- 1Department of Hematology and Oncology, Shenzhen Children’s Hospital, Shenzhen, Guangdong, China
- 2Shenzhen Children’s Hospital, China Medical University, Shenzhen, Guangdong, China
Hematopoietic stem cells (HSCs) provide a life-long supply of haemopoietic cells and are indispensable for clinical transplantation in the treatment of malignant hematological diseases. Clinical applications require vast quantities of HSCs with maintained stemness characteristics. Meeting this demand poses often insurmountable challenges for traditional culture methods. Creating a supportive artificial microenvironment for the culture of HSCs, which allows the expansion of the cells while maintaining their stemness, is becoming a new solution for the provision of these rare multipotent HSCs. Hydrogels with good biocompatibility, excellent hydrophilicity, tunable biochemical and biophysical properties have been applied in mimicking the hematopoietic niche for the efficient expansion of HSCs. This review focuses on recent progress in the use of hydrogels in this specialized application. Advanced biomimetic strategies use for the creation of an artificial haemopoietic niche are discussed, advances in combined use of hydrogel matrices and microfluidics, including the emerging organ-on-a-chip technology, are summarized. We also provide a brief description of novel stimulus-responsive hydrogels that are used to establish an intelligent dynamic cell microenvironment. Finally, current challenges and future perspectives of engineering hydrogels for HSC biomedicine are explored.
1 Introduction
Hematopoietic stem cells (HSCs) are self-renewing pluripotent stem cells. HSCs can differentiate into all types of blood cell in the body (Wilkinson et al., 2020). In the clinical treatment of hematopoietic diseases, transplantation of HSCs from healthy donors into patients can reconstitute hematopoiesis and the immune system in recipients (Chatterjee et al., 2021). There is a considerable demand for HSCs in clinical transplantation, gene therapy, and basic stem cell research (Wilkinson and Nakauchi, 2020). However, the lack of readily accessible HSC sources poses a barrier to the more widespread clinical and research use of these cells (Liu et al., 2022). The in vitro expansion of primitive pluripotent HSC populations promises to represent an effective solution to overcome this shortage (Liu et al., 2022).
HSCs used in transplantation are mainly derived from umbilical cord blood. These cells can be used with a relatively low risk of immune rejection, while the number of cells that can be recovered is limited (Ballen et al., 2013; Cohen et al., 2020).
In-depth studies regarding the molecular mechanism of self-renewal and differentiation in HSCs have explored various methods for HSCs expansion ex vivo, for example, supplementing hematopoietic stimulating factors, the addition of small molecule compounds into the suspension culture medium, and co-culture of HSCs and their supporting cells to maintain cell growth enhance the cellular connections (Zimran et al., 2021). However, it is difficult to are difficult to replicate cell–cell and cell–extracellular matrix (ECM) interactions that occur in the natural niche of the bone marrow (BM) using conventional two-dimensional (2D) culture methods (Langhans, 2018; Liu et al., 2022). To achieve a better in vitro hematopoietic microenvironment, research has focused on creating three-dimensional (3D) culture conditions in vitro (Huang et al., 2017). Hydrogel biomaterials have excellent biocompatibility, share some of the physical properties of the natural matrix, and provide a flexible solution to introduce biochemical and biophysical triggers. Ongoing efforts to establish an engineered BM-like niche for the in vitro expansion of HSCs is primarily focused on refining hydrogel-based culture methods (Yin and Cao, 2021).
This review summarizes hydrogel-based engineering methods used for recreation of the hematopoietic microenvironment for the purpose of the in vitro expansion of HSCs (Figure 1). We briefly describe the basic physiological characteristics of the hematopoietic niche, challenges culturing HSCs ex vivo, and recent culture approaches, followed by an overview of the engineering strategies used for replicating the natural hematopoietic niche. We also discuss new technologies for establishing an “intelligent microenvironment.” Finally, challenges and perspectives in the development of the hydrogel-based culture platforms for clinical application are evaluated.
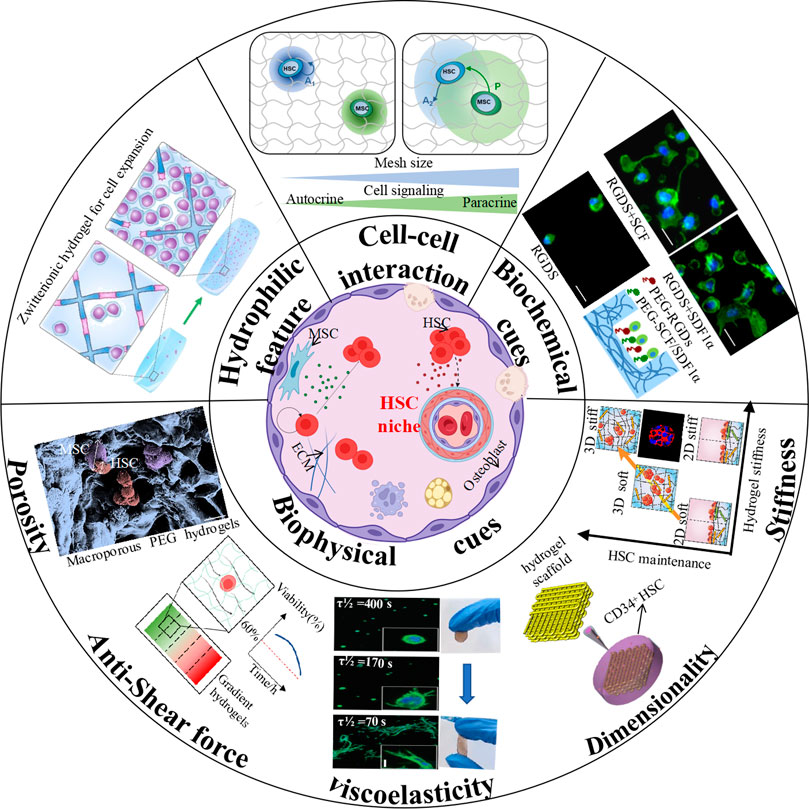
FIGURE 1. Schematic illustration of hydrogel engineering strategies for hematopoietic niche recapitulation. (Cell-cell interaction: (Gilchrist et al., 2019b) Copyright © 2019. Reproduced with permission from John Wiley and Sons; Biochemical cues: (Cuchiara et al., 2013) Copyright © 2019. Reproduced with permission from John Wiley and Sons; Stiffness: (Gvaramia et al., 2017) Copyright © 2017. Reproduced with permission from Elsevier; Dimensionality: (Zhou et al., 2020) Copyright © 2020. Reproduced with permission from Springer Nature; Viscoelasticity-Left: (Chaudhuri et al., 2016) Copyright © 2015. Reproduced with permission from Springer Nature; Viscoelaticity-Right: (Liang et al., 2022) Copyright © 2022. Reproduced with permission from American Chemical Society; Anti-shear force: (Mahadik et al., 2014) Copyright © 2013. Reproduced with permission from John Wiley and Sons; Porosity: (Raic et al., 2014) Copyright © 2014. Reproduced with permission from Elsevier; Hydrophilic feature: (Bai et al., 2019) Copyright © 2019. Reproduced with permission from Springer Nature.
2 The hematopoietic niche
In 1978, Schofield introduced the concept of the “niche”, which refers to the HSC microenvironment in the BM (Chatterjee et al., 2021). The niche provides indispensable factors for the self-renewal and differentiation of HSCs, controls the quiescence and stemness characteristics of HSCs, and regulates cell fate (Rasheed, 2022). In early studies, the hematopoietic niche was classified as the endosteal niche and perivascular niche based on the distribution of cell populations (Zhao and Li, 2016). The endosteal niche contains osteoblasts and osteoclasts at the endosteum. These cells regulate homing and the maintenance of quiescence in HSCs (Ingavle et al., 2019). The perivascular niche, formed in the vicinity of blood vessels, can promote the interaction of HSCs with endothelial, perivascular, and mesenchymal stem cells (MSCs) (Silberstein and Lin, 2013; Barnhouse et al., 2021). With increasing molecular mechanism studies, more and more cellular constituents have been identified in BM niche. Current research has showed that it is possible for different types of hematopoietic stem cells and progenitor cells (HSPCs) to have their own special niche created by multiple cell types. (Morrison and Scadden, 2014).
The HSC pool is highly heterogeneous in function and molecules it contains, which has increased the possibility that “specialized” niches are existed for “specialized” HSCs. This could also be inferred from the physiological distribution characteristics of HSCs. It has been reported that almost all HSC cells are in near range sinusoid vessels and are supported by the factors, such as Stem Cell Factor (SCF), C-X-C motif chemokine 12 (CXCL-12), and pleiotrophin, synthesized by leptin Receptor+ (LepR+) MSCs and endothelial cells (Christodoulou et al., 2020). Few HSCs are distributed in the BM, and <20% are located within 10 µ m from the BM intima (Lo Celso et al., 2009). These small HSC populations may be directly or indirectly regulated by factors near the bone surface (Cao et al., 2019; Zhao et al., 2019). In addition, some important factors for maintenance of HSCs are not synthesized in the BM, such as thrombopoietin (TPO), which is generated by the liver and transported to the BM through the blood (Nakamura-Ishizu et al., 2014). Therefore, the hematopoietic niche is composed of multiple components including different cells, biomolecules, and matrix, in which each component of HSCs has an important regulatory role.
2.1 Cellular compounds
2.1.1 Osteoblasts
Osteoblasts are particularly common in the trabecular bone of the endosteum and are important in regulating HSC maintenance (Calvi et al., 2003; Zhang et al., 2003). Primitive HSPCs primarily home and engraft in the endosteal niche, which is rich in immature osteoblasts and osteoblastic progenitor cells (Levesque et al., 2010). Along with other cytokines, these immature osteoblasts secrete angiopoietin-1 (Arai et al., 2004), osteopontin (Stier et al., 2005), and CXCL12 to support HSC quiescence and self-renewal (Matteini et al., 2021). In contrast, mature osteoblasts induce the proliferation of HSCs (Choi et al., 2015).
Osteoblasts were one of the first non-hematopoietic BM cells reported to participate in HSPC regulation (Nilsson et al., 2001). Early studies showed that osteoblasts differentiated from human BM stromal cells could produce hematopoietic cytokines and support the HSC maintenance (Calvi et al., 2003; Zhang et al., 2003). More recent studies showed that conditional deletion of CXCL12 or SCF in osteoblasts did not affect the frequency of HSC in BM, which indicates that although these two factors are necessary niche factors for maintaining HSC, osteoblasts do not support the maintenance of HSCs through these niche factors (Greenbaum et al., 2013; Crane et al., 2017). It has been reported that osteoblasts can promote the maintenance of early lymphoid progenitor cells by secreting extremely low levels of CXCL12. It is worth mentioning that most lymphoid cells are located in the perisinusoidal niche in which they rely on CXCL12, and Transforming Growth Factor (TGF-β), synthesized by LEPR+ cells (Shen et al., 2021).
2.1.2 Endothelial cells
Nutrient arteries entering the cortical bone divide into descending and ascending branches. These extend axially along the central cavity containing the BM, giving off radial branches. These enter the endosteal region of the cortical bone in a small network of Sca1+ arterioles and capillaries. These vessels anastomose with Sca1- venous sinuses (approximately 50–100 µm in diameter). These venous sinuses drain into the central longitudinal vein running in the BM (Ramasamy, 2017; Owen-Woods and Kusumbe, 2022).
Sca1+ arterial endothelial cells are present in transitional vessels (or type H vessels), arterioles and arteries (Langen et al., 2017). Type H vessels represent a specific subset of capillaries localizing exclusively to the endosteal region of the BM. They enhance angiogenesis and osteogenesis by transducing signals to osteoprogenitor cells (Spencer et al., 2014). Both H-type vessels and arterioles exhibit low permeability, protecting HSCs from high levels of reactive oxygen species (ROS). This phenomenon prevents the overactivation of the progenitor cells that could cause the loss of stemness (Kunisaki et al., 2013; Silberstein and Lin, 2013; Spencer et al., 2014). Sca1- sinusoidal endothelial cells represent the main endothelial components of L-type vessels (Langen et al., 2017). In contrast to the arteriolar system, around the sinusoids ROS levels are relatively high. This enhances the migratory capacity and activation of HSCs (Itkin et al., 2016). Nonetheless, some reports suggested that sinusoidal endothelial cells may also contribute to the maintenance of HSC quiescence (Acar et al., 2015).
2.1.3 Mesenchymal stem cells
BM-derived MSCs have excellent potential for self-renewal and multilineage differentiation. They can form osteocytes, fat cells, cartilage, muscle cells, nerves, liver cells, and form the substrate cells supporting hematopoiesis (Pievani et al., 2021). MSCs secrete a variety of cytokines, hematopoietic and non-hematopoietic growth factors, and chemokines. Together, these modulate the BM microenvironment and regulate the proliferation and differentiation of HSCs. It appears that the secretion of CXCL12 by MSCs can induce the CXC12-CXCR4 mediated homing and retention of HSCs, while the production of stem cell growth factor (SCF) is responsible for cell survival and proliferation (Mannino et al., 2022). The simultaneous transplantation of MSCs and HSCs can promote HSCs homing, promote hematopoietic reconstruction, and reduce graft rejection (Jing et al., 2010). MSCs secrete multiple biomolecules by interacting with HSCs to support their growth and further to promote the hematopoietics. Meanwhile, MSCs inhibit the immunogenicity and immunomodulatory and reduce the incidence of graft-versus-host disease (GVHD). Co-transplantation of MSCs and HSCs has been applied in clinical and achieved positive results. Because MSCs are relatively easy to obtain without major ethical and legal issues their potential to support the in vitro expansion of HSCs can be easily studied.
2.1.4 Adipocytes
Adipocytes are the most abundant cells in the BM of adults and are commonly seen in close contact with hematopoietic cells. Before birth the bone marrow cavity does not contain any fat cells but the amount of bone marrow adipose tissue (BMAT) increases with age and eventually occupies up to 50% of the BM cavity (Hardouin et al., 2014). Traditionally, adipocytes were thought to negatively regulate HSC function in the BM microenvironment (Naveiras et al., 2009). However, emerging evidence suggests that adipocytes may promote the maintenance of HSCs. Mattiucci (Matteini et al., 2021) demonstrated that BM-MSCs can differentiate into BM adipocytes expressing specific cytokines. Amongst these Interleukin-3 appear to support HSC survival.
2.1.5 Progeny of hematopoietic stem cells
In addition to the stromal niche components, various progenies of HSCs including macrophages, neutrophils, regulatory T cells, and megakaryocytes, also participate in the regulation of the hematopoietic niche in the BM (Pinho and Frenette, 2019; Torres et al., 2022). Macrophages can regulate HSC migration; Bone marrow macrophages promote the retention of HSCs by regulating osteolineage cells and MSCs, thus antagonizing the excretion of HSC from BM mediated by SNS (Méndez-Ferrer et al., 2010; Chow et al., 2011). Macrophages clear senescent neutrophils and change the function of CXCL12 antibody reticular cells and retain HSCs in BM (Casanova-Acebes et al., 2013). Neutrophils derived from the bone marrow can promote endothelial cell regeneration after transplantation by secreting TNF, thus accelerating hematopoietic recovery (Bowers et al., 2018). Studies have shown that allogeneic hematopoietic stem cells co-locate with FoxP3+ regulatory T cells in the endothelial area after transplantation and can survive for 1 month without immunosuppressive drugs (Fujisaki et al., 2011; Hirata et al., 2018). However, depletion of Tregs cells leads to the rapid loss of allogeneic hematopoietic stem cells, indicating that FoxP3+ Tregs provide immune privilege for HSC (Fujisaki et al., 2011). Megakaryocytes maintain HSC quiescence by secreting CXCL4 (Bruns et al., 2014), TGF-β (Zhao et al., 2014), and TPO (Nakamura-Ishizu et al., 2014).
2.2 Cytokines
Cytokines are small protein molecules secreted by a variety of cells. By interacting with specific receptors they regulate the biological behavior of other cells. SCF, CXCL12, and thrombopoietin (TPO) are essential growth factors for HSCs (Yucel and Kocabas, 2018). SCF, also known as KIT ligand, exists in membrane binding and dissolving configurations (Asada et al., 2017). Both forms bind to the c-kit receptor, a tyrosine kinase, on the surface of HSCs, and promote HSC expansion. TPO is expressed mainly in the liver and kidneys and is a soluble factor necessary for the survival and proliferation of CD34+ HSPCs (Chatterjee et al., 2021). CXCL12 (also known as SDF-1) is secreted by several cells in HSC niche. CAR cells found in perivascular regions represent the main sources of CXCL12 (Mendelson and Frenette, 2014; Kosan and Godmann, 2016). The CXCL12/CXCR4 signaling cascade plays an essential role in the homing, mobilization, and retention of HSCs (Verma et al., 2020).
2.3 Neural regulation
There is evidence to show that the autonomic nervous system (ANS) and SNS can regulate the fate of HSCs (Maryanovich et al., 2018). ANS signals are important for the circadian mobilization of HSCs in the BM niche (García-García et al., 2019). SNS fibers respond to Granulocyte Colony-Stimulating Factors (G-CSF) and release norepinephrine, which activates β3-adrenergic receptors to control rhythmic changes in CXCL2 expression (Asada et al., 2013; Matteini et al., 2021). Non-myelinating Schwann cells promote HSC quiescence and maintenance by activating TGF-β and Suppressor of Mothers Against Decapentaplegic (SMAD)-induced signaling pathways (Mannino et al., 2022). However, studies on the regulating mechanism of sensory fibers on HSC behaviors in the BM niche are very limited in recent. One study reported that nociceptive receptors of the sensory neuropeptides lcitonin gene-related peptide could regulate G-CSF-induced HSC mobilization (Gao et al., 2021).
2.4 Niche extracellular environment
The ECM is a scaffold network that contains embedded HSCs and stromal cells. It is rich in collagen, laminin, and elastin, and contains a mixture of cytokines regulating proliferation and differentiation behaviors (Bello et al., 2018). This network is composed of two main classes of macromolecules: gel matrix components and fibrous protein components. The gel matrix components form gel-like structures composed of polysaccharides, amino sugars, and a range of protein constituents. In contrast, fibrous proteins primarily include collagen and elastic proteins and form a 2D or 3D structure with tunable stiffness and elasticity. This provides spatial support for the survival of HSCs and regulates their growth through interactions with integrin molecules present on the surface of HSCs (Chatterjee et al., 2021). The most studied fibrous proteins of the natural niche are fibronectin and laminin, which can bind to integrins and regulate the migration and homing behavior of HSCs. For example, studies have shown that BM laminins promote adhesion and migration of human HSCs through interaction with the integrin α6 receptor for homing (Gu et al., 2003). Stiffer endosteal environments support the differentiation of HSCs into primitive myeloid progenitors, while softer substrates promote differentiation along the erythroid lineage. The adherence and motility of HSPCs is significantly higher on harder surfaces.
3 Hematopoietic stem cell culture ex vivo
3.1 Challenges of hematopoietic stem cell culture ex vivo
Two main types of HSCs were defined: long-term (LT)-HSCs, which are deeply quiescent in niches to maintain self-renewal and multilineage differentiation potential throughout their lives, and short-term (ST)-HSCs, which are maintained in more active niches with limited self-renewal potential (Choi et al., 2015). The main goal of ex vivo culture of HSCs is to expand the number of HSCs and HSPCs with a high capability of self-replicating and self-maintenance. However, few normal HSCs are in the cell cycle and undergo asymmetric mitosis. The asymmetric mitosis of HSCs is highly self-replicating and self-sustaining. After several mitoses, the cells stop entering the S phase and turn to the quiescent phase G0, therefore, it is difficult to effectively expand them ex vivo. If HSCs are induced to undergo symmetrical mitosis through gene mutation, that is, the cells expand indefinitely without differentiation, this will inevitably lead to malignant transformation of the cells, with a loss in the ability to self-renewal and self-maintenance. During cell culture, supplementation with inappropriate cytokines or long-term culturing can promote both proliferation and differentiation of cells and destroy the asymmetric mitosis of the cells. Therefore, long-term culture may obtain a large absolute number of CD34+ cells or nuclei cells; it also easily to lead to the differentiation and exhaustion of the early progenitor cells and only produces numerous HSPCs without hematopoietic reconstitution function.
Heterogeneity of HSCs is another important reason for the difficulty in their expansion in vitro. Ex vivo expansion methods of HSCs reported in the literatures are often quite different. Even under the same conditions of cytokine combination, the expansion ability and transplantation ability of cells are still significantly differ. The heterogeneity of HSCs also makes it challenging to understand the comprehensive signaling mechanism that defines the balance between homeostasis and self-renewal of among cells. Recent evidence has added new insights into the plasticity of BM niche and mitochondrial network, which synergistically determine the fate of HSCs. However, these new discoveries also increase the complexity and introduce a highly dynamic interrelationship in studies of HSC fate. Therefore, research on the complex mechanisms controlling the fate of HSCs remains limited.
3.2 Recent approaches to hematopoietic stem cell culture ex vivo
Compared with haplotype hematopoietic stem cell transplantation (haplo HSCT), umbilical cord blood stem cell transplantation (UCBT) can be easily obtained from CB resources. UCBT has high tolerance to human leukocyte antigen HLA mismatch, a high colony forming potential of HSCs and HSPCs, and a low incidence and recurrence rate of graft versus host disease (GVHD) following transplantation. Furthermore, HSCs are ideal vector cells for gene therapy. The long-term self-renewal and self-maintenance abilities of HSCs are conducive to the long-term expression of the target therapeutic genes in vivo. However, insufficient amounts limit the clinical application. Ex vivo expansion of HSCs is the basis both for the clinical promotion of UCBT and gene therapy. Therefore, many efforts have been focused on improving HSCs expansion methods.
Supplementing with the hematopoietic stimulators is a typical method for HSC expansion. Cytokines are one of the first molecular stimulators for HSC expansion ex vivo. After 5–8 days of culturing of human CB CD34+CD38− cells in serum free medium containing Fms Related Tyrosine Kinase 3 (Flt-3), SCF, Interleukin (IL)-3, IL-6 and G-CSF, the number of colony forming units (CFU) increased 100 -fold, and the number of long-term culture-initiating cells (LTC-IC) increased 4-fold (Conneally et al., 1997). The addition of Fibroblast Growth Factor 1 (FGF1) and FGF2 to the serum free medium during the co-culture of HSCs and osteoblast supported the expansion and regeneration of HSCs in vitro (Yeoh et al., 2006). Notch signaling pathway is an important pathway for HSC fate and lymphocyte generation. An engineered Delta-like ligand Delta1Ext−IgG (DXI) was used to activate the Notch signal pathway in human CB CD34+ cells to achieve clinically significant in vitro expansion of ST-HSPCs with and shorten the implantation time of neutrophils following transplantation (Delaney et al., 2005; Delaney et al., 2010). In addition, TNF Superfamily Member 15 (TNFSF15), the Wnt signaling pathway and Prostaglandin E2 (PGE2) were used ex vivo to expand the long-term reconstruction ability of HSPCs (Ding et al., 2020).
The addition of small molecule compounds such as copper chelator tetraethylenediamine (TEPA), nicotinamide, stem regin-1 and valproic acid, which simultaneously expand ST- and LT-HSCs, provide effective schemes for HSC amplification in vitro (Zimran et al., 2021). U1M171, an HSC agonist, is one of the most promising small molecules for HSC expansion in recent years (Fares et al., 2014). In 2014, Guy Sauvageau and Peter W zandstra (Fares et al., 2014; Pabst et al., 2014) were the first to show that UM171 can significantly expand umbilical CB stem cells and enhance multilineage hematopoietic reconstruction in rats. In 2019, the team published Phase I/II clinical trial results (NCT02668315) on the safety and feasibility of a single UM171 expanded umbilical CB graft in The Lancet Haematology (Cohen et al., 2020). The minimum dose of rapidly implanted umbilical CB cells in this clinical trail was 0.52 × 105 CD34+ cells, which has relatively low chronic GVHD and recurrence risk due to rapid T cell reconstruction.
MSCs are the main cell component of hematopoietic microenvironment and plays an important role in the growth, proliferation, and differentiation of HSCs. MSCs support and promote hematopoiesis by interacting with HSCs and secreting various cytokines, or expressing, and through the expression of adhesion molecules and ECM proteins related to adhesion and homing of HSCs. In vitro experiments have shown that MSCs plays an important role in the maintenance and expansion of CD34+ BM cells, but do not affect the erythroid differentiation ability (Lau et al., 2017). At present, great progress has been made in the research of MSC-promoting hematopoietic reconstitution following HSCT. MSC and HSC co transplantation supports the growth of HSCs and significantly improves megakaryocyte and platelet formation. In addition, MSCs may also play a role in epigenetic regulation during HSC differentiation (Zakrzewski et al., 2019).
Static culture systems, such as culture plates and flasks, generally require repeated processing of HSCs regarding fluid change. Furthermore, due to ineffective mixing, the non-homogeneous of compounds may result in differences in the initial stimulation of cultured cells. The continuous perfusion bioreactor is a fully closed dynamic culture automation device to create 3D constructed microenvironments with different cellular or biomechanical features (Bhaskar et al., 2018). For example, Martin’s group developed a perfusion bioreactor system analogue, which partially recapitulated the structural and compositional features of the human osteoblastic niche. Human purified CB-derived were cultured in the bioreactor; after 1 week culture, number of the CD34+ cells and colonies numbers significantly increased. Perfusion bioreactor systems provided important evidence for dynamic control of the expansion quality during ex vivo HSC culture (Bourgine et al., 2018).
The hematopoietic niche is composed of biophysical and biochemical factors, which play a key role in the static, self-renewal, implantation, migration, differentiation, and other behaviors of HSCs. HSCs in the niche are affected by the mechanical force matrix, physical or chemical stimulations from stromal cells and the environment, as well as the cytokines distributed in the 3D niche space. Biomaterials with adjustable physical, mechanical, and chemical properties can easily be modified by different biological molecules, which opens up new opportunities for controlling the bionics of the niche. Compared with inorganic or metal biomaterials, hydrogels are organic materials with excellent biocompatibility. In vivo, the hematopoietic niche is a 3D environment dominated by hydrophilic and amphiphilic cell membrane lipids. Hydrogels have good hydrophilicity and rich functional groups; it is easy to modify the hydrophobic groups and various biomolecules. Their controllable physical and chemical characteristics, such as hardness, porosity, elasticity, and functionalization of key cytokines, can be beneficial in creating an environment closer to the niche.
4 Engineering hydrogels based on niche physiology
When HSCs detach from their niche microenvironment, the cells easily exhaust and differentiate. 2D culturing is not consistent with the spatial feature of natural niches. The intricated chemical and physical cues inside niche are crucial to regulate HSCs behavior. Therefore, 3D culturing of HSCs has been extensively researched recently; hydrogel biomaterials with excellent biocompatibility have been gradually employed for establishing a 3D environment for HSCs. (Yan et al., 2022) (Maji and Lee, 2022).
Hydrogels with good hydrophilicity capacity can swell in aqueous media, minimize non-specific protein adsorption, and inhibit collagen capsule formation, thus preventing undesirable differentiations of HSCs (Zhang et al., 2015; Erathodiyil et al., 2020). In addition, the hematopoietic niche consists of a series of ECMs and interacting components (Winkler et al., 2020). Engineered hydrogels can be developed to contain interconnected polymer networks to spatially mimic the ECM networks and the cell–cell contacts in the natural niche. Stiffness can be achieved by regulating the degree of polymer cross-links (Rosales and Anseth, 2016; Tang et al., 2021; Maji and Lee, 2022). Such structures render hydrogels high permeable, supporting nutrient exchange between cells and the environment (Liu et al., 2019).
To better replicate cell–matrix interactions and improve the responsiveness of hydrogels to microenvironmental changes, they can be chemically and mechanically modified. These modifications enable the production of hydrogels with adjustable viscoelastic, mechanical, degradation, and cell adhesion characteristics, as desired (Zhang and Khademhosseini, 2017; Prince and Kumacheva, 2019; Caldwell et al., 2020; Liang et al., 2022). Aside from the provision of an artificial niche in cell cultures, hydrogels can also be used in the experimental studies of cellular behaviors and cell–material interactions (Costa et al., 2022). Various components can be introduced into engineered hydrogels to help the mimic the hematopoietic niche for the expansion of HSCs and the regulation of cellular behavior. These modifications of hydrogels are examined in the following sections. Recent engineering hydrogel strategies of the biomimetic hematopoietic niche and in vitro cultures are summarized in Table 1. Information on the characterization and functional assessments of cultured HSCs on these hydrogel-based studies are supplemented in Table 2.
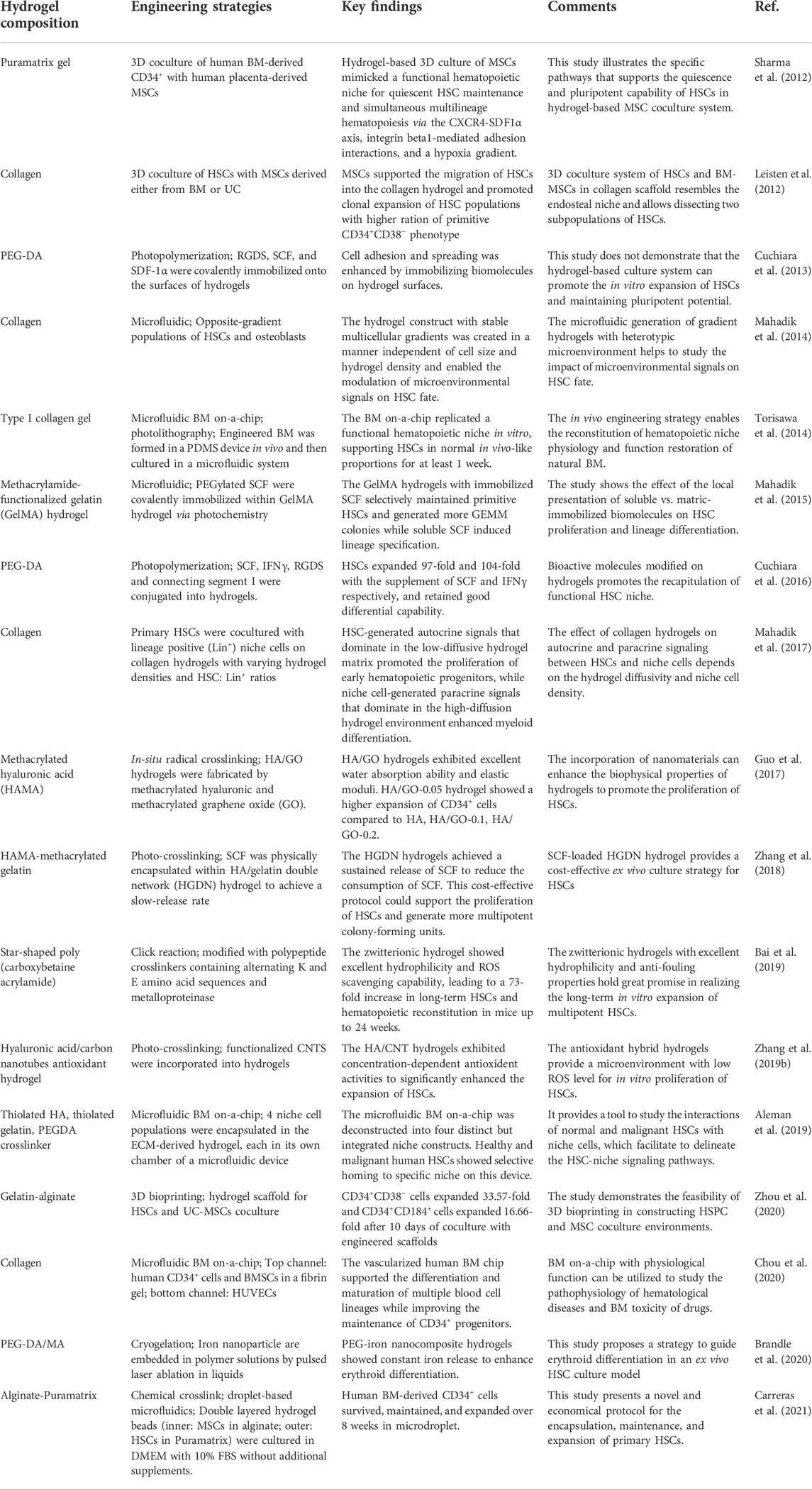
TABLE 1. Engineering hydrogel strategies of biomimetic hematopoietic niche and in vitro HSC culture.
4.1 Polymers for matrix networks
Hydrogels are fabricated by covalently or non-covalently cross-linking polymer chains dispersed in an aqueous solution. These 3D hydrogels are constructed by chemical cross-linking (Zhang and Khademhosseini, 2017; Yin and Cao, 2021) (Figure 2A), photo cross-linking (Nezhad-Mokhtari et al., 2019) (Figure 2B) and atom-transfer radical polymerization (Bai et al. (2019)) (Figure 2C). Depending on the source of polymers, hydrogels are classified into natural, synthetic, and hybrid polymers (Maji and Lee, 2022). As the name indicates, natural hydrogels contain polymers derived from biological sources. These matrices commonly contain alginate, hyaluronic acid (HA), chitosan, collagen, gelatin, and fibrin. Natural polymers are inherently biocompatible with living cells (Bao et al., 2019; Jiang et al., 2020; Peers et al., 2020); however, their practical use is limited by batch-to-batch compositional inconsistency, poorly controlled polymerization kinetics, and chain configuration (Echalier et al., 2017). These limitations can be overcome by using of bioengineered versions of natural polymers. These synthetic alternatives facilitate the controlled polymerization of the hydrogels (Zhao et al., 2016; Zhan et al., 2022). Polyethylene glycol (PEG), polylactic acid, polyvinyl alcohol, and polyurethane are synthetic polymers commonly utilized in tissue engineering and in vitro cell culture (Gjorevski et al., 2016). Hybrid hydrogels combine the best features of natural and synthetic polymers. Most research and practical use applications rely on these hybrid systems (Afewerki et al., 2019). Changing the composition and ratio of the incorporated polymers affects both the biocompatibility and mechanical properties (e.g., stiffness and degradation time), thus influencing cell fate and behavior (Wolf et al., 2015; Zhang et al., 2016). For example, keratin/PEG composite hydrogels exhibit a high compressive modulus of 45 kPa and long-term stability in physiological buffer solutions and cell culture media (Yue et al., 2018).
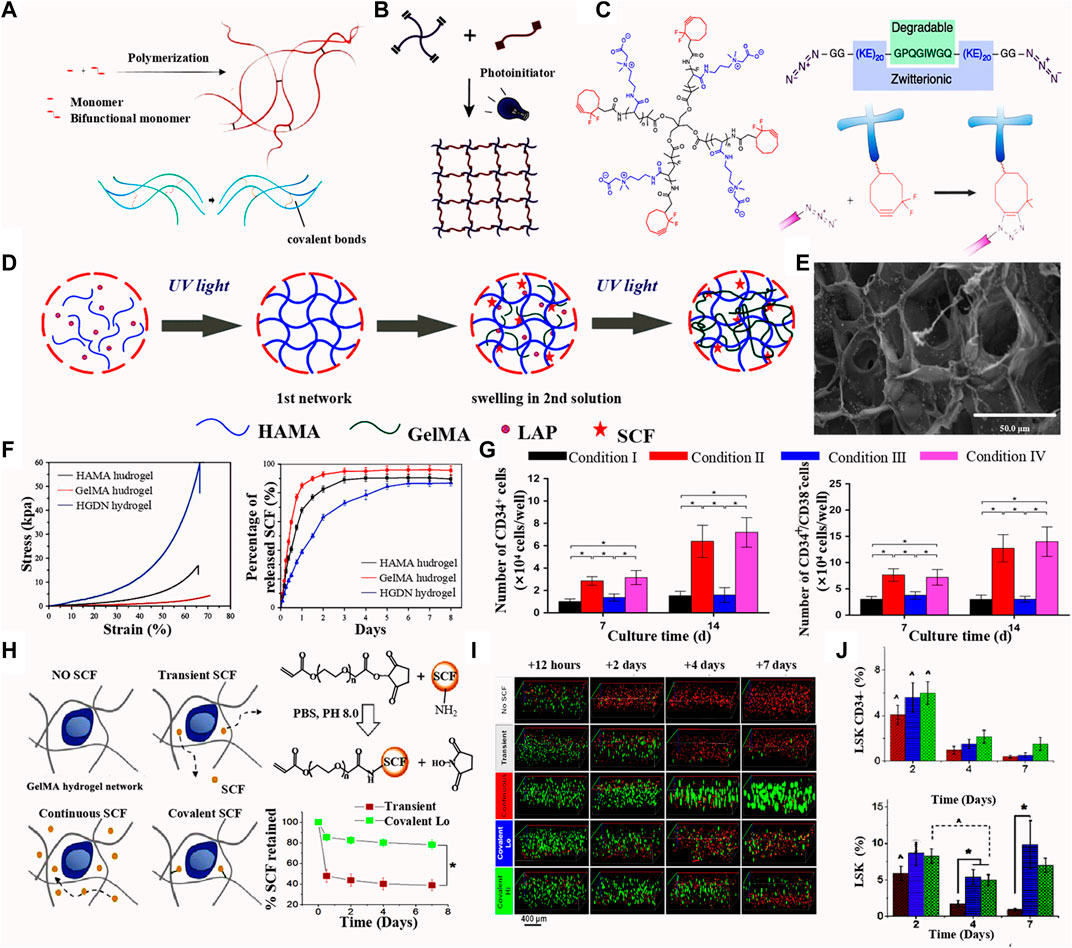
FIGURE 2. (A) Scheme of chemical crosslinking of hydrogel. Upper: (Yin and Cao, 2021) Copyright © 2021. Reproduced with permission from Elsevier. Lower: (Zhang and Khademhosseini, 2017) Copyright © 2017. Reproduced with permission from The American Association for the Advancement of Science. (B) Scheme of photo crosslinking. (Nezhad-Mokhtari et al., 2019 ) Copyright © 2019. Reproduced with permission from Elsevier. (C) Schemes of poly (carboxybetaine acrylamide) with a star-shape; polypeptide crosslinkers with KE sequences as the metalloproteinase degradable sites; The hydrogel synthesis basing on the step-growth polymerization mechanism of Huisgen cycloaddition. (Bai et al., 2019) Copyright © 2019. Reproduced with permission from Springer Nature. (D) Fabrication of the HGDN double network hydrogel and the physical loading of SCF. (E) Scanning electron microscopy images of the SCF-loaded HGDN double network hydrogel. (F) Stress-strain curves and SCF releases analysis of the HAMA, GelMA and HGDN hydrogels. (G) Percentages of CD34+ and CD34+CD38- phenotype of cells culture for 7 and 14 days. (Zhang et al., 2018) Figure 2D-G: Copyright © 2017. Reproduced with permission from John Wiley and Sons. (H) Different methods or SCF modification in hydrogels (left); SCF release curve of the transient and covalent modification of SCF. (I) Florescence images of the phenotype of cells cultured hydrogels with different release mode of SCF. (J) Percentage of LSK and CD34+ phenotype of cells culture in the SCF-loaded hydrogels with different culture time. (Mahadik et al., 2015) Figure 2H-J: Copyright © 2015. Reproduced with permission from Elsevier.
4.2 Regulation of bioactive molecules
The BM microenvironment contains various biologically active components, such as cell adhesion molecules, growth factors, cytokines, enzymes, and other signaling molecules. These represent specific signaling pathways and regulate diverse cellular processes, including proliferation, differentiation, migration, and apoptosis (Hastings et al., 2019; Winkler et al., 2020). Non-modified synthetic polymers and most natural polymers (e.g., alginate and chitosan) lack these bioactive molecules (Maji and Lee, 2022). Therefore, considerable effort has been devoted to developing hydrogel polymers containing biomolecules necessary for the creation of a cellular microenvironment that enables the precise control of cellular behaviors (Caldwell et al., 2020). These biomolecules can be incorporated by non-covalent means, such as physical entanglement (Bovone et al., 2021), electrostatic interactions (Sivakumaran et al., 2013; Wang et al., 2018), or through covalent tethering (Zhang and Khademhosseini, 2017; Bovone et al., 2021). Incorporating biomolecules via non-covalent weak interactions is more conducive towards the controlled release of nutrients and regulatory molecules.
Zhang group (Zhang et al., 2018) fabricated a HA/gelatin double network (HGDN) hydrogel that is physically loaded with stem SCF. The HA methacrylate (HAMA) hydrogel was fabricated by pouring the pre-gel HAMA solution and the photo-initiator phenyl-2,4,6-trimethylbenzoyl phosphonate lithium (LAP) into a round mold, which was then irradiated solution with ultraviolet light for a fast cross-linking. The HAMA network was then immersed in the GelMA solution with LAP until swelling equilibrium was reached and then photo-cross-linked to form a double network Figure 2D. This double network hydrogel is combined a fragile network and a double network and exhibits high mechanical strength and sustained release of SCF (Figure 2E, F). After 14 days of culturing, the total number of nucleated cells in the SCF-loaded HGDN hydrogel was approximately 3-fold higher than that of cells in the non-loading hydrogel, and the proportion of CD34+ CD38− cells was slightly higher in the non-SCF treated group (Figure 2G). A CFU assay showed SCF-loaded HGDN hydrogels had more primitive CFU-GEMM (granulocyte, erythrocyte, monocyte, megakaryocyte) phenotype compared to the cells in plated with SCF. The HGDN hydrogel retained the SCF release for 6 days. Chemical incorporation of biomolecules through covalent, ionic, or metallic binding is widely used in the construction of 3D hydrogel networks (Xin et al., 2019; Chimene et al., 2020). Mahadik et al. (2015) demonstrated a methacrylamide-functionalized gelatin (GelMA) hydrogel covalently modified with SCF for culturing of primary HSCs isolated from the BM of mice. SCF was linked to the PEG- N-hydroxysuccinimide (NHS) ester and reacted with GelMA hydrogel precursor in solution (Figure 2H). When comparing the performance of the continuous SCF and covalent SCF in GelMA hydrogels, continuous SCF greatly increased the cell number after 7 days of culture and quickly reduced the cell number in longer culture, while reducing the maintenance of the initial LSK phenotype and primitive CD34− LSK cells (Figures 2I, J). In contrast, covalent SCF showed increased maintenance of the 2 cell populations. At day 7, CFU colonies had increased for cells treated with covalent SCF, and the cell expansion reached 3 which was higher than that of day 1.
Cuchiara et al. (2013) covalently immobilized the cell adhesion peptide RGDS, the cell growth factor SCF, and the stromal derived factor 1a (SDF1α) on the surface of a PEG hydrogel to control the adhesion and spreading of HSCs. An anchorage-independent hematopoietic cell line, named 32D clone 3 cells were seeded into the PEG-diacrylate (DA) hydrogel wells modified with the above compounds. The number of adherent 32D cells was higher in the 25 μg cm−2 CF and SDF1α modified hydrogel well compared with other concentration, in addition to a larger adhere area (450 μm−2). Sustained activation of CXCR4 receptor in the 32D cells shows the effect of biomolecules on 32D cell migration in the modified PEG-DA hydrogel. A concept of biological cues introduced into the hydrogel well for HSC culture was revealed, while experiment on the measurement of more HSC biomarkers has been limited.
This research group also fabricated a HSPC culture substrate with the derivatives of PEG-DA hydrogels. PEG-DA hydrogel wells were fabricated via a microfabrication patterning technology assisted by a photomask and photoresist. Proteins and peptides including the key cytokines, SCF, interferon-g (IFNg) and RGDS connecting segment 1, were chemically modified onto PEG with certain ratios to form the PEG-protein and PEG-RGDS composite. The composite was immobilized on the well surface via a photo-induced linking reaction. C-kit+ cells collected from mouse BM cells were cultured in the PEG-DA hydrogel wells. After 2-week culture, the cell number increased by 11.6–19.0% for cells 3D encapsulated in hydrogel, which is higher than that in 2D culture, and 53.8% cells remined c-kit+ without an observed loss. Encapsulated c-kit+ cells could form all colonies which had similar results to that of 2D culture. However, 3D culture exhibited a larger number of multilineage HSPC-derived colonies than that of 2D culture. These experiments demonstrated that cytokines encapsulated in a 3D scaffold better replicated the cell–matrix interactions and showed advantages in controlling diffusion in cultures designed for LT-HSC expansion compared with the 2D hydrogel (Cuchiara et al., 2016) (Figures 3A,B). SCF is a key component of the native HSC niche and needs to be continuously and abundantly supplied in the medium when HSCs are cultured in vitro. Mahadik et al. (2015) reported the use of a composite hydrogel created by chemically immobilizing SCF within a degradable GelMA hydrogel matrix. Convective mixing of different precursor solutions of hydrogels was applied to creat a gradient environment in 3D hydrogels, which could influence cell aggregation and migration, or produce channels to transfer growth factors in gradient concentrations. By adjusting the geometry and the mixing models of the channels, they intend to use the device to develop a complex 3D culture environment that more closely mimics the elements of cell populations, matrix, and biological molecules within the BM. They observed single LSKs and Lin+ BM cells and found that using the spectral deconvolution methods could identify the LSKs (cKit+ Sca1+) from mature BM (Lin+) cells. The covalent incorporation ensured that the GelMA hydrogel retained its SCF content for longer than 7 days. The stable provision of this critical growth factor was sufficient to support the proliferation and lineage differentiation of HSCs.
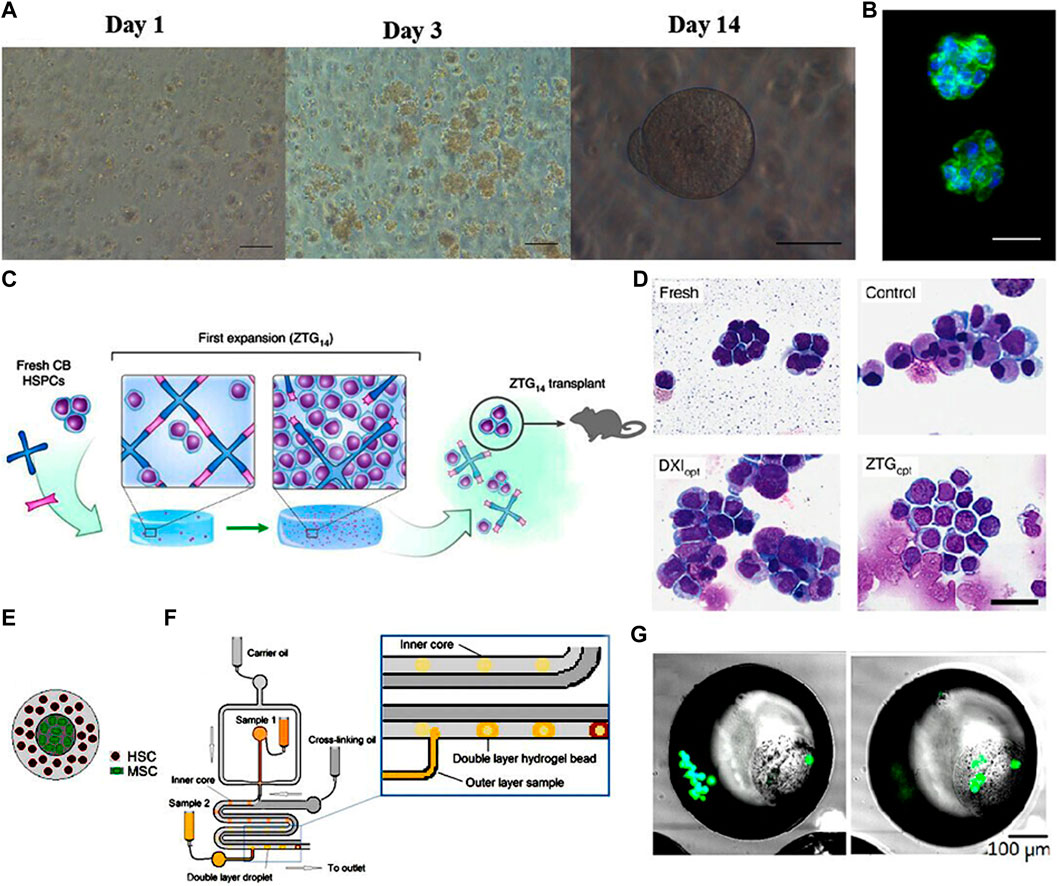
FIGURE 3. (A) Phase contrast images of HSCs encapsulated within bioactive PEG hydrogels at days 1, 3, and 14 showing the proliferation and round shape retention of HSCs. Scale bar: 100 mm (day 1, day 3), 50 mm (day 14). (B) DAPI (blue) and phalloidin (green) staining of HSCs after 14 days expansion showing the cell morphology retention and gel structure integrity. Scale bar: 25 mm. (Cuchiara et al., 2016) Figure 3A, B: Copyright © 2015. Reproduced with permission from John Wiley and Sons. (C) Schematic illustration of the zwitterionic hydrogels with superhydrophilicity and volumetric swelling. (D) Photomicrograph of Wright-Giemsa-stained HSCs showing the significant expansion of the primitive HSC population in zwitterionic hydrogels. Scale bar: 30 μm. (Bai et al., 2019) Figure 3C, D: Copyright © 2019. Reproduced with permission from Springer Nature. (E) Schematic representation of the double layered hydrogel bead with HSCs on the outer core and MSCs on the inner core. (F) Schematic illustration of the double layered structure fabrication process using droplet-based microfluidic. (G) Fluorescent staining of viable MSCs and HSCs show that both types of cells are successfully encapsulated in the double layered structure. (Carreras et al., 2018) Figure 3E-G: Copyright © 2018. Reproduced with permission from Elsevier.
In summary, modifying 3D hydrogels through the incorporation of important functional biomolecules via chemical or physical means can create a complex growth environment for HSC culture that more closely approximates the natural niche and allows the precise regulation of cell behavior to achieve extensive expansion of cells, while reducing undesired differentiation.
4.3 Regulating the biophysical features
Several groups have reported the beneficial effects of the biophysical characteristics of hydrogels during the culture of MSCs and embryonic stem cells (Lu et al., 2014; Wong et al., 2021; Costa et al., 2022). Based on prior experimental observation, hydrogels with tunable biophysical characteristics were developed to mimic the native spatial architecture of the hematopoietic niche (Zhang P. et al., 2019; Wong et al., 2021). The most important physical properties include the matrix architecture of the microenvironment, which can be appropriately modified by engineering changes (Vining and Mooney, 2017; Zhang Y. et al., 2019). The behavior of HSCs can be regulated by adjusting the stiffness, dimensionality, architectural features, viscoelasticity, and shear forces in hydrogel matrices.
Physiological compounds of the HSC niche vary in stiffnesses; for example, the stiffness of soft marrow or adipose tissue is lower than 1kPa, cell membranes is 1–3 kPa, and non-mineralized bone is >34 kPa (Patel et al., 2005; Discher et al., 2009; Chowdhury et al., 2010). Choi and Harley (2012) fabricated a polyacrylamide (PA) hydrogel substrate coated by collagen with varying stiffness to investigate the effect of substrate stiffness on the HSC behaviors. Elastic moduli of the PA hydrogel were adjusted by changing the ratio of the acrylamide and bis-acrylamide polymers to obtain a suitable cross-linking degree. They found that within the stiffness range of 0.01480–0.0442 kPa, varying the stiffness of the substrate, by altering its density, improved the viability of HSCs. The shape and spread area of 32D cells showed a similar trend to than of primary HSPCs when cultured on hydrogel with different stiffness. However, when the stiffness exceeded this range, the viability of the cells decreased with increasing substrate density. Additionally, the spreading of HSCs was enhanced significantly with the increased stiffness of the substrate. The effect of receptor-ligand interactions on HSPC behavior was also quantified, and it was observed that substrate stiffness significantly impacted the cytoskeletal distribution and density of HSPCs. Therefore, accurate control of hydrogel stiffness is important to ensure cell viability. Furthermore, HSCs cultured in a 3D hydrogel environment preserved their naïve state and rounded morphology better than when maintained on top of a 2D surface composed of the same components.
Hydrogel scaffolds containing macropores approximate the “spongy” architecture of natural trabecular bones. The resulting complex internal structure replicates the natural ECM of the hematopoietic niche and promotes the uniform diffusion of soluble factors by regulating their concentration and depletion (Raic et al., 2014). The pores can also aid the propagation of autocrine and paracrine signals involved in cell–cell communication (Raic et al., 2014; Gilchrist et al., 2019a; Gilchrist et al., 2019b). In one study, the poroelestic properties and Young’s modulus (E) of the GelMA hydrogels were controlled by adjusting the photo-initiator concentration and degree of methacrylamide functionalization (DOF). HSPCs and MSCs were both extracted from the tibia and femur BM of mice and were co-cultured in GelMA hydrogels with a high E value. After 7 days of culture, the hematopoietic population increased to the 11.11 ± 3.18% of the total, which is higher than that of a hydrogel with a low E value. This may be related to reduced myeloid differentiation of the cells. The percentage of quiescent (G0) LT-HSCs or HSCs to the initial HSPCs was highest for cells co-cultured in high E value hydrogel. This demonstrates that autocrine and paracrine signaling can be modulated by tuning the seeding density and poroelastic properties of the hydrogel matrix. Small mesh-sized pores resulted in slow diffusion, which reduced cell-to-cell communication, increasing the importance of autocrine regulatory dominated pathways. Under these conditions the quiescence of the primitive HSC population was better preserved (Gilchrist et al., 2019).
The viscoelasticity of hydrogels means that the stress relaxation characteristics of the matrix can be influenced by external pressure. These changes force cells to remodel the matrix through cell–ECM interactions (Tang et al., 2021). Chaudhuri et al. (2016) developed alginate hydrogels with tunable viscoelastic properties to adjust the differentiation of MSCs by modulating the nanoscale architecture of the matrix. They reported that at an elastic modulus of 17 kPa, the hydrogel showed fast stress relaxation and promoted MSCs to differentiate, resulting in the production of a mineralized collagen-1-rich matrix that showed some similarities to the structure of natural bone. Regulation of viscoelasticity can also affect mechanical stresses and substance diffusion (Chaudhuri, 2017). However, to date, most studies have relied on controlling the elasticity of the hydrogel during its preparation, rather than the adjusting the viscoelasticity dynamically (Chaudhuri et al., 2016). The modulation of hydrogel viscoelasticity may provide new insights to aid in the creation of an optimal microenvironment for the growth of HSCs. In the 3D hydrogel microenvironment, liquid movements result in a shear force being exerted over the HSCs (Rodell et al., 2013). Furthermore, hydrogel-based microfluidic systems are in development for high throughput cultures of HSCs. As microdroplets are formed in these systems, shear forces will change (Xia et al., 2017; Zhan et al., 2022). Studies have shown that shear stresses within the hydrogel suspension could reduce the survival of HSCs (Hosseinizand et al., 2016). Mahadik et al. (2014) fabricated a microfluidic multicellular gradient hydrogel platform and observed that the viability of MSCs and HSCs was not significantly influenced by flow characteristics. HSCs are exposed to a certain degree of shear force in their natural niche, so under ideal conditions this would need to be recreated in artificial matrices. Thus, shear forces in hydrogels need to be adjusted to a point where they support cell growth rather than reduce viability.
Zwitterionic materials have attracted attention recently due to their structural similarity to naturally occurring cell membrane lipids. Bai et al. (2019) developed zwitterionic polycarboxybetaine-based hydrogels modified with polypeptide cross-linkers. These exhibited enhanced hydration and swelled up markedly in aqueous solutions, which had an anti-fouling effect and improved biocompatibility (Figure 3C). HSCs cultured in this optimized zwitterionic hydrogel could undergo massive expansion without loss of their stemness (Figure 3D). A systematic study on expansion of primitive human HSCs using a zwitterionic hydrogel reported the construction of a uniform 3D zwitterionic hydrogel network (hereinafter referred to as ZTG hydrogel) through the click chemical reaction between alkyne terminated four arm polycarboxylated betaine acrylamide (pCBAA) and azide modified polypeptide polymerization (Azide–GG-(KE) 20-GPQGIWGQ-(KE) 20-GG Azide) to simulate the 3D environment dominated by hydrophilic and zwitterionic cell membrane lipids of HSPCs in vivo. ZTG hydrogel maintained the high expression of CD34 during the whole culture period, in which 93.7 ± 2% of the expanded cells were CD34+. Substantial expansion of primitive CD34+ BM- and CB-derived HSPCs for 24 days achieved 73-fold of increase in the number of LT-HSCs. The researchers also used limited dilution analysis (LDA) to detect the amplification frequency of medium and LT-HSCs; after 24–30 weeks of transplantation, the amplification frequency of LT-HSCs cultured in ZTOpt mode was much higher than that of other culture methods. Both LDA and secondary transplantation experiments showed that LT-HSC cells with long-term hematopoietic function could be cultured and expanded by ZTG. The LT-HSCs expanded in ZTG hydrogel were injected into immune-deficient NSG mice for primary and secondary BM transplantation. The experimental results showed that in HSPCs derived from CB, CD34+ was effectively amplified in ZTG hydrogel; the expanded HSPCs were used for BM transplantation, which also showed good amplification performance in vivo.
In the past, strategies for the in vitro expansion of HSCs mostly focused on the role of the ECM, while the effects of the metabolism of the HSCs themselves has received very little attention (Kohli and Passegue, 2014). Reactive oxygen species (ROS) produced by HSCs during proliferation is one of the key factors causing overactivation of the cells and results in the loss of hematopoietic potential (Suda et al., 2011). Incorporating ROS scavenging components into hydrogels represents a novel approach to maintain the quiescent status of HSCs. Gilchrist et al. (2021) encapsulated difficult-to-preserve HSPCs in a maleimide-functionalized gelatin (GelMAL) hydrogel containing added dithiol cross-linkers to absorb ROS. Bai et al. (2019) prepared 3D zwitterionic hydrogels that were unique in suppressing excess ROS production by inhibiting oxygen-related metabolism. Cells maintained in this environment showed reduced differentiation, thereby promoting their self-renewal.
4.4 Biomimicking cell–cell interactions
Cell–cell interactions within the hematopoietic niche provide both soluble factors and direct cell–cell contact, which are critical in determining the fate of HSCs (Walenda et al., 2010). Although supplementation with a cocktail of cytokines can increase the expansion of CD34+ HSCs (Li et al., 2019), cell–cell interactions during the co-culture of HSCs and MSCs can synergistically enhance cytokine-induced cell proliferation (Schmal et al., 2016). These observations prompted experiments in which various niche cells, such as MSCs and endothelial cells, were co-cultured with HSCs to investigate how the presence of another cell population affected the maintenance of stemness or the induction of desired lineage differentiation (Xiao et al., 2022). The results showed that MSCs and endothelial cells are the most important feeder cells in HSC cultures, as these cells produce more supporting factors than other BM stromal cells (Xiao et al., 2022). Although MSCs are relatively rare in the BM, they secrete several critical cytokines and growth factors, including CXCL12, which direct chemotaxis, survival, proliferation, and promote SFC. Furthermore, key differentiation factors, such as Flt3-ligand, TPO, and IL-6, are all produced primarily by BM-MSCs (Lo Iacono et al., 2017; Sarvar et al., 2022). Human umbilical vein endothelial cells (HUVECs) have also been used as feeder cells to support the proliferation and differentiation of HSCs in vitro. They express some of the same surface markers and transcription factors found in HSCs, including CD31, CD34, Runx1, and GATA-2 (Kuan et al., 2017; Li et al., 2019).
Co-culturing HSCs with MSCs or HUVECs provides important cell–cell interactions. However, because both MSCs and HUVECs are adherent cells, their interactions with HSCs on a 2D surface are suboptimal (Bello et al., 2018). To improve the cell–cell interactions in co-culturing, hydrogels were used to create an artificial matrix in which adhesion molecules present on MSCs and HUVECs interacted in a 3D spatial area with the HSCs, resulting in improved HSC proliferation (Tibbitt and Anseth, 2009).
Leisten et al. (2012) reported a collagen-based 3D microenvironment for co-culture of human BM or umbilical cord derived progenitor cells (HPCs) with MSCs. MSCs were embedded in the collagen hydrogel (denoted as niche II) and HPCs were seeded in the collagen-free suspension (denoted as niche I). After 14 days culture, HSCs migrated along the collagen fibers niche II and formed cobblestone-like cluster of 10 cells. After 14 days of culture, HPCs in the BM-MSC contained in niche II had a highest number of CD34+ cells, while the lowest number of CD34+ cells was observed in umbilical cord (UC)-MSC niche II, indicating that UC-MSC stimulated HPC differentiation. In niche II, expression of the pan-leukocyte marker CD45, and CD13 were observed, whilst CD56 was poorly expressed in niche II and myeloid differentiation was increased. The researchers established a collagen hydrogel-based artificial HSC niche with two statuses: HPCs in niche I are a proliferative and contained cell population of CD34+/CD38- maturing myeloid cells (CD38+, CD13+, CAE+), while HPCs in niche II contained high numbers of primitive CD34+/CD38- phenotype cells beginning myeloid (CD13+, CAE+) differentiation, resembling the endosteal part of the BM niche. These authors also reported that UC-MSCs were not appropriate feeder cells as they caused extensive differentiation and a complete loss of primitive HSC phenotype (Leisten et al., 2012). Hydrogels can contain a complex diffusion system with an anisotropic distribution of molecules that is more similar to the natural niche, potentially allowing a more precise regulation of cellular behavior (Gurkan et al., 2014). Tuning of the mesh size in hydrogels can precisely regulate cell–cell interactions between co-cultured HSCs and MSCs; this approach allows the balancing of autocrine and paracrine signals (Gilchrist et al., 2019). The co-culture of Lin+ BM niche cells and HSCs in different hydrogel matrices showed that proliferation rate and myeloid differentiation of HSCs closely depended on the rate of diffusion in the matrix (Mahadik et al., 2017). Integrating hydrogels with microfluidic technology resulted in a novel 3D artificial hematopoietic niche (Carreras et al., 2018; Carreras et al., 2021). In this double-layered model, alginate embedded MSCs were place in the middle of hydrogel beads. This core was surrounded by an outer layer of Puramatrix encapsulated HSCs. Figures 3E,F HSCs embedded in these double layered beads maintained their stemness even after 8 weeks of culture (Figure 3G).
4.5 Optimization: Integrating functional nanomaterials
Organic polymers used as hydrogel sources have distinct advantages due to the superior biocompatibility of their natural matrix (Guvendiren and Burdick, 2013). Unfortunately, these polymers lack the necessary physical features and properties to create a viable culture system (Billiet et al., 2012). Recently, inorganic nanomaterials with excellent mechanical strength, abundant functional groups, good optical properties, and a large surface area have gained increasing use in tissue engineering (Billiet et al., 2012). To improve the mechanical properties and extend the application of biologically derived hydrogels in the production of cultured HSCs, organic–inorganic 3D hybrid hydrogels were prepared via the physical or chemical cross-linking of biopolymers with functional nanomaterials in an aqueous phase (Motealleh and Kehr, 2017). These nanomaterial-containing hybrid hydrogels exhibited improved mechanical, electronic, and optical characteristics. Their stimulus-response properties and biocompatibility are also very promising (Gaharwar et al., 2014; Kehr et al., 2015; Motealleh and Kehr, 2017; Sharma et al., 2018; Guillet et al., 2019; Deng et al., 2021).
Zhang P. et al. (2019) fabricated a HAMA hydrogel via the esterification of MA and HA. The macromolecular ROS scavenger, carbon nanotubes (CNTs) were loaded into the HAMA hydrogel by mixing the CNTs suspension with HAMA and LAP in pre-gel solution and followed by a photo-cross-linking. CNT hybrid hydrogels showed better hydrophilicity because of the abundant carboxyl groups from the CNTs. CNTs also improved the modulus of the hydrogels from 5.0 to 7.5 kPa. Isolated CD34+ cells were cultured on the hydrogels supplied with the cytokine cocktail. HSC release of ROS decreases the survival of HSCs and causes the activation of HSCs. In the CNT-loaded HAMA hydrogel, ROS triggers the biodegradation of polysaccharides in the hydrogel, releasing the CNTs to scavenge ROS. The percentage of CD34+CD38− and CD34+ cells increased in the CNT hybrid hydrogel after 10 days of culture. CNT hybrid hydrogels also significantly promoted the total CFU, CFU-GM, and CFU-GEMM compared with non-CNT loaded hydrogels, indicating that integrating nanomaterials with antioxidant properties is beneficial for the hematopoietic function of HSCs. Another study combined methacrylated graphene oxide (MeGO) with HA hydrogels, which significantly increased the water absorption and elastic modulus of the hybrid material. The composite MeGO-HA matrix supported the proliferation of CD34+ cells, whilst maintaining their primitive CD34+CD38− phenotype, especially in a version containing 0.05% w/v MeGO (Guo et al., 2017). To promote the differentiation of HSCs into red blood cells, Brandle et al. (2020) developed an iron nanoparticle composite PEG hydrogel, which ensured the gradual release of iron, inducing some erythroid differentiation. However, they failed to achieve the desired level of differentiation due to iron overload in the 3D PEG-iron nanocomposite scaffolds.
5 Fabrication technologies to create a hydrogel-based artificial niche
5.1 Hydrogel coating on 2D substrates
Culturing HSCs on the 2D surface of polystyrene flasks is still the most widely used traditional culture method (Ribeiro-Filho et al., 2019). This approach has low costs, the flasks are easy to handle and the cells can be harvested with ease (Bello et al., 2018). However, the hydrophobicity of these plastic substrates can induce attachment and the excessive production of ROS, resulting in unintended differentiation and the rapid loss of stemness (Bai et al., 2019; Erathodiyil et al., 2020). It has been demonstrated that culturing cells on plastic surfaces coated with hydrophilic hydrogels inhibited this unnecessary differentiation (Cuchiara et al., 2016; Bai et al., 2019; Liu et al., 2021). Choi and Harley prepared type I collagen-coated polyacrylamide substrates of varying stiffness by altering the total polymer content and the density of cross-linkers. The spreading and morphology of HSCs was significantly influenced by the 2D hydrogel coating of the polyacrylamide surface (Choi and Harley, 2012). However, using a 2D environment for the culture of HSCs is substantially different from the 3D architecture of their natural niche. This leads to a non-physiological flattened spreading pattern with abnormal polarity that causes the loss of their multilineage differentiation potential (Caliari and Burdick, 2016). Compelling evidence suggests that 3D systems enhance cell-microenvironment interactions by improving mechano-sensing by HSCs resulting in increased proliferation and the maintenance of repopulating capacity even after long periods in culture (Cuchiara et al., 2016; Gvaramia et al., 2017; Gilchrist et al., 2019). Thus, current research trends are focusing on the development of optimized 3D hydrogel systems (Jensen and Teng, 2020).
5.2 3D hydrogel encapsulation
To better mimic the 3D hematopoietic microenvironment, the encapsulation of HSCs into a synthetic 3D hydrogel space has been extensively studied (Bello et al., 2018). As mentioned above, these hydrogels can contain natural or synthetic polymers, or a combination of the two. Some of the most commonly utilized natural hydrogels include HA, collagen, alginate, and chitosan, while synthetic hydrogels commonly include PEG, polyethylene oxide (PEO), and poly-l-lactic acid (Maji and Lee, 2022).
Traditional bulk hydrogels are usually relatively large and lack micropores, resulting in slow degradation and limited substance diffusion (Hsu et al., 2019). Micron-sized hydrogels, termed microgels, are expected to solve these problems. These microgels can act as a culture unit for a single cell or may contain a co-culture of multiple cells. Alternatively, the hydrogel can also be assembled into larger scaffolds (Carreras et al., 2018; Caldwell et al., 2020; Carreras et al., 2021). Carreras et al. (2021) reported the use of a droplet microfluidic device contained with double-layered hydrogel beads for the long-term culturing of human HSCs (CD34+ cells). They presented a 3D niche biomimetic model by fabricating an engineered double-layered bead with an alginate-based inner layer and a Puramatrix-based out layer. Numbers of CD34+ cells significantly increased at weeks 6–7, reaching to a maximum level at week 7 of approximately 4-fold that of week 1–2; it then gradually declining at week 8. At week 4, most cells were functional and remained in an undifferentiated status; however, there was a slight CD20 and CD38 signal for lymphoid differentiation, and CD33 signal for myeloid differentiation, as well as CD34+/HLA−DR+ for an intermediate stem cell population. The results indicated this biomimetic niche model had good potential for the long-term culture of HSCs and maintenance on functional cell numbers.
The above methods enabled the microencapsulation of co-cultured HSCs and MSCs with a population distribution approximating that of the natural niche. Encapsulating cells in 3D hydrogels has been demonstrated to regulate stem cell fate decisions by supporting tunable ligand incorporation, the delivery of soluble factors, and mechano-sensing (Gvaramia et al., 2017; Kim et al., 2019; Gilchrist et al., 2021).
5.3 Hydrogel-integrated microfluidic systems
Microfluidic technology has emerged as a powerful tool in the reconstruction of the cellular microenvironment. This approach enables the creation of micro-sized droplets matching the diameter of the cells and permits the precise microscale manipulation of fluid flow and the modulation of the concentration of various factors (Whitesides, 2006; Sackmann et al., 2014; An et al., 2019). However, conventional microfluidics manipulations usually take place in 2D environments (An et al., 2019). Incorporating hydrogel matrices into microfluidic systems enables the construction of 3D spatial environments and the provision of different biophysical and biochemical features mimicking the natural microenvironment (Liu et al., 2019).
The use of microfluidic systems can rapidly establish a concentration gradient of various chemical compounds, facilitating the precise control of culture conditions and experimental investigation of the effects of individual factors (An et al., 2019). Chemical gradients are created in microfluidic channels via both flow and diffusion (Kim et al., 2010). T- or Y-shaped channels also allow the mixing of two fluid streams entering from two separate inlets and converging at the intersection (An et al., 2019). Mahadik et al. (2015) reported the use of the PEG-SCF functionalized GelMA hydrogel; PEG functionalized SCF retains the natural biological activity of SCF, and >80% of PEG functionalized SCF can be stably incorporated and retained in the GelMA hydrogel within 7 days. Mouse BM HSCs were cultured in hydrogels with four SCF presentation modes (No SCF, transient SCF, continuous SCF, concurrent SCF). The effects of continuous and covalent SCF presentation patterns on the fate of HSCs were examined by surface antigen expression and CFU analysis. After 7 days, the total number of cells under both conditions increased significantly; however, the maintenance of LSK and CD34−LSK components decreased significantly under continuous SCF conditions, while in the hydrogel containing covalently fixed SCF, they remained unchanged over time. In GelMA hydrogel containing covalent fixation, the CFU-GEMM score was significantly higher than that of continuous SCF, indicating that the maintenance of more primitive hematopoietic progenitor cell population was improved. They also mixed two pre-polymer solutions (5% w/v GelMA; 5% w/v GelMA + 400 ng/ml PEG-SCF) through a microfluidic device using a computer-controlled syringe pump to form a GelMA hydrogel containing a covalently fixed SCF gradient, and observed that HSCs expanded with the SCF content gradient. SCF embedded in this 3D environment promoted the proliferation and the maintenance of primitive HSCs in a dose-dependent manner.
In addition to chemical gradients, microfluidics can also generate cellular gradients to mimic the complex multicellular microenvironment. Mahadik et al. (2014) used a traditional staggered herringbone shaped microfluidic mixer to generate a 3D collagen hydrogel containing adjustable, reverse gradient cells and biomaterial properties from two hydrogel precursor suspensions. To check the effect of flow through the mixer on cell viability, a 1-mg/ml collagen suspension containing MC3T3-E1 osteoblasts or mouse BM LSK cells was directly put into the glass dish (control) or entered the dish through the microfluidic mixer (device). For these 2 cell types, high relative viability was observed in the first 24 h of culture, which remained high after 3 days of culture (>60%). The mixer was then used to prepare the reverse gradient hydrogel of the above 2 cells in the 1 mg/ml collagen hydrogel. The relative gradient (2:1–1:2) of LSK and osteoblasts was quantified by fluorescence analysis of the whole structure. The relative gradient of mixed cells was similar to that of single cell hydrogel with opposite gradient, indicating that the relative gradient of mixed cells was generated. Single LSK and Lin+(GFP+) BM cells mixed in a single hydrogel were imaged using a multiphoton microscope. The spectral deconvolution method could identify LSK cells from mature BM (Lin+) cells. The multiple gradient hydrogel constructs developed here provide the potential for HSC fate manipulation and tracking analysis in 3D microenvironment models, and will become a powerful tool for studying cell–microenvironment interactions (Choi et al., 2015).
The human organ chip is a transformative biomedical technology that was voted as one of the “Top Ten Emerging Technologies” in 2016 (Domingues et al., 2017). In one application, scientists established a BM on-a-chip based on the integrations of hydrogels and a microfluidic system. The hydrogel element contained various encapsulated niche cells (Sieber et al., 2018; Chatterjee et al., 2021). This BM on-a-chip approach represents a sophisticated model of the living BM with cellular diversity and complex functionality, and was subsequently approved by the Food and Drug Administration (Liu et al., 2019). BM toxicity, radiation resistance, and drug testing can be conducted using these artificial BM models, replacing experimentation on animals (Chou et al., 2020).
These organ on-a-chip models closely approximate the environment of the natural BM. Torisawa et al. (2014) developed a BM on-a-chip system and tested whether HSCs cultured on these chips can maintain their capacity to self-renew and differentiate into multiple lineages. They fabricated a polydimethylsiloxane microdevice contained a central cavity filled with collagen, bone morphogenetic proteins, and demineralized bone powder. These devices were implanted into mice for a period of 4–8 weeks. On removal, the central cavity contained a well-developed BM compartment. These recovered BM chips were transferred into a microfluidic system for further observation (Figure 4A). After 7 days of in vitro culture, the amount and distribution of HSPCs and HSCs remained similar to those of the fresh BM (Torisawa et al., 2014) (Figure 4B). Aleman et al. (2019) created another version of the BM on-a-chip; the HA and gelatin hydrogel microenvironment was subdivided into four major hematopoietic areas, a periarterial, a perisinusoidal, a mesenchymal, and an osteoblastic niche (Figures 4C,D). In this system, healthy and malignant human HSCs showed unique and selective homing behavior into specific niches (Figure 4E). Chou et al. (2020) created a vascularized human BM on-a-chip system that consisted of two chambers connected by a porous membrane. The top “hematopoietic” chamber, representing the intraosseous niche, was filled with human CD34+ HSCs and BM-derived MSCs in fibrin gels. The bottom “vascular” chamber was lined by HUVECs and perfused with culture medium containing supportive cytokines (Figure 4F). Culturing HSCs within this in vitro model preserved stemness and supported myeloerythroid proliferation and differentiation (Chou et al., 2020). Research on the validation of the effectiveness of HSCs developed in these artificial environments is currently being carried out in living animals. The HSC populations expanded in the engineered environments in vitro are transplanted into mice for evaluation of their safety and their ability to reconstitute BM function (Baena et al., 2021). It is anticipated that future BM on-a-chip systems will be sophisticated enough to allow the validation and characterization of in vitro expanded HSCs without the need to sacrifice live animals.
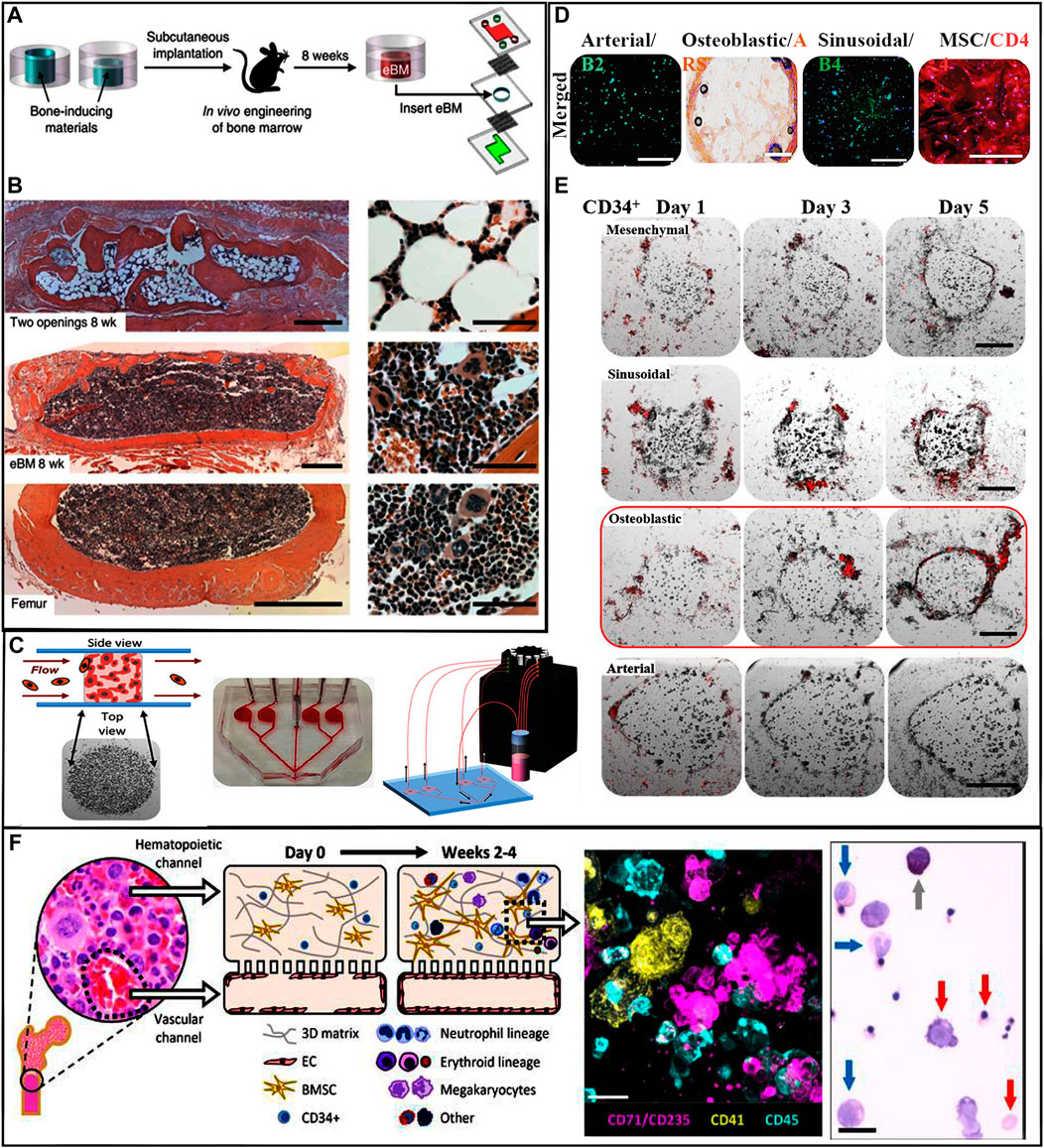
FIGURE 4. (A) Schematic of workflow to fabricate BM on-a-chip, in which eBM is formed in mouse and is then cultured in a microfluidic system. (B) Images show the establishment of eBM with in vivo-like histological BM structure. (Top) Hematoxylin-and-eosin-stained sections of the post-implanted eBM formed in the PDMS device with two opening. (Center) Histological sections of the post-implanted eBM formed in the PDMS device with one lower opening. (Bottom) Cross-section of BM in a normal adult mouse femur. Scale bars: 500 and 50 µm for low and high magnification views, respectively. (Torisawa et al., 2014) Figure 4A, B: Copyright © 2014. Reproduced with permission from Springer Nature. (C) Niche-on-chip microfluidic chip. (Left) Side and top view depictions of the niche constructs in the device chambers. (Center) Schematic of multi-niche-on-chip device. (Right) Schematic of operational recirculating multi-niche-on-a-chip systems. (D) Live/dead viability staining of each niche construct type. Scale bars: 100 µm. (E) Fluorescent images of niche-on-a-chip lodging/retention experiments using CD34+ HSCs. Scale bar: 250 µm. (Aleman et al., 2019) Figure 4C-E: Copyright © 2019. Reproduced with permission from John Wiley and Sons. (F) Schematic illustration of the vascularized BM on-a-chip with CD34+ progenitors and MSCs in the top gel channel and a vascular lining in the bottom channel. Immunofluorescence image of a vertical cross-section through the upper channel of the BM chip. Scale bar: 20 µm. Wright-Giemsa staining image of cells from the BM chip showing multilineage differentiation. Scale bar: 20 µm. (Chou et al., 2020). Copyright © 2020. Reproduced with permission from Springer Nature.
5.4 Stimulus-responsive hydrogel-based multifunctional hematopoietic stem cell culture platform
Stimulus-responsive hydrogels are promising tools that can sense signals coming from niche cells and/or cytokines in the matrix and respond to these signals by changes in conductive, chemical, or temperature characteristics. These “intelligent” microenvironments can adapt to the requirements of the cells growing within (Jiang et al., 2020; Deng et al., 2021). For example, an enzyme-responsive 3D hydrogel was developed containing conjugated polypeptides that could be hydrolyzed by the matric metalloproteases (MMPs) secreted by encapsulated HSCs. As HSC numbers expanded, their increased cumulative MMP production gradually cleaved the polypeptides in the matrix, facilitating the swelling of the hydrogel, thus providing a larger space for further increases in HSC number. In addition, the enzyme sensitive nature of the hydrogel also simplified the rapid harvesting of cells. By exposing the whole hydrogel compartment to exogenous MMPs, this resulted in the degradation of the matrix, so the encased cells could be easily collected (Bai et al., 2019). Although hydrogel-based 3D environments allow the successful expansion of HSCs, harvesting the encapsulated cells remains challenging (Bodenberger et al., 2017; Yin and Cao, 2021). Commonly the expanded cells are collected after mechanical disassembly or chemical dissociation of the matrix. However, these processes can damage the cells. Kwak and Lee, 2020 reported an inverted colloidal crystal hydrogel scaffold that was created from poly(N-isopropylacrylamide). The volume of this hydrogel shrank or swelled significantly depending on changes in the temperature within a physiological range (4–37°C). Due to this reversible thermoresponsive behavior of the hydrogel spheroids containing embedded cells could be created in a single step and the expanded cells could be rapidly recovered afterwards by controlling the volume of the hydrogel. By changing the temperature, the encapsulated HSCs could be selectively separated from spheroids.
Stimulus responsive hydrogels can be combined with biosensor technology to directly monitor key markers of living cells cultured in the biomimetic environment. This approach allows for the long-term tracking and monitoring of the encapsulated cells (Jiang et al., 2020; Deng et al., 2021). Lee et al., 2016 reported a 3D capacitance biosensor that worked in alginate hydrogels containing MSCs. This system allowed the real time monitoring of cell proliferation, migration, and differentiation, by measuring dynamic changes in capacitance and conductance. Because HSCs can easily lose their stemness during in vitro culture, the ability to monitor their differentiation and stemness markers, preferably in real time, has considerable advantages (De Leon et al., 2020; Allenby and Woodruff, 2022).
While current hydrogel methods enable the massive expansion of encapsulated cells, they are seldom equipped to sense and monitor cell characteristics (Fedi et al., 2022). Instead, the detection of biomarkers still relies on conventional fluorescence or immunological detection techniques requiring the extraction of cells from the matrix. Under these conditions, the real-time state of the bionic culture environment cannot be continuously monitored (Lim et al., 2007; Gilchrist et al., 2021). Stimulus-responsive hydrogels will provide new approaches in developing a comprehensive platform for the expansion and simultaneous monitoring of the state of encapsulated HSCs, potentially optimizing yield and quality.
6 Prospects and challenges
Hydrogel biomaterials show excellent biosafety, offer flexible possibilities for chemical modifications and biofunctionalization, and have been successfully used for the in vitro expansion of primitive HSCs. A combination of various chemical modifications, the addition of biomaterials, and advancements in technologies—such as microfluidics and organ on-a-chip—make it possible to establish hydrogel-based microenvironments with exquisite architecture and adjustable physical features that closely mimic the natural hematopoietic niche. Aside from their clinical relevance, these systems also facilitate studies on the behavior and fate of HSCs. However, due to the extraordinarily complex physiology of the natural hematopoietic niche, replicating it in vitro using engineered hydrogel-based systems still holds many challenges. Various features of the natural hematopoietic environment, such as the presence of other cells, biochemical cues, varying stiffness, and topography, are distributed in a heterogeneous environment and direct the behavior and fate of HSCs though multiple mechanisms. In contrast, most hydrogel-based culture systems exhibit isotropy and lack the anisotropic properties of the tissue microenvironment. Although the integration of hydrogels in microfluidic systems enabled the dynamic co-existence of two distinct cell populations, the gap between these and the natural niche remains wide. BM on-a-chip technology is a promising and powerful tool for ex-vivo HSC culture and the monitoring and scientific exploration of the hematopoietic process. However, in their current iteration, they can only accommodate no more than two distinct cell populations. In addition, their complex manufacturing and high costs currently limit their large-scale production for clinical applications.
In general, hydrogel have uniform internal structures, which differs significantly from the architecture of a natural niche (Zimmermann et al., 2019). 3D printing of biomaterials is powerful emerging tool for the fabrication of sophisticated and highly organized 3D hydrogel structures (Ong et al., 2018; Olate-Moya et al., 2020; Moore et al., 2021). Composite hydrogels with enhanced shape fidelity can facilitate the printing of scaffolds with mechanical and architectural details closely resembling the features of the in vivo niche (Zhou et al., 2020). A composite hydrogel composed of 4% methylcellulose and 2% alginate that also contained encapsulated BM niche cells was used to bioprint a scaffold to mimic the perivascular area of the BM (Moore et al., 2021). However, 3D printing for artificial niche mimicking is a new technology that requires further exploration.
The natural hematopoietic niche contains a viscoelastic matrix, while most hydrogels are primarily elastic materials. These artificial matrices cannot relax under strain forces. In contrast, the natural viscoelastic matrix is mechanically yielding, and the matrix can be dynamically remodeled. The development of better viscoelastic hydrogels, replicating the conditions for cell–ECM interactions in vivo, could significantly improve the outcomes of culturing HSCs in vitro.
7 Conclusion
HSCs from CB play an important role in clinical transplantation. CB provides an alternative source for allogeneic HSCT patients lacking HLA-matched donors (Rocha, 2016). Compared with BM and mobilized peripheral blood stem cells, CB has lower requirements on HLA matching, increased donor availability, and low rates of chronic GVHD. However, due to the limited number of HSCs and HSPCs within a single umbilical CB unit, the implantation of neutrophils is delayed, which increases the risk of early infection and transplant-related mortality. Therefore, increasing research has been devoted to the exploration of new techniques for functional HSC expansion in vitro. However, there are very limited to normal HSCs that undergo asymmetric mitosis. HSCs are very sensitive to the environment, which means after leaving the niche, stimulation from the external environment, such as mechanical force or redox reaction, can easily cause their differentiation and loss of the expression of original phenotype markers.
In the previous, Section 2, we discussed the complexity of the BM niche and the correlation of several elements of this niche in HSC physiology. Recent HSC culture methods such as adding cytokines, small molecule compounds and perfusion reactor in the laboratory, have been introduced. In addition, building a 3D culture environment that is closer to the HSC niche in terms of space and biological interaction has gradually become a new trend in the study of HSC expansion in vitro. The chemical and engineering strategies and techniques for improving the niche of biomimetic hematopoiesis were summarized. The potential of stimulus-responsive hydrogels in the establishment of an intelligent microenvironment was also described. Finally, the remaining challenges in the use of the hydrogel culture platform and the broad prospects for development were reviewed. The improved artificial microenvironment for HSC amplification in vitro and the bionic BM for testing the safety and effectiveness of HSC treatment are exciting developments, providing new solutions for the increasing use of hydrogel-based HSC culture platforms in clinical applications and scientific research. However, due to the complexity of the niche, in the current technology, it remains difficult to artificially reconstruct all these elements into functional BM. The development of an effective bioengineering strategy requires further research on the molecular mechanism of HSC behavior in the niche and the progression of biomaterial technology.
Author contributions
XH: conceptualization, methodology, writing-original draft, data curation, and writing-review; YW: writing-original draft and writing-review; FW: supervision and funding acquisition; TW: writing-review; SL: investigation, supervision, and funding acquisition; GO: conceptualization, supervision, writing-reviewing and editing.
Funding
This work was supported by Shenzhen Fund for Guangdong Provincial High-Level Clinical Key Specialties (No. SZGSP012) Shenzhen Key Medical Discipline Construction Fund (No. SZXK034) and Guangdong High-level Hospital Construction Fund.
Conflict of interest
The authors declare that the research was conducted in the absence of any commercial or financial relationships that could be construed as a potential conflict of interest.
Publisher’s note
All claims expressed in this article are solely those of the authors and do not necessarily represent those of their affiliated organizations, or those of the publisher, the editors and the reviewers. Any product that may be evaluated in this article, or claim that may be made by its manufacturer, is not guaranteed or endorsed by the publisher.
References
Acar, M., Kocherlakota, K. S., Murphy, M. M., Peyer, J. G., Oguro, H., Inra, C. N., et al. (2015). Deep imaging of bone marrow shows non-dividing stem cells are mainly perisinusoidal. Nature 526 (7571), 126–130. doi:10.1038/nature15250
Afewerki, S., Sheikhi, A., Kannan, S., Ahadian, S., and Khademhosseini, A. (2019). Gelatin-polysaccharide composite scaffolds for 3D cell culture and tissue engineering: Towards natural therapeutics. Bioeng. Transl. Med. 4 (1), 96–115. doi:10.1002/btm2.10124
Ahmed, E. M. (2015). Hydrogel: Preparation, characterization, and applications: A review. J. Adv. Res. 6 (2), 105–121. doi:10.1016/j.jare.2013.07.006
Aleman, J., George, S. K., Herberg, S., Devarasetty, M., Porada, C. D., Skardal, A., et al. (2019). Deconstructed microfluidic bone marrow on-a-Chip to study normal and malignant hemopoietic cell-niche interactions. Small 15 (43), 1902971. doi:10.1002/smll.201902971
Allenby, M. C., and Woodruff, M. A. (2022). Image analyses for engineering advanced tissue biomanufacturing processes. Biomaterials 284, 121514. doi:10.1016/j.biomaterials.2022.121514
An, S., Han, S. Y., and Cho, S. W. (2019). Hydrogel-integrated microfluidic systems for advanced stem cell engineering. BioChip J. 13 (4), 306–322. doi:10.1007/s13206-019-3402-5
Arai, F., Hirao, A., Ohmura, M., Sato, H., Matsuoka, S., Takubo, K., et al. (2004). Tie2/Angiopoietin-1 signaling regulates hematopoietic stem cell quiescence in the bone marrow niche. Cell 118 (2), 149–161. doi:10.1016/j.cell.2004.07.004
Asada, N., Katayama, Y., Sato, M., Minagawa, K., Wakahashi, K., Kawano, H., et al. (2013). Matrix-embedded osteocytes regulate mobilization of hematopoietic stem/progenitor cells. Cell Stem Cell 12 (6), 737–747. doi:10.1016/j.stem.2013.05.001
Asada, N., Kunisaki, Y., Pierce, H., Wang, Z., Fernandez, N. F., Birbrair, A., et al. (2017). Differential cytokine contributions of perivascular haematopoietic stem cell niches. Nat. Cell Biol. 19 (3), 214–223. doi:10.1038/ncb3475
Baena, A. R. Y., Manso, B. A., and Forsberg, E. C. (2021). Cfu-S assay: A historical single-cell assay that offers modern insight into clonal hematopoiesis. Exp. Hematol. 104, 1–8. doi:10.1016/j.exphem.2021.10.003
Bai, T., Li, J., Sinclair, A., Imren, S., Merriam, F., Sun, F., et al. (2019). Expansion of primitive human hematopoietic stem cells by culture in a zwitterionic hydrogel. Nat. Med. 25 (10), 1566–1575. doi:10.1038/s41591-019-0601-5
Ballen, K. K., Gluckman, E., and Broxmeyer, H. E. (2013). Umbilical cord blood transplantation: The first 25 Years and beyond. Blood 122 (4), 491–498. doi:10.1182/blood-2013-02-453175
Bao, Z., Xian, C., Yuan, Q., Liu, G., and Wu, J. (2019). Natural polymer-based hydrogels with enhanced mechanical performances: Preparation, structure, and property. Adv. Healthc. Mat. 8 (17), 1900670. doi:10.1002/adhm.201900670
Barnhouse, V., Petrikas, N., Crosby, C., Zoldan, J., and Harley, B. (2021). Perivascular secretome influences hematopoietic stem cell maintenance in a gelatin hydrogel. Ann. Biomed. Eng. 49 (2), 780–792. doi:10.1007/s10439-020-02602-0
Bello, A. B., Park, H., and Lee, S. H. (2018). Current approaches in biomaterial-based hematopoietic stem cell niches. Acta Biomater. 72, 1–15. doi:10.1016/j.actbio.2018.03.028
Bhaskar, B., Owen, R., Bahmaee, H., Rao, P. S., and Reilly, G. C. (2018). Design and assessment of a dynamic perfusion bioreactor for large bone tissue engineering scaffolds. Appl. Biochem. Biotechnol. 185 (2), 555–563. doi:10.1007/s12010-017-2671-5
Billiet, T., Vandenhaute, M., Schelfhout, J., Van Vlierberghe, S., and Dubruel, P. (2012). A review of trends and limitations in hydrogel-rapid prototyping for tissue engineering. Biomaterials 33 (26), 6020–6041. doi:10.1016/j.biomaterials.2012.04.050
Bodenberger, N., Kubiczek, D., Trösch, L., Gawanbacht, A., Wilhelm, S., Tielker, D., et al. (2017). Lectin-mediated reversible immobilization of human cells into a glycosylated macroporous protein hydrogel as a cell culture matrix. Sci. Rep. 7, 6151. doi:10.1038/s41598-017-06240-w
Bourgine, P. E., Klein, T., Paczulla, A. M., Shimizu, T., Kunz, L., Kokkaliaris, K. D., et al. (2018). In vitro biomimetic engineering of a human hematopoietic niche with functional properties. Proc. Natl. Acad. Sci. U. S. A. 115 (25), E5688–e5695. doi:10.1073/pnas.1805440115
Bovone, G., Dudaryeva, O. Y., Marco-Dufort, B., and Tibbitt, M. W. (2021). Engineering hydrogel adhesion for biomedical applications via chemical design of the junction. ACS Biomater. Sci. Eng. 7 (9), 4048–4076. doi:10.1021/acsbiomaterials.0c01677
Bowers, E., Slaughter, A., Frenette, P. S., Kuick, R., Pello, O. M., and Lucas, D. (2018). Granulocyte-derived TNFα promotes vascular and hematopoietic regeneration in the bone marrow. Nat. Med. 24 (1), 95–102. doi:10.1038/nm.4448
Brandle, K., Bergmann, T. C., Raic, A., Li, Y., Million, N., Rehbock, C., et al. (2020). Iron nanoparticle composite hydrogels for studying effects of iron ion release on red blood cell in vitro production. ACS Appl. Bio Mat. 3 (8), 4766–4778. doi:10.1021/acsabm.0c00297
Bruns, I., Lucas, D., Pinho, S., Ahmed, J., Lambert, M. P., Kunisaki, Y., et al. (2014). Megakaryocytes regulate hematopoietic stem cell quiescence through CXCL4 secretion. Nat. Med. 20 (11), 1315–1320. doi:10.1038/nm.3707
Caldwell, A. S., Aguado, B. A., and Anseth, K. S. (2020). Designing microgels for cell culture and controlled assembly of tissue microenvironments. Adv. Funct. Mat. 30 (37), 1907670. doi:10.1002/adfm.201907670
Caliari, S. R., and Burdick, J. A. (2016). A practical guide to hydrogels for cell culture. Nat. Methods 13 (5), 405–414. doi:10.1038/nmeth.3839
Calvi, L. M., Adams, G. B., Weibrecht, K. W., Weber, J. M., Olson, D. P., Knight, M. C., et al. (2003). Osteoblastic cells regulate the haematopoietic stem cell niche. Nature 425 (6960), 841–846. doi:10.1038/nature02040
Cao, H., Cao, B., Heazlewood, C. K., Domingues, M., Sun, X., Debele, E., et al. (2019). Osteopontin is an important regulative component of the fetal bone marrow hematopoietic stem cell niche. Cells 8 (9), 985. doi:10.3390/cells8090985
Carreras, P., Chaves, R. C., Gallardo, M., Ortiz, A., Lopez, J. M., and Sia, S. K. (2018). Microengineering double layer hydrogel structures towards the recapitulation of the hematopoietic stem cell niche. Sci. Bull. (Beijing). 63 (20), 1319–1323. doi:10.1016/j.scib.2018.09.003
Carreras, P., González, I., Gallardo, M., Ortiz-Ruiz, A., Morales, M. L., Encinas, J., et al. (2021). Long-term human hematopoietic stem cell culture in microdroplets. Micromachines 12 (1), 90. doi:10.3390/mi12010090
Casanova-Acebes, M., Pitaval, C., Weiss, L. A., Nombela-Arrieta, C., Chèvre, R., N, A. G., et al. (2013). Rhythmic modulation of the hematopoietic niche through neutrophil clearance. Cell 153 (5), 1025–1035. doi:10.1016/j.cell.2013.04.040
Chatterjee, C., Schertl, P., Frommer, M., Ludwig-Husemann, A., Mohra, A., Dilger, N., et al. (2021). Rebuilding the hematopoietic stem cell niche: Recent developments and future prospects. Acta Biomater. 132, 129–148. doi:10.1016/j.actbio.2021.03.061
Chaudhuri, O., Gu, L., Klumpers, D., Darnell, M., Bencherif, S. A., Weaver, J. C., et al. (2016). Hydrogels with tunable stress relaxation regulate stem cell fate and activity. Nat. Mat. 15 (3), 326–334. doi:10.1038/nmat4489
Chaudhuri, O. (2017). Viscoelastic hydrogels for 3D cell culture. Biomater. Sci. 5 (8), 1480–1490. doi:10.1039/c7bm00261k
Chimene, D., Kaunas, R., and Gaharwar, A. K. (2020). Hydrogel bioink reinforcement for additive manufacturing: A focused review of emerging strategies. Adv. Mat. 32 (1), 1902026. doi:10.1002/adma.201902026
Choi, J. S., and Harley, B. A. (2012). The combined influence of substrate elasticity and ligand density on the viability and biophysical properties of hematopoietic stem and progenitor cells. Biomaterials 33 (18), 4460–4468. doi:10.1016/j.biomaterials.2012.03.010
Choi, J. S., Mahadik, B. P., and Harley, B. A. (2015). Engineering the hematopoietic stem cell niche: Frontiers in biomaterial science. Biotechnol. J. 10 (10), 1529–1545. doi:10.1002/biot.201400758
Chou, D. B., Frismantas, V., Milton, Y., David, R., Pop-Damkov, P., Ferguson, D., et al. (2020). On-chip recapitulation of clinical bone marrow toxicities and patient-specific pathophysiology. Nat. Biomed. Eng. 4 (4), 394–406. doi:10.1038/s41551-019-0495-z
Chow, A., Lucas, D., Hidalgo, A., Méndez-Ferrer, S., Hashimoto, D., Scheiermann, C., et al. (2011). Bone marrow CD169+ macrophages promote the retention of hematopoietic stem and progenitor cells in the mesenchymal stem cell niche. J. Exp. Med. 208 (2), 261–271. doi:10.1084/jem.20101688
Chowdhury, F., Na, S., Li, D., Poh, Y. C., Tanaka, T. S., Wang, F., et al. (2010). Material properties of the cell dictate stress-induced spreading and differentiation in embryonic stem cells. Nat. Mat. 9 (1), 82–88. doi:10.1038/nmat2563
Christodoulou, C., Spencer, J. A., Yeh, S. A., Turcotte, R., Kokkaliaris, K. D., Panero, R., et al. (2020). Live-animal imaging of native haematopoietic stem and progenitor cells. Nature 578 (7794), 278–283. doi:10.1038/s41586-020-1971-z
Cohen, S., Roy, J., Lachance, S., Delisle, J. S., Marinier, A., Busque, L., et al. (2020). Hematopoietic stem cell transplantation using single um171-expanded cord blood: A single-arm, phase 1-2 safety and feasibility study. Lancet Haematol. 7 (2), e134–e145. doi:10.1016/s2352-3026(19)30202-9
Conneally, E., Cashman, J., Petzer, A., and Eaves, C. (1997). Expansion in vitro of transplantable human cord blood stem cells demonstrated using a quantitative assay of their lympho-myeloid repopulating activity in nonobese diabetic-scid/scid mice. Proc. Natl. Acad. Sci. U. S. A. 94 (18), 9836–9841. doi:10.1073/pnas.94.18.9836
Costa, B. N. L., Adão, R. M. R., Maibohm, C., Accardo, A., Cardoso, V. F., and Nieder, J. B. (2022). Cellular interaction of bone marrow mesenchymal stem cells with polymer and hydrogel 3D microscaffold templates. ACS Appl. Mat. Interfaces 14 (11), 13013–13024. doi:10.1021/acsami.1c23442
Crane, G. M., Jeffery, E., and Morrison, S. J. (2017). Adult haematopoietic stem cell niches. Nat. Rev. Immunol. 17 (9), 573–590. doi:10.1038/nri.2017.53
Cuchiara, M. L., Coskun, S., Banda, O. A., Horter, K. L., Hirschi, K. K., and West, J. L. (2016). Bioactive poly (ethylene glycol) hydrogels to recapitulate the HSC niche and facilitate HSC expansion in culture. Biotechnol. Bioeng. 113 (4), 870–881. doi:10.1002/bit.25848
Cuchiara, M. L., Horter, K. L., Banda, O. A., and West, J. L. (2013). Covalent immobilization of stem cell factor and stromal derived factor 1α for in vitro culture of hematopoietic progenitor cells. Acta Biomater. 9 (12), 9258–9269. doi:10.1016/j.actbio.2013.08.012
De Leon, S. E., Pupovac, A., and McArthur, S. L. (2020). Three-dimensional (3D) cell culture monitoring: Opportunities and challenges for impedance spectroscopy. Biotechnol. Bioeng. 117 (4), 1230–1240. doi:10.1002/bit.27270
Delaney, C., Heimfeld, S., Brashem-Stein, C., Voorhies, H., Manger, R. L., and Bernstein, I. D. (2010). Notch-mediated expansion of human cord blood progenitor cells capable of rapid myeloid reconstitution. Nat. Med. 16 (2), 232–236. doi:10.1038/nm.2080
Delaney, C., Varnum-Finney, B., Aoyama, K., Brashem-Stein, C., and Bernstein, I. D. (2005). Dose-dependent effects of the Notch ligand Delta1 on ex vivo differentiation and in vivo marrow repopulating ability of cord blood cells. Blood. 106 (8), 2693–2699. doi:10.1182/blood-2005-03-1131
Deng, Z., Yu, R., and Guo, B. (2021). Stimuli-responsive conductive hydrogels: Design, properties, and applications. Mat. Chem. Front. 5 (5), 2092–2123. doi:10.1039/d0qm00868k
Ding, Y., Gao, S., Shen, J., Bai, T., Yang, M., Xu, S., et al. (2020). TNFSF15 facilitates human umbilical cord blood haematopoietic stem cell expansion by activating Notch signal pathway. J. Cell. Mol. Med. 24 (19), 11146–11157. doi:10.1111/jcmm.15626
Discher, D. E., Mooney, D. J., and Zandstra, P. W. (2009). Growth factors, matrices, and forces combine and control stem cells. Science 324 (5935), 1673–1677. doi:10.1126/science.1171643
Domingues, M. J., Cao, H., Heazlewood, S. Y., Cao, B., and Nilsson, S. K. (2017). Niche extracellular matrix components and their influence on HSC. J. Cell. Biochem. 118 (8), 1984–1993. doi:10.1002/jcb.25905
Echalier, C., Jebors, S., Laconde, G., Brunel, L., Verdie, P., Causse, L., et al. (2017). Sol-gel synthesis of collagen-inspired peptide hydrogel. Mat. TodayKidlingt. 20 (2), 59–66. doi:10.1016/j.mattod.2017.02.001
Erathodiyil, N., Chan, H. M., Wu, H., and Ying, J. Y. (2020). Zwitterionic polymers and hydrogels for antibiofouling applications in implantable devices. Mat. TodayKidlingt. 38, 84–98. doi:10.1016/j.mattod.2020.03.024
Fares, I., Chagraoui, J., Gareau, Y., Gingras, S., Ruel, R., Mayotte, N., et al. (2014). Pyrimidoindole derivatives are agonists of human hematopoietic stem cell self-renewal. Science 345 (6203), 1509–1512. doi:10.1126/science.1256337
Fedi, A., Vitale, C., Giannoni, P., Caluori, G., and Marrella, A. (2022). Biosensors to monitor cell activity in 3D hydrogel-based tissue models. Sensors 22 (4), 1517. doi:10.3390/s22041517
Fujisaki, J., Wu, J., Carlson, A. L., Silberstein, L., Putheti, P., Larocca, R., et al. (2011). In vivo imaging of Treg cells providing immune privilege to the haematopoietic stem-cell niche. Nature 474 (7350), 216–219. doi:10.1038/nature10160
Gaharwar, A. K., Peppas, N. A., and Khademhosseini, A. (2014). Nanocomposite hydrogels for biomedical applications. Biotechnol. Bioeng. 111 (3), 441–453. doi:10.1002/bit.25160
Gao, X., Zhang, D., Xu, C., Li, H., Caron, K. M., and Frenette, P. S. (2021). Nociceptive nerves regulate haematopoietic stem cell mobilization. Nature 589 (7843), 591–596. doi:10.1038/s41586-020-03057-y
García-García, A., Korn, C., García-Fernández, M., Domingues, O., Villadiego, J., Martín-Pérez, D., et al. (2019). Dual cholinergic signals regulate daily migration of hematopoietic stem cells and leukocytes. Blood 133 (3), 224–236. doi:10.1182/blood-2018-08-867648
Gilchrist, A. E., Lee, S., Hu, Y., and Harley, B. A. (2019). Soluble signals and remodeling in a synthetic gelatin-based hematopoietic stem cell niche. Adv. Healthc. Mat. 289553, e1900751. doi:10.1002/adhm.201900751
Gilchrist, A. E., Serrano, J. F., Ngo, M. T., Hrnjak, Z., Kim, S., and Harley, B. A. C. (2021). Encapsulation of murine hematopoietic stem and progenitor cells in a thiol-crosslinked maleimide-functionalized gelatin hydrogel. Acta Biomater. 131, 138–148. doi:10.1016/j.actbio.2021.06.028
Gjorevski, N., Sachs, N., Manfrin, A., Giger, S., Bragina, M. E., Ordóñez-Morán, P., et al. (2016). Designer matrices for intestinal stem cell and organoid culture. Nature 539 (7630), 560–564. doi:10.1038/nature20168
Greenbaum, A., Hsu, Y.-M. S., Day, R. B., Schuettpelz, L. G., Christopher, M. J., Borgerding, J. N., et al. (2013). CXCL12 in early mesenchymal progenitors is required for haematopoietic stem-cell maintenance. Nature 495 (7440), 227–230. doi:10.1038/nature11926
Guillet, J. F., Valdez-Nava, Z., Golzio, M., and Flahaut, E. (2019). Electrical properties of double-wall carbon nanotubes nanocomposite hydrogels. Carbon 146, 542–548. doi:10.1016/j.carbon.2019.01.090
Guo, W., Pan, X., Zhang, Y., Cai, H., and Zhang, W. (2017). A hyaluronic acid/graphene oxide hydrogel for enhanced ex vivo expansion of cord blood-derived CD34+ cells. Mat. Lett. 205, 253–256. doi:10.1016/j.matlet.2017.06.023
Gurkan, U. A., El Assal, R., Yildiz, S. E., Sung, Y., Trachtenberg, A. J., Kuo, W. P., et al. (2014). Engineering anisotropic biomimetic fibrocartilage microenvironment by bioprinting mesenchymal stem cells in nanoliter gel droplets. Mol. Pharm. 11 (7), 2151–2159. doi:10.1021/mp400573g
Guvendiren, M., and Burdick, J. A. (2013). Engineering synthetic hydrogel microenvironments to instruct stem cells. Curr. Opin. Biotechnol. 24 (5), 841–846. doi:10.1016/j.copbio.2013.03.009
Gu, Y. C., Kortesmaa, J., Tryggvason, K., Persson, J., Ekblom, P., Jacobsen, S. E., et al. (2003). Laminin Isoform-Specific Promotion of Adhesion and Migration of Human Bone Marrow Progenitor Cells. Blood. 101 (3), 877–885. doi:10.1182/blood-2002-03-0796
Gvaramia, D., Muller, E., Muller, K., Atallah, P., Tsurkan, M., Freudenberg, U., et al. (2017). Combined influence of biophysical and biochemical cues on maintenance and proliferation of hematopoietic stem cells. Biomaterials 138, 108–117. doi:10.1016/j.biomaterials.2017.05.023
Hardouin, P., Pansini, V., and Cortet, B. (2014). Bone marrow fat. Jt. Bone Spine 81 (4), 313–319. doi:10.1016/j.jbspin.2014.02.013
Hastings, J. F., Skhinas, J. N., Fey, D., Croucher, D. R., and Cox, T. R. (2019). The extracellular matrix as a key regulator of intracellular signalling networks. Br. J. Pharmacol. 176 (1), 82–92. doi:10.1111/bph.14195
Hirata, Y., Furuhashi, K., Ishii, H., Li, H. W., Pinho, S., Ding, L., et al. (2018). CD150(high) bone marrow Tregs maintain hematopoietic stem cell quiescence and immune privilege via adenosine. Cell Stem Cell 22 (3), 445–453. doi:10.1016/j.stem.2018.01.017
Hosseinizand, H., Ebrahimi, M., and Abdekhodaie, M. J. (2016). Agitation Increases Expansion of Cord Blood Hematopoietic Cells and Promotes Their Differentiation into Myeloid Lineage. Cytotechnology. 68 (4), 969–978. doi:10.1007/s10616-015-9851-3
Hsu, R. S., Chen, P. Y., Fang, J. H., Chen, Y. Y., Chang, C. W., Lu, Y. J., et al. (2019). Adaptable microporous hydrogels of propagating NGF-gradient by injectable building blocks for accelerated axonal outgrowth. Adv. Sci. 6 (16), 1900520. doi:10.1002/advs.201900520
Huang, G., Li, F., Zhao, X., Ma, Y., Li, Y., Lin, M., et al. (2017). Functional and biomimetic materials for engineering of the three-dimensional cell microenvironment. Chem. Rev. 117 (20), 12764–12850. doi:10.1021/acs.chemrev.7b00094
Ingavle, G., Vaidya, A., and Kale, V. (2019). Constructing three-dimensional microenvironments using engineered biomaterials for hematopoietic stem cell expansion. Tissue Eng. Part B Rev. 25 (4), 312–329. doi:10.1089/ten.TEB.2018.0286
Itkin, T., Gur-Cohen, S., Spencer, J. A., Schajnovitz, A., Ramasamy, S. K., Kusumbe, A. P., et al. (2016). Distinct bone marrow blood vessels differentially regulate haematopoiesis. Nature 532 (7599), 323–328. doi:10.1038/nature17624
Jensen, C., and Teng, Y. (2020). Is it time to start transitioning from 2D to 3D cell culture? Front. Mol. Biosci. 7, 33. doi:10.3389/fmolb.2020.00033
Jiang, Y., Wang, Y., Li, Q., Yu, C., and Chu, W. (2020). Natural polymer-based stimuli-responsive hydrogels. Curr. Med. Chem. 27 (16), 2631–2657. doi:10.2174/0929867326666191122144916
Jing, D., Fonseca, A. V., Alakel, N., Fierro, F. A., Muller, K., Bornhauser, M., et al. (2010). Hematopoietic stem cells in Co-culture with mesenchymal stromal cells-modeling the niche compartments in vitro. Haematologica 95 (4), 542–550. doi:10.3324/haematol.2009.010736
Kehr, N. S., Atay, S., and Ergün, B. (2015). Self-assembled monolayers and nanocomposite hydrogels of functional nanomaterials for tissue engineering applications. Macromol. Biosci. 15 (4), 445–463. doi:10.1002/mabi.201400363
Kim, H., Bae, C., Kook, Y. M., Koh, W. G., Lee, K., and Park, M. H. (2019). Mesenchymal stem cell 3D encapsulation technologies for biomimetic microenvironment in tissue regeneration. Stem Cell Res. Ther. 10 (1), 51. doi:10.1186/s13287-018-1130-8
Kim, S., Kim, H. J., and Jeon, N. L. (2010). Biological applications of microfluidic gradient devices. Integr. Biol. 2 (11-12), 584–603. doi:10.1039/c0ib00055h
Kohli, L., and Passegue, E. (2014). Surviving change: The metabolic journey of hematopoietic stem cells. Trends Cell Biol. 24 (8), 479–487. doi:10.1016/j.tcb.2014.04.001
Kosan, C., and Godmann, M. (2016). Genetic and epigenetic mechanisms that maintain hematopoietic stem cell function. Stem Cells Int. 2016, 1–14. doi:10.1155/2016/5178965
Kuan, I. I., Liang, K. H., Wang, Y. P., Kuo, T. W., Meir, Y. J. J., Wu, S. C. Y., et al. (2017). EpEX/EpCAM and Oct4 or Klf4 alone are sufficient to generate induced pluripotent stem cells through STAT3 and HIF2α. Sci. Rep. 7 (1), 41852–41914. doi:10.1038/srep41852
Kunisaki, Y., Bruns, I., Scheiermann, C., Ahmed, J., Pinho, S., Zhang, D., et al. (2013). Arteriolar niches maintain haematopoietic stem cell quiescence. Nature 502 (7473), 637–643. doi:10.1038/nature12612
Kwak, J. G., and Lee, J. (2020). Thermoresponsive inverted colloidal crystal hydrogel scaffolds for lymphoid tissue engineering. Adv. Healthc. Mat. 9 (6), 1901556. doi:10.1002/adhm.201901556
Langen, U. H., Pitulescu, M. E., Kim, J. M., Enriquez-Gasca, R., Sivaraj, K. K., Kusumbe, A. P., et al. (2017). Cell-matrix signals specify bone endothelial cells during developmental osteogenesis. Nat. Cell Biol. 19 (3), 189–201. doi:10.1038/ncb3476
Langhans, S. A. (2018). Three-dimensional in vitro cell culture models in drug discovery and drug repositioning. Front. Pharmacol. 9, 6. doi:10.3389/fphar.2018.00006
Lau, S. X., Leong, Y. Y., Ng, W. H., Ng, A. W. P., Ismail, I. S., Yusoff, N. M., et al. (2017). Human mesenchymal stem cells promote CD34(+) hematopoietic stem cell proliferation with preserved red blood cell differentiation capacity. Cell Biol. Int. 41 (6), 697–704. doi:10.1002/cbin.10774
Lee, S. M., Han, N., Lee, R., Choi, I. H., Park, Y. B., Shin, J. S., et al. (2016). Real-time monitoring of 3D cell culture using a 3D capacitance biosensor. Biosensors and Bioelectronics. 77, 56–61. doi:10.1016/j.bios.2015.09.005
Leisten, I., Kramann, R., Ferreira, M. S. V., Bovi, M., Neuss, S., Ziegler, P., et al. (2012). 3D Co-culture of hematopoietic stem and progenitor cells and mesenchymal stem cells in collagen scaffolds as a model of the hematopoietic niche. Biomaterials 33 (6), 1736–1747. doi:10.1016/j.biomaterials.2011.11.034
Levesque, J. P., Helwani, F. M., and Winkler, I. G. (2010). The endosteal ‘osteoblastic’ niche and its role in hematopoietic stem cell homing and mobilization. Leukemia 24 (12), 1979–1992. doi:10.1038/leu.2010.214
Liang, Y., Li, M., Yang, Y., Qiao, L., Xu, H., Guo, B., et al. (2022). pH/Glucose dual responsive metformin release hydrogel dressings with adhesion and self-healing via dual-dynamic bonding for athletic diabetic foot wound healing. ACS Nano. 16 (2), 3194–3207. doi:10.1021/acsnano.1c11040
Li, J. H., Wu, C. T., Chu, P. K., and Gelinsky, M. (2020). 3D printing of hydrogels: Rational design strategies and emerging biomedical applications. Mater. Sci. Eng. R Rep. 140, 100543. doi:10.1016/j.mser.2020.100543
Li, Q., Zhao, D., Chen, Q., Luo, M., Huang, J., Yang, C., et al. (2019). Wharton’s jelly mesenchymal stem cell-based or umbilical vein endothelial cell-based serum-free coculture with cytokines supports the ex vivo expansion/maintenance of cord blood hematopoietic stem/progenitor cells. Stem Cell Res. Ther. 10 (1), 376–379. doi:10.1186/s13287-019-1502-8
Lim, M., Ye, H., Panoskaltsis, N., Drakakis, E. M., Yue, X., Cass, A. E., et al. (2007). Intelligent bioprocessing for haemotopoietic cell cultures using monitoring and design of experiments. Biotechnol. Adv. 25 (4), 353–368. doi:10.1016/j.biotechadv.2007.02.002
Liu, B., Tao, C., Wu, Z., Yao, H., and Wang, D. A. (2022). Engineering strategies to achieve efficient in vitro expansion of haematopoietic stem cells: Development and improvement. J. Mat. Chem. B 10 (11), 1734–1753. doi:10.1039/d1tb02706a
Liu, H., Wang, Y., Cui, K., Guo, Y., Zhang, X., and Qin, J. (2019). Advances in hydrogels in organoids and organs-on-a-chip. Adv. Mat. 31 (50), 1902042. doi:10.1002/adma.201902042
Liu, J. J., Qu, S. X., Suo, Z. G., and Yang, W. (2021). Functional hydrogel coatings. Natl. Sci. Rev. 8 (2), nwaa254. doi:10.1093/nsr/nwaa254
Lo Celso, C., Fleming, H. E., Wu, J. W., Zhao, C. X., Miake-Lye, S., Fujisaki, J., et al. (2009). Live-animal tracking of individual haematopoietic stem/progenitor cells in their niche. Nature 457 (7225), 92–96. doi:10.1038/nature07434
Lo Iacono, M., Anzalone, R., La Rocca, G., Baiamonte, E., Maggio, A., and Acuto, S. (2017). Wharton’s jelly mesenchymal stromal cells as a feeder layer for the ex vivo expansion of hematopoietic stem and progenitor cells: A review. Stem Cell Rev. Rep. 13 (1), 35–49. doi:10.1007/s12015-016-9702-4
Lu, D., Luo, C., Zhang, C., Li, Z., and Long, M. (2014). Differential regulation of morphology and stemness of mouse embryonic stem cells by substrate stiffness and topography. Biomaterials 35 (13), 3945–3955. doi:10.1016/j.biomaterials.2014.01.066
Mahadik, B. P., Bharadwaj, N. A., Ewoldt, R. H., and Harley, B. A. (2017). Regulating dynamic signaling between hematopoietic stem cells and niche cells via a hydrogel matrix. Biomaterials 125, 54–64. doi:10.1016/j.biomaterials.2017.02.013
Mahadik, B. P., Haba, S. P., Skertich, L. J., and Harley, B. A. C. (2015). The use of covalently immobilized stem cell factor to selectively affect hematopoietic stem cell activity within a gelatin hydrogel. Biomaterials 67, 297–307. doi:10.1016/j.biomaterials.2015.07.042
Mahadik, B. P., Wheeler, T. D., Skertich, L. J., Kenis, P. J., and Harley, B. A. (2014). Microfluidic generation of gradient hydrogels to modulate hematopoietic stem cell culture environment. Adv. Healthc. Mat. 3 (3), 449–458. doi:10.1002/adhm.201300263
Maji, S., and Lee, H. (2022). Engineering hydrogels for the development of three-dimensional in vitro models. Int. J. Mol. Sci. 23 (5), 2662. doi:10.3390/ijms23052662
Mannino, G., Russo, C., Maugeri, G., Musumeci, G., Vicario, N., Tibullo, D., et al. (2022). Adult stem cell niches for tissue homeostasis. J. Cell. Physiol. 237 (1), 239–257. doi:10.1002/jcp.30562
Maryanovich, M., Takeishi, S., and Frenette, P. S. (2018). Neural regulation of bone and bone marrow. Cold Spring Harb. Perspect. Med. 8 (9), a031344. doi:10.1101/cshperspect.a031344
Matteini, F., Mulaw, M. A., and Florian, M. C. (2021). Aging of the hematopoietic stem cell niche: New tools to answer an old question. Front. Immunol. 12, 738204. doi:10.3389/fimmu.2021.738204
Mendelson, A., and Frenette, P. S. (2014). Hematopoietic stem cell niche maintenance during homeostasis and regeneration. Nat. Med. 20 (8), 833–846. doi:10.1038/nm.3647
Méndez-Ferrer, S., Michurina, T. V., Ferraro, F., Mazloom, A. R., Macarthur, B. D., Lira, S. A., et al. (2010). Mesenchymal and haematopoietic stem cells form a unique bone marrow niche. Nature 466 (7308), 829–834. doi:10.1038/nature09262
Moore, C. A., Siddiqui, Z., Carney, G. J., Naaldijk, Y., Guiro, K., Ferrer, A. I., et al. (2021). A 3D bioprinted material that recapitulates the perivascular bone marrow structure for sustained hematopoietic and cancer models. Polymers 13 (4), 480. doi:10.3390/polym13040480
Morrison, S. J., and Scadden, D. T. (2014). The bone marrow niche for haematopoietic stem cells. Nature 505 (7483), 327–334. doi:10.1038/nature12984
Motealleh, A., and Kehr, N. S. (2017). Nanocomposite hydrogels and their applications in tissue engineering. Adv. Healthc. Mat. 6 (1), 1600938. doi:10.1002/adhm.201600938
Nakamura-Ishizu, A., Takubo, K., Fujioka, M., and Suda, T. (2014). Megakaryocytes are essential for HSC quiescence through the production of thrombopoietin. Biochem. Biophys. Res. Commun. 454 (2), 353–357. doi:10.1016/j.bbrc.2014.10.095
Naveiras, O., Nardi, V., Wenzel, P. L., Hauschka, P. V., Fahey, F., and Daley, G. Q. (2009). Bone-marrow adipocytes as negative regulators of the haematopoietic microenvironment. Nature 460 (7252), 259–263. doi:10.1038/nature08099
Nezhad-Mokhtari, P., Ghorbani, M., Roshangar, L., and Rad, J. S. (2019). Chemical gelling of hydrogels-based biological macromolecules for tissue engineering: Photo-and enzymatic-crosslinking methods. Int J Biol Macromol. 139, 760–772. doi:10.1016/j.ijbiomac.2019.08.047
Nilsson, S. K., Johnston, H. M., and Coverdale, J. A. (2001). Spatial localization of transplanted hemopoietic stem cells: Inferences for the localization of stem cell niches. Blood 97 (8), 2293–2299. doi:10.1182/blood.v97.8.2293
Olate-Moya, F., Arens, L., Wilhelm, M., Mateos-Timoneda, M. A., Engel, E., and Palza, H. (2020). Chondroinductive alginate-based hydrogels having graphene oxide for 3D printed scaffold fabrication. ACS Appl. Mat. Interfaces 12 (4), 4343–4357. doi:10.1021/acsami.9b22062
Ong, C. S., Yesantharao, P., Huang, C. Y., Mattson, G., Boktor, J., Fukunishi, T., et al. (2018). 3D bioprinting using stem cells. Pediatr. Res. 83 (1), 223–231. doi:10.1038/pr.2017.252
Owen-Woods, C., and Kusumbe, A. (2022). Fundamentals of bone vasculature: Specialization, interactions and functions. Semin. Cell Dev. Biol. 123, 36–47. doi:10.1016/j.semcdb.2021.06.025
Pabst, C., Krosl, J., Fares, I., Boucher, G., Ruel, R., Marinier, A., et al. (2014). Identification of small molecules that support human leukemia stem cell activity ex vivo. Nat. Methods 11 (4), 436–442. doi:10.1038/nmeth.2847
Patel, P. N., Smith, C. K., and Patrick, C. W. (2005). Rheological and recovery properties of poly(ethylene glycol) diacrylate hydrogels and human adipose tissue. J. Biomed. Mat. Res. A 73 (3), 313–319. doi:10.1002/jbm.a.30291
Peers, S., Montembault, A., and Ladavière, C. (2020). Chitosan hydrogels for sustained drug delivery. J. Control. Release 326, 150–163. doi:10.1016/j.jconrel.2020.06.012
Pievani, A., Savoldelli, R., Poelchen, J., Mattioli, E., Anselmi, G., Girardot, A., et al. (2021). Harnessing mesenchymal stromal cells for the engineering of human hematopoietic niches. Front. Immunol. 12, 631279. doi:10.3389/fimmu.2021.631279
Pinho, S., and Frenette, P. S. (2019). Haematopoietic stem cell activity and interactions with the niche. Nat. Rev. Mol. Cell Biol. 20 (5), 303–320. doi:10.1038/s41580-019-0103-9
Prince, E., and Kumacheva, E. (2019). Design and applications of man-made biomimetic fibrillar hydrogels. Nat. Rev. Mat. 4 (2), 99–115. doi:10.1038/s41578-018-0077-9
Raic, A., Rödling, L., Kalbacher, H., and Lee-Thedieck, C. (2014). Biomimetic macroporous PEG hydrogels as 3D scaffolds for the multiplication of human hematopoietic stem and progenitor cells. Biomaterials 35 (3), 929–940. doi:10.1016/j.biomaterials.2013.10.038
Ramasamy, S. K. (2017). Structure and functions of blood vessels and vascular niches in bone. Stem Cells Int. 2017, 1–10. doi:10.1155/2017/5046953
Rasheed, A. (2022). Niche regulation of hematopoiesis: The environment is "micro, " but the influence is large. Arterioscler. Thromb. Vasc. Biol. 42 (6), 691–699. doi:10.1161/atvbaha.121.316235
Ribeiro-Filho, A. C., Levy, D., Ruiz, J. L. M., Mantovani, M. D. C., and Bydlowski, S. P. (2019). Traditional and advanced cell cultures in hematopoietic stem cell studies. Cells 8 (12), 1628. doi:10.3390/cells8121628
Rocha, V. (2016). Umbilical cord blood cells from unrelated donor as an alternative source of hematopoietic stem cells for transplantation in children and adults. Semin Hematol. 53 (4), 237–245. doi:10.1053/j.seminhematol.2016.08.002
Rodell, C. B., Kaminski, A. L., and Burdick, J. A. (2013). Rational design of network properties in guest-host assembled and shear-thinning hyaluronic acid hydrogels. Biomacromolecules 14 (11), 4125–4134. doi:10.1021/bm401280z
Rosales, A. M., and Anseth, K. S. (2016). The design of reversible hydrogels to capture extracellular matrix dynamics. Nat. Rev. Mat. 1 (2), 15012–15015. doi:10.1038/natrevmats.2015.12
Sackmann, E. K., Fulton, A. L., and Beebe, D. J. (2014). The present and future role of microfluidics in biomedical research. Nature 507 (7491), 181–189. doi:10.1038/nature13118
Sarvar, D. P., Effatpanah, H., Akbarzadehlaleh, P., and Shamsasenjan, K. (2022). Mesenchymal stromal cell-derived extracellular vesicles: Novel approach in hematopoietic stem cell transplantation. Stem Cell Res. Ther. 13 (1), 202–210. doi:10.1186/s13287-022-02875-3
Schmal, O., Seifert, J., Schäffer, T. E., Walter, C. B., Aicher, W. K., and Klein, G. (2016). Hematopoietic stem and progenitor cell expansion in contact with mesenchymal stromal cells in a hanging drop model uncovers disadvantages of 3D culture. Stem Cells Int. 2016, 1–13. doi:10.1155/2016/4148093
Sharma, G., Thakur, B., Naushad, M., Kumar, A., Stadler, F. J., Alfadul, S. M., et al. (2018). Applications of nanocomposite hydrogels for biomedical engineering and environmental protection. Environ. Chem. Lett. 16 (1), 113–146. doi:10.1007/s10311-017-0671-x
Sharma, M. B., Limaye, L. S., and Kale, V. P. (2012). Mimicking the functional hematopoietic stem cell niche in vitro: Recapitulation of marrow physiology by hydrogel-based three-dimensional cultures of mesenchymal stromal cells. Haematologica 97 (5), 651–660. doi:10.3324/haematol.2011.050500
Shen, B., Tasdogan, A., Ubellacker, J. M., Zhang, J., Nosyreva, E. D., Du, L., et al. (2021). A mechanosensitive peri-arteriolar niche for osteogenesis and lymphopoiesis. Nature 591 (7850), 438–444. doi:10.1038/s41586-021-03298-5
Sieber, S., Wirth, L., Cavak, N., Koenigsmark, M., Marx, U., Lauster, R., et al. (2018). Bone marrow-on-a-chip: Long-term culture of human haematopoietic stem cells in a three-dimensional microfluidic environment. J. Tissue Eng. Regen. Med. 12 (2), 479–489. doi:10.1002/term.2507
Silberstein, L. E., and Lin, C. P. (2013). A new image of the hematopoietic stem cell vascular niche. Cell Stem Cell 13 (5), 514–516. doi:10.1016/j.stem.2013.10.012
Sivakumaran, D., Maitland, D., Oszustowicz, T., and Hoare, T. (2013). Tuning drug release from smart microgel-hydrogel composites via cross-linking. J. Colloid Interface Sci. 392, 422–430. doi:10.1016/j.jcis.2012.07.096
Spencer, J. A., Ferraro, F., Roussakis, E., Klein, A., Wu, J., Runnels, J. M., et al. (2014). Direct measurement of local oxygen concentration in the bone marrow of live animals. Nature 508 (7495), 269–273. doi:10.1038/nature13034
Stier, S., Ko, Y., Forkert, F., Lutz, C., Neuhaus, T., Grunewald, E., et al. (2005). Osteopontin is a hematopoietic stem cell niche component that negatively regulates stem cell pool size. J. Exp. Med. 201 (11), 1781–1791. doi:10.1084/jem.20041992
Suda, T., Takubo, K., and Semenza, G. L. (2011). Metabolic regulation of hematopoietic stem cells in the hypoxic niche. Cell Stem Cell 9 (4), 298–310. doi:10.1016/j.stem.2011.09.010
Tang, S. C., Richardson, B. M., and Anseth, K. S. (2021). Dynamic covalent hydrogels as biomaterials to mimic the viscoelasticity of soft tissues. Prog. Mat. Sci. 120, 100738. doi:10.1016/j.pmatsci.2020.100738
Tibbitt, M. W., and Anseth, K. S. (2009). Hydrogels as extracellular matrix mimics for 3D cell culture. Biotechnol. Bioeng. 103 (4), 655–663. doi:10.1002/bit.22361
Torisawa, Y. S., Spina, C. S., Mammoto, T., Mammoto, A., Weaver, J. C., Tat, T., et al. (2014). Bone marrow-on-a-chip replicates hematopoietic niche physiology in vitro. Nat. Methods 11 (6), 663–669. doi:10.1038/nmeth.2938
Torres, L. S., Asada, N., Weiss, M. J., Trumpp, A., Suda, T., Scadden, D. T., et al. (2022). Recent advances in "sickle and niche" research - tribute to Dr. Paul S Frenette. Stem Cell Rep. 17 (7), 1509–1535. doi:10.1016/j.stemcr.2022.06.004
Verma, D., Zanetti, C., Godavarthy, P. S., Kumar, R., Minciacchi, V. R., Pfeiffer, J., et al. (2020). Bone marrow niche-derived extracellular matrix-degrading enzymes influence the progression of B-cell acute lymphoblastic leukemia. Leukemia 34 (6), 1540–1552. doi:10.1038/s41375-019-0674-7
Vining, K. H., and Mooney, D. J. (2017). Mechanical forces direct stem cell behaviour in development and regeneration. Nat. Rev. Mol. Cell Biol. 18 (12), 728–742. doi:10.1038/nrm.2017.108
Walenda, T., Bork, S., Horn, P., Wein, F., Saffrich, R., Diehlmann, A., et al. (2010). Co-culture with mesenchymal stromal cells increases proliferation and maintenance of haematopoietic progenitor cells. J. Cell. Mol. Med. 14 (1-2), 337–350. doi:10.1111/j.1582-4934.2009.00776.x
Wang, W., Zhao, Y., Bai, H., Zhang, T., Ibarra-Galvan, V., and Song, S. (2018). Methylene blue removal from water using the hydrogel beads of poly (vinyl alcohol)-sodium alginate-chitosan-montmorillonite. Carbohydr. Polym. 198, 518–528. doi:10.1016/j.carbpol.2018.06.124
Whitesides, G. M. (2006). The origins and the future of microfluidics. Nature 442 (7101), 368–373. doi:10.1038/nature05058
Wilkinson, A. C., Igarashi, K. J., and Nakauchi, H. (2020). Haematopoietic stem cell self-renewal in vivo and ex vivo. Nat. Rev. Genet. 21 (9), 541–554. doi:10.1038/s41576-020-0241-0
Wilkinson, A. C., and Nakauchi, H. (2020). Stabilizing hematopoietic stem cells in vitro. Curr. Opin. Genet. Dev. 64, 1–5. doi:10.1016/j.gde.2020.05.035
Winkler, J., Abisoye-Ogunniyan, A., Metcalf, K. J., and Werb, Z. (2020). Concepts of extracellular matrix remodelling in tumour progression and metastasis. Nat. Commun. 11 (1), 5120–5219. doi:10.1038/s41467-020-18794-x
Wolf, M. T., Dearth, C. L., Sonnenberg, S. B., Loboa, E. G., and Badylak, S. F. (2015). Naturally derived and synthetic scaffolds for skeletal muscle reconstruction. Adv. Drug Deliv. Rev. 84, 208–221. doi:10.1016/j.addr.2014.08.011
Wong, S. W., Lenzini, S., Giovanni, R., Knowles, K., and Shin, J. W. (2021). Matrix biophysical cues direct mesenchymal stromal cell functions in immunity. Acta Biomater. 133, 126–138. doi:10.1016/j.actbio.2021.07.075
Xia, B., Jiang, Z., Debroy, D., Li, D., and Oakey, J. (2017). Cytocompatible cell encapsulation via hydrogel photopolymerization in microfluidic emulsion droplets. Biomicrofluidics 11 (4), 044102. doi:10.1063/1.4993122
Xiao, Y., McGuinness, C. S., Doherty-Boyd, W. S., Salmeron-Sanchez, M., Donnelly, H., and Dalby, M. J. (2022). Current insights into the bone marrow niche: From biology in vivo to bioengineering ex vivo. Biomaterials 286, 121568. doi:10.1016/j.biomaterials.2022.121568
Xin, S., Chimene, D., Garza, J. E., Gaharwar, A. K., and Alge, D. L. B. s. (2019). Clickable PEG hydrogel microspheres as building blocks for 3D bioprinting. Biomater. Sci. 7 (3), 1179–1187. doi:10.1039/c8bm01286e
Yan, L., Yang, X., Zhao, Y., Wu, Y., Moutloali, R. M., Mamba, B. B., et al. (2022). Bio-inspired mineral-hydrogel hybrid coating on hydrophobic PVDF membrane boosting oil/water emulsion separation. Sep. Purif. Technol. 285, 120383. doi:10.1016/j.seppur.2021.120383
Yeoh, J. S., van Os, R., Weersing, E., Ausema, A., Dontje, B., Vellenga, E., et al. (2006). Fibroblast growth factor-1 and -2 preserve long-term repopulating ability of hematopoietic stem cells in serum-free cultures. Stem Cells 24 (6), 1564–1572. doi:10.1634/stemcells.2005-0439
Yin, S., and Cao, Y. (2021). Hydrogels for large-scale expansion of stem cells. Acta Biomater. 128, 1–20. doi:10.1016/j.actbio.2021.03.026
Yucel, D., and Kocabas, F. (2018). Developments in hematopoietic stem cell expansion and gene editing technologies. Adv. Exp. Med. Biol. 1079, 103–125. doi:10.1007/5584_2017_114
Yue, K., Liu, Y., Byambaa, B., Singh, V., Liu, W., Li, X., et al. (2018). Visible light crosslinkable human hair keratin hydrogels. Bioeng. Transl. Med. 3 (1), 37–48. doi:10.1002/btm2.10077
Zakrzewski, W., Dobrzyński, M., Szymonowicz, M., and Rybak, Z. (2019). Stem cells: Past, present, and future. Stem Cell Res. Ther. 10 (1), 68. doi:10.1186/s13287-019-1165-5
Zhan, Z., Liu, Z. Y., Nan, H. C., Li, J. J., Xie, Y., and Hu, C. Z. (2022). Heterogeneous spheroids with tunable interior morphologies by droplet-based microfluidics. Biofabrication 14 (2), 025024. doi:10.1088/1758-5090/ac5e12
Zhang, H. J., Sun, T. L., Zhang, A. K., Ikura, Y., Nakajima, T., Nonoyama, T., et al. (2016). Tough physical double-network hydrogels based on amphiphilic triblock copolymers. Adv. Mat. 28 (24), 4884–4890. doi:10.1002/adma.201600466
Zhang, J., Niu, C., Ye, L., Huang, H., He, X., Tong, W. G., et al. (2003). Identification of the haematopoietic stem cell niche and control of the niche size. Nature 425 (6960), 836–841. doi:10.1038/nature02041
Zhang, P., Sun, F., Tsao, C., Liu, S., Jain, P., Sinclair, A., et al. (2015). Zwitterionic gel encapsulation promotes protein stability, enhances pharmacokinetics, and reduces immunogenicity. Proc. Natl. Acad. Sci. U. S. A. 112 (39), 12046–12051. doi:10.1073/pnas.1512465112
Zhang, P., Zhang, C., Li, J., Han, J., Liu, X., and Yang, H. (2019a). The physical microenvironment of hematopoietic stem cells and its emerging roles in engineering applications. Stem Cell Res. Ther. 10 (1), 327. doi:10.1186/s13287-019-1422-7
Zhang, Y., Pan, X., Shi, Z., Cai, H., Gao, Y., and Zhang, W. (2018). Sustained release of stem cell factor in a double network hydrogel for ex vivo culture of cord blood-derived CD34+ cells. Cell Prolif. 51 (2), e12407. doi:10.1111/cpr.12407
Zhang, Y. S., and Khademhosseini, A. (2017). Advances in engineering hydrogels. Science 356 (6337), eaaf3627. doi:10.1126/science.aaf3627
Zhang, Y., Tong, Y., Pan, X., Cai, H., Gao, Y., and Zhang, W. (2019b). Promoted proliferation of hematopoietic stem cells enabled by a hyaluronic acid/carbon nanotubes antioxidant hydrogel. Macromol. Mat. Eng. 304 (4), 1800630. doi:10.1002/mame.201800630
Zhao, M., and Li, L. (2016). Dissecting the bone marrow HSC niches. Cell Res. 26 (9), 975–976. doi:10.1038/cr.2016.71
Zhao, M., Perry, J. M., Marshall, H., Venkatraman, A., Qian, P., He, X. C., et al. (2014). Megakaryocytes maintain homeostatic quiescence and promote post-injury regeneration of hematopoietic stem cells. Nat. Med. 20 (11), 1321–1326. doi:10.1038/nm.3706
Zhao, M., Tao, F., Venkatraman, A., Li, Z., Smith, S. E., Unruh, J., et al. (2019). N-Cadherin-Expressing bone and marrow stromal progenitor cells maintain reserve hematopoietic stem cells. Cell Rep. 26 (3), 652–669. doi:10.1016/j.celrep.2018.12.093
Zhao, X., Lang, Q., Yildirimer, L., Lin, Z. Y., Cui, W. G., Annabi, N., et al. (2016). Photocrosslinkable gelatin hydrogel for epidermal tissue engineering. Adv. Healthc. Mat. 5 (1), 108–118. doi:10.1002/adhm.201500005
Zhou, D. Z., Chen, L. D., Ding, J. J., Zhang, X. X., Nie, Z. G., Li, X. D., et al. (2020). A 3D engineered scaffold for hematopoietic progenitor/stem cell Co-culture in vitro. Sci. Rep. 10 (1), 11485. doi:10.1038/s41598-020-68250-5
Zimmermann, R., Hentschel, C., Schron, F., Moedder, D., Buttner, T., Atallah, P., et al. (2019). High resolution bioprinting of multi-component hydrogels. Biofabrication 11 (4), 045008. doi:10.1088/1758-5090/ab2aa1
Keywords: hydrogels, hematopoietic stem cells, in vitro expansion, niche biomimicking, cell microenvironment
Citation: Huang X, Wang Y, Wang T, Wen F, Liu S and Oudeng G (2022) Recent advances in engineering hydrogels for niche biomimicking and hematopoietic stem cell culturing. Front. Bioeng. Biotechnol. 10:1049965. doi: 10.3389/fbioe.2022.1049965
Received: 21 September 2022; Accepted: 07 November 2022;
Published: 24 November 2022.
Edited by:
Yang Kang, Sun Yat-sen University, ChinaReviewed by:
Betül Çelebi Saltik, Hacettepe University, TurkeyYuting Wang, Seventh Affiliated Hospital, Sun Yat-sen University, China
Copyright © 2022 Huang, Wang, Wang, Wen, Liu and Oudeng. This is an open-access article distributed under the terms of the Creative Commons Attribution License (CC BY). The use, distribution or reproduction in other forums is permitted, provided the original author(s) and the copyright owner(s) are credited and that the original publication in this journal is cited, in accordance with accepted academic practice. No use, distribution or reproduction is permitted which does not comply with these terms.
*Correspondence: Feiqiu Wen, ZndlbjYyQDEyNi5jb20=; Sixi Liu, c2l4aV9saXVAMTYzLmNvbQ==; Gerile Oudeng, Z2VyaWxlLm91ZGVuZ0Bjb25uZWN0LnBvbHl1Lmhr
†These authors have contributed equally to this work and share first authorship