- 1Department of Hepatopancreatobiliary Surgery and Organ Transplantation Center, Department of General Surgery, The First Affiliated Hospital of Anhui Medical University, Hefei, China
- 2Department of Internal Medicine IV (Gastroenterology, Hepatology, and Infectious Diseases), Jena University Hospital, Jena, Germany
- 3Department of Burns, The First Affiliated Hospital of Anhui Medical University, Hefei, China
- 4Department of Urology, Jena University Hospital, Jena, Germany
- 5Department of Anesthesiology, The First Affiliated Hospital of Anhui Medical University, Hefei, China
Liver transplantation is currently the only effective treatment for patients with end-stage liver disease; however, donor liver scarcity is a notable concern. As a result, extensive endeavors have been made to diversify the source of donor livers. For example, the use of a decellularized scaffold in liver engineering has gained considerable attention in recent years. The decellularized scaffold preserves the original orchestral structure and bioactive chemicals of the liver, and has the potential to create a de novo liver that is fit for transplantation after recellularization. The structure of the liver and hepatic extracellular matrix, decellularization, recellularization, and recent developments are discussed in this review. Additionally, the criteria for assessment and major obstacles in using a decellularized scaffold are covered in detail.
1 Introduction
Globally, end-stage liver disease is a primary cause of morbidity and mortality, accounting for more than one million deaths per year (Cheemerla and Balakrishnan, 2021). End-stage liver disease is a growing public health hazard for which liver transplantation is the only available remedy.
Since Thomas Starzl conducted the first liver transplantation in 1963, liver transplantation has evolved significantly over the last 50 years. Along with the renewal of immunosuppressive drugs, liver transplantation technology has increasingly advanced, resulting in the survival of numerous patients with end-stage liver disease (Song et al., 2014). Unfortunately, donor scarcity continues to be a significant issue. Aside from increasing citizen donation rates and marginal liver utilization, efforts are required to develop alternative viable treatments (Muller et al., 2020; Yagi et al., 2020).
In recent years, the use of decellularized scaffolds in liver engineering has garnered considerable attention (Figure 1). Decellularized scaffolds from xenogeneic animals (such as pigs, sheep, and cattle) and discarded human livers preserve the multifaceted extracellular matrix (ECM) of the liver and serve as a reservoir for growth factors, cytokines, and signaling molecules that are critical and indispensable for cellular growth, proliferation, differentiation, and neovascularization (Choudhury et al., 2020). Decellularized scaffolds are subsequently repopulated with primary hepatocytes, endothelial cells, and stem cells, which ultimately develop new livers that can be transplanted into patients with end-stage liver disease to replace the original decompensated livers. Increasing evidence suggests that decellularized scaffolds can sustain implanted cells for extended periods, enabling subsequent implantation (Kakabadze et al., 2018; Yang et al., 2018; Liu et al., 2019; Everwien et al., 2020b; Shaheen et al., 2020).
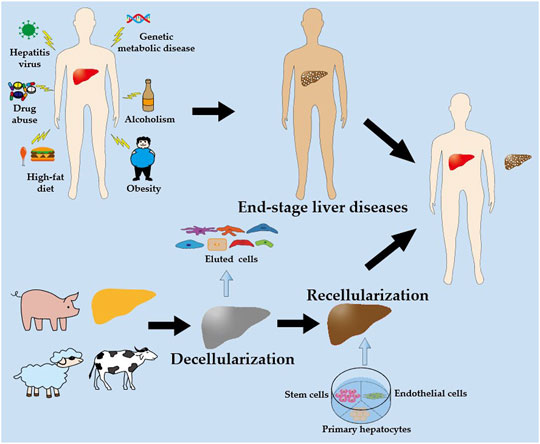
FIGURE 1. A schematic illustration of the liver engineering based on decellularized scaffold. Livers obtained from xenogeneic animals (such as pigs, sheep and cattle) and discarded human livers are decellularized to produce decellularized scaffolds. The decellularized scaffolds are then recellularized with hepatocytes, endothelial cells, and stem cells, which are perfused in vitro to obtain a new liver. Ultimately, the new liver is transplanted into the patient with end-stage liver disease to replace the original decompensated liver.
Therefore, the recellularization of decellularized scaffolds holds great therapeutic potential for creating transplantable grafts for treating end-stage liver disease. This review provides an overview of the liver, hepatic ECM, decellularization, recellularization, and recent advances in liver engineering. Additionally, the review presents the detailed evaluation criteria and main obstacles for utilizing decellularized scaffolds.
2 Liver and Hepatic ECM
2.1 Liver Structure
The liver is the largest internal organ in the body and is composed of various cell types, including hepatocytes, liver sinusoidal endothelial cells (LSECs), Kupffer cells, stellate cells, and cholangiocytes (Gordillo et al., 2015; Trefts et al., 2017; Stamataki and Swadling, 2020). These cells function in tandem to regulate liver function.
The liver receives a dual blood supply from the hepatic artery (HA) and portal vein (PV), and transports the bile that is synthesized by hepatocytes to the gallbladder and duodenum via the common bile duct (Figure 2A). The liver lobule (Figure 2B) is the anatomical structural unit of the liver and comprises hexagonal cords of hepatocytes, which are the principal parenchymal cells of the liver; these cells account for approximately 80% of the cellular composition of the liver. The central vein is located in the middle of each liver lobule, while the portal triad is located on the periphery and is composed of closely bundled branches of the HA, PV, and bile duct.
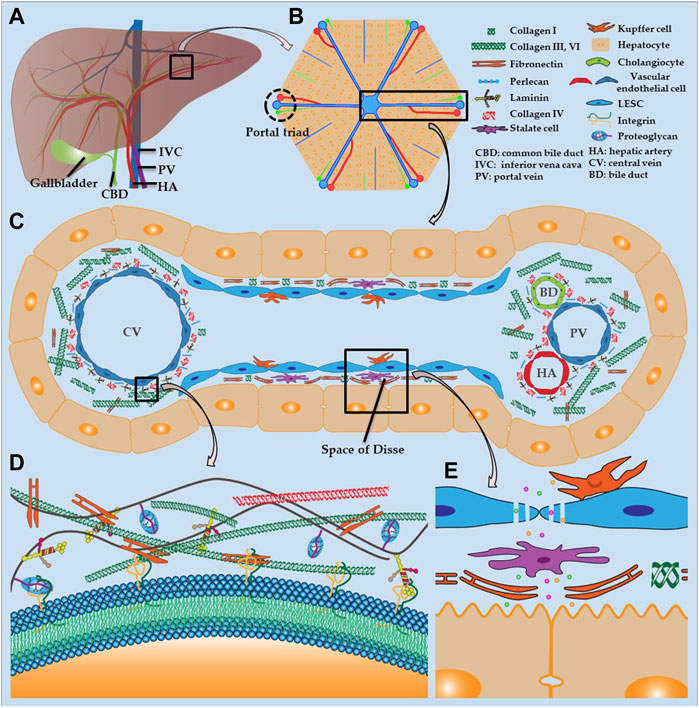
FIGURE 2. Liver structure and hepatic ECM (A) Distribution of hepatic vasculature and bile ducts (B) Histology of liver lobule (C) Composition of hepatic sinusoids and ECM (D) ECM structure (E) Fenestration of LSECs and structure of space of Disse.
The hepatic sinusoid is a capillary-like channel lined with LSECs and flanked by hepatocyte plates and drains blood from the terminal branches of HA and PV into the central vein (Figure 2C) (McQuitty et al., 2020). Kupffer cells are resident macrophages that are localized in the hepatic sinusoid and adhere to LSECs. These cells are responsible for the uptake and destruction of foreign materials that are transported to the liver, such as bacteria, endotoxins, and microbial debris. The sinusoidal endothelium is primarily composed of LSECs, which are highly specialized endothelial cells and the most abundant non-parenchymal cells in the liver. These cells are located at the interface between the liver parenchyma and circulatory system (Poisson et al., 2017; Gracia-Sancho et al., 2021). Unlike other endothelial cells, LSECs have a unique phenotype because they lack the basement membrane and have a profusion of fenestrations (Figure 2E) (Li et al., 2020). Consequently, LSEC fenestrations and slow blood flow in the hepatic sinusoid enable hepatocytes to exchange sufficient substances with the blood in the space of Disse to perform essential functions, such as the uptake of toxins for processing and the secretion of synthesized serum proteins. Stellate cells are present in the space of Disse and allow the liver to respond to injury and heal certain types of damage. The surface of hepatocytes, which face the hepatic sinusoid, consists of a basolateral membrane with many microvilli, which dramatically increase the contact area between hepatocytes and plasma (Figure 2E). The smooth lateral membrane connects to adjacent hepatocytes and forms a narrow, tubular channel, which drains toxins, bile, and other digestive molecules secreted by hepatocytes to the bile duct. Cholangiocytes are epithelial cells of the bile duct that release bicarbonate and water, which contribute to bile secretion.
2.2 Hepatic ECM
The hepatic ECM is a well-organized non-cellular tridimensional scaffold composed of collagens, proteoglycan, fibronectin, laminin and several other glycoproteins, all secreted by resident cells in liver, and provides a microenvironment for adhesion, growth, replication, migration, differentiation, and other vital activities of the surrounding cells (Figure 2D). The hepatic ECM consists of the interstitial matrix, which fills in the intercellular space, and a basement membrane, which separates the endothelium from the underlying connective tissue. The basement membrane, which is composed of laminin, collagen type IV, and perlecan, is mainly distributed around the portal triad and central vein (Figure 2C) (Mak and Mei, 2017; Zhang et al., 2018). The liver parenchyma lacks a complete basement membrane, and the fibronectin and collagen I in the space of Disse separate the hepatocytes and hepatic sinusoids. The interstitial matrix comprises collagen types I, III, VI, and fibronectin, which provides a place for cells to attach and reside, and buffers against compression that is placed on the liver. Likewise, integrin is a transmembrane receptor that acts as a cement and modulator by binding to ligands, such as fibronectin, collagen, laminin, and cell surface proteins, and promotes cell-cell or cell-matrix adhesion and interaction (Figure 2D).
The composition of the hepatic ECM fluctuates with age. For example, older livers tend to have greater amounts of total collagen, sulfated glycosaminoglycans (GAGs), and lower levels of growth factors. Therefore, donor age should be considered as a critical component in determining the quality of the neo-liver during liver engineering (Acun et al., 2021).
3 Decellularization
Liver engineering is presently considered a promising alternative strategy for organ scarcity. Additionally, the use of synthetic and decellularized scaffolds in liver engineering has emerged as a research hotspot in liver engineering. The decellularized scaffold is more advantageous than the synthetic scaffold because it preserves the natural microscopic structures and microenvironment, retains bioactive molecules, and allows for implantation of the liver through directly anastomosing the native vessels of the scaffold and the vessels of the recipient (McCrary et al., 2020). Decellularization is the elimination of cellular components from native tissues or organs, while conserving the ECM and bioactive molecules. Physical methods, chemical reagents, and enzymatic treatments are commonly used for decellularization.
3.1 Physical Methods
3.1.1 Sonication
Sonication uses an ultrasonic bath or probe to transfer acoustic power into a solvent containing tissues or organs to disrupt cell membranes; an ultrasonic bath is preferred for decellularization. The collapse of microscopic vacuum bubbles generated during sonication releases violent shockwaves that shear the cell; the resulting cellular debris is removed by perfusion. The energy generated from the collision of the sound waves generates heat in the solution. Therefore, monitoring the temperature during sonication and cooling in a chiller are necessary to avoid heating and denaturation of the proteins and molecules. Sonication is mainly used with detergents to decellularize dense tissues, such as tendons, ligaments, and cartilage, as well as thin tissues, such as skin and blood vessels, where sonication allows the detergents to penetrate the tissues more effectively (Yusof et al., 2019; Shen et al., 2020; Dang et al., 2021; Lin et al., 2021; Suss et al., 2021). Sonication has only been used to decellularize whole parenchymal organs in the kidney, and has likely not been used in the liver because of the colossal size of liver and the need for high power to disrupt the hepatic microstructure (Say et al., 2019; Manalastas et al., 2021).
3.1.2 Freeze-Thaw
Rapid thermal changes in the freeze-thaw effectively lyse cells and aid in their subsequent removal (Tao et al., 2021). Hence, a single freeze-thaw cycle is commonly used as the first step to reduce the quantities of chemical reagents for liver decellularization (Cheng et al., 2019). However, a single freeze-thaw cycle can still impact the microstructure and mechanical properties of the ECM due to the formation of ice crystals (Cheng et al., 2019). As a result, some researchers have advocated the use of cryoprotectants in perfusion-mediated decellularization to mitigate the detrimental effects of the freeze-thaw cycle without affecting cell lysis. For example, Pulver reported that pretreatment with 5% trehalose achieved the same result as an overnight freeze-thaw cycle, but with lower microstructural damage (Pulver. et al., 2014).
3.1.3 Immersion and Agitation
Immersing tissues or organs in a reagent is the most straightforward method of decellularization, and when paired with agitation, the outcomes of decellularization are improved. The reaction efficiency depends on the reagent type, duration of immersion, and intensity of agitation. A reagent for the removal of the cellular components is required, and the total duration should be minimized to avoid damaging the ECM scaffold (Mattei et al., 2014). However, this approach is usually only appropriate for epidermal tissues and smaller organs, such as the small intestine submucosa, blood vessels, trachea, cornea, nerve, and thyroid gland, and is not appropriate for larger parenchymal organs (Syed et al., 2014; Guimaraes et al., 2019; Visscher et al., 2021; Changchen et al., 2021; Weng et al., 2021). The required DNA content of 50 ng/mg in the decellularized tissue is only achieved when the thickness of the liver slices or discs is less than 5 mm (Mattei et al., 2014; Mazza et al., 2019).
3.2 Chemical Methods
3.2.1 Detergents
Ionic, non-ionic, and zwitterionic detergents are commonly used for decellularization. The most extensively used ionic detergents are sodium dodecyl sulfate (SDS) and sodium deoxycholate (SDC), which solubilize cellular membranes and denature proteins (Zhang et al., 2018; Willemse et al., 2020; Felgendreff et al., 2021). Triton X-100 is the most widely used non-ionic detergent and is capable of breaking lipid-lipid, lipid-protein, and DNA-protein interactions. Triton X-100 is considered a gentler detergent than SDS. Furthermore, Jeong reported that SDS and SDC significantly disrupted GAGs and elastin, albeit more efficiently than Triton X-100 (Jeong et al., 2021). Additionally, the protocol using Triton X-100 alone conserved 1.5-fold more collagen and 2.5-fold more GAGs in the liver than that using Triton X-100 + SDS (Willemse et al., 2020). Zwitterionic detergents (such as 3-[(3-cholamidopropyl) dimethylammonio]-1-propanesulfonate (CHAPS)) exhibit both non-ionic and ionic characteristics, and are less harmful to proteins because of the net-zero electrical charge on the hydrophilic groups (O'Neill et al., 2013). Because of its low permeability, CHAPS is frequently used in thin tissues and organs, and its use in the liver has been rarely reported (O'Neill et al., 2013; Tajima et al., 2018; Shimoda et al., 2019).
Although diverse detergents are frequently combined for optimal decellularization, the efficiency of detergents nonetheless varies linearly with the exposure time and concentration, and varies inversely with the tissue thickness and density. Prolonged exposure times and high detergent concentrations can maximize the extent to which the ECM of the scaffold approaches the cell-free state. However, the ECM can also be disrupted and residual detergents may be present, particularly if SDS is used, which can penetrate deeply into the tissue and slowly dissipate. The disruption of the ECM and residual detergents may result in the formation of thrombi and cytotoxicity after recellularization and implantation, particularly in the liver because of its abundant blood flow and metabolic importance.
3.2.2 Hypertonic and Hypotonic Solutions
Hypertonic and hypotonic solutions promote cell shrinkage and swelling due to osmosis and ultimately induce lysis, while having a negligible effect on the ECM (Kim et al., 2016). Although osmosis can kill cells, it is inefficient and must be coupled with detergents or enzymes to obliterate the cellular components in the liver (Kobes et al., 2018; Lewis et al., 2018). Joseph reported that the sequential treatment of mouse liver with a hypotonic solution and Triton X-100 resulted in a scaffold that was devoid of cells and maintained its structural integrity, as confirmed by the scanning electron microscopy (SEM) images (Kobes et al., 2018).
3.2.3 Acids and Bases
Although acids remove DNA by dissolving cytoplasm and breaking nucleic acids during decellularization, they are more currently and popularly used as disinfectants because they are strong oxidants (Vishwakarma et al., 2019; Gao et al., 2020; Hussein et al., 2020). In a highly alkaline solution, double-stranded DNA denatures low-viscosity single-stranded DNA, which is easily removed by perfusion (Sengyoku et al., 2018). Relevant studies have demonstrated that a highly alkaline NaOH-PBS solution could achieve the same effect as SDS and CHAPS during decellularization while also enhancing biocompatibility and vascular regeneration of scaffolds (van Steenberghe et al., 2017; Sengyoku et al., 2018; van Steenberghe et al., 2018). Additionally, highly alkaline solutions with a pH of 12 or more can inactivate conventional bacteria and viruses, as well as unconventional prions, which are pathogens that are often associated with the use of biomaterials (van Steenberghe et al., 2017; Sengyoku et al., 2018). However, acids and bases can damage collagen and undermine protein-protein bonds, leading to microstructural destruction and decreased viscoelasticity (Reing et al., 2010). In addition, sodium hydroxide cleaves nearly all the growth factors, such as fibroblast growth factor and vascular endothelial growth factor (Reing et al., 2010).
3.2.4 Alcohols
Because of the polar hydroxyl groups, alcohols can diffuse into cells and resultantly dehydrate and lyse cells (Flynn, 2010). Because alcohol is more efficient at removing lipids than lipase, alcohols (such as glycerol) are frequently used to extract lipids from adipose tissue (Flynn, 2010; Brown et al., 2011). Alcohols can precipitate proteins and destroy the ultrastructure of the ECM; therefore, care must be taken when they are used to remove cellular components. Compared to acids, alcohols are more routinely used to sterilize decellularized matrix materials. Ethanol (4%) and peracetic acid (0.1%) are frequently used to sterilize decellularized scaffolds in liver engineering (Xiang et al., 2016; Devalliere et al., 2018; Nishiguchi and Taguchi, 2021; Sassi et al., 2021).
3.2.5 Chelates
Chelates (such as ethylenediaminetetraacetic acid (EDTA) and ethylene glycol tetraacetic acid (EGTA)) bind metallic ions that are essential for protein interaction, resulting in the disconnection of intercellular integral proteins and disruption of cellular adhesion in the ECM. However, chelates alone are unable to completely remove cells and should be combined with detergents or enzymes (Zhao C et al., 2020; Faccioli et al., 2020; Lorvellec et al., 2020). Additionally, using EDTA in the decellularization technique reduces the residual DNA and produces more intact ECM (Maghsoudlou et al., 2016).
3.3 Enzymatic Treatments
Nucleases, trypsin, dispase, lipase, and collagenase are commonly used in decellularization, whereas nucleases and trypsin are most frequently used in liver decellularization. Enzymes can precisely remove cellular components, prevent unfavorable immune responses, and improve the efficacy of detergent-based decellularization.
Nucleases are enzymes that cleave the phosphodiester bonds between nucleotides in nucleic acids. They typically expedite the removal of nucleotides and minimize the risk of immune responses during decellularization. Endonucleases, such as DNase and RNase, are frequently used to remove nucleic acids during liver decellularization (Ahmed et al., 2020; Everwien et al., 2020b; Wu et al., 2020). Trypsin is a serine protease that hydrolyzes the proteins involved in cellular attachment; consequently, trypsin dissociates cells from adhering tissue. The regular replacement of trypsin is necessary because protease inhibitors that are released from broken cells restrict trypsin activity after prolonged circulation (Prasertsung et al., 2008). Additionally, the enzymes can penetrate deeper into the tissue under pressurized conditions to increase the reaction between the enzyme and the substrate, which aids in cellular removal and shortens the reaction time (Prasertsung et al., 2008).
3.4 Combination
Each of the aforementioned methods (Sections 3.1–3.3) has advantages and disadvantages. Although physical methods are less disruptive to the microstructure of the tissue, they do not remove cellular components. Comparatively, although chemical agents can significantly remove cellular components, they inevitably destroy the microstructure and ECM. The preferred method of decellularization varies tremendously among different tissues and organs, and the ideal protocol should include at least two of the aforementioned methods to maximize their respective advantages and minimize the disruption of the ECM. Currently, the most frequently used protocol for liver decellularization is a freeze-thaw cycle followed by a combination of detergents, enzymes, and chelating agents (details presented in Table 1).
Perfusion via the HA and PV is the preferred method for whole liver decellularization. To lyse and remove cells in the liver, detergents, enzymes, and other reagents are perfused into the vasculature of the liver; this approach ensures that the maximal integrity of the ECM is maintained. For example, decellularized liver contained less residual DNA, with a more homogeneous ECM, under oscillatory pressure and pressure-controlled perfusion; this approach also required a relatively short time, and more collagen and GAGs were retained in the decellularized liver (Struecker et al., 2017; Shaheen et al., 2020). Benjamin first reported that applying oscillatory pressure to rat livers that were perfused with 1% Triton X-100 and 1% SDS further enhanced microperfusion and the homogeneity of decellularization by simulating intra-abdominal conditions during respiration (Struecker et al., 2017). Shaheen noted that porcine livers decellularized by PV perfusion of 1x Triton X-100 and 0.6% SDS maintained a perfusion pressure of 8–12 mm Hg and preserved the parenchymal liver lobule structures when compared with the native liver (Shaheen et al., 2020).
3.5 Evaluation of Decellularized Liver ECM
The ultimate goal of decellularization is to remove all the cellular components while retaining an intact ECM for recellularization. However, no method has been able to satisfy this standard. Following recellularization, the remaining cellular components in the ECM create an unfavorable host immune response, and the deterioration of the ECM will also compromise the bioactivity of the implanted cells. Thus, establishing a quality standard for decellularization and scaffolds can facilitate the rapid and organized development of liver engineering and better satisfy the requirements for clinical applications.
3.5.1 Evaluation of Decellularization Efficiency
Presently, the degree of decellularization is primarily determined by the residual DNA, which is ubiquitous in tissues and cells and is directly associated with the host immune response. The generally acknowledged criteria are as follows: 1) DNA content <50 ng/mg dry ECM weight; 2) DNA fragment length <200 base pairs; and 3) lack of visible nuclear content with DAPI and HE staining. Narciso et al. lately described a novel method that combines phase-contrast images of decellularized tissues and fluorescent images of DNA staining of cell nuclei, allowing faster and easier quantification of DNA content (Narciso et al., 2021). However, investigations have shown that the cytoplasm and components of the cell membrane can also cause immune responses and inflammation (Chakraborty et al., 2020). Therefore, these aforementioned criteria should be upgraded and modernized to ensure accuracy and uniformity.
Although the abovementioned criteria have been widely adopted, they all lead to destructive end-points in analyses of the scaffold, and the degree of decellularization cannot be determined in real-time. Consequently, it is difficult to tailor decellularization to the distinctive attributes of each liver. Therefore, it is essential to develop a new non-invasive method to monitor and adjust decellularization in real-time to achieve individualization (Hulsmann et al., 2015; Geerts et al., 2016).
Akhyari et al. employed rheological evaluation and biomass compositional analysis of the perfusate for the real-time and non-invasive monitoring of heart decellularization. They discovered that the total protein, DNA, and biomass content in the perfusate was proportional to its viscosity. Based on this finding, the decellularization protocol could be adapted for a single organ. Furthermore, this finding demonstrated that the adverse effects of reagents on the ECM could be minimized if the pump profile, perfusion pressure, and revolution speed in the feedback control system were controlled (Hulsmann et al., 2015). Geerts et al. found that the HU value in CT scans was positively correlated with the quantity of DNA remaining in the scaffold during liver decellularization. These results suggest that the residual DNA could be used to predict the extent of decellularization. This established methodology when combined with characterization of the perfusate could be optimized to create a well-preserved scaffold (Geerts et al., 2016).
3.5.2 Quality Assessment of Decellularized Liver Scaffold
As the mold for liver engineering, the quality of the decellularized scaffold is crucial for successful liver recellularization and transplantation. However, there is currently no unified criteria for assessing the quality of the scaffold. The majority of qualitative and semi-quantitative studies have used morphological and molecular characteristics to assess the decellularized scaffold. The morphological assessment uses the appearance, HE staining, and SEM of the scaffold to evaluate its remaining microscopic anatomy and three-dimensional structure. The molecular assessment includes quantitative assessment of the residual DNA, cytokines, and ECM proteins. In addition, many studies have compared the mechanical strength, tension resistance, and other physical properties of the decellularized liver with those of the native liver (Everwien et al., 2020a).
Philipp et al. recently used macroscopic, microscopic, and morphological assessments to develop a morphological workflow for analyzing the microstructure of decellularized mouse liver scaffolds with mild histological damage (hepatic steatosis, hepatic fibrosis, and nodular regenerative hyperplasia) (details presented in Table 2). The results indicated that this procedure aided in evaluating the quality of decellularized scaffolds and in identifying the tissues with the best-preserved microstructure and matrix components; these results are also fundamental for future repopulation and transplantation trials (Felgendreff et al., 2021).
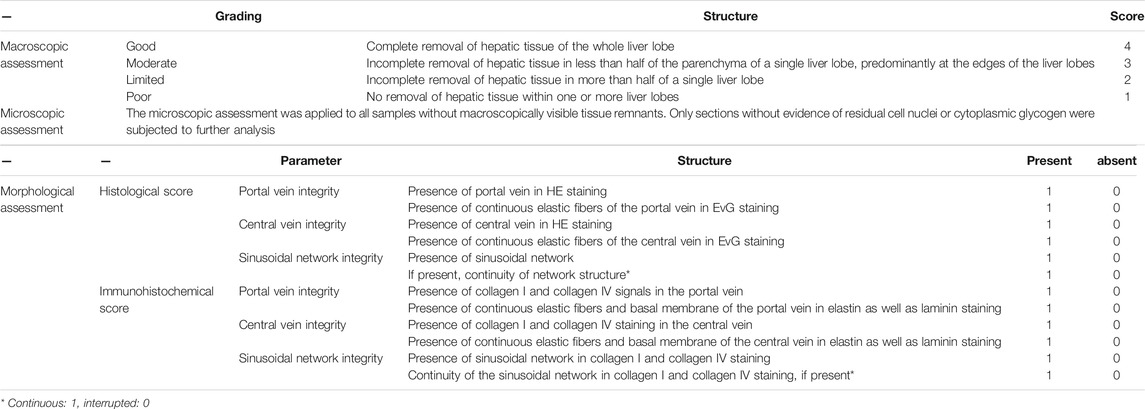
TABLE 2. The sequential morphological workflow to identify liver scaffold-sections with well-preserved microarchitecture.
However, morphological scoring methods vary depending on the expertise and subjectivity of the observer. Therefore, it is necessary to create digital image analysis to decrease this variance and achieved increased accuracy. Magliaro et al. developed a faster and more intuitive open-source image analysis software, HisTOOLogy, and applied it to analyze the effects of different decellularization protocols, showing a positive linear relationship between cell count and eosin staining area with total DNA and total protein quantification (Magliaro et al., 2015). The results demonstrated HisTOOLogy’s ability to analyze and provide quantitative information on histological sections, which aids in the efficiency and accuracy of evaluating decellularized scaffolds (Mattei et al., 2017). Besides, Moulisová et al. combined semiquantitative histological analysis with a newly developed software application (ScaffAn), which uses automated image analysis (details presented in Table 3) (Moulisova et al., 2020), to create a novel multi-scale morphological evaluation system for determining the quality of decellularized liver scaffolds. Comparatively, the conventional semi-quantitative histological analysis is based on seven criteria and a matching three-level grading system, which uses the morphological quality based on HE staining and SEM. In the quantitative analysis, the length of the sinusoidal structure and number of sinusoidal network branches are calculated based on high-resolution slide scans of the HE stains by using the newly developed software. Therefore, this approach complements the conventional analysis and effectively improves the discriminative power.
Along with morphological and molecular characteristics, mechanical properties also play a significant role in hepatic homeostasis. Likewise, the viscoelastic properties of the scaffold are critical to the morphology, viability, and metabolic activities of the implanted cells in liver engineering. For example, stiff or floppy scaffolds and substrates resulted in decreased vitality, spreading, and albumin (ALB) expression in repopulated HepG2 cells and hepatocytes (You et al., 2013; Mattei et al., 2018). Thus, establishing comprehensive and integrated decellularization assessment criteria will improve the quality and clinical translation of decellularized liver scaffolds.
4 Recellularization
The decellularized scaffold must be repopulated with functional cells to execute various physiological functions. The most vital cells are hepatic parenchymal cells, hepatocytes; however, the long-term in vitro survival of hepatocytes remains challenging. In addition, loss of the typical morphology and liver-specific functions provide evidence that the prolonged culturing of hepatocytes results in de-differentiation. Nonetheless, different stem cells and endothelial cells that have been applied in recellularization show great potential.
4.1 Primary Hepatocyte
Healthy livers can regenerate rapidly to maintain their standard size and function after a hepatectomy of 70%. However, primary hepatocytes scarcely proliferate in vitro. Therefore, obtaining sufficient viable hepatocytes is the biggest impediment to using primary hepatocytes for recellularization. The primary hepatocytes in a decellularized liver scaffold, cultured in a dynamic perfusion system, have higher viability, function, and can most crucially, proliferate, when compared to those cultured under static conditions (Kojima et al., 2018; Yang et al., 2018; Debnath et al., 2020; Sassi et al., 2021). Nonetheless, the prolonged culturing of primary hepatocytes results in the onset of apoptosis and a persistent decline in the function of certain cells. For example, the synthetic and metabolic abilities of primary hepatocytes were severely diminished after 1 week of recellularization, although histologically viable hepatocytes could still be observed (Butter et al., 2017; Debnath et al., 2020). Fetal hepatocytes have strong proliferative capacity and are a promising cell source for recellularization because of the progenitor cells in the fetal liver (Gridelli et al., 2012; Ogiso et al., 2016). Proliferation markers of fetal hepatocytes are 45-fold higher than those of adult hepatocytes, and reach the level of immortalized HepG2 cells. However, their hepatic function is relatively limited, and their use has generated much ethical concern (Gridelli et al., 2012; Ogiso et al., 2016).
4.2 Stem Cells
4.2.1 Embryonic Stem Cell
Embryonic stem cells (ESCs) are pluripotent cells that are derived from the blastocyst stage of early mammalian embryos and can differentiate into various cell types and are capable of self-renewal (Damdimopoulou et al., 2016; Zhao H. et al., 2020). Under certain culture conditions, ESCs can differentiate into functional hepatocytes, cholangiocytes, and endothelial cells. Therefore, they are a promising source of cells for liver engineering (Touboul et al., 2010; Dianat et al., 2014; Lorvellec et al., 2017; Zhao H. et al., 2020). In contrast with static cultures, ESCs differentiate more efficiently in the liver scaffold, expressing less of the fetal hepatocyte marker, alpha fetoprotein (AFP), and more of the adult hepatocyte indicators, ALB, and lipid, as well as increased production of glycogen; however, ECSs have only half the level of ALB expressed in induced pluripotent stem cells (iPSCs) (Lorvellec et al., 2017). The primary concern for implanting ESCs into a scaffold is tumorigenicity, namely the development of teratoma and teratocarcinoma (Ben-David and Benvenisty, 2011; Damdimopoulou et al., 2016). The primary strategies for improving the safety of ESCs include ensuring ultimate differentiation, eliminating remnant pluripotent stem cells, interfering with the genes associated with tumor progression, and routine post-transplant monitoring (Ben-David and Benvenisty, 2011; Damdimopoulou et al., 2016).
Furthermore, ESCs research is ethically controversial because the extraction of ESCs necessitates the destruction of embryos (Damdimopoulou et al., 2016). It has instead been proposed that ESCs can be obtained from surplus embryos that are used for in vitro fertilization with the consent of the donor (Damdimopoulou et al., 2016). It is also feasible to isolate ESCs from cloned embryos using donor eggs and patient cells, and even individual blastocyst cells without harming the embryos (Rodin et al., 2014; Damdimopoulou et al., 2016).
4.2.2 Induced Pluripotent Stem Cells
IPSCs can be obtained from adult tissue cells, where each pluripotent stem cell line is unique and would not cause an immune rejection response (Ben-David and Benvenisty, 2011; Vasylovska et al., 2020). IPSCs and derived hepatocyte-like cells (iPSC-HLCs) are likely cell sources for liver engineering. For example, human iPSC-HLCs and rat decellularized liver scaffolds were used to develop an artificial liver that could express ALB and CYP3A4. The new liver exhibited characteristic hepatic functions, such as protein synthesis and drug metabolism (Minami et al., 2019; Lorvellec et al., 2020). Nonetheless, AFP was still expressed in the recellularized liver, and the level of ALB in the recellularized liver was approximately 1/5 that of human iPSC-HLCs in vitro and 1/100 that of the recellularized liver with adult rat primary hepatocytes (Minami et al., 2019; Lorvellec et al., 2020). These results indicate that the HiPSC-HLCs in the de novo liver were immature, and induction and isolation of the HiPSC-HLCs caused cellular damage and reduced the functioning of hepatocytes. Takeishi implanted human iPSC-derived hepatocytes, endothelial cells, and cholangiocytes into rat liver scaffolds to generate a mini liver, which achieved 75% coverage of the liver vasculature and 66% coverage of the bile ducts (Takeishi et al., 2020). The human iPSC-derived mini liver had cell-cell and cell-ECM molecules, and functioned in immunocompromised rats for 4 days after auxiliary liver transplantation (Takeishi et al., 2020). Therefore, it is feasible to create a patient-specific bioengineered liver without immunogenicity. Additionally, the tumorigenic properties of iPSCs are roughly comparable to those of ESCs, and even iPSCs are more tumorigenic than ESCs because of genetic and epigenetic reasons (Ben-David and Benvenisty, 2011).
4.2.3 Mesenchymal Stem Cells
Mesenchymal stem cells (MSCs) are multipotent stem cells with high proliferative capacity for self-renewal and can differentiate into the cell lines of the resident tissues (Li et al., 2017; Alfaifi et al., 2018; Kang et al., 2020). MSCs were initially identified and isolated from bone marrow. However, this approach requires invasive procedures, yields limited cell numbers, and the differentiation ability declines with aging. MSCs have also been isolated from the umbilical cord, adipose tissue, placenta, synovial membrane, and amniotic fluid (Alfaifi et al., 2018; Kang et al., 2020). The umbilical cord is the ideal source of MSCs because these cells are easily accessible and are more primitive, abundant, and proliferative than cells from other tissues (Alfaifi et al., 2018). Adipose tissue is also an alternative source of MSCs, where these cells have high proliferative capacity and are easily obtained by adiposuction (Kang et al., 2020). For example, after cultivation with human umbilical MSCs, a porcine liver scaffold expressed liver-specific proteins ALB, CK-18, and glycogen, and successfully differentiated from the hUC-MSC phenotype to the primary human hepatocyte phenotype (Li et al., 2017). More importantly, unlike ESCs and iPSCs, MCSs are devoid of ethical and tumorigenic issues, making them potential sources of cells for liver engineering (Xiang et al., 2016; Li et al., 2017).
4.3 Endothelial Cells
The daedal vasculature system of the liver is indispensable in metabolite transport and homeostasis. Therefore, endothelialization of the decellularized scaffold is crucial for successfully reconstructing a bioengineered liver. Currently, human umbilical vein endothelial cells (HUVECs) and LSECs are the most frequently used endothelial cells. To create an ideal whole liver scaffold, Verstegen et al. decellularized human discarded liver and demonstrated that implanting HUVECs into the scaffold effectively rebuilt the endothelial system (Verstegen et al., 2017). Similarly, recellularized livers with HUVECs, which were implanted in immunosuppressed pigs, supported perfusion for up to 15 days (Shaheen et al., 2020). Instillation of LSECs in rat decellularized livers forms an endothelial layer that reduces platelet deposition and improves the function of the co-implanted hepatocytes (Kojima et al., 2018).
The vasculature in the liver has a hierarchy, which involves the hepatic sinusoids to the larger vessels. However, most studies on revascularization have focused on larger vessels, instead of the microvasculature in the hepatic sinusoids. The absence of basement membrane resulted in severe damage to the microstructure of the hepatic sinusoids during decellularization. As previously mentioned (Section 2.1), the hepatic sinusoids and space of Disse are the sites of substance exchange between the liver and the blood, making microvascular revascularization at the sinusoids top-drawer to the new liver function. Watanabe et al. successfully used HUVECs coated with fibronectin and flow-induced mechanical stimulation to reconstruct the microvessels of the hepatic sinusoids (Watanabe et al., 2019). However, HUVECs and LSECs have distinct cell surface markers and permeability as aforementioned (Section 2.1), and LSECs are the preferred cells for re-endothelialization (Poisson et al., 2017; Shetty et al., 2018).
4.4 Combination
Several cells collaboratively accomplish hepatic functions; therefore, the liver cannot be recellularized with only 1 cell type to perform complicated hepatic functions. To generate a competent liver, the decellularized scaffold must be methodically infused with various cells, and these cells grow in the right site. A combination of the HA, PV, vena cava, and common bile ducts can be used for recellularlization. The common bile duct guides the hepatocytes to their anatomical sites, and re-endothelialization of the HA or PV can prevent thrombosis and guarantee sufficient blood perfusion to the rebuilt liver.
Cholangiocytes and hepatocytes that are perfused into the liver via the common bile duct and PV, respectively, can rebuild bile duct-like structures, while hepatocytes can settle in the liver parenchyma (Chen et al., 2019). In addition, hepatocytes and LSECs can be distributed in the hepatic parenchymal region and PV lumen after perfusion via the common bile duct and PV, respectively (Kojima et al., 2018). Takeishi demonstrated that the implantation of human iPSC-derived hepatocytes, endothelial cells, and cholangiocytes could cover the liver vasculature and bile ducts of decellularized rat liver (Takeishi et al., 2020). Anderson et al. implanted primary porcine hepatocytes into liver scaffolds previously reendothelialized with HUVECs could achieve ALB production, ammonia detoxification, and urea synthesis. Additionally, they found that the ectopic transplantation of engineered livers into pigs that have undergone a portocaval shunt can slow the accumulation of ammonia in the blood (Anderson et al., 2021). Kakabadze et al. discovered that the perfusion of liver tissue fragments that contained all cell types successfully hepatized decellularized placenta, resulting in the expression of ALB and glycogen (Kakabadze et al., 2018). The histological results also revealed that the hepatic cord and hepatic sinusoids were lined with endothelial cells, Kupffer cells, and other types of cells. The animal experimental results also indicated that the implantation of a hepatized placenta could prevent acute liver failure caused by excessive hepatectomy (Kakabadze et al., 2018). Multi-step perfusion and pressure control have also been shown to improve the implantation efficiency, cellular function, and microvascular formation in hepatic sinusoids (Soto-Gutierrez et al., 2011; Watanabe et al., 2019; Anderson et al., 2021).
5 Main Obstacles
5.1 Thrombogenicity
The primary advantage of whole decellularized liver scaffolds is that the intact vascular network is preserved. However, this advantage is at the expense of losing the endothelial layer (Shaheen et al., 2020). In the absence of an endothelial layer, the exposed components of the ECM, especially collagen, trigger platelet activation and aggregation, and generate thrombin and fibrin, which initiates the formation of a thrombus (Figure 3A). Additionally, residual DNA may activate platelets and exert significant pro-inflammatory and pro-thrombotic effects in the extracellular space, hence promoting the development of immunothrombosis (Fuchs et al., 2010; Shi et al., 2020). The resultant thrombosis contributes to an inadequate supply of nutrients and oxygen to the implanted cells, which impedes the long-term survival of the neo-liver (Sassi et al., 2021). Thus, re-establishing blood flow to the new liver entails inhibiting thrombosis and constructing a new endothelial layer. Heparin is the most prevalent anticoagulant and is extensively used in the lining of grafts to improve their hemocompatibility (Figure 3B) (Wu et al., 2021). Both in vivo and in vitro studies have shown that the layer-by-layer deposition of heparin onto decellularized scaffolds immobilizes heparin, which effectively prevents thrombosis, and neither recellularization nor the function of the hepatocytes is affected (Bruinsma BG et al., 2015; Liu et al., 2019). Jiang reported an easy-to-implement method for covalently binding collagen-binding peptides to heparin, where the modified heparin was selectively bound to collagen and dramatically reduced the adhesion of platelets and formation of thrombi (Jiang et al., 2016). Furthermore, scaffolds that were recellularized with HUVECs maintained stable blood perfusion under physiological stress when ectopically implanted in immunosuppressed pigs (Shaheen et al., 2020; Anderson et al., 2021). However, no study to date has achieved long-term in vivo perfusion because of imperfect revascularization, which remains a critical problem that must be addressed.
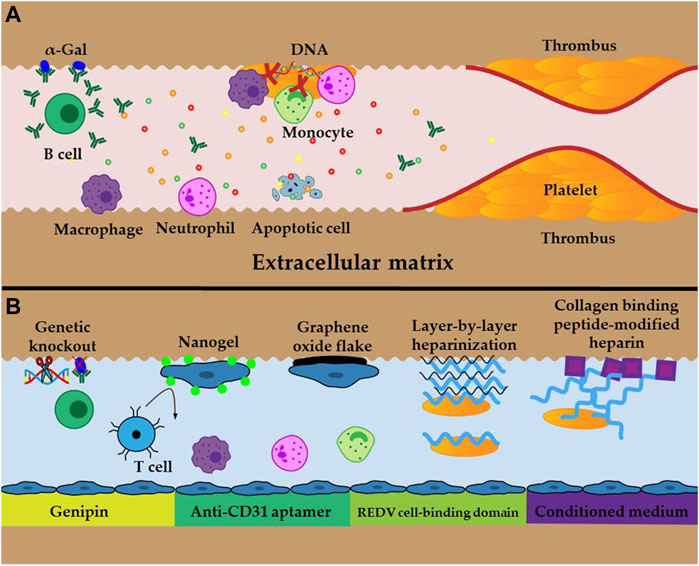
FIGURE 3. Schematic illustration of obstacles and approaches (A) Inflammatory response, oxidative stress, cell apoptosis, and thrombosis caused by residual DNA and Gal epitopes and exposed ECM (B) Examples of cross-linking and techniques to improve immunogenicity and thrombosis.
5.2 Anoikis
Anoikis is a particular type of apoptosis that results from the disruption of cell adhesion to the ECM (Chiarugi and Giannoni, 2008). Ex vivo perfusion and restoration of blood flow after transplantation generate reactive oxygen species (ROS) that hamper cell adhesion to the ECM and induce anoikis (Figure 3A), leading to low engraftment and survival of the implanted cells (Park et al., 2015). In addition, oxidative stress and inflammation suppress cell proliferation and enhance cellular senescence and death, which further reduce the success of the engrafted cells (Panahi et al., 2020). Therefore, it has been proposed that the transplant should be supplemented with antioxidants to scavenge ROS to increase the tolerance of the implanted cells to apoptosis. In addition, cross-linking approaches, such as the use of nanogels, graphene oxide sheets, anti-CD31 inducers, conditioned media, and REDV cell binding domains, also significantly improve cell adhesion to the ECM and increase engraftment in the implant (Figure 3B) (Park et al., 2015; Tang et al., 2017; Devalliere et al., 2018; Caires-Junior et al., 2021; Kim et al., 2021). These approaches are promising for the long-term survival of bioengineered livers because they increase cell engraftment and survival rates, which accelerate and improve vasculature reconstruction.
5.3 Immunogenicity
The prerequisite for the long-term in vivo survival of recellularized livers is low immunogenicity, which can be determined from the critical indicators of xenoantigenic residues (Figure 3A), such as residual DNA and galactose-a1,3-galactose (a-Gal) (Liu et al., 2018; Stahl et al., 2018). Reducing residual DNA and a-Gal involves improving the decellularization protocol and genetic knockout of a-Gal; these are proven approaches that diminish the immune response after implantation (Figure 3B) (Liu et al., 2018; Stahl et al., 2018). When sections of the liver scaffold were implanted subcutaneously in live animals, no immunogenicity was observed after 3 weeks, and there was low immunogenicity after 10 weeks (Mirmalek-Sani et al., 2013). Conversely, significant lymphocytic infiltration was observed 1 week after implantation, whereas at 3 weeks, the graft was almost completely degraded, and therefore, the inflammatory response was significantly reduced (Wang et al., 2016). Cross-linking the decellularized scaffolds with genipin and glutaraldehyde could minimize exposure of the immune cells to the ECM and increase cell adhesion of the implanted cells to the ECM (Figure 3B). When this approach is combined with immunosuppressive therapy, it can further improve the biocompatibility and long-term survival of the graft (Wang et al., 2016). Compared with glutaraldehyde, the genipin-crosslinked liver ECM exhibited superior biocompatibility and mechanical characteristics, and the in vivo immune responses of the host were lower (Gao et al., 2019). The graft from recellularized whole livers that was transplanted into living pigs was lost within 3 days without immunosuppression. Comparatively, this time period increased to more than 15 days when an immunosuppressive regimen was applied to the decellularized liver, and can potentially last longer if the immunosuppressive treatment is sustained (Shaheen et al., 2020). Therefore, more research into the concrete mechanism of immune rejection and strategies for suppressing immune rejection are critical for the long-term survival of bioengineered livers.
5.4 Shortage of Human Liver Scaffolds
Due to the shortage of discarded human livers, some researchers have proposed to populate human cells into the decellularized animal liver scaffolds to mimic human livers for transplantation. Xenotransplantation of genetically modified pig organs has attracted significant attention in recent years, particularly following the recent transplantation of a genetically modified pig kidney into a brain-dead human (Lu et al., 2019; Cooper, 2021). In contrast to the unsatisfactory microstructure of discarded human livers, animal livers are ideal and readily available. Human cells can colonize, proliferate, differentiate, and express ALB and glycogen in animal liver scaffolds (Li et al., 2017; Shaheen et al., 2020). However, the use of xenogeneic liver in human transplantation raises concerns due to the presence of the a-Gal. It has been shown that decellularized a-Gal knockout pig tissues behaved comparably to decellularized wild-type pig tissues (Stahl et al., 2018). Removing the a-Gal gene epitope reduced the adverse immune response of the recipient associated with prolonged exposure to decellularized xenografts (Stahl et al., 2018). Nevertheless, substantial concern has been expressed regarding the possibility of transmitting porcine endogenous retroviruses to the recipient and caregivers (Cooper, 2021).
Because of the risk of transmitting zoonosis, and immunological rejection of the xenogeneic liver, it is worthwhile to seek alternative human organs to replace the liver scaffold. The spleen and placenta are suitable alternative sources of scaffolds for bioengineered livers because they are readily available, have a vascular network for arterial and venous blood exchange, and contain ECM with diverse growth factors that support cell implantation (Maltepe and Fisher, 2015; Xiang et al., 2015; Liu et al., 2019). Decellularized spleen and placenta that are repopulated with primary hepatocytes and fresh liver fragments showed a characteristic hepatic cord structure, and expressed notable levels of ALB, urea, and glycogen after their ectopic transplantation into rats and sheep (Kakabadze et al., 2018; Liu et al., 2019). Moreover, this approach can mitigate against acute liver failure caused by extensive hepatectomy and promote regeneration of the original impaired liver. Consequently, other organs, such as the spleen and placenta, should be further explored as options to diversify the supply of organs for liver engineering (Kakabadze et al., 2018).
6 Conclusion and Prospects
This review discusses the structure of liver and hepatic ECM, and highlights the recent advances in decellularization and recellularization of the liver. An evaluation of decellularized scaffolds and obstacles is also presented. Although scaffold-based liver is a promising option for addressing the scarcity of donor liver, there are many limitations to the practical application of decellularized scaffolds: 1) ineluctable damage and denaturation of ECM proteins during decellularization, which hinders adhesion and proliferation of implanted cells; 2) imperfect revascularization, which lowers the hemocompatibility of the scaffold and increases the risk of thrombosis; and 3) elusory immune response, which causes inflammatory and rejection responses, resulting in loss of the graft. Future research that concentrates on these limitations will be valuable. Additionally, a better understanding of decellularization and recellularization of the liver is fundamental for producing transplantable liver grafts and ensuring the long-term survival of these grafts, especially if these insights are coupled with a deeper understanding of the mechanisms of the immune response.
Author Contributions
QD and WJ summarized and wrote the article. FH, FS, JZ, and HZ commented and revised the submitted version.
Funding
This work was funded by the National Natural Science Foundation of China (grant number 81701073).
Conflict of Interest
The authors declare that the research was conducted in the absence of any commercial or financial relationships that could be construed as a potential conflict of interest.
Publisher’s Note
All claims expressed in this article are solely those of the authors and do not necessarily represent those of their affiliated organizations, or those of the publisher, the editors and the reviewers. Any product that may be evaluated in this article, or claim that may be made by its manufacturer, is not guaranteed or endorsed by the publisher.
References
Acun, A., Oganesyan, R., Uygun, K., Yeh, H., Yarmush, M. L., and Uygun, B. E. (2021). Liver Donor Age Affects Hepatocyte Function through Age-dependent Changes in Decellularized Liver Matrix. Biomaterials 270, 120689. doi:10.1016/j.biomaterials.2021.120689
Ahmed, E., Saleh, T., Yu, L., Song, S. H., Park, K. M., Kwak, H. H., et al. (2020). Decellularized Extracellular Matrix‐rich Hydrogel-Silver Nanoparticle Mixture as a Potential Treatment for Acute Liver Failure Model. J. Biomed. Mater. Res. 108 (12), 2351–2367. doi:10.1002/jbm.a.36988
Alfaifi, M., Eom, Y. W., Newsome, P. N., and Baik, S. K. (2018). Mesenchymal Stromal Cell Therapy for Liver Diseases. J. Hepatol. 68 (6), 1272–1285. doi:10.1016/j.jhep.2018.01.030
Anderson, B. D., Nelson, E. D., Joo, D., Amiot, B. P., Katane, A. A., Mendenhall, A., et al. (2021). Functional Characterization of a Bioengineered Liver after Heterotopic Implantation in Pigs. Commun. Biol. 4 (1), 1157. doi:10.1038/s42003-021-02665-2
Ben-David, U., and Benvenisty, N. (2011). The Tumorigenicity of Human Embryonic and Induced Pluripotent Stem Cells. Nat. Rev. Cancer 11 (4), 268–277. doi:10.1038/nrc3034
Brown, B. N., Freund, J. M., Han, L., Rubin, J. P., Reing, J. E., Jeffries, E. M., et al. (2011). Comparison of Three Methods for the Derivation of a Biologic Scaffold Composed of Adipose Tissue Extracellular Matrix. Tissue Eng. C: Methods 17 (4), 411–421. doi:10.1089/ten.TEC.2010.0342
Bruinsma Bg, K. Y., Berendsen, T. A., Ozer, S., Yarmush, M. L., and Uygun, B. E. (2015). Layer-by-layer Heparinization of Decellularized Liver Matrices to Reduce Thrombogenicity of Recellularized Liver Grafts. JCTRes 1, 48–56. doi:10.18053/jctres.201501.004
Bühler, N. E. M., Schulze-Osthoff, K., Königsrainer, A., and Schenk, M. (2015). Controlled Processing of a Full-Sized Porcine Liver to a Decellularized Matrix in 24 H. J. Biosci. Bioeng. 119 (5), 609–613. doi:10.1016/j.jbiosc.2014.10.019
Butter, A., Aliyev, K., Hillebrandt, K. H., Raschzok, N., Kluge, M., Seiffert, N., et al. (2017). Evolution of Graft Morphology and Function after Recellularization of Decellularized Rat Livers. J. Tissue Eng. Regen. Med. 12 (2), e807–e816. doi:10.1002/term.2383
Caires-Júnior, L. C., Goulart, E., Telles-Silva, K. A., Araujo, B. H. S., Musso, C. M., Kobayashi, G., et al. (2021). Pre-coating Decellularized Liver with HepG2-Conditioned Medium Improves Hepatic Recellularization. Mater. Sci. Eng. C 121, 111862. doi:10.1016/j.msec.2020.111862
Chakraborty, J., Roy, S., and Ghosh, S. (2020). Regulation of Decellularized Matrix Mediated Immune Response. Biomater. Sci. 8 (5), 1194–1215. doi:10.1039/c9bm01780a
Changchen, W., Hongquan, W., Bo, Z., Leilei, X., Haiyue, J., and Bo, P. (2021). The Characterization, Cytotoxicity, Macrophage Response and Tissue Regeneration of Decellularized Cartilage in Costal Cartilage Defects. Acta Biomater. 136, 147–158. doi:10.1016/j.actbio.2021.09.031
Cheemerla, S., and Balakrishnan, M. (2021). Global Epidemiology of Chronic Liver Disease. Clin. Liver Dis. 17 (5), 365–370. doi:10.1002/cld.1061
Chen, Y., Devalliere, J., Bulutoglu, B., Yarmush, M. L., and Uygun, B. E. (2019). Repopulation of Intrahepatic Bile Ducts in Engineered Rat Liver Grafts. Technology 07 (1-2), 46–55. doi:10.1142/s2339547819500043
Cheng, J., Wang, C., and Gu, Y. (2019). Combination of Freeze-Thaw with Detergents: A Promising Approach to the Decellularization of Porcine Carotid Arteries. Bme 30 (2), 191–205. doi:10.3233/BME-191044
Chiarugi, P., and Giannoni, E. (2008). Anoikis: a Necessary Death Program for anchorage-dependent Cells. Biochem. Pharmacol. 76 (11), 1352–1364. doi:10.1016/j.bcp.2008.07.023
Choudhury, D., Yee, M., Sheng, Z. L. J., Amirul, A., and Naing, M. W. (2020). Decellularization Systems and Devices: State-Of-The-Art. Acta Biomater. 115, 51–59. doi:10.1016/j.actbio.2020.07.060
Cooper, D. K. C. (2021). Genetically Engineered Pig Kidney Transplantation in a Brain‐dead Human Subject. Xenotransplantation 28 (6), e12718. doi:10.1111/xen.12718
Coronado, R. E., Somaraki-Cormier, M., Natesan, S., Christy, R. J., Ong, J. L., and Halff, G. A. (2017). Decellularization and Solubilization of Porcine Liver for Use as a Substrate for Porcine Hepatocyte Culture. Cel Transpl. 26 (12), 1840–1854. doi:10.1177/0963689717742157
Damdimopoulou, P., Rodin, S., Stenfelt, S., Antonsson, L., Tryggvason, K., and Hovatta, O. (2016). Human Embryonic Stem Cells. Best Pract. Res. Clin. Obstet. Gynaecol. 31, 2–12. doi:10.1016/j.bpobgyn.2015.08.010
Dang, L. H., Tseng, Y., Tseng, H., and Hung, S.-H. (2021). Partial Decellularization for Segmental Tracheal Scaffold Tissue Engineering: A Preliminary Study in Rabbits. Biomolecules 11 (6), 866. doi:10.3390/biom11060866
Debnath, T., Mallarpu, C. S., and Chelluri, L. K. (2020). Development of Bioengineered Organ Using Biological Acellular Rat Liver Scaffold and Hepatocytes. Organogenesis 16 (2), 61–72. doi:10.1080/15476278.2020.1742534
Devalliere, J., Chen, Y., Dooley, K., Yarmush, M. L., and Uygun, B. E. (2018). Improving Functional Re-endothelialization of Acellular Liver Scaffold Using REDV Cell-Binding Domain. Acta Biomater. 78, 151–164. doi:10.1016/j.actbio.2018.07.046
Dianat, N., Dubois‐Pot‐Schneider, H., Steichen, C., Desterke, C., Leclerc, P., Raveux, A., et al. (2014). Generation of Functional Cholangiocyte‐like Cells from Human Pluripotent Stem Cells and HepaRG Cells. Hepatology 60 (2), 700–714. doi:10.1002/hep.27165
Everwien, H., Ariza de Schellenberger, A., Haep, N., Tzschätzsch, H., Pratschke, J., Sauer, I. M., et al. (2020a). Magnetic Resonance Elastography Quantification of the Solid-To-Fluid Transition of Liver Tissue Due to Decellularization. J. Mech. Behav. Biomed. Mater. 104, 103640. doi:10.1016/j.jmbbm.2020.103640
Everwien, H., Keshi, E., Hillebrandt, K. H., Ludwig, B., Weinhart, M., Tang, P., et al. (2020b). Engineering an Endothelialized, Endocrine Neo-Pancreas: Evaluation of Islet Functionality in an Ex Vivo Model. Acta Biomater. 117, 213–225. doi:10.1016/j.actbio.2020.09.022
Faccioli, L. A. P., Dias, G. S., Hoff, V., Dias, M. L., Pimentel, C. F., Hochman-Mendez, C., et al. (2020). Optimizing the Decellularized Porcine Liver Scaffold Protocol. Cells Tissues Organs 211, 1–10. doi:10.1159/000510297
Felgendreff, P., Schindler, C., Mussbach, F., Xie, C., Gremse, F., Settmacher, U., et al. (2021). Identification of Tissue Sections from Decellularized Liver Scaffolds for Repopulation Experiments. Heliyon 7 (2), e06129. doi:10.1016/j.heliyon.2021.e06129
Flynn, L. E. (2010). The Use of Decellularized Adipose Tissue to Provide an Inductive Microenvironment for the Adipogenic Differentiation of Human Adipose-Derived Stem Cells. Biomaterials 31 (17), 4715–4724. doi:10.1016/j.biomaterials.2010.02.046
Fuchs, T. A., Brill, A., Duerschmied, D., Schatzberg, D., Monestier, M., Myers, D. D., et al. (2010). Extracellular DNA Traps Promote Thrombosis. Proc. Natl. Acad. Sci. 107 (36), 15880–15885. doi:10.1073/pnas.1005743107
Gao, M., Wang, Y., He, Y., Li, Y., Wu, Q., Yang, G., et al. (2019). Comparative Evaluation of Decellularized Porcine Liver Matrices Crosslinked with Different Chemical and Natural Crosslinking Agents. Xenotransplantation 26 (1), e12470. doi:10.1111/xen.12470
Gao, Y., Li, Z., Hong, Y., Li, T., Hu, X., Sun, L., et al. (2020). Decellularized Liver as a Translucent Ex Vivo Model for Vascular Embolization Evaluation. Biomaterials 240, 119855. doi:10.1016/j.biomaterials.2020.119855
Geerts, S., Ozer, S., Jaramillo, M., Yarmush, M. L., and Uygun, B. E. (2016). Nondestructive Methods for Monitoring Cell Removal during Rat Liver Decellularization. Tissue Eng. Part C: Methods 22 (7), 671–678. doi:10.1089/ten.TEC.2015.0571
Gordillo, M., Evans, T., and Gouon-Evans, V. (2015). Orchestrating Liver Development. Development 142 (12), 2094–2108. doi:10.1242/dev.114215
Gracia-Sancho, J., Caparrós, E., Fernández-Iglesias, A., and Francés, R. (2021). Role of Liver Sinusoidal Endothelial Cells in Liver Diseases. Nat. Rev. Gastroenterol. Hepatol. 18 (6), 411–431. doi:10.1038/s41575-020-00411-3
Gridelli, B., Vizzini, G., Pietrosi, G., Luca, A., Spada, M., Gruttadauria, S., et al. (2012). Efficient Human Fetal Liver Cell Isolation Protocol Based on Vascular Perfusion for Liver Cell-Based Therapy and Case Report on Cell Transplantation. Liver Transpl. 18 (2), 226–237. doi:10.1002/lt.22322
Guimaraes, A. B., Correia, A. T., Alves, B. P., Da Silva, R. S., Martins, J. K., Pêgo-Fernandes, P. M., et al. (2019). Evaluation of a Physical-Chemical Protocol for Porcine Tracheal Decellularization. Transplant. Proc. 51 (5), 1611–1613. doi:10.1016/j.transproceed.2019.01.042
Hülsmann, J., Aubin, H., Bandesha, S. T., Kranz, A., Stoldt, V. R., Lichtenberg, A., et al. (2015). Rheology of Perfusates and Fluid Dynamical Effects during Whole Organ Decellularization: a Perspective to Individualize Decellularization Protocols for Single Organs. Biofabrication 7 (3), 035008. doi:10.1088/1758-5090/7/3/035008
Hussein, K. H., Park, K.-M., Yu, L., Kwak, H.-H., and Woo, H.-M. (2020). Decellularized Hepatic Extracellular Matrix Hydrogel Attenuates Hepatic Stellate Cell Activation and Liver Fibrosis. Mater. Sci. Eng. C 116, 111160. doi:10.1016/j.msec.2020.111160
Jeong, W., Kim, M. K., and Kang, H.-W. (2021). Effect of Detergent Type on the Performance of Liver Decellularized Extracellular Matrix-Based Bio-Inks. J. Tissue Eng. 12, 204173142199709. doi:10.1177/2041731421997091
Jiang, B., Suen, R., Wertheim, J. A., and Ameer, G. A. (2016). Targeting Heparin to Collagen within Extracellular Matrix Significantly Reduces Thrombogenicity and Improves Endothelialization of Decellularized Tissues. Biomacromolecules 17 (12), 3940–3948. doi:10.1021/acs.biomac.6b01330
Kakabadze, Z., Kakabadze, A., Chakhunashvili, D., Karalashvili, L., Berishvili, E., Sharma, Y., et al. (2018). Decellularized Human Placenta Supports Hepatic Tissue and Allows rescue in Acute Liver Failure. Hepatology 67 (5), 1956–1969. doi:10.1002/hep.29713
Kang, S. H., Kim, M. Y., Eom, Y. W., and Baik, S. K. (2020). Mesenchymal Stem Cells for the Treatment of Liver Disease: Present and Perspectives. Gut and Liver 14 (3), 306–315. doi:10.5009/gnl18412
Kim, D.-H., Ahn, J., Kang, H. K., Kim, M.-S., Kim, N.-G., Kook, M. G., et al. (2021). Development of Highly Functional Bioengineered Human Liver with Perfusable Vasculature. Biomaterials 265, 120417. doi:10.1016/j.biomaterials.2020.120417
Kim, J. K., Koh, Y.-D., Kim, J. O., and Seo, D. H. (2016). Development of a Decellularization Method to Produce Nerve Allografts Using Less Invasive Detergents and Hyper/hypotonic Solutions. J. Plast. Reconstr. Aesthet. Surg. 69 (12), 1690–1696. doi:10.1016/j.bjps.2016.08.016
Kobes, J. E., Georgiev, G. I., Louis, A. V., Calderon, I. A., Yoshimaru, E. S., Klemm, L. M., et al. (2018). A Comparison of Iron Oxide Particles and Silica Particles for Tracking Organ Recellularization. Mol. Imaging 17, 153601211878732. doi:10.1177/1536012118787322
Kojima, H., Yasuchika, K., Fukumitsu, K., Ishii, T., Ogiso, S., Miyauchi, Y., et al. (2018). Establishment of Practical Recellularized Liver Graft for Blood Perfusion Using Primary Rat Hepatocytes and Liver Sinusoidal Endothelial Cells. Am. J. Transpl. 18 (6), 1351–1359. doi:10.1111/ajt.14666
Lewis, P. L., Su, J., Yan, M., Meng, F., Glaser, S. S., Alpini, G. D., et al. (2018). Complex Bile Duct Network Formation within Liver Decellularized Extracellular Matrix Hydrogels. Sci. Rep. 8 (1), 12220. doi:10.1038/s41598-018-30433-6
Li, P., Zhou, J., Li, W., Wu, H., Hu, J., Ding, Q., et al. (2020). Characterizing Liver Sinusoidal Endothelial Cell Fenestrae on Soft Substrates upon AFM Imaging and Deep Learning. Biochim. Biophys. Acta (Bba) - Gen. Subjects 1864 (12), 129702. doi:10.1016/j.bbagen.2020.129702
Li, Y., Wu, Q., Wang, Y., Li, L., Chen, F., Shi, Y., et al. (2017). Construction of Bioengineered Hepatic Tissue Derived from Human Umbilical Cord Mesenchymal Stem Cells via Aggregation Culture in Porcine Decellularized Liver Scaffolds. Xenotransplantation 24 (1), e12285. doi:10.1111/xen.12285
Lin, C.-H., Hsia, K., Su, C.-K., Chen, C.-C., Yeh, C.-C., Ma, H., et al. (2021). Sonication-Assisted Method for Decellularization of Human Umbilical Artery for Small-Caliber Vascular Tissue Engineering. Polymers 13 (11), 1699. doi:10.3390/polym13111699
Liu, P., Tian, B., Yang, L., Zheng, X., Zhang, X., Li, J., et al. (2019). Hemocompatibility Improvement of Decellularized Spleen Matrix for Constructing Transplantable Bioartificial Liver. Biomed. Mater. 14 (2), 025003. doi:10.1088/1748-605X/aaf375
Liu, X., Li, N., Gong, D., Xia, C., and Xu, Z. (2018). Comparison of Detergent-Based Decellularization Protocols for the Removal of Antigenic Cellular Components in Porcine Aortic Valve. Xenotransplantation 25 (2), e12380. doi:10.1111/xen.12380
Lorvellec, M., Pellegata, A. F., Maestri, A., Turchetta, C., Alvarez Mediavilla, E., Shibuya, S., et al. (2020). An In Vitro Whole-Organ Liver Engineering for Testing of Genetic Therapies. iScience 23 (12), 101808. doi:10.1016/j.isci.2020.101808
Lorvellec, M., Scottoni, F., Crowley, C., Fiadeiro, R., Maghsoudlou, P., Pellegata, A. F., et al. (2017). Mouse Decellularised Liver Scaffold Improves Human Embryonic and Induced Pluripotent Stem Cells Differentiation into Hepatocyte-like Cells. PLoS One 12 (12), e0189586. doi:10.1371/journal.pone.0189586
Lu, S., Cuzzucoli, F., Jiang, J., Liang, L.-G., Wang, Y., Kong, M., et al. (2018). Development of a Biomimetic Liver Tumor-On-A-Chip Model Based on Decellularized Liver Matrix for Toxicity Testing. Lab. Chip 18 (22), 3379–3392. doi:10.1039/c8lc00852c
Lu, T., Yang, B., Wang, R., and Qin, C. (2019). Xenotransplantation: Current Status in Preclinical Research. Front. Immunol. 10, 3060. doi:10.3389/fimmu.2019.03060
Maghsoudlou, P., Georgiades, F., Smith, H., Milan, A., Shangaris, P., Urbani, L., et al. (2016). Optimization of Liver Decellularization Maintains Extracellular Matrix Micro-architecture and Composition Predisposing to Effective Cell Seeding. PLoS One 11 (5), e0155324. doi:10.1371/journal.pone.0155324
Magliaro, C., Tirella, A., Mattei, G., Pirone, A., and Ahluwalia, A. (2015). HisTOOLogy: an Open-Source Tool for Quantitative Analysis of Histological Sections. J. Microsc. 260 (3), 260–267. doi:10.1111/jmi.12292
Mak, K. M., and Mei, R. (2017). Basement Membrane Type IV Collagen and Laminin: An Overview of Their Biology and Value as Fibrosis Biomarkers of Liver Disease. Anat. Rec. 300 (8), 1371–1390. doi:10.1002/ar.23567
Maltepe, E., and Fisher, S. J. (2015). Placenta: the Forgotten Organ. Annu. Rev. Cel Dev. Biol. 31, 523–552. doi:10.1146/annurev-cellbio-100814-125620
Manalastas, T. M., Dugos, N., Ramos, G., and Mondragon, J. M. (2021). Effect of Decellularization Parameters on the Efficient Production of Kidney Bioscaffolds. Appl. Biochem. Biotechnol. 193 (5), 1239–1251. doi:10.1007/s12010-020-03338-2
Mattei, G., Di Patria, V., Tirella, A., Alaimo, A., Elia, G., Corti, A., et al. (2014). Mechanostructure and Composition of Highly Reproducible Decellularized Liver Matrices. Acta Biomater. 10 (2), 875–882. doi:10.1016/j.actbio.2013.10.023
Mattei, G., Magliaro, C., Pirone, A., and Ahluwalia, A. (2018). Bioinspired Liver Scaffold Design Criteria. Organogenesis 14 (3), 129–146. doi:10.1080/15476278.2018.1505137
Mattei, G., Magliaro, C., Pirone, A., and Ahluwalia, A. (2017). Decellularized Human Liver Is Too Heterogeneous for Designing a Generic Extracellular Matrix Mimic Hepatic Scaffold. Artif. Organs 41 (12), E347–E355. doi:10.1111/aor.12925
Mazza, G., Telese, A., Al-Akkad, W., Frenguelli, L., Levi, A., Marrali, M., et al. (2019). Cirrhotic Human Liver Extracellular Matrix 3D Scaffolds Promote Smad-dependent TGF-Β1 Epithelial Mesenchymal Transition. Cells 9 (1), 83. doi:10.3390/cells9010083
McCrary, M. W., Bousalis, D., Mobini, S., Song, Y. H., and Schmidt, C. E. (2020). Decellularized Tissues as Platforms for In Vitro Modeling of Healthy and Diseased Tissues. Acta Biomater. 111, 1–19. doi:10.1016/j.actbio.2020.05.031
McQuitty, C. E., Williams, R., Chokshi, S., and Urbani, L. (2020). Immunomodulatory Role of the Extracellular Matrix within the Liver Disease Microenvironment. Front. Immunol. 11, 574276. doi:10.3389/fimmu.2020.574276
Minami, T., Ishii, T., Yasuchika, K., Fukumitsu, K., Ogiso, S., Miyauchi, Y., et al. (2019). Novel Hybrid Three-Dimensional Artificial Liver Using Human Induced Pluripotent Stem Cells and a Rat Decellularized Liver Scaffold. Regenerative Ther. 10, 127–133. doi:10.1016/j.reth.2019.03.002
Mirmalek-Sani, S.-H., Sullivan, D. C., Zimmerman, C., Shupe, T. D., and Petersen, B. E. (2013). Immunogenicity of Decellularized Porcine Liver for Bioengineered Hepatic Tissue. Am. J. Pathol. 183 (2), 558–565. doi:10.1016/j.ajpath.2013.05.002
Miyauchi, Y., Yasuchika, K., Fukumitsu, K., Ishii, T., Ogiso, S., Minami, T., et al. (2017). A Novel Three-Dimensional Culture System Maintaining the Physiological Extracellular Matrix of Fibrotic Model Livers Accelerates Progression of Hepatocellular Carcinoma Cells. Sci. Rep. 7 (1), 9827. doi:10.1038/s41598-017-09391-y
Moulisová, V., Jiřík, M., Schindler, C., Červenková, L., Pálek, R., Rosendorf, J., et al. (2020). Novel Morphological Multi-Scale Evaluation System for Quality Assessment of Decellularized Liver Scaffolds. J. Tissue Eng. 11, 204173142092112. doi:10.1177/2041731420921121
Müller, P. C., Kabacam, G., Vibert, E., Germani, G., and Petrowsky, H. (2020). Current Status of Liver Transplantation in Europe. Int. J. Surg. 82, 22–29. doi:10.1016/j.ijsu.2020.05.062
Narciso, M., Otero, J., Navajas, D., Farré, R., Almendros, I., and Gavara, N. (2021). Image-Based Method to Quantify Decellularization of Tissue Sections. Ijms 22 (16), 8399. doi:10.3390/ijms22168399
Nishiguchi, A., and Taguchi, T. (2021). A pH-Driven Genipin Gelator to Engineer Decellularized Extracellular Matrix-Based Tissue Adhesives. Acta Biomater. 131, 211–221. doi:10.1016/j.actbio.2021.06.033
O'Neill, J. D., Anfang, R., Anandappa, A., Costa, J., Javidfar, J., Wobma, H. M., et al. (2013). Decellularization of Human and Porcine Lung Tissues for Pulmonary Tissue Engineering. Ann. Thorac. Surg. 96 (3), 1046–1056. doi:10.1016/j.athoracsur.2013.04.022
Ogiso, S., Yasuchika, K., Fukumitsu, K., Ishii, T., Kojima, H., Miyauchi, Y., et al. (2016). Efficient Recellularisation of Decellularised Whole-Liver Grafts Using Biliary Tree and Foetal Hepatocytes. Sci. Rep. 6, 35887. doi:10.1038/srep35887
Panahi, M., Rahimi, B., Rahimi, G., Yew Low, T., Saraygord‐Afshari, N., and Alizadeh, E. (2020). Cytoprotective Effects of Antioxidant Supplementation on Mesenchymal Stem Cell Therapy. J. Cel Physiol 235 (10), 6462–6495. doi:10.1002/jcp.29660
Park, J., Kim, B., Han, J., Oh, J., Park, S., Ryu, S., et al. (2015). Graphene Oxide Flakes as a Cellular Adhesive: Prevention of Reactive Oxygen Species Mediated Death of Implanted Cells for Cardiac Repair. ACS Nano 9 (5), 4987–4999. doi:10.1021/nn507149w
Poisson, J., Lemoinne, S., Boulanger, C., Durand, F., Moreau, R., Valla, D., et al. (2017). Liver Sinusoidal Endothelial Cells: Physiology and Role in Liver Diseases. J. Hepatol. 66 (1), 212–227. doi:10.1016/j.jhep.2016.07.009
Prasertsung, I., Kanokpanont, S., Bunaprasert, T., Thanakit, V., and Damrongsakkul, S. (2008). Development of Acellular Dermis from Porcine Skin Using Periodic Pressurized Technique. J. Biomed. Mater. Res. 85B (1), 210–219. doi:10.1002/jbm.b.30938
Pulver, , Shevtsov, A., Leybovich, B., Artyuhov, I., Maleev, Y., and Peregudov, A. (2014). Production of Organ Extracellular Matrix Using a Freeze-Thaw Cycle Employing Extracellular Cryoprotectants. Cryo Lett. 35 (5), 400–406.
Reing, J. E., Brown, B. N., Daly, K. A., Freund, J. M., Gilbert, T. W., Hsiong, S. X., et al. (2010). The Effects of Processing Methods upon Mechanical and Biologic Properties of Porcine Dermal Extracellular Matrix Scaffolds. Biomaterials 31 (33), 8626–8633. doi:10.1016/j.biomaterials.2010.07.083
Rodin, S., Antonsson, L., Niaudet, C., Simonson, O. E., Salmela, E., Hansson, E. M., et al. (2014). Clonal Culturing of Human Embryonic Stem Cells on Laminin-521/e-Cadherin Matrix in Defined and Xeno-free Environment. Nat. Commun. 5, 3195. doi:10.1038/ncomms4195
Sassi, L., Ajayi, O., Campinoti, S., Natarajan, D., McQuitty, C., Siena, R. R., et al. (2021). A Perfusion Bioreactor for Longitudinal Monitoring of Bioengineered Liver Constructs. Nanomaterials 11 (2), 275. doi:10.3390/nano11020275
Say, S., Dugos, N. P., Roces, S. A., and Mondragon, J. M. (2019). Sonication-assisted Perfusion Decellularization of Whole Porcine Kidney. Int. J. Biol. Biomed. Eng. 13, 78–81.
Sengyoku, H., Tsuchiya, T., Obata, T., Doi, R., Hashimoto, Y., Ishii, M., et al. (2018). Sodium Hydroxide Based Non-detergent Decellularizing Solution for Rat Lung. Organogenesis 14 (2), 94–106. doi:10.1080/15476278.2018.1462432
Shaheen, M. F., Joo, D. J., Ross, J. J., Anderson, B. D., Chen, H. S., Huebert, R. C., et al. (2020). Sustained Perfusion of Revascularized Bioengineered Livers Heterotopically Transplanted into Immunosuppressed Pigs. Nat. Biomed. Eng. 4 (4), 437–445. doi:10.1038/s41551-019-0460-x
Shen, W., Berning, K., Tang, S. W., and Lam, Y. W. (2020). Rapid and Detergent-free Decellularization of Cartilage. Tissue Eng. Part C: Methods 26 (4), 201–206. doi:10.1089/ten.TEC.2020.0008
Shetty, S., Lalor, P. F., and Adams, D. H. (2018). Liver Sinusoidal Endothelial Cells - Gatekeepers of Hepatic Immunity. Nat. Rev. Gastroenterol. Hepatol. 15 (9), 555–567. doi:10.1038/s41575-018-0020-y
Shi, C., Yang, L., Braun, A., and Anders, H.-J. (2020). Extracellular DNA-A Danger Signal Triggering Immunothrombosis. Front. Immunol. 11, 568513. doi:10.3389/fimmu.2020.568513
Shimoda, H., Yagi, H., Higashi, H., Tajima, K., Kuroda, K., Abe, Y., et al. (2019). Decellularized Liver Scaffolds Promote Liver Regeneration after Partial Hepatectomy. Sci. Rep. 9 (1), 12543. doi:10.1038/s41598-019-48948-x
Song, A. T. W., Avelino-Silva, V. I., Pecora, R. A., Pugliese, V., D'Albuquerque, L. A., and Abdala, E. (2014). Liver Transplantation: Fifty Years of Experience. Wjg 20 (18), 5363–5374. doi:10.3748/wjg.v20.i18.5363
Soto-Gutierrez, A., Zhang, L., Medberry, C., Fukumitsu, K., Faulk, D., Jiang, H., et al. (2011). A Whole-Organ Regenerative Medicine Approach for Liver Replacement. Tissue Eng. Part C: Methods 17 (6), 677–686. doi:10.1089/ten.tec.2010.0698
Stahl, E. C., Bonvillain, R. W., Skillen, C. D., Burger, B. L., Hara, H., Lee, W., et al. (2018). Evaluation of the Host Immune Response to Decellularized Lung Scaffolds Derived from α-Gal Knockout Pigs in a Non-human Primate Model. Biomaterials 187, 93–104. doi:10.1016/j.biomaterials.2018.09.038
Stamataki, Z., and Swadling, L. (2020). The Liver as an Immunological Barrier Redefined by Single‐cell Analysis. Immunology 160 (2), 157–170. doi:10.1111/imm.13193
Struecker, B., Butter, A., Hillebrandt, K., Polenz, D., Reutzel-Selke, A., Tang, P., et al. (2017). Improved Rat Liver Decellularization by Arterial Perfusion under Oscillating Pressure Conditions. J. Tissue Eng. Regen. Med. 11 (2), 531–541. doi:10.1002/term.1948
Suss, P. H., Ribeiro, V. S. T., Motooka, C. E., de Melo, L. C., and Tuon, F. F. (2021). Comparative Study of Decellularization Techniques to Obtain Natural Extracellular Matrix Scaffolds of Human Peripheral-Nerve Allografts. Cell Tissue Bank. Online ahead of print. doi:10.1007/s10561-021-09977-x
Syed, O., Walters, N. J., Day, R. M., Kim, H.-W., and Knowles, J. C. (2014). Evaluation of Decellularization Protocols for Production of Tubular Small Intestine Submucosa Scaffolds for Use in Oesophageal Tissue Engineering. Acta Biomater. 10 (12), 5043–5054. doi:10.1016/j.actbio.2014.08.024
Tajima, K., Yagi, H., and Kitagawa, Y. (2018). Human-Scale Liver Harvest and Decellularization for Preclinical Research. Methods Mol. Biol. 1577, 327–335. doi:10.1007/7651_2018_195
Takeishi, K., Collin de l’Hortet, A., Wang, Y., Handa, K., Guzman-Lepe, J., Matsubara, K., et al. (2020). Assembly and Function of a Bioengineered Human Liver for Transplantation Generated Solely from Induced Pluripotent Stem Cells. Cel Rep. 31 (9), 107711. doi:10.1016/j.celrep.2020.107711
Tang, J., Cui, X., Caranasos, T. G., Hensley, M. T., Vandergriff, A. C., Hartanto, Y., et al. (2017). Heart Repair Using Nanogel-Encapsulated Human Cardiac Stem Cells in Mice and Pigs with Myocardial Infarction. ACS Nano 11 (10), 9738–9749. doi:10.1021/acsnano.7b01008
Tao, M., Liang, F., He, J., Ye, W., Javed, R., Wang, W., et al. (2021). Decellularized Tendon Matrix Membranes Prevent post-surgical Tendon Adhesion and Promote Functional Repair. Acta Biomater. 134, 160–176. doi:10.1016/j.actbio.2021.07.038
Thanapirom, K., Caon, E., Papatheodoridi, M., Frenguelli, L., Al-Akkad, W., Zhenzhen, Z., et al. (2021). Optimization and Validation of a Novel Three-Dimensional Co-culture System in Decellularized Human Liver Scaffold for the Study of Liver Fibrosis and Cancer. Cancers 13 (19), 4936. doi:10.3390/cancers13194936
Touboul, T., Hannan, N. R. F., Corbineau, S., Martinez, A., Martinet, C., Branchereau, S., et al. (2010). Generation of Functional Hepatocytes from Human Embryonic Stem Cells under Chemically Defined Conditions that Recapitulate Liver Development. Hepatology 51 (5), 1754–1765. doi:10.1002/hep.23506
Trefts, E., Gannon, M., and Wasserman, D. H. (2017). The Liver. Curr. Biol. 27 (21), R1147–R1151. doi:10.1016/j.cub.2017.09.019
van Steenberghe, M., Schubert, T., Guiot, Y., Bouzin, C., Bollen, X., and Gianello, P. (2017). Enhanced Vascular Biocompatibility of Decellularized Xeno-/allogeneic Matrices in a Rodent Model. Cell Tissue Bank 18 (2), 249–262. doi:10.1007/s10561-017-9610-0
van Steenberghe, M., Schubert, T., Xhema, D., Bouzin, C., Guiot, Y., Duisit, J., et al. (2018). Enhanced Vascular Regeneration with Chemically/physically Treated Bovine/human Pericardium in Rodents. J. Surg. Res. 222, 167–179. doi:10.1016/j.jss.2017.09.043
Vasylovska, S., Schuster, J., Brboric, A., Carlsson, P.-O., Dahl, N., and Lau, J. (2021). Generation of Human Induced Pluripotent Stem Cell (iPSC) Lines (UUMCBi001-A, UUMCBi002-A) from Two Healthy Donors. Stem Cel Res. 50, 102114. doi:10.1016/j.scr.2020.102114
Verstegen, M. M. A., Willemse, J., van den Hoek, S., Kremers, G.-J., Luider, T. M., van Huizen, N. A., et al. (2017). Decellularization of Whole Human Liver Grafts Using Controlled Perfusion for Transplantable Organ Bioscaffolds. Stem Cell Develop. 26 (18), 1304–1315. doi:10.1089/scd.2017.0095
Vishwakarma, S. K., Bardia, A., Lakkireddy, C., Raju, N., Paspala, S. A. B., Habeeb, M. A., et al. (2019). Intraperitoneal Transplantation of Bioengineered Humanized Liver Grafts Supports Failing Liver in Acute Condition. Mater. Sci. Eng. C 98, 861–873. doi:10.1016/j.msec.2019.01.045
Visscher, D. O., Lee, H., van Zuijlen, P. P. M., Helder, M. N., Atala, A., Yoo, J. J., et al. (2021). A Photo-Crosslinkable Cartilage-Derived Extracellular Matrix Bioink for Auricular Cartilage Tissue Engineering. Acta Biomater. 121, 193–203. doi:10.1016/j.actbio.2020.11.029
Wang, Y., Bao, J., Wu, X., Wu, Q., Li, Y., Zhou, Y., et al. (2016). Genipin Crosslinking Reduced the Immunogenicity of Xenogeneic Decellularized Porcine Whole-Liver Matrices through Regulation of Immune Cell Proliferation and Polarization. Sci. Rep. 6, 24779. doi:10.1038/srep24779
Watanabe, M., Yano, K., Okawa, K., Yamashita, T., Tajima, K., Sawada, K., et al. (2019). Construction of Sinusoid-Scale Microvessels in Perfusion Culture of a Decellularized Liver. Acta Biomater. 95, 307–318. doi:10.1016/j.actbio.2018.12.042
Weng, J., Chen, B., Xie, M., Wan, X., Wang, P., Zhou, X., et al. (2021). Rabbit Thyroid Extracellular Matrix as a 3D Bioscaffold for Thyroid Bioengineering: a Preliminary In Vitro Study. Biomed. Eng. Online 20 (1), 18. doi:10.1186/s12938-021-00856-w
Willemse, J., Verstegen, M. M. A., Vermeulen, A., Schurink, I. J., Roest, H. P., van der Laan, L. J. W., et al. (2020). Fast, Robust and Effective Decellularization of Whole Human Livers Using Mild Detergents and Pressure Controlled Perfusion. Mater. Sci. Eng. C 108, 110200. doi:10.1016/j.msec.2019.110200
Wu, G., Wu, D., Lo, J., Wang, Y., Wu, J., Lu, S., et al. (2020). A Bioartificial Liver Support System Integrated with a DLM/GelMA-based Bioengineered Whole Liver for Prevention of Hepatic Encephalopathy via Enhanced Ammonia Reduction. Biomater. Sci. 8 (10), 2814–2824. doi:10.1039/c9bm01879d
Wu, Q., Li, Y., Yang, Z., Li, L., Yang, J., Zhu, X., et al. (2022). Ectopic Expansion and Vascularization of Engineered Hepatic Tissue Based on Heparinized Acellular Liver Matrix and Mesenchymal Stromal Cell Spheroids. Acta Biomater. 137, 79–91. doi:10.1016/j.actbio.2021.10.017
Xiang, J.-X., Zheng, X.-L., Gao, R., Wu, W.-Q., Zhu, X.-L., Li, J.-H., et al. (2015). Liver Regeneration Using Decellularized Splenic Scaffold: a Novel Approach in Tissue Engineering. Hepatobiliary Pancreat. Dis. Int. 14 (5), 502–508. doi:10.1016/s1499-3872(15)60423-4
Xiang, J., Zheng, X., Liu, P., Yang, L., Dong, D., Wu, W., et al. (2016). Decellularized Spleen Matrix for Reengineering Functional Hepatic-like Tissue Based on Bone Marrow Mesenchymal Stem Cells. Organogenesis 12 (3), 128–142. doi:10.1080/15476278.2016.1185584
Yagi, S., Singhal, A., Jung, D.-H., and Hashimoto, K. (2020). Living-donor Liver Transplantation: Right versus Left. Int. J. Surg. 82, 128–133. doi:10.1016/j.ijsu.2020.06.022
Yang, W., Chen, Q., Xia, R., Zhang, Y., Shuai, L., Lai, J., et al. (2018). A Novel Bioscaffold with Naturally-Occurring Extracellular Matrix Promotes Hepatocyte Survival and Vessel Patency in Mouse Models of Heterologous Transplantation. Biomaterials 177, 52–66. doi:10.1016/j.biomaterials.2018.05.026
You, J., Park, S.-A., Shin, D.-S., Patel, D., Raghunathan, V. K., Kim, M., et al. (2013). Characterizing the Effects of Heparin Gel Stiffness on Function of Primary Hepatocytes. Tissue Eng. A 19 (23-24), 2655–2663. doi:10.1089/ten.TEA.2012.0681
Yusof, F., Sha'ban, M., and Azhim, A. (2019). Development of Decellularized Meniscus Using Closed Sonication Treatment System: Potential Scaffolds for Orthopedics Tissue Engineering Applications. Ijn Vol. 14, 5491–5502. doi:10.2147/ijn.S207270
Zhang, H., Siegel, C. T., Li, J., Lai, J., Shuai, L., Lai, X., et al. (2018). Functional Liver Tissue Engineering by an Adult Mouse Liver-Derived Neuro-Glia Antigen 2-expressing Stem/progenitor Population. J. Tissue Eng. Regen. Med. 12 (1), e190–e202. doi:10.1002/term.2311
Zhao, C., Li, Y., Peng, G., Lei, X., Zhang, G., and Gao, Y. (2020). Decellularized Liver Matrix-Modified Chitosan Fibrous Scaffold as a Substrate for C3A Hepatocyte Culture. J. Biomater. Sci. Polym. Edition 31 (8), 1041–1056. doi:10.1080/09205063.2020.1738690
Keywords: liver engineering, scaffold, decellularization, recellularization, implantation
Citation: Dai Q, Jiang W, Huang F, Song F, Zhang J and Zhao H (2022) Recent Advances in Liver Engineering With Decellularized Scaffold. Front. Bioeng. Biotechnol. 10:831477. doi: 10.3389/fbioe.2022.831477
Received: 08 December 2021; Accepted: 24 January 2022;
Published: 10 February 2022.
Edited by:
V. Prasad Shastri, University of Freiburg, GermanyReviewed by:
Chiara Magliaro, University of Pisa, ItalyElizabeth Stahl, University of California, Berkeley, United States
Copyright © 2022 Dai, Jiang, Huang, Song, Zhang and Zhao. This is an open-access article distributed under the terms of the Creative Commons Attribution License (CC BY). The use, distribution or reproduction in other forums is permitted, provided the original author(s) and the copyright owner(s) are credited and that the original publication in this journal is cited, in accordance with accepted academic practice. No use, distribution or reproduction is permitted which does not comply with these terms.
*Correspondence: Jiqian Zhang, amlxaWFuemhAbWFpbC51c3RjLmVkdS5jbg==; Hongchuan Zhao, emhjMDExN0BzaW5hLmNvbQ==
†These authors have contributed equally to this work