Strategies and Considerations for Improving Recombinant Antibody Production and Quality in Chinese Hamster Ovary Cells
- 1Institutes of Health Central Plains, Xinxiang Medical University, Xinxiang, China
- 2Department of Biochemistry and Molecular Biology, Xinxiang Medical University, Xinxiang, China
- 3Henan International Joint Laboratory of Recombinant Pharmaceutical Protein Expression System, Xinxiang Medical University, Xinxiang, China
Recombinant antibodies are rapidly developing therapeutic agents; approximately 40 novel antibody molecules enter clinical trials each year, most of which are produced from Chinese hamster ovary (CHO) cells. However, one of the major bottlenecks restricting the development of antibody drugs is how to perform high-level expression and production of recombinant antibodies. The high-efficiency expression and quality of recombinant antibodies in CHO cells is determined by multiple factors. This review provides a comprehensive overview of several state-of-the-art approaches, such as optimization of gene sequence of antibody, construction and optimization of high-efficiency expression vector, using antibody expression system, transformation of host cell lines, and glycosylation modification. Finally, the authors discuss the potential of large-scale production of recombinant antibodies and development of culture processes for biopharmaceutical manufacturing in the future.
Introduction
In recent years, recombinant antibody drugs are emerging with the rapid development of modern molecular biology technology as well as in-depth exploration on the three-dimensional structure and mechanism of action of antibody molecules. Recombinant antibody drugs undergo the stages of mouse monoclonal antibody, human-mouse chimeric antibody, humanized antibody, and fully human antibody (Figure 1), which have been applied in many fields, such as anti-tumor, anti-autoimmune diseases, and biosensor (DeLuca et al., 2021; Galvani et al., 2021; Liao et al., 2021; Rudenko et al., 2021; Rybchenko et al., 2021; Yang G et al., 2021). Improving the affinity of antibodies and reducing their immunogenicity are two basic principles of genetic engineering of antibody drugs. The development of antibody drugs is relatively rapid. According to industry statistics, the global annual sales of antibody drugs in 1997 were only $300 million, more than $60 billion in 2012, exceeded $100 billion for the first time in 2017, and reached $123.2 billion in 2018. At present, antibody drug development has become one of the fastest growing, most profitable, and most feasible biopharmaceutical fields in the pharmaceutical industry; in particular, monoclonal antibodies have become one of the most important therapeutic recombinant antibodies in the global pharmaceutical market (Masuda et al., 2021; Savizi et al., 2021). Up to now, more than 300 biological drugs have been approved by the U.S. Food and Drug Administration (FDA), among which monoclonal antibodies are developing rapidly (Tihanyi and Nyitray, 2020). The production of recombinant antibody drugs is mostly achieved by constructing expression vectors in vitro through genetic engineering technology. Given that recombinant antibodies often need to undergo a series of post-translational modifications (such as glycosylation modification), folding, and correct cleavage, antibody drugs with biological activity and low immunogenicity can be produced, therefore, mammalian cells have become the dominant system for the production of recombinant antibodies, especially for full-length monoclonal antibodies (Hussain et al., 2021; Kim et al., 2021; Ha et al., 2022).
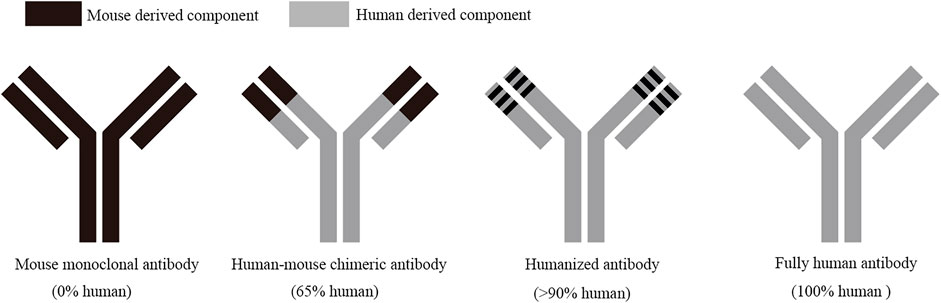
FIGURE 1. Development process of recombinant antibody drugs. Mouse monoclonal antibodies are all mouse-derived components, with large side effects and high immunogenicity; human-mouse chimeric antibodies are 65% human-derived components, with lower side effects than mouse monoclonal antibody; human-derived components in humanized antibodies are over 90%, with mild side effects and very low immunogenicity; fully human antibodies are all human-derived components, which can obviously remove the immunogenicity and side effects, and with good therapeutic effect.
Compared with Escherichia coli and other expression systems, the molecular structure and glycosylation type of recombinant antibodies produced by mammalian cell expression systems are similar to natural antibodies, and mammalian cells can be cultured in suspension or serum-free medium on a large scale (Kunert and Reinhart, 2016). Chinese hamster ovary (CHO) cells are the preferred system for the production of recombinant antibodies in mammalian cell expression systems (Ritacco et al., 2018; Gupta et al., 2021). In 1986, the first recombinant therapeutic protein, tissue plasminogen activator (tPA), was approved for marketing (Kaufman et al., 1985), its expression titer was less than 50 mg/L. After 30 years of rapidly development and technological breakthroughs, progress in therapeutic antibody field has been accelerated, the antibody expression titers have increased more than 100–200 times, even the yield of therapeutic antibody has exceeded 10 g/L (Li et al., 2010; Weng et al., 2020). The approved therapeutic monoclonal antibodies produced in CHO cells until 2020 are listed in Table 1. However, the low expression level of the CHO cells system and high investments during large-scale cell culture lead to high costs for producing recombinant antibody drugs (Donaldson et al., 2021; Torres and Dickson, 2021). Therefore, to improve the productivity and quality of recombinant antibodies, researchers need to optimize and design the gene sequences of antibody and expression vectors, to optimize antibody expression system and transform the host cell lines of antibodies, and to control glycosylation modification, for upgrading key parameters during industrial production and promoting the healthy development of recombinant antibody drugs and even the biomedical industry. A workflow for optimizing recombinant antibody production and quality in CHO cells is listed in Figure 2.
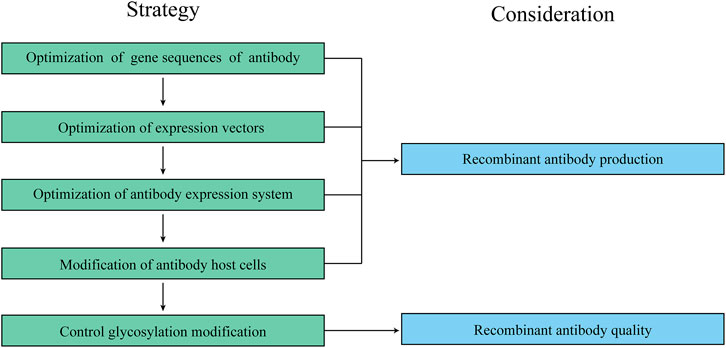
FIGURE 2. A workflow for optimizing recombinant antibody production and quality in CHO cells. Too many factors can impact recombinant antibody production and quality in CHO cells, therefore, it is important to follow a specific workflow when dealing with recombinant antibody production and quality.
Optimization of Gene Sequences of Antibody
Control the Proportion of Light and Heavy Chains
Most IgG molecules have a symmetrical structure consisting of four polypeptide chains including two heavy chains (HC) and two light chains (LC) (Figure 3). In the endoplasmic reticulum, the antibody binding protein (BiP) briefly binds to the heavy-chain polypeptide of the antibody, and the light chain is secreted out of the cell as polymerized dimers. Before assembling into a tetramer, BiP maintains a binding state with the heavy-chain polypeptide. Once the light-chain polypeptide is lacking, the heavy chain will not be secreted outside the cell, causing the proteasome in the cell to be unable to degrade the heavy-chain polypeptides secreted to extracellularly; thus, the unit productivity of antibody is significantly reduced (Vanhove et al., 2001; Ha et al., 2019). Therefore, excess light-chain polypeptides should be ensured to improve the expression of antibodies. In general, in the traditional process of antibody preparation, researchers often construct light- and heavy-chain genes of antibodies into two vectors to further express the antibody molecules. The disadvantage of this method is that the ratio of light and heavy chains is not controllable. In addition, the ratio of light and heavy chains affects the quality of monoclonal antibodies, such as the formation of aggregates and glycosylation modification. Chung et al. confirmed that excessive heavy-chain polypeptides may be the main cause of polymerization (Chung et al., 2018). In transient expression, the ratio of polypeptides can be controlled by adjusting the relative amount of each vector. However, this method is difficult to operate because the integration of plasmids is often random, and the number of gene copies and integration sites cannot be controlled artificially (Schlatter et al., 2005). Therefore, the industrial production of antibody drugs requires stable transfection of cell lines and proper control of the ratio of light and heavy chains, which are particularly important to achieve high expression, low polymerization, and consistent N-glycosylation profile of antibodies.
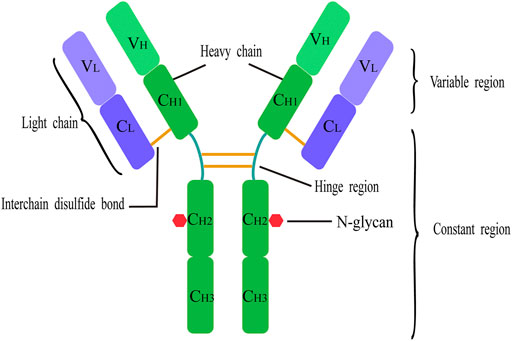
FIGURE 3. Basic structure of antibody. Each IgG antibody molecule consists of four polypeptide chains (two identical light chains and two identical heavy chains joined by disulfide bonds) and has two antigen-binding sites. Each light chain and each heavy chain consists of a variable region and a constant region. Each heavy chain consists of a variable domain (VH) and three or four constant domains (CH); each light chain consists of a variable domain (VL) and a single constant domain (CL). Human IgG structure with glycans attached at Asn297 N-glycosylation site in the CH2.
Change of Gene Arrangement
The heavy- and light-chain genes of antibodies use different vectors and then enter the host cells by co-transfection. This method has very low requirements on the vector, but the transfection efficiency is high, which is a common strategy for recombinant antibody expression (Carrara et al., 2021). However, the main defect of this strategy lies in the random integration of light- and heavy-chain genes into chromosomes, and the insertion positions and numbers are different, resulting in uncontrollable expression levels of light and heavy chains (Ahmadi et al., 2016). The second arrangement is to construct an independent expression unit of light and heavy chains on the same vector; the light and heavy chain genes use separate promoters and polyadenylic acid (Poly A) tail sequences of different lengths. This strategy can theoretically achieve equal mole expression levels of light- and heavy-chain genes. However, such adjacent reading frames may cause transcriptional interference, resulting in the imbalanced transcription of light and heavy chain and ultimately inhibiting the expression of recombinant antibodies (Davies et al., 2011). Based on the advantages and disadvantages of the two gene arrangements, researchers adopted a third arrangement, in which an internal ribosome enter site (IRES) or 2A peptide in the same open reading frame to connect light-chain genes, namely, triscistronic expression vector. This strategy can effectively avoid the occurrence of transcription interference and control the proportion of light and heavy chain expression. When expressing monoclonal antibodies using IRES-mediated tricistronic vector, the IRES connects heavy-chain genes, light-chain genes, and resistance genes for expression on the same vector. This strategy can maintain over 70% productivity of positive clones and 2-fold increase in the yield of recombinant antibodies (Li et al., 2007; Yeo et al., 2018). Ho et al. designed four IRES-mediated tricistronic vectors to control the ratio of light and heavy chains, depending on the different positions of the light and heavy chains and selection marker genes on the vector; they also compared the expression level and quality of antibodies, including aggregate formation, N-glycosylation, and conformational stability. The results show that excess light chain is essential for high expression of antibody and also reduces the occurrence of polymerization. In addition, the main cause of polymerization is that the excessive heavy chains cannot effectively fold with the limited light chains and can change the N-glycosylation and reduce the conformational stability (Ho et al., 2013b). Controlling the ratio of light and heavy chains is crucial for the expression and quality of monoclonal antibodies under stable transfection conditions (Ho et al., 2012). In particular, excessive light chains cannot only achieve high antibody expression but also reduce polymerization and maintain low fragmentation levels, which are very beneficial for obtaining high-yield antibodies (Ho et al., 2013b). However, the fragment length of IRES itself is relatively large, which occupies more space, and the expression of IRES upstream and downstream antibody molecules is seriously imbalanced (Bayat et al., 2018). In addition, the activity of IRES is not easy to adjust, which will affect the biological activities of other expressed proteins. In recent years, a prominent approach is based on self-cleavage 2A peptide-mediated multi-gene construction method (Chng et al., 2015; Van der Weken et al., 2019). This strategy effectively avoids the disadvantage of low expression of downstream genes caused by the simultaneous expression of multiple genes and is commonly used in multi-gene expression (Luke and Ryan, 2018; Li et al., 2020). Ho et al. compared the effects of F2A and IRES on the production of a monoclonal antibody in CHO-DG44 cells. The expression level of the monoclonal antibody produced by F2A-mediated tricistronic vector was significantly higher than that of IRES-mediated vector under transient or stable transfection conditions; the expression level of the recombinant antibody was affected by the position of light- and heavy-chain cistrons (Ho et al., 2013a).
Codon Optimization
Antibody molecules usually have the molecular structure of tetramer glycoprotein. Light- and heavy-chain genes in antibody molecules need to have coordinated expression to biosynthesize tetramer IgG. However, when expressing recombinant antibodies, the special secondary structure in the gene sequence and other factors often lead to low expression of antibody, which can be remedied by codon optimization strategy. Codon optimization involves gene synthesis, gene transcription, mRNA translation, etc., with the ultimate goal of efficiently expressing recombinant antibodies (Ayyar et al., 2017; Mauro and Chappell, 2018; You et al., 2018). Before expressing a gene, the rare codon in the gene should be searched first. If there are too many rare codons, then the translation rate of antibody will be affected. The expression of the target gene in transgenic hosts can be increased by selecting the codon preferred by the receptor without changing the amino acid sequence (Mauro, 2018). When the codon of the variable region of an antibody is replaced with the preferred codon of the natural human antibody gene, the antibody expression levels in mammalian cells are significantly increased by two- to 3-fold (Carton et al., 2007).
Signal Peptide Optimization
Signal peptide is a key factor for the secretion of recombinant antibodies, and the high-efficiency expression of these antibodies is closely related to the signal peptide (Haryadi et al., 2015; Wang et al., 2016). When expressing recombinant antibodies, in addition to the use of their own signal peptides, the following strategies are often applied: ① replace the signal peptide sequence of the efficiently expressed secreted proteins (Attallah et al., 2017); ② modifying the primary structure of the signal peptide sequence in the original antibody; ③ replace the protein signal peptide sequence in some viruses; however, viral vectors are generally not recommended when considering the safety of the expressed recombinant antibodies; and ④ select the preferred host codon. When selecting a suitable signal peptide, the preferred codons of the expression host should be fully considered, and the signal peptide should be further optimized. Ramezani et al. showed a 2-fold increase of the pertuzumab production in CHO cells by optimizing codons and selecting appropriate signal peptide strategies (Ramezani et al., 2017).
Construction and Optimization of Expression Vectors
The construction of high-efficiency expression vectors is considered an important strategy to improve the expression level of recombinant antibodies. The expression level of the target genes in mammalian cells is mainly affected by the state of chromosome region integrated by the gene of interest, the copy number of the target gene, and its transcription and translation efficiency (Hung et al., 2010; Gupta et al., 2019; Carver et al., 2020; Hoseinpoor et al., 2020). Therefore, vector construction strategy should consider optimizing the integration site and position effect on the chromosome and improving the transcription and translation efficiency to effectively improve the expression level of recombinant antibodies.
Optimization of Integration Site and Position Effect
The integration site status of target gene on the mammalian cell chromosome plays a decisive role in its expression level and its stability in host cells. Only clones formed by cells whose integration sites are in transcription active region of chromosomes can express the target gene at a high level. However, transgene silencing often occurs due to the random integration of the target gene after transfer into cells (Hilliard and Lee, 2021). During the expansion of cell culture, the promoter methylation will lead to the attenuation of expression. Therefore, selecting an appropriate promoter and optimizing the combination of promoters and different regulatory elements can improve the expression of recombinant antibodies and increase the stability of expression. Some chromatin-modifying elements can prevent transgene silencing, including matrix attachment regions (MAR) (Buceta et al., 2011; Mohammadian et al., 2019; Zhang et al., 2020), locus control regions (LCR) (Sharma et al., 2019; Morgan et al., 2020), ubiquitous chromatin opening elements (UCOEs) (Pfaff et al., 2013; Harraghy et al., 2015; Nematpour et al., 2017; Rocha-Pizaña et al., 2017), stabilizing anti-repressor elements (STAR) (Kwaks et al., 2003; Otte et al., 2007; Van Blokland et al., 2007), and insulators (Benabdellah et al., 2014; Chetverina et al., 2014; Naderi et al., 2018; Pérez-González and Caro, 2019). Moreover, artificial chromosome expression (ACE) and targeted integration technology, including Flp-In and recombinase-mediated cassette exchange system (RMCE), can overcome the shortcomings of random integration (Kennard et al., 2009; Soler et al., 2018; Reinhart et al., 2019; Ng et al., 2021). The introduction of these functional elements into the vector construction can greatly increase the proportion of high-expression clones and shorten the construction cycle of engineered host cells (Table 2).
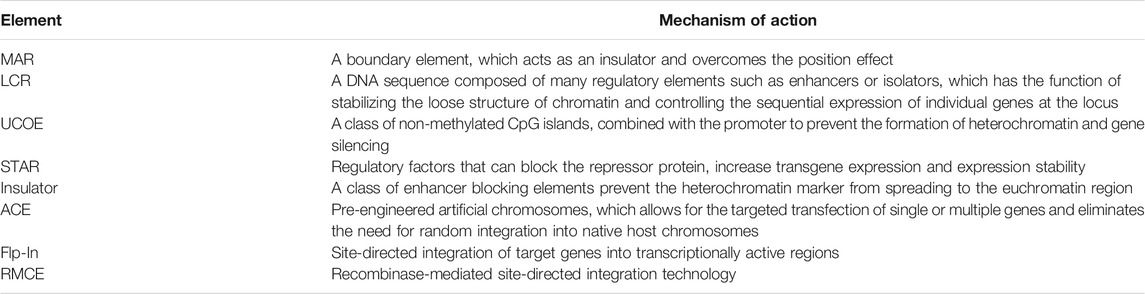
TABLE 2. Optimization strategies of integration site and position effect for improving recombinant antibody expression.
Improvement of Transcription and Translation Efficiency
Transcription is the initial step of gene expression, among which promoters, enhancers, and transcription termination signals have very important impacts on transcription efficiency and mRNA stability. CMV promoters are considered to be one of the strongest viral promoters, and hEF-1α promoters have the stronger transcription initiation efficiency and are more suitable for large-scale production of recombinant antibodies (Ebadat et al., 2017). In recent years, the construction of synthetic or heterozygous promoters will become an effective strategy to improve the transcription efficiency (Patel et al., 2021).
Enhancers can improve the transcription efficiency and can function over long distances. Constructing heterozygous enhancers is a good strategy to improve transcription efficiency and obtain high transcriptional activity. Xu et al. used the CMV promoter and CA hybrid promoter to insert the SV40 enhancers downstream of SV40 Poly A; compared with the CMV promoter, cells driven by the CA hybrid promoter can increase the production of target proteins by two times (Xu et al., 2001).
Introns can increase the expression of foreign genes. Xu et al. compared the effects of five different introns on transgene expression in CHO cells. Under transient and stable transfection conditions, the SV40 intron can obtain the highest transgene expression level among five introns, which can also obtain high-level of recombinant protein production in CHO cells (Xu et al., 2018).
The regulation of translation level and processing efficiency of translation products will also significantly affect the expression of target gene. Eisenhut et al. developed the 5′-untranslated region (UTR) RNA-structures to impact translation efficiency, further systematically tune protein expression levels in mammalian cells and eventually help to optimize recombinant protein expression (Eisenhut et al., 2020). Vivirius et al. found a universal translation enhancer element in the 5′-end non-translation area of Hsp70 mRNA, which can enhance the translation efficiency of the cap-dependent structure (Vivinus et al., 2001).
Optimzation of Antibody Expression System
Gene Amplification Screening System
At present, the two common gene amplification screening systems are dihydrofolate reductase (DHFR) and glutamine synthetase (GS) (Budge et al., 2021; Huhn et al., 2021). The CHO-DHFR amplification system mainly uses the CHO cell line of DHFR defect to obtain high yields of recombinant antibodies in the presence of methotrexate (MTX). Akbarzade-sharbaf et al. used DHFR system to express a therapeutic antibody (Trastuzumab); after seven rounds of MTX, the total antibody valence can be as high as 50–60 mg/L/day (Akbarzadeh-Sharbaf et al., 2013). As another mature and widely used system, the GS amplification system is an explicit gene amplification selection marker, which usually requires pressure and stable selection of two rounds of methionine sulphoximine (MSX) to obtain high-expression cell lines and reduce the screening time. However, the two systems have some defects, such as the decline in the antibody production after long-term screening; therefore, these systems need to be further optimized. The strategy of using low activity of GS as the screening marker could obtain high-yield clones after MSX removal, further efficiently increase the antibody production (Lin et al., 2019).
Screening Marker Weakening
Two strategies can be employed to weaken the screening marker. One is to mutate the screening marker and reduce its activity. Neomycin-phosphotransferase (NPT) is one of the commonly used screening markers in eukaryotic expression systems. The second strategy is to reduce the expression of the screening marker. Noguchi et al. started the transcription of DHFR through the late promoter of weak promoter SV40 and used the method of IR/MAR-dhfr fusion to easily separate the cell line of high-expression recombinant protein, which further improved the production of the recombinant proteins (Noguchi et al., 2012).
Modification of Antibody Host Cells
Many mammalian cells can produce recombinant antibodies, including CHO, NS0, and SP2/0 cells, which can produce non-human glycosylated modification. Owing to the difference between the growth and metabolic characteristics of animal cells, the expression level and modification ability of recombinant antibody are also different (Dhara et al., 2018). Therefore, we can further transform the host cells, improve the expression level by engineering cell line, and meet the production capacity and quality requirement of antibody drugs as much as possible (Lu et al., 2018; Liu et al., 2021; Zhou et al., 2021).
Glycosylation Engineering
The enzymes responsible for glycosylation can be modified in non-humanized cells, so the expressed recombinant antibody is more similar to the natural humanized protein in terms of glycosylation level and type, thereby improving the biological effect of recombinant antibody. Glycosylation mainly includes genetic and non-genetic modification strategies. The glycosylation of protein can affect the pharmacological activity and pharmacodynamics of the antibody, so researchers can optimize the recombinant antibodies through genetic engineering to achieve the glycosylation modification of cell lines (Yang Y et al., 2021). For example, the therapeutic activity of recombinant IgG3 antibodies was significantly improved after transfecting murine 2, 6-sialyltransferase into CHO cells (Jassal et al., 2001).
Anti-Apoptosis
Anti-apoptosis is a hot spot in the current host cell transformation strategy, and the overexpression of anti-apoptotic genes is one of the common strategies. Effective anti-apoptotic genes include Bcl-2, Bcl-xL, and so on. Lee et al. achieved the overexpression of Bcl-2 and Beclin-1 in CHO-DG44 cells; the results showed that the time of cell culture became longer, the cell survival rate was significantly improved, and the occurrence of apoptosis was suppressed (Lee et al., 2013). Kim et al. overexpressed Bcl-xL in recombinant CHO cells, which can improve cell survival rate, prolong culture time, and inhibit apoptosis and autophagy by inhibiting the activation of caspase-3and caspase-7 (Kim et al., 2009).
Cellular Metabolic Engineering
In mammalian cell culture, the metabolic engineering strategy changes the cell metabolism pathway, which can effectively promote cell growth and product synthesis, discover more novel metabolite additives, and reduce the accumulation of metabolic inhibitors (Toussaint et al., 2016; Gupta et al., 2017; Fouladiha et al., 2020; Yao et al., 2021). In cells with low lactate/glucose, the expression levels of lactate dehydrogenase and the accumulation of lactate are reduced, resulting in an increase in the synthetic products. Zhou et al. used siRNA technology to reduce the expression levels of lactate dehydrogenase A (LDHa) and pyruvate dehydrogenase kinase (PDHK) genes, reducing the lactate levels by 90% and increasing the production of therapeutic monoclonal antibodies (Zhou et al., 2011). In addition, the production of recombinant antibodies can be multiplied by several times by effectively blocking engineered cells in the G1 phase.
Cellular Cycle Regulation Engineering
In the large-scale cell culture process, with the continuous exploration of the regulatory mechanism of cell cycle, researchers have applied cell cycle regulation genes to cell proliferation control. Fussenegger et al. found that p21, p27, and p53 are cell cycle Gl/S suppressor proteins; after CHO cells express these proteins, they can prevent cells from entering the S phase, keep the growth in a static state, and increase the production of secreted alkaline phosphatase (SEAP) to 10–15 times (Fussenegger et al., 1998).
Control Glycosylation Modification
Recombinant antibodies are biological macromolecular drugs, and the normal performance of their biological functions is inseparable from the complex post-translational modification process. As the most important type of post-translational modification of recombinant antibodies, glycosylation modification has certain effects on the biological activity of antibody, immunogenicity, in vivo metabolism, antibody-dependent cytotoxicity, and complement-dependent cytotoxicity. Therefore, glycosylation modification of antibody molecules has been widely used in the development of novel antibody drugs.
Type of Antibody Glycosylation Modification
According to different connection modes, antibody glycosylation is usually divided into N-linked glycosylation and O-linked glycosylation. The Fc fragments of two heavy chains in the monoclonal antibody molecule contain an N-glycosylation modification site at the 297th aspartic acid. The N-glycosylation modification of monoclonal antibody drugs usually presents biantennary sugar chains, and sometimes fucosylation and sialylation may occur (Szabo et al., 2022). In addition, some monoclonal antibody drugs undergo O-glycosylation modification. For example, a humanized monoclonal antibody drug produced by CHO cells has O-glycosylation modified by a single glucose molecule (Tanaka et al., 2013). At present, most of the antibody drugs on the market are produced by CHO cells. CHO cells can produce antibodies that are close to the glycotype of human serum antibodies; however, the glycosylation of antibodies produced by most engineered cell lines is different from that of human serum antibodies. For example, antibodies produced from mouse-derived animal cells have a high proportion of fucose modifications and a low proportion of galactosylation modifications (An, 2009). In contrast to human cells, CHO cells lack the expression of α-2,6-sialyltransferase, while only express α-2,3-sialyltransferase (Jenkins et al., 1996; Lin et al., 2015; Chung et al., 2020). Consequently, CHO cells inherently cannot produce glycoproteins with similar terminal sialic acid content as compared to human cells (Yin et al., 2017). Furthermore, CHO cells lack N-acetylglucosamine transferase (GnT-III) expressed by human cells, resulting in the differences in the modification of N-acetylglucosamine glycosylation from human cells (Butler, 2005). These findings indicate that different cell types produce different types of antibody glycosylation (Attallah et al., 2020; Dyukova et al., 2021; Zhao et al., 2021). In summary, the type of glycosylation modification of monoclonal antibody drugs is closely related to the production system, selected cell line, and incubation process.
Control Strategy of Antibody Glycosylation Modification
Although the mass of sugar chains only accounts for 2% of the total molecular weight of antibody, the sugar chains on the Fc and Fab fragments play a very important role in the affinity, structural maintenance, metabolism, and immunogenicity of the antibody molecule (Krapp et al., 2003; Chung et al., 2008; Alessandri et al., 2012). The physiological activity of therapeutic antibodies is mainly mediated by two mechanisms: one is mediated by the affinity between the variable region of the antibody and antigen, causing the neutralization or apoptosis of the target antigen, which is mainly by means of the Fab fragment of antibody to recognize and bind antigenic substances; the other is the immune effect mediated by the Fc fragment of antibody, including antibody-dependent cell-mediated cytotoxicity (ADCC) and complement-dependent cytotoxicity (CDC) (Salinas-Jazmín et al., 2022). Metabolic analysis and mathematical model can be used to analyze the production process to control the type and degree of glycosylation modification of recombinant antibodies to reduce the immunogenicity of antibody drugs and optimize their effector function (Sha et al., 2016). The types of glycosylation modification of recombinant antibodies are shown in Figure 4.
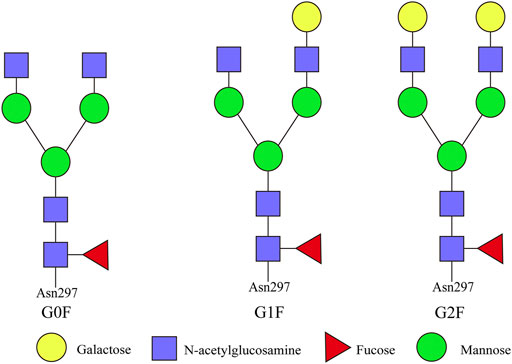
FIGURE 4. Types of glycosylation modification of recombinant antibodies. The representative N-glycan structures identified on antibody Fc fragment are G0F, G1F, and G2F. G0F: asialo, agalactose, biantennary complex, core substituted with fucose; G1F: asialo, mono-galactosylated, biantennary complex, core substituted with fucose; G2F: asialo, galactosylated, biantennary complex, core substituted with fucose.
Sialylation modification: Sialylatedglycans are the key components of glycoproteins. According to relevant studies, monoclonal antibody sialylation can inhibit inflammatory response and reduce cell toxicity by means of different receptors in the Fc fragment (Scallon et al., 2007). The anti-inflammatory activity of the over-sialylated monoclonal antibody obtained by affinity chromatography purification will be significantly enhanced. Moreover, the higher degree of monoclonal antibody sialylation is closely related to a decrease in ADCC activity (Zhang et al., 2019; Nguyen et al., 2020).
Core fucosylation modification: Related studies have shown that core fucose is an important glycosylation structure that affects the ADCC activity of recombinant antibodies (Matsumiya et al., 2007). Most recombinant IgG produced by CHO cells have core fucose in their Fc sugar chains (Zimmermann et al., 2021). To improve the binding activity of the low-affinity receptor IIIa (FcγR3a) of the antibody to the Fc fragment as well as ADCC activity, researchers have adopted a variety of strategies to reduce the level of fucosylation of IgG, including the use of α-1,6-fucose cell lines in which α-1,6-fucosetransferase gene is knocked out (Davies et al., 2001; Wang et al., 2018).
Galactosylation modification: Approximately 95% of recombinant IgG produced by CHO cells contains galactose as the terminal sugar. Terminal galactosylation plays an important role in the conformation of the Fc fragment, and the core fucosylation modification alone has slight effect on the conformation of the Fc fragment (Kotidis et al., 2019). The existing results indicate that galactosylation at the end of the Fc fragment will seriously affect the CDC activity of IgG, and the reduction of its glycosylation level will also weaken the CDC activity (Wei et al., 2021).
Mannosylation modification: The content of a high-mannose structure should be minimized as much as possible during the production of recombinant antibodies due to the high immunogenicity of high-mannose structures. In addition, the content of mannose in the Fc fragment of the antibody varies greatly between different cultured cells and different batches (Brantley et al., 2021). Recombinant antibodies have two clearance pathways in organisms; one is the asialoglycoprotein receptor in the liver that binds and mediates the clearance, and the other is to bind to mannose receptors on the surface of macrophages in the liver. The high-mannose structure antibody molecules in the Fc fragment can be quickly eliminated from the plasma, further reducing the efficacy of recombinant antibodies (Liu, 2015).
Expression of GnT-III: Studies have shown that the bisected glycosylated epidermal growth factor receptor (EGFR) monoclonal antibody is prepared by introducing the GnT-III gene and highly expresses bisecting acetylglucosamine residues; this strategy can increase the ADCC activity by 3 times and increase the anti-proliferative activity by 1.36 times, and almost no α-Gal was detected. The bisecting EGFR monoclonal antibody prepared by glycosylation engineering contains only a small amount of α-Gal, which greatly improves the biological activity in vitro. At present, this study has not been further verified in vivo (Jenkins and Curling, 1994; Yi et al., 2014).
Conclusion and Future Perspectives
In recent years, with the development and application of proteomics technology, the development of large-scale culture of animal cells for antibody drug production has devolved from the simple optimization of some process parameters to the recent omics research, e.g., transcriptomics, proteomics, metabolomics, glycomics, and fluxomics. The complex metabolic network of production cells and production mechanism of recombinant antibodies have gradually become clear. Raab et al. developed a cell line through genetic engineering by a novel bottom-up microRNA (miRNA) screening approach for optimizing the production and secretion of therapeutic antibodies (Raab et al., 2022). At present, the expression level and quality control of recombinant antibodies has always been one of the important bottlenecks restricting the development of antibody drugs.
The high-efficiency expression and quality of recombinant antibodies can be affected by multiple factors, which can be achieved by genetic engineering, including the optimization of antibody gene sequence, construction of efficient expression vector, optimization of antibody expression system, modification of antibody host cells, and glycosylation site modification (Table 3). The application of these optimization strategies can effectively shorten the time of antibody generation and improve the expression of target antibodies. However, different optimization strategies have advantages and disadvantages, and how to effectively integrate these optimization strategies to make it an efficient operation system needs further study. Under different process conditions, the differences in the yield of recombinant antibodies by the production cell lines can be analyzed from the levels of genomics, proteomics, and metabolomics, laying a solid foundation for large-scale cell culture processes. In the future, the large-scale production of recombinant antibodies and development of culture processes will develop rapidly in the direction of stabilizing production capacity and improving quality. Furthermore, big data and multi-omics technologies are also beneficial to provide new research directions, more and more process analytical technologies are applied to cell culture processes, which will provide more ideas for improving the efficiency of recombinant antibodies. Therefore, the focus shifts towards how to control the quality of recombinant antibody drugs scientifically and rationally, researchers should further combine the clinical evaluation and post-market safety monitoring, and continue to explore quality control.
Author Contributions
J-HZ: manuscript design, preparation and revision. L-LS, FL, and C-YD: proofreading and manuscript revision. J-JL: manuscript revision.
Funding
This work was supported by the grant from the National Natural Science Foundation of China (No. U1604193) and Open Program of International Joint Research Laboratory for Recombinant Pharmaceutical Protein Expression System of Henan (No. KFKTYB202205).
Conflict of Interest
The authors declare that the research was conducted in the absence of any commercial or financial relationships that could be construed as a potential conflict of interest.
Publisher’s Note
All claims expressed in this article are solely those of the authors and do not necessarily represent those of their affiliated organizations, or those of the publisher, the editors and the reviewers. Any product that may be evaluated in this article, or claim that may be made by its manufacturer, is not guaranteed or endorsed by the publisher.
References
Ahmadi, M., Mahboudi, F., Akbari Eidgahi, M. R., Nematpour, F., Ahmadi, S., Ebadat, S., et al. (2016). Evaluating the Efficiency of phiC31 Integrase-Mediated Monoclonal Antibody Expression in CHO Cells. Biotechnol. Prog. 32, 1570–1576. doi:10.1002/btpr.2362
Akbarzadeh-Sharbaf, S., Yakhchali, B., Minuchehr, Z., Shokrgozar, M. A., and Zeinali, S. (2013). Expression Enhancement in Trastuzumab Therapeutic Monoclonal Antibody Production Using Genomic Amplification with Methotrexate. Avicenna J. Med. Biotechnol. 5, 87–95.
Alessandri, L., Ouellette, D., Acquah, A., Rieser, M., Leblond, D., Saltarelli, M., et al. (2012). Increased Serum Clearance of Oligomannose Species Present on a Human IgG1 Molecule. MAbs 4, 509–520. doi:10.4161/mabs.20450
An, Z. Q. (2009). Therapeutic Monoclonal Antibodies: From Bench to Clinic. New Jersey: John Wiley & Sons, 73–75.
Attallah, C., Aguilar, M. F., Forno, G., Etcheverrigaray, M., Brigido, M. D. M., Maranhão, A. Q., et al. (2020). The Glycosylation of Anti-rhIFN-α2b Recombinant Antibodies Influences the Antigen-Neutralizing Activity. Biotechnol. Lett. 42, 1369–1381. doi:10.1007/s10529-020-02879-0
Attallah, C., Etcheverrigaray, M., Kratje, R., and Oggero, M. (2017). A Highly Efficient Modified Human Serum Albumin Signal Peptide to Secrete Proteins in Cells Derived from Different Mammalian Species. Protein Expr. Purif. 132, 27–33. doi:10.1016/j.pep.2017.01.003
Ayyar, B. V., Arora, S., and Ravi, S. S. (2017). Optimizing Antibody Expression: The Nuts and Bolts. Methods 116, 51–62. doi:10.1016/j.ymeth.2017.01.009
Bayat, H., Hoseinzadeh, S., Pourmaleki, E., Ahani, R., and Rahimpour, A. (2018). Evaluation of Different Vector Design Strategies for the Expression of Recombinant Monoclonal Antibody in CHO Cells. Prep. Biochem. Biotechnol. 48, 160–164. doi:10.1080/10826068.2017.1421966
Benabdellah, K., Gutierrez-Guerrero, A., Cobo, M., Muñoz, P., and Martín, F. (2014). A Chimeric HS4-SAR Insulator (IS2) that Prevents Silencing and Enhances Expression of Lentiviral Vectors in Pluripotent Stem Cells. PLoS One 9, e84268. doi:10.1371/journal.pone.0084268
Brantley, T. J., Mitchelson, F. G., and Khattak, S. F. (2021). A Class of Low-Cost Alternatives to Kifunensine for Increasing High Mannose N-Linked Glycosylation for Monoclonal Antibody Production in Chinese Hamster Ovary Cells. Biotechnol. Prog. 37, e3076. doi:10.1002/btpr.3076
Buceta, M., Galbete, J. L., Kostic, C., Arsenijevic, Y., and Mermod, N. (2011). Use of Human MAR Elements to Improve Retroviral Vector Production. Gene Ther. 18, 7–13. doi:10.1038/gt.2010.115
Budge, J. D., Roobol, J., Singh, G., Mozzanino, T., Knight, T. J., Povey, J., et al. (2021). A Proline Metabolism Selection System and its Application to the Engineering of Lipid Biosynthesis in Chinese Hamster Ovary Cells. Metab. Eng. Commun. 13, e00179. doi:10.1016/j.mec.2021.e00179
Butler, M. (2005). Animal Cell Cultures: Recent Achievements and Perspectives in the Production of Biopharmaceuticals. Appl. Microbiol. Biotechnol. 68, 283–291. doi:10.1007/s00253-005-1980-8
Carrara, S. C., Fiebig, D., Bogen, J. P., Grzeschik, J., Hock, B., and Kolmar, H. (2021). Recombinant Antibody Production Using a Dual-Promoter Single Plasmid System. Antibodies 10, 18. doi:10.3390/antib10020018
Carton, J. M., Sauerwald, T., Hawley-Nelson, P., Morse, B., Peffer, N., Beck, H., et al. (2007). Codon Engineering for Improved Antibody Expression in Mammalian Cells. Protein Expr. Purif. 55, 279–286. doi:10.1016/j.pep.2007.05.017
Carver, J., Ng, D., Zhou, M., Ko, P., Zhan, D., Yim, M., et al. (2020). Maximizing Antibody Production in a Targeted Integration Host by Optimization of Subunit Gene Dosage and Position. Biotechnol. Prog. 36, e2967. doi:10.1002/btpr.2967
Chetverina, D., Aoki, T., Erokhin, M., Georgiev, P., and Schedl, P. (2014). Making Connections: Insulators Organize Eukaryotic Chromosomes into Independent Cis-Regulatory Networks. Bioessays 36, 163–172. doi:10.1002/bies.201300125
Chng, J., Wang, T., Nian, R., Lau, A., Hoi, K. M., Ho, S. C., et al. (2015). Cleavage Efficient 2A Peptides for High Level Monoclonal Antibody Expression in CHO Cells. MAbs 7, 403–412. doi:10.1080/19420862.2015.1008351
Chung, C. H., Mirakhur, B., Chan, E., Le, Q.-T., Berlin, J., Morse, M., et al. (2008). Cetuximab-Induced Anaphylaxis and IgE Specific for Galactose-α-1,3-Galactose. N. Engl. J. Med. 358, 1109–1117. doi:10.1056/nejmoa074943
Chung, C. Y., Wang, Q., Yang, S., Chough, S., Seo, Y., Cipollo, J. F., et al. (2020). The Impact of Sialylation Linkage‐type on the Pharmacokinetics of Recombinant Butyrylcholinesterases. Biotechnol. Bioeng. 117, 157–166. doi:10.1002/bit.27174
Chung, H. H., Buck, L., Daris, K., Welborn, B., Luo, Q., and Wypych, J. (2018). Investigation of the Free Heavy Chain Homodimers of a Monoclonal Antibody. Biotechnol. Prog. 34, 738–745. doi:10.1002/btpr.2611
Davies, J., Jiang, L., Pan, L.-Z., LaBarre, M. J., Anderson, D., and Reff, M. (2001). Expression of GnTIII in a Recombinant Anti-CD20 CHO Production Cell Line: Expression of Antibodies with Altered Glycoforms Leads to an Increase in ADCC through Higher Affinity for FC?RIII. Biotechnol. Bioeng. 74, 288–294. doi:10.1002/bit.1119
Davies, S. L., O'Callaghan, P. M., McLeod, J., Pybus, L. P., Sung, Y. H., Rance, J., et al. (2011). Impact of Gene Vector Design on the Control of Recombinant Monoclonal Antibody Production by Chinese Hamster Ovary Cells. Biotechnol. Prog. 27, 1689–1699. doi:10.1002/btpr.692
DeLuca, K. F., Mick, J. E., Ide, A. H., Lima, W. C., Sherman, L., Schaller, K. L., et al. (2021). Generation and Diversification of Recombinant Monoclonal Antibodies. Elife 10, e72093. doi:10.7554/eLife.72093
Dhara, V. G., Naik, H. M., Majewska, N. I., and Betenbaugh, M. J. (2018). Recombinant Antibody Production in CHO and NS0 Cells: Differences and Similarities. BioDrugs 32, 571–584. doi:10.1007/s40259-018-0319-9
Donaldson, J. S., Dale, M. P., and Rosser, S. J. (2021). Decoupling Growth and Protein Production in CHO Cells: A Targeted Approach. Front. Bioeng. Biotechnol. 9, 658325. doi:10.3389/fbioe.2021.658325
Dyukova, I., Ben Faleh, A., Warnke, S., Yalovenko, N., Yatsyna, V., Bansal, P., et al. (2021). A New Approach for Identifying Positional Isomers of Glycans Cleaved from Monoclonal Antibodies. Analyst 146, 4789–4795. doi:10.1039/d1an00780g
Ebadat, S., Ahmadi, S., Ahmadi, M., Nematpour, F., Barkhordari, F., Mahdian, R., et al. (2017). Evaluating the Efficiency of CHEF and CMV Promoter with IRES and Furin/2A Linker Sequences for Monoclonal Antibody Expression in CHO Cells. PLoS One 12, e0185967. doi:10.1371/journal.pone.0185967
Eisenhut, P., Mebrahtu, A., Moradi Barzadd, M., Thalén, N., Klanert, G., Weinguny, M., et al. (2020). Systematic Use of Synthetic 5′-UTR RNA Structures to Tune Protein Translation Improves Yield and Quality of Complex Proteins in Mammalian Cell Factories. Nucleic Acids Res. 48, e119. doi:10.1093/nar/gkaa847
Fouladiha, H., Marashi, S.-A., Torkashvand, F., Mahboudi, F., Lewis, N. E., and Vaziri, B. (2020). A Metabolic Network-Based Approach for Developing Feeding Strategies for CHO Cells to Increase Monoclonal Antibody Production. Bioproc. Biosyst. Eng. 43, 1381–1389. doi:10.1007/s00449-020-02332-6
Fussenegger, M., Schlatter, S., Dätwyler, D., Mazur, X., and Bailey, J. E. (1998). Controlled Proliferation by Multigene Metabolic Engineering Enhances the Productivity of Chinese Hamster Ovary Cells. Nat. Biotechnol. 16, 468–472. doi:10.1038/nbt0598-468
Galvani, N. C., Machado, A. S., Lage, D. P., Freitas, C. S., Vale, D. L., de Oliveira, D., et al. (2021). ChimLeish, a New Recombinant Chimeric Protein Evaluated as a Diagnostic and Prognostic Marker for Visceral Leishmaniasis and Human Immunodeficiency Virus Coinfection. Parasitol. Res. 120, 4037–4047. doi:10.1007/s00436-021-07342-1
Gupta, K., Modi, D., Jain, R., and Dandekar, P. (2021). A Stable CHO K1 Cell Line for Producing Recombinant Monoclonal Antibody against TNF-α. Mol. Biotechnol. 63, 828–839. doi:10.1007/s12033-021-00329-4
Gupta, K., Parasnis, M., Jain, R., and Dandekar, P. (2019). Vector-related Stratagems for Enhanced Monoclonal Antibody Production in Mammalian Cells. Biotechnol. Adv. 37, 107415. doi:10.1016/j.biotechadv.2019.107415
Gupta, S. K., Srivastava, S. K., Sharma, A., Nalage, V. H. H., Salvi, D., Kushwaha, H., et al. (2017). Metabolic Engineering of CHO Cells for the Development of a Robust Protein Production Platform. PLoS One 12, e0181455. doi:10.1371/journal.pone.0181455
Ha, T. K., Hansen, A. H., Kildegaard, H. F., and Lee, G. M. (2019). BiP Inducer X: An ER Stress Inhibitor for Enhancing Recombinant Antibody Production in CHO Cell Culture. Biotechnol. J. 14, e1900130. doi:10.1002/biot.201900130
Ha, T. K., Kim, D., Kim, C. L., Grav, L. M., and Lee, G. M. (2022). Factors Affecting the Quality of Therapeutic Proteins in Recombinant Chinese Hamster Ovary Cell Culture. Biotechnol. Adv. 54, 107831. doi:10.1016/j.biotechadv.2021.107831
Harraghy, N., Calabrese, D., Fisch, I., Girod, P.-A., LeFourn, V., Regamey, A., et al. (2015). Epigenetic Regulatory Elements: Recent Advances in Understanding Their Mode of Action and Use for Recombinant Protein Production in Mammalian Cells. Biotechnol. J. 10, 967–978. doi:10.1002/biot.201400649
Haryadi, R., Ho, S., Kok, Y. J., Pu, H. X., Zheng, L., Pereira, N. A., et al. (2015). Optimization of Heavy Chain and Light Chain Signal Peptides for High Level Expression of Therapeutic Antibodies in CHO Cells. PLoS One 10, e0116878. doi:10.1371/journal.pone.0116878
Hilliard, W., and Lee, K. H. (2021). Systematic Identification of Safe Harbor Regions in the CHO Genome through a Comprehensive Epigenome Analysis. Biotechnol. Bioeng. 118, 659–675. doi:10.1002/bit.27599
Ho, S. C. L., Bardor, M., Feng, H., Mariati, T., Tong, Z., Yap, M. G. S., et al. (2012). IRES-mediated Tricistronic Vectors for Enhancing Generation of High Monoclonal Antibody Expressing CHO Cell Lines. J. Biotechnol. 157, 130–139. doi:10.1016/j.jbiotec.2011.09.023
Ho, S. C. L., Bardor, M., Li, B., Lee, J. J., Song, Z., Tong, Y. W., et al. (2013a). Comparison of Internal Ribosome Entry Site (IRES) and Furin-2A (F2A) for Monoclonal Antibody Expression Level and Quality in CHO Cells. PLoS One 8, e63247. doi:10.1371/journal.pone.0063247
Ho, S. C. L., Koh, E. Y. C., van Beers, M., Mueller, M., Wan, C., Teo, G., et al. (2013b). Control of IgG LC:HC Ratio in Stably Transfected CHO Cells and Study of the Impact on Expression, Aggregation, Glycosylation and Conformational Stability. J. Biotechnol. 165, 157–166. doi:10.1016/j.jbiotec.2013.03.019
Hoseinpoor, R., Kazemi, B., Rajabibazl, M., and Rahimpour, A. (2020). Improving the Expression of anti-IL-2Rα Monoclonal Antibody in the CHO Cells through Optimization of the Expression Vector and Translation Efficiency. J. Biotechnol. 324, 112–120. doi:10.1016/j.jbiotec.2020.09.006
Huhn, S. C., Ou, Y., Tang, X., Jiang, B., Liu, R., Lin, H., et al. (2021). Improvement of the Efficiency and Quality in Developing a New CHO Host Cell Line. Biotechnol. Prog. 37, e3185. doi:10.1002/btpr.3185
Hung, F., Deng, L., Ravnikar, P., Condon, R., Li, B., Do, L., et al. (2010). mRNA Stability and Antibody Production in CHO Cells: Improvement through Gene Optimization. Biotechnol. J. 5, 393–401. doi:10.1002/biot.200900192
Hussain, H., Patel, T., Ozanne, A. M. S., Vito, D., Ellis, M., Hinchliffe, M., et al. (2021). A Comparative Analysis of Recombinant Fab and Full‐length Antibody Production in Chinese Hamster Ovary Cells. Biotechnol. Bioeng. 118, 4815–4828. doi:10.1002/bit.27944
Jassal, R., Jenkins, N., Charlwood, J., Camilleri, P., Jefferis, R., and Lund, J. (2001). Sialylation of Human IgG-Fc Carbohydrate by Transfected Rat α2,6-Sialyltransferase. Biochem. Biophysical Res. Commun. 286, 243–249. doi:10.1006/bbrc.2001.5382
Jenkins, N., and Curling, E. M. A. (1994). Glycosylation of Recombinant Proteins: Problems and Prospects. Enzyme Microb. Technology 16, 354–364. doi:10.1016/0141-0229(94)90149-x
Jenkins, N., Parekh, R. B., and James, D. C. (1996). Getting the Glycosylation Right: Implications for the Biotechnology Industry. Nat. Biotechnol. 14, 975–981. doi:10.1038/nbt0896-975
Kaufman, R. J., Wasley, L. C., Spiliotes, A. J., Gossels, S. D., Latt, S. A., Larsen, G. R., et al. (1985). Coamplification and Coexpression of Human Tissue-type Plasminogen Activator and Murine Dihydrofolate Reductase Sequences in Chinese Hamster Ovary Cells. Mol. Cel Biol. 5, 1750–1759. doi:10.1128/mcb.5.7.1750-1759.1985
Kennard, M. L., Goosney, D. L., Monteith, D., Zhang, L., Moffat, M., Fischer, D., et al. (2009). The Generation of Stable, High MAb Expressing CHO Cell Lines Based on the Artificial Chromosome Expression (ACE) Technology. Biotechnol. Bioeng. 104, 540–553. doi:10.1002/bit.22406
Kim, D., Yoon, C., and Lee, G. M. (2021). Small Molecule Epigenetic Modulators for Enhancing Recombinant Antibody Production in CHO Cell Cultures. Biotechnol. Bioeng. 119 (3), 820–831. doi:10.1002/bit.28013
Kim, Y.-G., Kim, J. Y., Mohan, C., and Lee, G. M. (2009). Effect of Bcl-xLoverexpression on Apoptosis and Autophagy in Recombinant Chinese Hamster Ovary Cells under Nutrient-Deprived Condition. Biotechnol. Bioeng. 103, 757–766. doi:10.1002/bit.22298
Kotidis, P., Jedrzejewski, P., Sou, S. N., Sellick, C., Polizzi, K., Del Val, I. J., et al. (2019). Model‐based Optimization of Antibody Galactosylation in CHO Cell Culture. Biotechnol. Bioeng. 116, 1612–1626. doi:10.1002/bit.26960
Krapp, S., Mimura, Y., Jefferis, R., Huber, R., and Sondermann, P. (2003). Structural Analysis of Human IgG-Fc Glycoforms Reveals a Correlation between Glycosylation and Structural Integrity. J. Mol. Biol. 325, 979–989. doi:10.1016/s0022-2836(02)01250-0
Kunert, R., and Reinhart, D. (2016). Advances in Recombinant Antibody Manufacturing. Appl. Microbiol. Biotechnol. 100, 3451–3461. doi:10.1007/s00253-016-7388-9
Kwaks, T. H. J., Barnett, P., Hemrika, W., Siersma, T., Sewalt, R. G. A. B., Satijn, D. P. E., et al. (2003). Identification of Anti-repressor Elements that Confer High and Stable Protein Production in Mammalian Cells. Nat. Biotechnol. 21, 553–558. doi:10.1038/nbt814
Lee, J. S., Ha, T. K., Park, J. H., and Lee, G. M. (2013). Anti-cell Death Engineering of CHO Cells: Co-overexpression of Bcl-2 for Apoptosis Inhibition, Beclin-1 for Autophagy Induction. Biotechnol. Bioeng. 110, 2195–2207. doi:10.1002/bit.24879
Li, F., Vijayasankaran, N., Shen, A., Kiss, R., and Amanullah, A. (2010). Cell Culture Processes for Monoclonal Antibody Production. MAbs 2, 466–479. doi:10.4161/mabs.2.5.12720
Li, J., Menzel, C., Meier, D., Zhang, C., Dübel, S., and Jostock, T. (2007). A Comparative Study of Different Vector Designs for the Mammalian Expression of Recombinant IgG Antibodies. J. Immunological Methods 318, 113–124. doi:10.1016/j.jim.2006.10.010
Li, Y.-m., Wang, M., Wang, T.-y., Wei, Y.-g., Guo, X., Mi, C.-l., et al. (2020). Effects of Different 2A Peptides on Transgene Expression Mediated by Tricistronic Vectors in Transfected CHO Cells. Mol. Biol. Rep. 47, 469–475. doi:10.1007/s11033-019-05153-3
Liao, X., Liang, H., Pan, J., Zhang, Q., Niu, J., Xue, C., et al. (2021). Preparation and Characterization of a Fully Human Monoclonal Antibody Specific for Human Tumor Necrosis Factor Alpha. Bioengineered 12, 10821–10834. doi:10.1080/21655979.2021.1967710
Lin, N., Mascarenhas, J., Sealover, N. R., George, H. J., Brooks, J., Kayser, K. J., et al. (2015). Chinese Hamster Ovary (CHO) Host Cell Engineering to Increase Sialylation of Recombinant Therapeutic Proteins by Modulating Sialyltransferase Expression. Biotechnol. Prog. 31, 334–346. doi:10.1002/btpr.2038
Lin, P.-C., Chan, K. F., Kiess, I. A., Tan, J., Shahreel, W., Wong, S.-Y., et al. (2019). Attenuated Glutamine Synthetase as a Selection Marker in CHO Cells to Efficiently Isolate Highly Productive Stable Cells for the Production of Antibodies and Other Biologics. MAbs 11, 965–976. doi:10.1080/19420862.2019.1612690
Liu, L. (2015). Antibody Glycosylation and its Impact on the Pharmacokinetics and Pharmacodynamics of Monoclonal Antibodies and Fc-Fusion Proteins. J. Pharm. Sci. 104, 1866–1884. doi:10.1002/jps.24444
Liu, R., Zhang, Y., Kumar, A., Huhn, S., Hullinger, L., and Du, Z. (2021). Modulating Tyrosine Sulfation of Recombinant Antibodies in CHO Cell Culture by Host Selection and Sodium Chlorate Supplementation. Biotechnol. J. 16, e2100142. doi:10.1002/biot.202100142
Lu, Y., Zhou, Q., Han, Q., Wu, P., Zhang, L., Zhu, L., et al. (2018). Inactivation of Deubiquitinase CYLD Enhances Therapeutic Antibody Production in Chinese Hamster Ovary Cells. Appl. Microbiol. Biotechnol. 102, 6081–6093. doi:10.1007/s00253-018-9070-x
Luke, G. A., and Ryan, M. D. (2018). Using the 2A Protein Coexpression System: Multicistronic 2A Vectors Expressing Gene(s) of Interest and Reporter Proteins. Methods Mol. Biol. 1755, 31–48. doi:10.1007/978-1-4939-7724-6_3
Masuda, K., Watanabe, K., Ueno, T., Nakazawa, Y., Tanabe, Y., Ushiki-Kaku, Y., et al. (2021). Novel Cell Line Development Strategy for Monoclonal Antibody Manufacturing Using Translational Enhancing Technology. J. Biosci. Bioeng. S1389-1723 (21), 00325–X. doi:10.1016/j.jbiosc.2021.11.010
Matsumiya, S., Yamaguchi, Y., Saito, J.-i., Nagano, M., Sasakawa, H., Otaki, S., et al. (2007). Structural Comparison of Fucosylated and Nonfucosylated Fc Fragments of Human Immunoglobulin G1. J. Mol. Biol. 368, 767–779. doi:10.1016/j.jmb.2007.02.034
Mauro, V. P., and Chappell, S. A. (2018). Considerations in the Use of Codon Optimization for Recombinant Protein Expression. Methods Mol. Biol. 1850, 275–288. doi:10.1007/978-1-4939-8730-6_18
Mauro, V. P. (2018). Codon Optimization in the Production of Recombinant Biotherapeutics: Potential Risks and Considerations. BioDrugs 32, 69–81. doi:10.1007/s40259-018-0261-x
Mohammadian, O., Rajabibazl, M., Pourmaleki, E. h., Bayat, H., Ahani, R., and Rahimpour, A. (2019). Development of an Improved Lentiviral Based Vector System for the Stable Expression of Monoclonal Antibody in CHO Cells. Prep. Biochem. Biotechnol. 49, 822–829. doi:10.1080/10826068.2019.1621893
Morgan, R. A., Unti, M. J., Aleshe, B., Brown, D., Osborne, K. S., Koziol, C., et al. (2020). Improved Titer and Gene Transfer by Lentiviral Vectors Using Novel, Small β-Globin Locus Control Region Elements. Mol. Ther. 28, 328–340. doi:10.1016/j.ymthe.2019.09.020
Naderi, F., Hashemi, M., Bayat, H., Mohammadian, O., Pourmaleki, E. h., Etemadzadeh, M. H., et al. (2018). The Augmenting Effects of the tDNA Insulator on Stable Expression of Monoclonal Antibody in Chinese Hamster Ovary Cells. Monoclonal Antibodies Immunodiagnosis Immunother. 37, 200–206. doi:10.1089/mab.2018.0015
Nematpour, F., Mahboudi, F., Vaziri, B., Khalaj, V., Ahmadi, S., Ahmadi, M., et al. (2017). Evaluating the Expression Profile and Stability of Different UCOE Containing Vector Combinations in mAb-Producing CHO Cells. BMC Biotechnol. 17, 18. doi:10.1186/s12896-017-0330-0
Ng, D., Zhou, M., Zhan, D., Yip, S., Ko, P., Yim, M., et al. (2021). Development of a Targeted Integration Chinese Hamster Ovary Host Directly Targeting Either One or Two Vectors Simultaneously to a Single Locus Using the Cre/Lox Recombinase-Mediated Cassette Exchange System. Biotechnol. Prog. 37, e3140. doi:10.1002/btpr.3140
Nguyen, T. S., Misaki, R., Ohashi, T., and Fujiyama, K. (2020). Enhancement of Sialylation in rIgG in Glyco-Engineered Chinese Hamster Ovary Cells. Cytotechnology 72, 343–355. doi:10.1007/s10616-020-00381-z
Noguchi, C., Araki, Y., Miki, D., and Shimizu, N. (2012). Fusion of the Dhfr/Mtx and IR/MAR Gene Amplification Methods Produces a Rapid and Efficient Method for Stable Recombinant Protein Production. PLoS One 7, e52990. doi:10.1371/journal.pone.0052990
Otte, A. P., Kwaks, T. H. J., van Blokland, R. J. M., Verhees, J., Klaren, V. N. A., Siersma, T. K., et al. (2007). Various Expression-Augmenting DNA Elements Benefit from STAR-Select, a Novel High Stringency Selection System for Protein Expression. Biotechnol. Prog. 23, 801–807. doi:10.1002/bp070107r
Patel, Y. D., Brown, A. J., Zhu, J., Rosignoli, G., Gibson, S. J., Hatton, D., et al. (2021). Control of Multigene Expression Stoichiometry in Mammalian Cells Using Synthetic Promoters. ACS Synth. Biol. 10, 1155–1165. doi:10.1021/acssynbio.0c00643
Pérez-González, A., and Caro, E. (2019). Benefits of Using Genomic Insulators Flanking Transgenes to Increase Expression and Avoid Positional Effects. Sci. Rep. 9, 8474. doi:10.1038/s41598-019-44836-6
Pfaff, N., Lachmann, N., Ackermann, M., Kohlscheen, S., Brendel, C., Maetzig, T., et al. (2013). A Ubiquitous Chromatin Opening Element Prevents Transgene Silencing in Pluripotent Stem Cells and Their Differentiated Progeny. Stem Cells 31, 488–499. doi:10.1002/stem.1316
Raab, N., Zeh, N., Schlossbauer, P., Mathias, S., Lindner, B., Stadermann, A., et al. (2022). A Blueprint from Nature: miRNome Comparison of Plasma Cells and CHO Cells to Optimize Therapeutic Antibody Production. New Biotechnol. 66, 79–88. doi:10.1016/j.nbt.2021.10.005
Ramezani, A., Mahmoudi Maymand, E., Yazdanpanah-Samani, M., Hosseini, A., Toghraie, F. S., and Ghaderi, A. (2017). Improving Pertuzumab Production by Gene Optimization and Proper Signal Peptide Selection. Protein Expr. Purif. 135, 24–32. doi:10.1016/j.pep.2017.04.013
Reinhart, D., Damjanovic, L., Kaisermayer, C., Sommeregger, W., Gili, A., Gasselhuber, B., et al. (2019). Bioprocessing of Recombinant CHO-K1, CHO-DG44, and CHO-S: CHO Expression Hosts Favor Either mAb Production or Biomass Synthesis. Biotechnol. J. 14, e1700686. doi:10.1002/biot.201700686
Ritacco, F. V., Wu, Y., and Khetan, A. (2018). Cell Culture media for Recombinant Protein Expression in Chinese Hamster Ovary (CHO) Cells: History, Key Components, and Optimization Strategies. Biotechnol. Prog. 34, 1407–1426. doi:10.1002/btpr.2706
Rocha-Pizaña, M. D. R., Ascencio-Favela, G., Soto-García, B. M., Martinez-Fierro, M. d. l. L., and Alvarez, M. M. (2017). Evaluation of Changes in Promoters, Use of UCOES and Chain Order to Improve the Antibody Production in CHO Cells. Protein Expr. Purif. 132, 108–115. doi:10.1016/j.pep.2017.01.014
Rudenko, N., Fursova, K., Shepelyakovskaya, A., Karatovskaya, A., and Brovko, F. (2021). Antibodies as Biosensors' Key Components: State-Of-The-Art in Russia 2020-2021. Sensors 21, 7614. doi:10.3390/s21227614
Rybchenko, V. S., Panina, A. A., Aliev, T. K., Solopova, O. N., Balabashin, D. S., Novoseletsky, V. N., et al. (2021). Bispecific Antibodies for IFN-β Delivery to ErbB2+ Tumors. Biomolecules 11, 1915. doi:10.3390/biom11121915
Salinas-Jazmín, N., Medina-Rivero, E., and Velasco-Velázquez, M. A. (2022). For the Evaluation of and of. Methods Mol. Biol. 2313, 281–294. doi:10.1007/978-1-0716-1450-1_17
Savizi, I. S. P., Motamedian, E., E Lewis, N. N., Jimenez Del Val, I., and Shojaosadati, S. A. (2021). An Integrated Modular Framework for Modeling the Effect of Ammonium on the Sialylation Process of Monoclonal Antibodies Produced by CHO Cells. Biotechnol. J. 16, e2100019. doi:10.1002/biot.202100019
Scallon, B. J., Tam, S. H., McCarthy, S. G., Cai, A. N., and Raju, T. S. (2007). Higher Levels of Sialylated Fc Glycans in Immunoglobulin G Molecules Can Adversely Impact Functionality. Mol. Immunol. 44, 1524–1534. doi:10.1016/j.molimm.2006.09.005
Schlatter, S., Stansfield, S. H., Dinnis, D. M., Racher, A. J., Birch, J. R., and James, D. C. (2005). On the Optimal Ratio of Heavy to Light Chain Genes for Efficient Recombinant Antibody Production by CHO Cells. Biotechnol. Prog. 21, 122–133. doi:10.1021/bp049780w
Sha, S., Agarabi, C., Brorson, K., Lee, D.-Y., and Yoon, S. (2016). N-glycosylation Design and Control of Therapeutic Monoclonal Antibodies. Trends Biotechnol. 34, 835–846. doi:10.1016/j.tibtech.2016.02.013
Sharma, B. S., Swain, P. K., and Verma, R. J. (2019). A Systematic Bioinformatics Approach to Motif-Based Analysis of Human Locus Control Regions. J. Comput. Biol. 26, 1427–1437. doi:10.1089/cmb.2019.0155
Soler, D., Young, A., Vahedi-Faridi, A., and McCormick, T. (2018). Generation of Flp-Intm-Ready DG44 and Lec 3.2.8.1 CHO Cell Lines for Quick and Easy Constitutive Protein Expression. Biotechniques 65, 41–46. doi:10.2144/btn-2018-0075
Szabo, M., Filep, C., Nagy, M., Sarkozy, D., Szigeti, M., Sperling, E., et al. (2022). N-glycosylation Structure - Function Characterization of Omalizumab, an Anti-asthma Biotherapeutic Product. J. Pharm. Biomed. Anal. 209, 114483. doi:10.1016/j.jpba.2021.114483
Tanaka, M., Koga, A., Kobe, A., Sekimori, Y., Aso, Y., and Terada, K. (2013). O-linked Glucosylation of a Therapeutic Recombinant Humanised Monoclonal Antibody Produced in CHO Cells. Eur. J. Pharmaceutics Biopharmaceutics 83, 123–130. doi:10.1016/j.ejpb.2012.11.001
Tihanyi, B., and Nyitray, L. (2020). Recent Advances in CHO Cell Line Development for Recombinant Protein Production. Drug Discov. Today Tech. 38, 25–34. doi:10.1016/j.ddtec.2021.02.003
Torres, M., and Dickson, A. J. (2021). Overexpression of Transcription Factor BLIMP1/prdm1 Leads to Growth Inhibition and Enhanced Secretory Capacity in Chinese Hamster Ovary Cells. Metab. Eng. 67, 237–249. doi:10.1016/j.ymben.2021.07.004
Toussaint, C., Henry, O., and Durocher, Y. (2016). Metabolic Engineering of CHO Cells to Alter Lactate Metabolism during Fed-Batch Cultures. J. Biotechnol. 217, 122–131. doi:10.1016/j.jbiotec.2015.11.010
Van Blokland, H., Kwaks, T., Sewalt, R., Verhees, J., Klaren, V., Siersma, T., et al. (2007). A Novel, High Stringency Selection System Allows Screening of Few Clones for High Protein Expression. J. Biotechnol. 128, 237–245. doi:10.1016/j.jbiotec.2006.09.023
Van der Weken, H., Cox, E., and Devriendt, B. (2019). Rapid Production of a Chimeric Antibody-Antigen Fusion Protein Based on 2A-Peptide Cleavage and green Fluorescent Protein Expression in CHO Cells. MAbs 11, 559–568. doi:10.1080/19420862.2019.1574531
Vanhove, M., Usherwood, Y.-K., and Hendershot, L. M. (2001). Unassembled Ig Heavy Chains Do Not Cycle from BiP In Vivo but Require Light Chains to Trigger Their Release. Immunity 15, 105–114. doi:10.1016/s1074-7613(01)00163-7
Vivinus, S., Baulande, S., van Zanten, M., Campbell, F., Topley, P., Ellis, J. H., et al. (2001). An Element within the 5′ Untranslated Region of humanHsp70mRNA Which Acts as a General Enhancer of mRNA Translation. Eur. J. Biochem. 268, 1908–1917. doi:10.1046/j.1432-1327.2001.02064.x
Wang, Q., Chung, C.-Y., Rosenberg, J. N., Yu, G., and Betenbaugh, M. J. (2018). Application of the CRISPR/Cas9 Gene Editing Method for Modulating Antibody Fucosylation in CHO Cells. Methods Mol. Biol. 1850, 237–257. doi:10.1007/978-1-4939-8730-6_16
Wang, X., Liu, H., Yuan, W., Cheng, Y., and Han, W. (2016). Efficient Production of CYTL1 Protein Using Mouse IgGκ Signal Peptide in the CHO Cell Expression System. Acta Biochim. Biophys. Sin 48, 391–394. doi:10.1093/abbs/gmw007
Wei, B., Gao, X., Cadang, L., Izadi, S., Liu, P., Zhang, H.-M., et al. (2021). Fc Galactosylation Follows Consecutive Reaction Kinetics and Enhances Immunoglobulin G Hexamerization for Complement Activation. MAbs 13, 1893427. doi:10.1080/19420862.2021.1893427
Weng, Z., Jin, J., Shao, C., and Li, H. (2020). Reduction of Charge Variants by CHO Cell Culture Process Optimization. Cytotechnology 72, 259–269. doi:10.1007/s10616-020-00375-x
Xu, D. H., Wang, X. Y., Jia, Y. L., Wang, T. Y., Tian, Z. W., Feng, X., et al. (2018). SV40 Intron, a Potent strong Intron Element that Effectively Increases Transgene Expression in Transfected Chinese Hamster Ovary Cells. J. Cel. Mol. Med. 22, 2231–2239. doi:10.1111/jcmm.13504
Xu, Z.-L., Mizuguchi, H., Ishii-Watabe, A., Uchida, E., Mayumi, T., and Hayakawa, T. (2001). Optimization of Transcriptional Regulatory Elements for Constructing Plasmid Vectors. Gene 272, 149–156. doi:10.1016/s0378-1119(01)00550-9
Yang G, G., Wang, Q., Chen, L., Betenbaugh, M. J., and Zhang, H. (2021). Glycoproteomic Characterization of FUT8 Knock-Out CHO Cells Reveals Roles of FUT8 in the Glycosylation. Front. Chem. 9, 755238. doi:10.3389/fchem.2021.755238
Yang Y, Y., Nian, S., Li, L., Wen, X., Liu, Q., Zhang, B., et al. (2021). Fully Human Recombinant Antibodies against EphA2 from a Multi-Tumor Patient Immune Library Suitable for Tumor-Targeted Therapy. Bioengineered 12, 10379–10400. doi:10.1080/21655979.2021.1996807
Yao, G., Aron, K., Borys, M., Li, Z., Pendse, G., and Lee, K. (2021). A Metabolomics Approach to Increasing Chinese Hamster Ovary (CHO) Cell Productivity. Metabolites 11, 823. doi:10.3390/metabo11120823
Yeo, J. H. M., Mariati, Y., and Yang, Y. (2018). An IRES-Mediated Tricistronic Vector for Efficient Generation of Stable, High-Level Monoclonal Antibody Producing CHO DG44 Cell Lines. Methods Mol. Biol. 1827, 335–349. doi:10.1007/978-1-4939-8648-4_17
Yi, C.-h., Ruan, C.-p., Wang, H., Xu, X.-y., Zhao, Y.-p., Fang, M., et al. (2014). Function Characterization of a Glyco-Engineered Anti-EGFR Monoclonal Antibody Cetuximab In Vitro. Acta Pharmacol. Sin. 35, 1439–1446. doi:10.1038/aps.2014.77
Yin, B., Wang, Q., Chung, C. Y., Bhattacharya, R., Ren, X., Tang, J., et al. (2017). A Novel Sugar Analog Enhances Sialic Acid Production and Biotherapeutic Sialylation in CHO Cells. Biotechnol. Bioeng. 114, 1899–1902. doi:10.1002/bit.26291
You, M., Yang, Y., Zhong, C., Chen, F., Wang, X., Jia, T., et al. (2018). Efficient mAb Production in CHO Cells with Optimized Signal Peptide, Codon, and UTR. Appl. Microbiol. Biotechnol. 102, 5953–5964. doi:10.1007/s00253-018-8986-5
Zhang, J.-H., Zhang, J.-H., Wang, X.-Y., Xu, D.-H., and Wang, T.-Y. (2020). Distance Effect Characteristic of the Matrix Attachment Region Increases Recombinant Protein Expression in Chinese Hamster Ovary Cells. Biotechnol. Lett. 42, 187–196. doi:10.1007/s10529-019-02775-2
Zhang, Z., Shah, B., and Richardson, J. (2019). Impact of Fc N-Glycan Sialylation on IgG Structure. MAbs 11, 1381–1390. doi:10.1080/19420862.2019.1655377
Zhao, J., Song, E., Huang, Y., Yu, A., and Mechref, Y. (2021). Variability in the Glycosylation Patterns of Gp120 Proteins from Different Human Immunodeficiency Virus Type 1 Isolates Expressed in Different Host Cells. J. Proteome Res. 20, 4862–4874. doi:10.1021/acs.jproteome.1c00587
Zhou, M., Crawford, Y., Ng, D., Tung, J., Pynn, A. F. J., Meier, A., et al. (2011). Decreasing Lactate Level and Increasing Antibody Production in Chinese Hamster Ovary Cells (CHO) by Reducing the Expression of Lactate Dehydrogenase and Pyruvate Dehydrogenase Kinases. J. Biotechnol. 153, 27–34. doi:10.1016/j.jbiotec.2011.03.003
Zhou, Q., Zhang, Y., Lu, X., Wang, C., Pei, X., Lu, Y., et al. (2021). Stable Overexpression of Mutated PTEN in Chinese Hamster Ovary Cells Enhances Their Performance and Therapeutic Antibody Production. Biotechnol. J. 16, e2000623. doi:10.1002/biot.202000623
Keywords: recombinant antibody, Chinese hamster ovary cells, expression vector, glycosylation, genetic engineering
Citation: Zhang J-H, Shan L-L, Liang F, Du C-Y and Li J-J (2022) Strategies and Considerations for Improving Recombinant Antibody Production and Quality in Chinese Hamster Ovary Cells. Front. Bioeng. Biotechnol. 10:856049. doi: 10.3389/fbioe.2022.856049
Received: 16 January 2022; Accepted: 16 February 2022;
Published: 04 March 2022.
Edited by:
Zivko Nikolov, Texas A and M University, United StatesReviewed by:
Qiong Wang, Johns Hopkins University, United StatesLaura Soto Sierra, Molecular Templates, Inc. (MTEM), United States
Ayswarya Ravi, Corteva Agriscience™, United States
Copyright © 2022 Zhang, Shan, Liang, Du and Li. This is an open-access article distributed under the terms of the Creative Commons Attribution License (CC BY). The use, distribution or reproduction in other forums is permitted, provided the original author(s) and the copyright owner(s) are credited and that the original publication in this journal is cited, in accordance with accepted academic practice. No use, distribution or reproduction is permitted which does not comply with these terms.
*Correspondence: Jun-He Zhang, zjh@xxmu.edu.cn