pajorova, J., Bacakova, M., Skogberg, A., Kallio, P., Kolarova, K., et al. (2019). Versatile Application of Nanocellulose: from Industry to Skin Tissue Engineering and Wound Healing. Nanomaterials 9 (2), 164. doi:10.3390/nano9020164
Badshah, M., Ullah, H., Khan, S. A., Park, J. K., and Khan, T. (2017). Preparation, Characterization and In-Vitro Evaluation of Bacterial Cellulose Matrices for Oral Drug Delivery. Cellulose 24, 5041–5052. doi:10.1007/s10570-017-1474-8
Bao, L., Hong, F. F., Li, G., Hu, G., and Chen, L. (2021). Implantation of Air-Dried Bacterial Nanocellulose Conduits in a Small-Caliber Vascular Prosthesis Rabbit Model. Mater. Sci. Eng. C 122, 111922. doi:10.1016/j.msec.2021.111922
Bao, L., Tang, J., Hong, F. F., Lu, X., and Chen, L. (2020). Physicochemical Properties and In Vitro Biocompatibility of Three Bacterial Nanocellulose Conduits for Blood Vessel Applications. Carbohydr. Polym. 239, 116246. doi:10.1016/j.carbpol.2020.116246
Bayazidi, P., Almasi, H., and Asl, A. K. (2018). Immobilization of Lysozyme on Bacterial Cellulose Nanofibers: Characteristics, Antimicrobial Activity and Morphological Properties. Int. J. Biol. Macromolecules 107, 2544–2551. doi:10.1016/j.ijbiomac.2017.10.137
Benziman, M., Haigler, C. H., Brown, R. M., White, A. R., and Cooper, K. M. (1980). Cellulose Biogenesis: Polymerization and Crystallization Are Coupled Processes in Acetobacter Xylinum. Proc. Natl. Acad. Sci. 77, 6678–6682. doi:10.1073/pnas.77.11.6678
Bielecki, S., Kalinowska, H., Krystynowicz, A., Kubiak, K., Kołodziejczyk, M., and de Groeve, M. (2016). Wound Dressings and Cosmetic Materials from Bacterial Nanocellulose. Bact. Nanocellulose, 157–174. doi:10.1201/b12936-9
Binnetoglu, A., Demir, B., Akakin, D., Kervancioglu Demirci, E., and Batman, C. (2020). Bacterial Cellulose Tubes as a Nerve Conduit for Repairing Complete Facial Nerve Transection in a Rat Model. Eur. Arch. Otorhinolaryngol. 277, 277–283. doi:10.1007/s00405-019-05637-9
Blanco, F. G., Hernández, N., Rivero-Buceta, V., Maestro, B., Sanz, J. M., Mato, A., et al. (2021). From Residues to Added-Value Bacterial Biopolymers as Nanomaterials for Biomedical Applications. Nanomaterials 11, 1492. doi:10.3390/nano11061492
Brown, R. M., Willison, J. H., and Richardson, C. L. (1976). Cellulose Biosynthesis in Acetobacter Xylinum: Visualization of the Site of Synthesis and Direct Measurement of the In Vivo Process. Proc. Natl. Acad. Sci. 73, 4565–4569. doi:10.1073/pnas.73.12.4565
Cacicedo, M. L., Islan, G. A., León, I. E., Álvarez, V. A., Chourpa, I., Allard-Vannier, E., et al. (2018). Bacterial Cellulose Hydrogel Loaded with Lipid Nanoparticles for Localized Cancer Treatment. Colloids Surf. B: Biointerfaces 170, 596–608. doi:10.1016/j.colsurfb.2018.06.056
Cacicedo, M. L., Pacheco, G., Islan, G. A., Alvarez, V. A., Barud, H. S., and Castro, G. R. (2020). Chitosan-bacterial Cellulose Patch of Ciprofloxacin for Wound Dressing: Preparation and Characterization Studies. Int. J. Biol. Macromolecules 147, 1136–1145. doi:10.1016/j.ijbiomac.2019.10.082
Catanzano, O., Quaglia, F., and Boateng, J. S. (2021). Wound Dressings as Growth Factor Delivery Platforms for Chronic Wound Healing. Expert Opin. Drug Deliv. 18, 737–759. doi:10.1080/17425247.2021.1867096
Cavalcante, A. R. T., Lima, R. P. d., Souza, V. S. B. d., Pinto, F. C. M., Campos Júnior, O., Silva, J. G. M. d., et al. (2018). Effects of Bacterial Cellulose Gel on the Anorectal Resting Pressures in Rats Submitted to Anal Sphincter Injury. Heliyon 4, e01058. doi:10.1016/j.heliyon.2018.e01058
Cavalcanti, L. M., Pinto, F. C. M., Oliveira, G. M. D., Lima, S. V. C., Aguiar, J. L. D. A., and Lins, E. M. (2017). Efficacy of Bacterial Cellulose Membrane for the Treatment of Lower Limbs Chronic Varicose Ulcers: a Randomized and Controlled Trial. Rev. Col. Bras. Cir. 44, 72–80. doi:10.1590/0100-69912017001011
Chen, G., Wu, G., Chen, L., Wang, W., Hong, F. F., and Jönsson, L. J. (2019). Comparison of Productivity and Quality of Bacterial Nanocellulose Synthesized Using Culture media Based on Seven Sugars from Biomass. Microb. Biotechnol. 12, 677–687. doi:10.1111/1751-7915.13401
Chen, S.-Q., Lopez-Sanchez, P., Wang, D., Mikkelsen, D., and Gidley, M. J. (2018). Mechanical Properties of Bacterial Cellulose Synthesised by Diverse Strains of the Genus Komagataeibacter. Food Hydrocolloids 81, 87–95. doi:10.1016/j.foodhyd.2018.02.031
Chen, S.-Q., Mikkelsen, D., Lopez-Sanchez, P., Wang, D., Martinez-Sanz, M., Gilbert, E. P., et al. (2017). Characterisation of Bacterial Cellulose from Diverse Komagataeibacter Strains and Their Application to Construct Plant Cell wall Analogues. Cellulose 24, 1211–1226. doi:10.1007/s10570-017-1203-3
Ciolacu, D. E., Nicu, R., and Ciolacu, F. (2020). Cellulose-based Hydrogels as Sustained Drug-Delivery Systems. Materials 1, 1–37. doi:10.3390/ma13225270
Coelho Junior, E. R., Costa, L. O. B. F., Alencar, A. V., Barbosa, A. P. G., Pinto, F. C. M., and Aguiar, J. L. d. A. (2015). Prevention of Peritoneal Adhesion Using a Bacterial Cellulose Hydrogel, in Experimental Study. Acta Cir. Bras. 30, 194–198. doi:10.1590/S0102-865020150030000005
Czaja, W., Kawecki, M., Krystynowicz, A., Wysota, K., Sakiel, S., Wroblewski, P., et al. (2004). CELL 157 - Application of Bacterial Cellulose in Treatment of Second and Third Degree burns. 227th ACS Natl. Meet. Anaheim, CA. Available at: http://oasys2.confex.com/acs/227nm/techprogram/P716781.HTM (Accessed October 11, 2021).
Czaja, W., Krystynowicz, A., Bielecki, S., and Brownjr, R. (2006). Microbial Cellulose-The Natural Power to Heal Wounds. Biomaterials 27, 145–151. doi:10.1016/j.biomaterials.2005.07.035
Czaja, W., Krystynowicz, A., Kawecki, M., Wysota, K., Sakiel, S., Wróblewski, P., et al. (2020). Biomedical Applications of Microbial Cellulose in Burn Wound Recovery. Chapter 17.
Czaja, W. (2020). SYNTHECEL ® Dura Repair — Biosynthesized Cellulose for Dura Repair Bionanocellulose.
Dawidowska, K., Siondalski, P., and Kołaczkowska, M. (2020). In Vitro Study of a Stentless Aortic Bioprosthesis Made of Bacterial Cellulose. Cardiovasc. Eng. Tech. 11, 646–654. doi:10.1007/s13239-020-00500-z
de Abreu, G. F., Batista, L. L., Adeodato, D. C., de Albuquerque, A. V., Ferraz-Carvalho, R. S., de Lima, R. P., et al. (2020). Use of Bacterial Cellulose Film for Repair of Bile Duct Injury in Pigs. J. Biomater. Appl. 35, 331–341. doi:10.1177/0885328220928221
Demir, B., Sarı, M., Binnetoglu, A., Yumusakhuylu, A. C., Filinte, D., Tekin, İ. Ö., et al. (2018). Comparison of Pharyngocutaneous Fistula Closure with and without Bacterial Cellulose in a Rat Model. Auris Nasus Larynx 45, 301–305. doi:10.1016/j.anl.2017.04.005
Dissemond, J., Augustin, M., Dietlein, M., Faust, U., Keuthage, W., Lobmann, R., et al. (2020). Efficacy of MMP-Inhibiting Wound Dressings in the Treatment of Chronic Wounds: a Systematic Review. J. Wound Care 29, 102–118. doi:10.12968/jowc.2020.29.2.102
Drozd, R., Szymańska, M., Rakoczy, R., Junka, A., Szymczyk, P., and Fijałkowski, K. (2019). Functionalized Magnetic Bacterial Cellulose Beads as Carrier for Lecitase Ultra Immobilization. Appl. Biochem. Biotechnol. 187, 176–193. doi:10.1007/s12010-018-2816-1
Emre Oz, Y., Keskin-Erdogan, Z., Safa, N., and Esin Hames Tuna, E. (2021). A Review of Functionalised Bacterial Cellulose for Targeted Biomedical fields. J. Biomater. Appl. 36, 648–681. doi:10.1177/0885328221998033
Farahani, M., and Shafiee, A. (2021). Wound Healing: From Passive to Smart Dressings. Adv. Healthc. Mater. 10, 2100477. doi:10.1002/adhm.202100477
Fonseca, D. F. S., Vilela, C., Pinto, R. J. B., Bastos, V., Oliveira, H., Catarino, J., et al. (2021). Bacterial Nanocellulose-Hyaluronic Acid Microneedle Patches for Skin Applications: In Vitro and In Vivo Evaluation. Mater. Sci. Eng. C 118, 111350. doi:10.1016/j.msec.2020.111350
Fu, G., Bandaria, J. N., Le Gall, A. V., Fan, X., Yildiz, A., Mignot, T., et al. (2018). MotAB-like Machinery Drives the Movement of MreB Filaments during Bacterial Gliding Motility. Proc. Natl. Acad. Sci. USA 115, 2484–2489. doi:10.1073/pnas.1716441115
Goldschmidt, E., Cacicedo, M., Kornfeld, S., Valinoti, M., Ielpi, M., Ajler, P. M., et al. (20162016). Construction Andin Vitrotesting of a Cellulose Dura Mater Graft. Neurol. Res. 38 (1), 25–31. doi:10.1080/01616412.2015.1122263
Gorgieva, S., and Trček, J. (2019). Bacterial Cellulose: Production, Modification and Perspectives in Biomedical Applications. Nanomaterials 9, 1352. doi:10.3390/nano9101352
Grzela, T., Krejner, A., and Litwiniuk, M. (2016). Matrix Metalloproteinases in the Wound Microenvironment: Therapeutic Perspectives. Cwcmr 3, 29. doi:10.2147/CWCMR.S73819
Hosking, E. R., Vogt, C., Bakker, E. P., and Manson, M. D. (2006). The Escherichia coli MotAB Proton Channel Unplugged. J. Mol. Biol. 364, 921–937. doi:10.1016/j.jmb.2006.09.035
Islam, S. U., Ul-Islam, M., Ahsan, H., Ahmed, M. B., Shehzad, A., Fatima, A., et al. (2021). Potential Applications of Bacterial Cellulose and its Composites for Cancer Treatment. Int. J. Biol. Macromolecules 168, 301–309. doi:10.1016/j.ijbiomac.2020.12.042
Ivanova, L. A., Ustinovich, K. B., Khamova, T. V., Eneyskaya, E. V., Gorshkova, Y. E., Tsvigun, N. V., et al. (2020). Crystal and Supramolecular Structure of Bacterial Cellulose Hydrolyzed by Cellobiohydrolase from Scytalidium Candidum 3C: A Basis for Development of Biodegradable Wound Dressings. Materials 13, 2087. doi:10.3390/ma13092087
Jacek, P., Kubiak, K., Ryngajłło, M., Rytczak, P., Paluch, P., and Bielecki, S. (2019a). Modification of Bacterial Nanocellulose Properties through Mutation of Motility Related Genes in Komagataeibacter Hansenii ATCC 53582. New Biotechnol. 52, 60–68. doi:10.1016/j.nbt.2019.05.004
Jacek, P., Ryngajłło, M., and Bielecki, S. (2019b). Structural Changes of Bacterial Nanocellulose Pellicles Induced by Genetic Modification of Komagataeibacter Hansenii ATCC 23769. Appl. Microbiol. Biotechnol. 103, 5339–5353. doi:10.1007/s00253-019-09846-4
Jacek, P., Silva, F. A. G. S. d., Dourado, F., Bielecki, S., and Gama, M. (2021). Optimization and Characterization of Bacterial Nanocellulose Produced by Komagataeibacter Rhaeticus K3. Carbohydr. Polym. Tech. Appl. 2, 100022. doi:10.1016/j.carpta.2020.100022
Jacek, P., Szustak, M., Kubiak, K., Gendaszewska-Darmach, E., Ludwicka, K., and Bielecki, S. (2018). Scaffolds for Chondrogenic Cells Cultivation Prepared from Bacterial Cellulose with Relaxed Fibers Structure Induced Genetically. Nanomaterials 8, 1066. doi:10.3390/nano8121066
Jang, W. D., Kim, T. Y., Kim, H. U., Shim, W. Y., Ryu, J. Y., Park, J. H., et al. (2019). Genomic and Metabolic Analysis ofKomagataeibacter xylinusDSM 2325 Producing Bacterial Cellulose Nanofiber. Biotechnol. Bioeng. 116, 3372–3381. doi:10.1002/bit.27150
Jaroennonthasit, W., Lam, N. T., and Sukyai, P. (2021). Evaluation of Carbon Sources from Sugar Industry to Bacterial Nanocellulose Produced by Komagataeibacter Xylinus. Int. J. Biol. Macromolecules 191, 299–304. doi:10.1016/j.ijbiomac.2021.09.028
Jiji, S., Udhayakumar, S., Maharajan, K., Rose, C., Muralidharan, C., and Kadirvelu, K. (2020). Bacterial Cellulose Matrix with In Situ Impregnation of Silver Nanoparticles via Catecholic Redox Chemistry for Third Degree Burn Wound Healing. Carbohydr. Polym. 245, 116573. doi:10.1016/j.carbpol.2020.116573
Jing, W., Chunxi, Y., Yizao, W., Honglin, L., Fang, H., Kerong, D., et al. (2013). Laser Patterning of Bacterial Cellulose Hydrogel and its Modification with Gelatin and Hydroxyapatite for Bone Tissue Engineering. Soft Mater. 11, 173–180. doi:10.1080/1539445X.2011.611204
Jing, Y., Ma, X., Xu, C., Tian, H.-l., and Chenwen, S.-w. (2020). Repair of Dural Defects with Electrospun Bacterial Cellulose Membranes in a Rabbit Experimental Model. Mater. Sci. Eng. C 117, 111246. doi:10.1016/j.msec.2020.111246
Kim, E. A., Price-Carter, M., Carlquist, W. C., and Blair, D. F. (2008). Membrane Segment Organization in the Stator Complex of the Flagellar Motor: Implications for Proton Flow and Proton-Induced Conformational Change. Biochemistry 47, 11332–11339. doi:10.1021/bi801347a
Kim, H., Eun Song, J., Silva, C., and Kim, H. R. (2020). Production of Conductive Bacterial Cellulose-Polyaniline Membranes in the Presence of Metal Salts. Textile Res. J. 90, 1517–1526. doi:10.1177/0040517519893717
Klemm, D., Petzold-Welcke, K., Kramer, F., Richter, T., Raddatz, V., Fried, W., et al. (2021). Biotech Nanocellulose: A Review on Progress in Product Design and Today's State of Technical and Medical Applications. Carbohydr. Polym. 254 (2021), 117313. doi:10.1016/j.carbpol.2020.117313
Kołaczkowska, M., Siondalski, P., Kowalik, M. M., Pęksa, R., Długa, A., Zając, W., et al. (2019). Assessment of the Usefulness of Bacterial Cellulose Produced by Gluconacetobacter Xylinus E25 as a New Biological Implant. Mater. Sci. Eng. C 97, 302–312. doi:10.1016/j.msec.2018.12.016
Kowalik, M. M., Siondalski, P., Kołaczkowska, M., Zając, W., Pałczyńska, P., Cackowska, E., et al. (2020). Challenges in Using Anesthesia for Open Chest and Aorta Surgery in Swine. Medycyna Weterynaryjna 76, 6426–2020. doi:10.21521/mw.6426
Kowalska-Ludwicka, K., Cala, J., Grobelski, B., Sygut, D., Jesionek-Kupnicka, D., Kolodziejczyk, M., et al. (2013). - New Methods Modified Bacterial Cellulose Tubes for Regeneration of Damaged Peripheral Nerves. aoms 3, 527–534. doi:10.5114/aoms.2013.33433
Krystynowicz, A., Czaja, W., Wiktorowska-Jezierska, A., Gonalves-Mikiewicz, M., Turkiewicz, M., and Bielecki, S. (2002). Factors Affecting the Yield and Properties of Bacterial Cellulose. J. Ind. Microbiol. Biotechnol. 29, 189–195. doi:10.1038/sj.jim.7000303
Kwak, M. H., Kim, J. E., Go, J., Koh, E. K., Song, S. H., Son, H. J., et al. (2015). Bacterial Cellulose Membrane Produced by Acetobacter Sp. A10 for Burn Wound Dressing Applications. Carbohydr. Polym. 122, 387–398. doi:10.1016/j.carbpol.2014.10.049
La China, S., Bezzecchi, A., Moya, F., Petroni, G., Di Gregorio, S., and Gullo, M. (2020). Genome Sequencing and Phylogenetic Analysis of K1G4: a New Komagataeibacter Strain Producing Bacterial Cellulose from Different Carbon Sources. Biotechnol. Lett. 42, 807–818. doi:10.1007/s10529-020-02811-6
Lang, N., Merkel, E., Fuchs, F., Schumann, D., Klemm, D., Kramer, F., et al. (2015). Bacterial Nanocellulose as a New Patch Material for Closure of Ventricular Septal Defects in a Pig Model. Eur. J. Cardio-thoracic Surg. 47, 1013–1021. doi:10.1093/ejcts/ezu292
Li, N., Yang, L., Pan, C., Saw, P. E., Ren, M., Lan, B., et al. (2020). Naturally-occurring Bacterial Cellulose-Hyperbranched Cationic Polysaccharide derivative/MMP-9 siRNA Composite Dressing for Wound Healing Enhancement in Diabetic Rats. Acta Biomater. 102, 298–314. doi:10.1016/j.actbio.2019.11.005
Lima, F. d. M. T. d., Pinto, F. C. M., Andrade-da-Costa, B. L. d. S., Silva, J. G. M. d., Campos Júnior, O., and Aguiar, J. L. d. A. (2017a). Biocompatible Bacterial Cellulose Membrane in Dural Defect Repair of Rat. J. Mater. Sci. Mater. Med. 28, 37. doi:10.1007/s10856-016-5828-9
Lima, S. V. C., Machado, M. R., Pinto, F. C. M., Lira, M. M. d. M., Albuquerque, A. V. d., Lustosa, E. S., et al. (2017b). A New Material to Prevent Urethral Damage after Implantation of Artificial Devices: an Experimental Study. Int. Braz. J Urol. 43, 335–344. doi:10.1590/S1677-5538.IBJU.2016.0271
Lipkova, J., Chen, T. Y., Lu, M. Y., Shady, M., Williams, M., Wang, J., et al. (2021). International Evaluation of Weakly-Supervised Ai-Model for Cardiac Allograft Rejection Screening. J. Am. Coll. Cardiol. 77 (18Suppl. 1), 520. doi:10.1016/S0735-1097(21)01879-9
Ludwicka, K., Kolodziejczyk, M., Gendaszewska-Darmach, E., Chrzanowski, M., Jedrzejczak- Krzepkowska, M., Rytczak, P., et al. (2018). Stable Composite of Bacterial Nanocellulose and Perforated Polypropylene Mesh for Biomedical Applications. J. Biomed. Mater. Res. Part. B Appl. Biomater. in press. doi:10.1002/jbm.b.34191
Machado, R. T. A., Gutierrez, J., Tercjak, A., Trovatti, E., Uahib, F. G. M., Moreno, G. d. P., et al. (2016). Komagataeibacter Rhaeticus as an Alternative Bacteria for Cellulose Production. Carbohydr. Polym. 152, 841–849. doi:10.1016/j.carbpol.2016.06.049
Maia, A. L., Lins, E. M., Aguiar, J. L. A., Pinto, F. C. M., Rocha, F. A., Batista, L. L., et al. (2019). Curativo com filme e gel de biopolímero de celulose bacteriana no tratamento de feridas isquêmicas após revascularização de membros inferiores. Rev. Col. Bras. Cir. 46. doi:10.1590/0100-6991e-20192260
Maia, G. T. d. S., Albuquerque, A. V. d., Martins Filho, E. D., Lira Neto, F. T. d., Souza, V. S. B. d., Silva, A. A. d., et al. (2018). Bacterial Cellulose to Reinforce Urethrovesical Anastomosis. A Translational Study. Acta Cir. Bras. 33, 673–683. doi:10.1590/s0102-865020180080000003
Mandour, Y. M. H., Mohammed, S., and Menem, M. o. A. (2019). Bacterial Cellulose Graft versus Fat Graft in Closure of Tympanic Membrane Perforation. Am. J. Otolaryngol. 40, 168–172. doi:10.1016/j.amjoto.2018.12.008
Martínez Ávila, H., Feldmann, E.-M., Pleumeekers, M. M., Nimeskern, L., Kuo, W., de Jong, W. C., et al. (2015). Novel Bilayer Bacterial Nanocellulose Scaffold Supports Neocartilage Formation In Vitro and In Vivo. Biomaterials 44, 122–133. doi:10.1016/j.biomaterials.2014.12.025
Meamar, R., Chegini, S., Varshosaz, J., Aminorroaya, A., Amini, M., and Siavosh, M. (2021). Alleviating Neuropathy of Diabetic Foot Ulcer by Co-delivery of Venlafaxine and Matrix Metalloproteinase Drug-Loaded Cellulose Nanofiber Sheets: Production, In Vitro Characterization and Clinical Trial. Pharmacol. Rep. 73, 806–819. doi:10.1007/s43440-021-00220-8
Nan, B., Bandaria, J. N., Moghtaderi, A., Sun, I.-H., Yildiz, A., and Zusman, D. R. (2013). Flagella Stator Homologs Function as Motors for Myxobacterial Gliding Motility by Moving in Helical Trajectories. Proc. Natl. Acad. Sci. 110, E1508–E1513. doi:10.1073/pnas.1219982110
Naritomi, T., Kouda, T., Yano, H., and Yoshinaga, F. (1998). Effect of Ethanol on Bacterial Cellulose Production from Fructose in Continuous Culture. J. Ferment. Bioeng. 85, 598–603. doi:10.1016/S0922-338X(98)80012-3
Nascimento, F. X., Torres, C. A. V., Freitas, F., Reis, M. A. M., and Crespo, M. T. B. (2021). Functional and Genomic Characterization of Komagataeibacter Uvaceti FXV3, a Multiple Stress Resistant Bacterium Producing Increased Levels of Cellulose. Biotechnol. Rep. 30, e00606. doi:10.1016/j.btre.2021.e00606
Nimeskern, L., Martínez Ávila, H., Sundberg, J., Gatenholm, P., Müller, R., and Stok, K. S. (2013). Mechanical Evaluation of Bacterial Nanocellulose as an Implant Material for Ear Cartilage Replacement. J. Mech. Behav. Biomed. Mater. 22, 12–21. doi:10.1016/j.jmbbm.2013.03.005
Nugroho, D. A., and Aji, P. (2015). Characterization of Nata de Coco Produced by Fermentation of Immobilized Acetobacter xylinum. Agric. Agric. Sci. Proced. 3, 278–282. doi:10.1016/j.aaspro.2015.01.053
Oliveira, M. H., Pinto, F. C. M., Ferraz-Carvalho, R. S., Albuquerque, A. V., and Aguiar, J. L. (2020). BIO-NAIL: a Bacterial Cellulose Dressing as a New Alternative to Preserve the Nail Bed after Avulsion. J. Mater. Sci. Mater. Med. 31. doi:10.1007/s10856-020-06456-9
Osorio, M., Cañas, A., Puerta, J., Díaz, L., Naranjo, T., Ortiz, I., et al. (20192019). Ex Vivo and In Vivo Biocompatibility Assessment (Blood and Tissue) of Three-Dimensional Bacterial Nanocellulose Biomaterials for Soft Tissue Implants. Sci. Rep. 9, 10553. doi:10.1038/s41598-019-46918-x
Pandey, M., Mohamad, N., Low, W.-L., Martin, C., and Mohd Amin, M. C. I. (2017). Microwaved Bacterial Cellulose-Based Hydrogel Microparticles for the Healing of Partial Thickness Burn Wounds. Drug Deliv. Transl. Res. 7, 89–99. doi:10.1007/s13346-016-0341-8
Pang, M., Huang, Y., Meng, F., Zhuang, Y., Liu, H., Du, M., et al. (2020). Application of Bacterial Cellulose in Skin and Bone Tissue Engineering. Eur. Polym. J. 122, 109365. doi:10.1016/j.eurpolymj.2019.109365
Piasecka-Zelga, J., Zelga, P., Szulc, J., Wietecha, J., and Ciechańska, D. (2018). An In Vivo Biocompatibility Study of Surgical Meshes Made from Bacterial Cellulose Modified with Chitosan. Int. J. Biol. Macromolecules 116, 1119–1127. doi:10.1016/j.ijbiomac.2018.05.123
Poddar, M. K., and Dikshit, P. K. (2021). Recent Development in Bacterial Cellulose Production and Synthesis of Cellulose Based Conductive Polymer Nanocomposites. Nano Select 2, 1605–1628. doi:10.1002/nano.202100044
Pogorelova, N., Rogachev, E., Digel, I., Chernigova, S., and Nardin, D. (2020). Bacterial Cellulose Nanocomposites: Morphology and Mechanical Properties. Materials 13, 2849. doi:10.3390/ma13122849
Qiu, Y., Qiu, L., Cui, J., and Wei, Q. (2016). Bacterial Cellulose and Bacterial Cellulose-Vaccarin Membranes for Wound Healing. Mater. Sci. Eng. C 59, 303–309. doi:10.1016/j.msec.2015.10.016
Rosen, C. L., Steinberg, G. K., DeMonte, F., Delashaw, J. B., Lewis, S. B., Shaffrey, M. E., et al. (2011). Results of the Prospective, Randomized, Multicenter Clinical Trial Evaluating a Biosynthesized Cellulose Graft for Repair of Dural Defects. Neurosurgery 69, 1093–1104. doi:10.1227/NEU.0b013e3182284aca
Ryngajłło, M., Jacek, P., Cielecka, I., Kalinowska, H., and Bielecki, S. (2019a). Effect of Ethanol Supplementation on the Transcriptional Landscape of Bionanocellulose Producer Komagataeibacter Xylinus E25. Appl. Microbiol. Biotechnol. 103, 6673–6688. doi:10.1007/s00253-019-09904-x
Ryngajłło, M., Jędrzejczak-Krzepkowska, M., Kubiak, K., Ludwicka, K., and Bielecki, S. (2020). Towards Control of Cellulose Biosynthesis by Komagataeibacter Using Systems-Level and Strain Engineering Strategies: Current Progress and Perspectives. Appl. Microbiol. Biotechnol. 104, 6565–6585. doi:10.1007/s00253-020-10671-3
Ryngajłło, M., Kubiak, K., Jędrzejczak‐Krzepkowska, M., Jacek, P., and Bielecki, S. (2019b). Comparative Genomics of theKomagataeibacterstrains-Efficient Bionanocellulose Producers. Microbiologyopen 8, e00731. doi:10.1002/mbo3.731
Shao, W., Liu, H., Wang, S., Wu, J., Huang, M., Min, H., et al. (2016). Controlled Release and Antibacterial Activity of Tetracycline Hydrochloride-Loaded Bacterial Cellulose Composite Membranes. Carbohydr. Polym. 145, 114–120. doi:10.1016/j.carbpol.2016.02.065
Shi, X., Zheng, Y., Wang, G., Lin, Q., and Fan, J. (2014). PH- and Electro-Response Characteristics of Bacterial Cellulose Nanofiber/sodium Alginate Hybrid Hydrogels for Dual Controlled Drug Delivery. RSC Adv. 4, 47056–47065. doi:10.1039/c4ra09640a
Silva, L. G., Albuquerque, A. V., Pinto, F. C. M., Ferraz-Carvalho, R. S., Aguiar, J. L. A., and Lins, E. M. (2021). Bacterial Cellulose an Effective Material in the Treatment of Chronic Venous Ulcers of the Lower Limbs. J. Mater. Sci. Mater. Med. 32, 1–10. doi:10.1007/s10856-021-06539-1
Silva, N. H. C. S., Rodrigues, A. F., Almeida, I. F., Costa, P. C., Rosado, C., Neto, C. P., et al. (2014). Bacterial Cellulose Membranes as Transdermal Delivery Systems for Diclofenac: In Vitro Dissolution and Permeation Studies. Carbohydr. Polym. 106, 264–269. doi:10.1016/j.carbpol.2014.02.014
Silveira, F. C. A., Pinto, F. C. M., Caldas Neto, S. d. S., Leal, M. d. C., Cesário, J., and Aguiar, J. L. d. A. (2016a). Treatment of Tympanic Membrane Perforation Using Bacterial Cellulose: A Randomized Controlled Trial. Braz. J. Otorhinolaryngol. 82, 203–208. doi:10.1016/j.bjorl.2015.03.015
Silveira, R. K., Coelho, A. R. B., Pinto, F. C. M., de Albuquerque, A. V., de Melo Filho, D. A., and de Andrade Aguiar, J. L. (2016b). Bioprosthetic Mesh of Bacterial Cellulose for Treatment of Abdominal Muscle Aponeurotic Defect in Rat Model. J. Mater. Sci. Mater. Med. 27, 1–9. doi:10.1007/s10856-016-5744-z
Sriplai, N., and Pinitsoontorn, S. (2021). Bacterial Cellulose-Based Magnetic Nanocomposites: A Review. Carbohydr. Polym. 254, 117228. doi:10.1016/j.carbpol.2020.117228
Stoica, A. E., Chircov, C., and Grumezescu, A. M. (2020). Hydrogel Dressings for the Treatment of Burn Wounds: An Up-To-Date Overview. Materials 13, 1–24. doi:10.3390/ma13122853
Sultan, S., Siqueira, G., Zimmermann, T., and Mathew, A. P. (2017). 3D Printing of Nano-Cellulosic Biomaterials for Medical Applications. Curr. Opin. Biomed. Eng. 2, 29–34. doi:10.1016/j.cobme.2017.06.002
Taweecheep, P., Naloka, K., Matsutani, M., Yakushi, T., Matsushita, K., and Theeragool, G. (2019). Superfine Bacterial Nanocellulose Produced by Reverse Mutations in the bcsC Gene during Adaptive Breeding of Komagataeibacter Oboediens. Carbohydr. Polym. 226, 115243. doi:10.1016/j.carbpol.2019.115243
Thomason, H. A., Lovett, J. M., Spina, C. J., Stephenson, C., McBain, A. J., and Hardman, M. J. (2018). Silver Oxysalts Promote Cutaneous Wound Healing Independent of Infection. Wound Rep. Reg. 26, 144–152. doi:10.1111/wrr.12627
Tort, S., Demiröz, F. T., Coşkun Cevher, Ş., Sarıbaş, S., Özoğul, C., and Acartürk, F. (2020). The Effect of a New Wound Dressing on Wound Healing: Biochemical and Histopathological Evaluation. Burns 46, 143–155. doi:10.1016/j.burns.2019.02.013
Troncoso, O. P., and Torres, F. G. (2020). Bacterial Cellulose-Graphene Based Nanocomposites. Ijms 21, 1–17. doi:10.3390/ijms21186532
Vielreicher, M., Kralisch, D., Völkl, S., Sternal, F., Arkudas, A., and Friedrich, O. (2018). Bacterial Nanocellulose Stimulates Mesenchymal Stem Cell Expansion and Formation of Stable Collagen-I Networks as a Novel Biomaterial in Tissue Engineering. Sci. Rep. 8, 9401–9414. doi:10.1038/s41598-018-27760-z
Vigentini, I., Fabrizio, V., Dellacà, F., Rossi, S., Azario, I., Mondin, C., et al. (2019). Set-Up of Bacterial Cellulose Production from the Genus Komagataeibacter and its Use in a Gluten-free Bakery Product as a Case Study. Front. Microbiol. 10, 1953. doi:10.3389/fmicb.2019.01953
Vilar, F. d. O., Pinto, F. C. M., Albuquerque, A. V., Martins, A. G. S., Araújo, L. A. P. d., Aguiar, J. L. d. A., et al. (2016). A Wet Dressing for Male Genital Surgery: A Phase II Clinical Trial. Int. Braz. J Urol. 42, 1220–1227. doi:10.1590/s1677-5538.ibju.2016.0109
Villarreal-Soto, S. A., Beaufort, S., Bouajila, J., Souchard, J.-P., and Taillandier, P. (2018). Understanding Kombucha Tea Fermentation: A Review. J. Food Sci. 83, 580–588. doi:10.1111/1750-3841.14068
Volova, T. G., Shumilova, A. A., Nikolaeva, E. D., Kirichenko, A. K., and Shishatskaya, E. I. (2019). Biotechnological Wound Dressings Based on Bacterial Cellulose and Degradable Copolymer P(3HB/4HB). Int. J. Biol. Macromolecules 131, 230–240. doi:10.1016/j.ijbiomac.2019.03.068
Wacker, M., Kießwetter, V., Slottosch, I., Awad, G., Paunel-Görgülü, A., Varghese, S., et al. (2020). In vitro hemo- and cytocompatibility of bacterial nanocelluose small diameter vascular grafts: Impact of fabrication and surface characteristics. PLoS One 15 (6), e0235168. doi:10.1371/journal.pone.0235168
Wang, B., Lv, X., Li, Z., Zhang, M., Yao, J., Sheng, N., et al. (2020). Urethra-inspired Biomimetic Scaffold: A Therapeutic Strategy to Promote Angiogenesis for Urethral Regeneration in a Rabbit Model. Acta Biomater. 102, 247–258. doi:10.1016/j.actbio.2019.11.026
Wang, S., Jiang, F., Xu, X., Kuang, Y., Fu, K., Hitz, E., et al. (2017). Super-Strong, Super-stiff Macrofibers with Aligned, Long Bacterial Cellulose Nanofibers. Adv. Mater. 29, 1702498. doi:10.1002/adma.201702498
Weber, C., Reinhardt, S., Eghbalzadeh, K., Wacker, M., Guschlbauer, M., Maul, A., et al. (2018). Patency and In Vivo Compatibility of Bacterial Nanocellulose Grafts as Small-Diameter Vascular Substitute. J. Vasc. Surg. 68, 177S–187S. doi:10.1016/j.jvs.2017.09.038
Wen, X., Zheng, Y., Wu, J., Yue, L., Wang, C., Luan, J., et al. (2015). In Vitro and In Vivo Investigation of Bacterial Cellulose Dressing Containing Uniform Silver Sulfadiazine Nanoparticles for Burn Wound Healing. Prog. Nat. Sci. Mater. Int. 25, 197–203. doi:10.1016/j.pnsc.2015.05.004
Wu, J., Zheng, Y., Wen, X., Lin, Q., Chen, X., and Wu, Z. (2014). Silver Nanoparticle/bacterial Cellulose Gel Membranes for Antibacterial Wound Dressing: Investigation In Vitro and In Vivo. Biomed. Mater. 9, 035005. doi:10.1088/1748-6041/9/3/035005
Wu, S.-C., Wu, S.-M., and Su, F.-M. (2017). Novel Process for Immobilizing an Enzyme on a Bacterial Cellulose Membrane through Repeated Absorption. J. Chem. Technol. Biotechnol. 92, 109–114. doi:10.1002/jctb.4994
Yang, J., Wang, L., Zhang, W., Sun, Z., Li, Y., Yang, M., et al. (2018). Reverse Reconstruction and Bioprinting of Bacterial Cellulose-Based Functional Total Intervertebral Disc for Therapeutic Implantation. Small 14, 1702582. doi:10.1002/smll.201702582
Zaar, K. (1979). Visualization of Pores (export Sites) Correlated with Cellulose Production in the Envelope of the Gram-Negative Bacterium Acetobacter Xylinum. J. Cel Biol. 80, 773–777. doi:10.1083/jcb.80.3.773
Zhang, H., Xu, X., Chen, C., Chen, X., Huang, Y., and Sun, D. (2019). In Situ controllable Fabrication of Porous Bacterial Cellulose. Mater. Lett. 249, 104–107. doi:10.1016/j.matlet.2019.04.026
Zhang, L., de Salvo, R., Trapp, S., Wigger-Alberti, W., Williams, R., Delcour, L., et al. (2020). Evaluation of BepanGel Hydrogel Efficacy and Tolerability Using an Abrasive Wound Model in a Within-Person, Single-Center, Randomized, Investigator-Blind Clinical Investigation. Dermatol. Ther. (Heidelb) 10, 1075–1088. doi:10.1007/s13555-020-00432-5
Keywords: bacterial cellulose (BC), reconstructive surgery, wound dressing, mechanical properties, biocompatibility and biodegradability
Citation: Jankau J, Błażyńska‐Spychalska A, Kubiak K, Jędrzejczak-Krzepkowska M, Pankiewicz T, Ludwicka K, Dettlaff A and Pęksa R (2022) Bacterial Cellulose Properties Fulfilling Requirements for a Biomaterial of Choice in Reconstructive Surgery and Wound Healing. Front. Bioeng. Biotechnol. 9:805053. doi: 10.3389/fbioe.2021.805053
Received: 29 October 2021; Accepted: 28 December 2021;
Published: 11 February 2022.
Edited by:
Selestina Gorgieva, University of Maribor, SloveniaReviewed by:
Helena P. Felgueiras, University of Minho, PortugalJorge Padrão, University of Minho, Portugal
Karol Fijałkowski, West Pomeranian University of Technology, Poland
Copyright © 2022 Jankau, Błażyńska‐Spychalska, Kubiak, Jedrzejczak Krzepkowska, Pankiewicz, Ludwicka, Pęksa and Dettlaff. This is an open-access article distributed under the terms of the Creative Commons Attribution License (CC BY). The use, distribution or reproduction in other forums is permitted, provided the original author(s) and the copyright owner(s) are credited and that the original publication in this journal is cited, in accordance with accepted academic practice. No use, distribution or reproduction is permitted which does not comply with these terms.
*Correspondence: Jerzy Jankau, amphbmthdUBndW1lZC5lZHUucGw=