- 1Department of Biotechnology, Delft University of Technology, Delft, Netherlands
- 2Department of Bionanoscience, Delft University of Technology, Delft, Netherlands
Synthetic Genomics focuses on the construction of rationally designed chromosomes and genomes and offers novel approaches to study biology and to construct synthetic cell factories. Currently, progress in Synthetic Genomics is hindered by the inability to synthesize DNA molecules longer than a few hundred base pairs, while the size of the smallest genome of a self-replicating cell is several hundred thousand base pairs. Methods to assemble small fragments of DNA into large molecules are therefore required. Remarkably powerful at assembling DNA molecules, the unicellular eukaryote Saccharomyces cerevisiae has been pivotal in the establishment of Synthetic Genomics. Instrumental in the assembly of entire genomes of various organisms in the past decade, the S. cerevisiae genome foundry has a key role to play in future Synthetic Genomics developments.
Introduction
Synthetic Genomics (SG) is a recent Synthetic Biology discipline that focuses on the construction of rationally designed chromosomes and genomes. SG offers a novel approach to address fundamental biological questions by restructuring, recoding, and minimizing (parts of) genomes (as recently reviewed by (Coradini et al., 2020)). SG is now spurring technological developments in academia and has a strong future potential in industry (Schindler, 2020; Zhang et al., 2020)). Humankind’s best microbial friend, the baker’s yeast Saccharomyces cerevisiae, has played, and continues to play a key role in SG advances, both by enabling the construction of chromosomes for other hosts, and in the refactoring of its own genome. This mini review explores the reasons for this strategic positioning of S. cerevisiae in SG, surveys the main achievements enabled by this yeast and reflects on future developments.
Current Limitations of Genome Assembly
While small-sized viral chromosomes were the first to be chemically synthetized, the breakthrough in the field of SG came with the synthesis and assembly of the 592 kilobase (kb) chromosome of Mycoplasma genitalium (Gibson et al., 2008a; Gibson et al., 2008b). The unicellular eukaryote Saccharomyces cerevisiae has made a key contribution to this famous milestone. To understand how this microbe, commonly used in food and beverages, contributes to the assembly of synthetic genomes, let us recapitulate how synthetic chromosomes can be constructed (Figure 1).
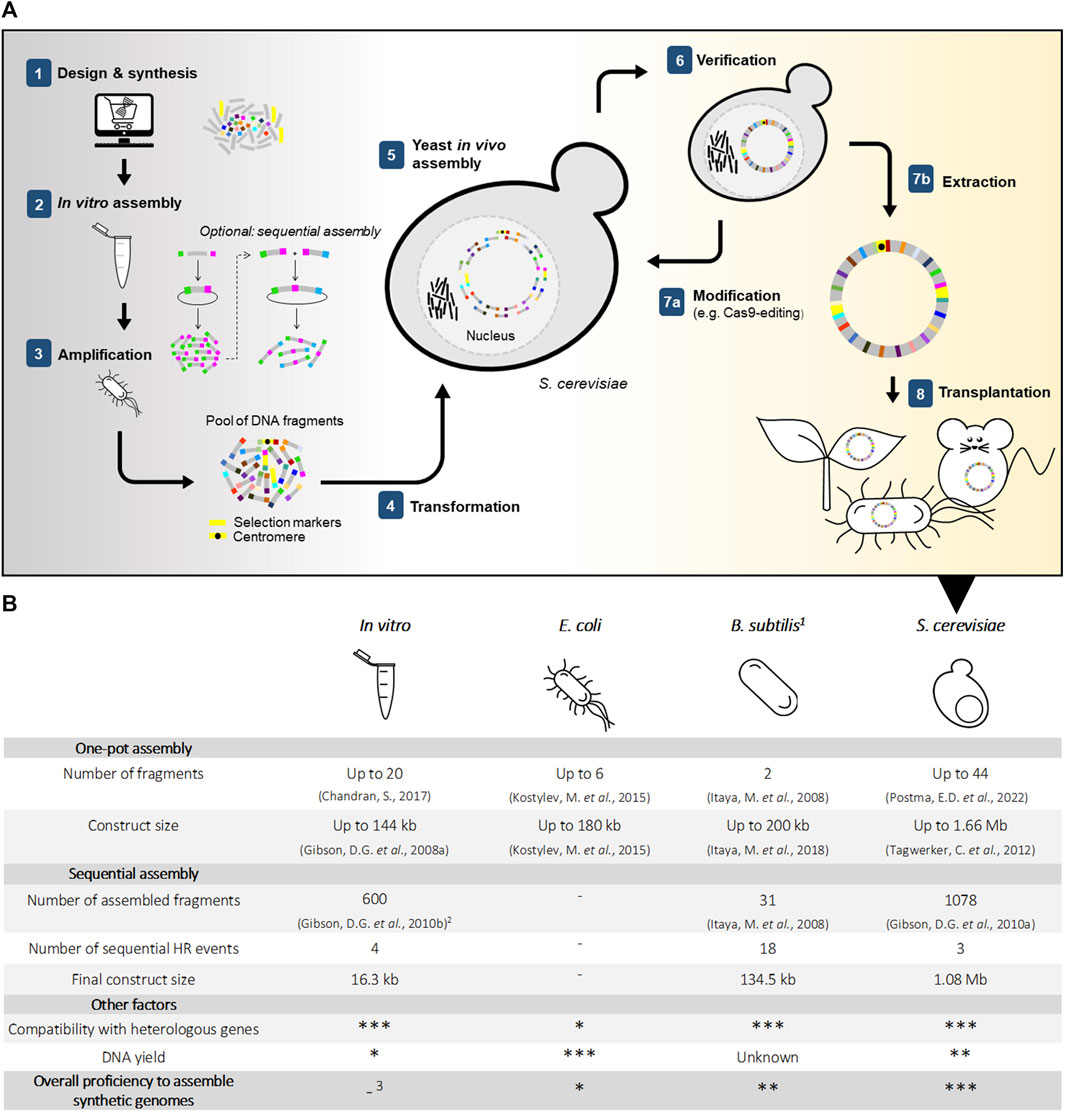
FIGURE 1. In vivo and in vitro approaches for DNA assembly in synthetic genomics (A) Simplified overview of chromosome construction using Saccharomyces cerevisiae for genome assembly and production (B) Strengths and weaknesses of in vitro and in vivo assembly methods. (1) Assembly of fragments in B. subtilis is performed by integration into the host genome. (2) Between rounds of sequential assembly, transformation into E. coli is conventional for selection and amplification of constructs. (3) Requires in vivo amplification and selection in a microbial host.
It starts with the customized synthesis of short DNA molecules called oligonucleotides. Oligonucleotides are mostly synthetized using phosphoramidite chemistry, a 40 year-old method (Beaucage and Caruthers, 1981) that, despite decades of technological developments, struggles to deliver error-free oligonucleotides longer than 200 base pairs (bp). While the implementation of microarrays has substantially decreased the synthesis cost, it has not increased oligo length, an achievement that requires new synthesis methods (Hughes and Ellington, 2017). Enzymatic alternatives for DNA synthesis are under development (Lee H. H. et al., 2019; Lee et al., 2020), but still have considerable shortcomings regarding automation and scalability that must be overcome before commercial scale can be considered (reviewed in (Ostrov et al., 2019; Eisenstein, 2020a; Hao et al., 2020; Paul et al., 2021)). Considering that a theoretical minimal genome would be around 113 kb long (Forster and Church, 2006) and that the first fully synthesized genome of M. genitalium contains 583 kb (Gibson et al., 2008a), thousands of oligos must be stitched together to construct a complete synthetic genome. These DNA oligos can be assembled into longer DNA fragments by using a plethora of in vitro methods (reviewed in (Chao et al., 2014; Casini et al., 2015; Paul et al., 2021)). A method that has gained tremendous popularity since its development is the homology-based Gibson isothermal assembly (Gibson et al., 2009), devised to assemble the M. genitalium genome. As all in vitro methods, Gibson assembly is limited by the number of fragments that can reliably be stitched together in one reaction, usually around a dozen, requiring a stepwise assembly procedure of increasingly large genomic DNA constructs (Gibson et al., 2010b). DNA must be recovered from the reaction, amplified and verified in each round, to allow further processing. Selection and amplification of correctly cloned DNA is routinely performed in Escherichia coli, however, maintenance of large constructs of exogenous DNA, especially from prokaryotic origins, in this bacterium is often limited by expression and toxicity of gene products (Karas et al., 2015). In vitro alternatives for efficient and faithful selection and amplification of correctly assembled DNA are under development, but these are currently limited in length of amplified DNA and scalability (Su’etsugu et al., 2017; van Nies et al., 2018; Libicher et al., 2020; Mukai et al., 2020). While in principle stepwise in vitro assembly can lead to a DNA molecule of any size, and selection and amplification in E. coli worked well for DNA constructs up to 72 kb, E. coli had great difficulties maintaining quarter M. genitalium genomes, causing Gibson and others to turn to baker’s yeast (Gibson et al., 2008a; Gibson et al., 2008b).
Saccharomyces Cerevisiae as a Genome Foundry
S. cerevisiae seems a logical host for SG as it naturally maintains a 12 Mb genome consisting of 16 chromosomes ranging from 230 to 1,500 kb in its haploid version, lives as polyploid in natural environments, and is extremely robust to changes in genome content and architecture (Shao et al., 2018). The extreme robustness of S. cerevisiae to supernumerary, chimeric chromosomes, a key feature for SG, was already demonstrated in the late ‘80s (Burke et al., 1987; Larionov et al., 1996). A second key feature of S. cerevisiae is its preference for homologous recombination (HR) to repair double-strand DNA breaks (Kunes et al., 1985), a rare trait among eukaryotes. S. cerevisiae ability to efficiently and with high fidelity stitch together linear DNA molecules that present homologous regions as short as 40 bp (Noskov et al., 2001) at their ends, was rapidly valorized for genetic manipulations and assembly of heterologous DNA. Recently renamed in vivo assembly, this cloning technique (Figure 1) contributes to the remarkable genetic tractability and popularity of S. cerevisiae as model and industrial microbe (Larionov et al., 1994; Gibson et al., 2009). The combination of S. cerevisiae’s HR efficiency and fidelity, chromosome maintenance and propagation enabled the construction of the full Mycoplasma genome. Reflecting that “in the future, it may be advantageous to make greater use of yeast recombination to assemble chromosomes”, this study propelled S. cerevisiae as powerful ‘genome foundry’ (Gibson et al., 2008a). In the challenge to synthesize genomes, Ostrov and others rightfully identified assembly of these long DNA constructs as “the most critical hurdle” (Ostrov et al., 2019). To date, S. cerevisiae has been key to assembling entire or partial genomes in most synthetic genome projects (Table 1). For instance, the entire 785 kb refactored Caulobacter crescentus (renamed C. ethensis) genome was assembled in vivo from 16 fragments (Venetz et al., 2019), while the recoded E. coli genome was split over 10 fragments of 91–136 kb which were individually assembled in yeast, and then sequentially integrated in the E. coli chromosome to replace native segments (Fredens et al., 2019) (Table 1). In vivo assembly also proved to be powerful in assembling and modifying genomes of organisms that are poorly amenable to genome editing; the rapid and faithful HR-based assembly of S. cerevisiae recently enabled the reconstruction of a synthetic SARS-CoV-2 genome in a single week (Thao et al., 2020), and has been shown to be a promising host for in vivo assembly and modification of other viral genomes (Vashee et al., 2020) as well as the genomes of various pathogens (Benders et al., 2010) and even a 101 kb human gene, which was transplanted into mouse embryonic cells (Mitchell et al., 2021) (Table 1). Moreover, S. cerevisiae was selected for the construction of the first synthetic eukaryotic genome. The international Sc2.0 consortium, spearheaded by Jef Boeke, undertook less than 10 years ago the daunting task of synthesizing recoded versions of the 16 yeast chromosomes. Via stepwise, systematic replacement of 30–40 kb (using ca. 12 DNA fragments of 2–4 kb) of the native yeast sequence, the consortium is close to the completion of the largest synthetic genome to date (Pretorius and Boeke, 2018; Eisenstein, 2020b), with the ambition to reshape and minimize the S. cerevisiae genome (Dai et al., 2020).
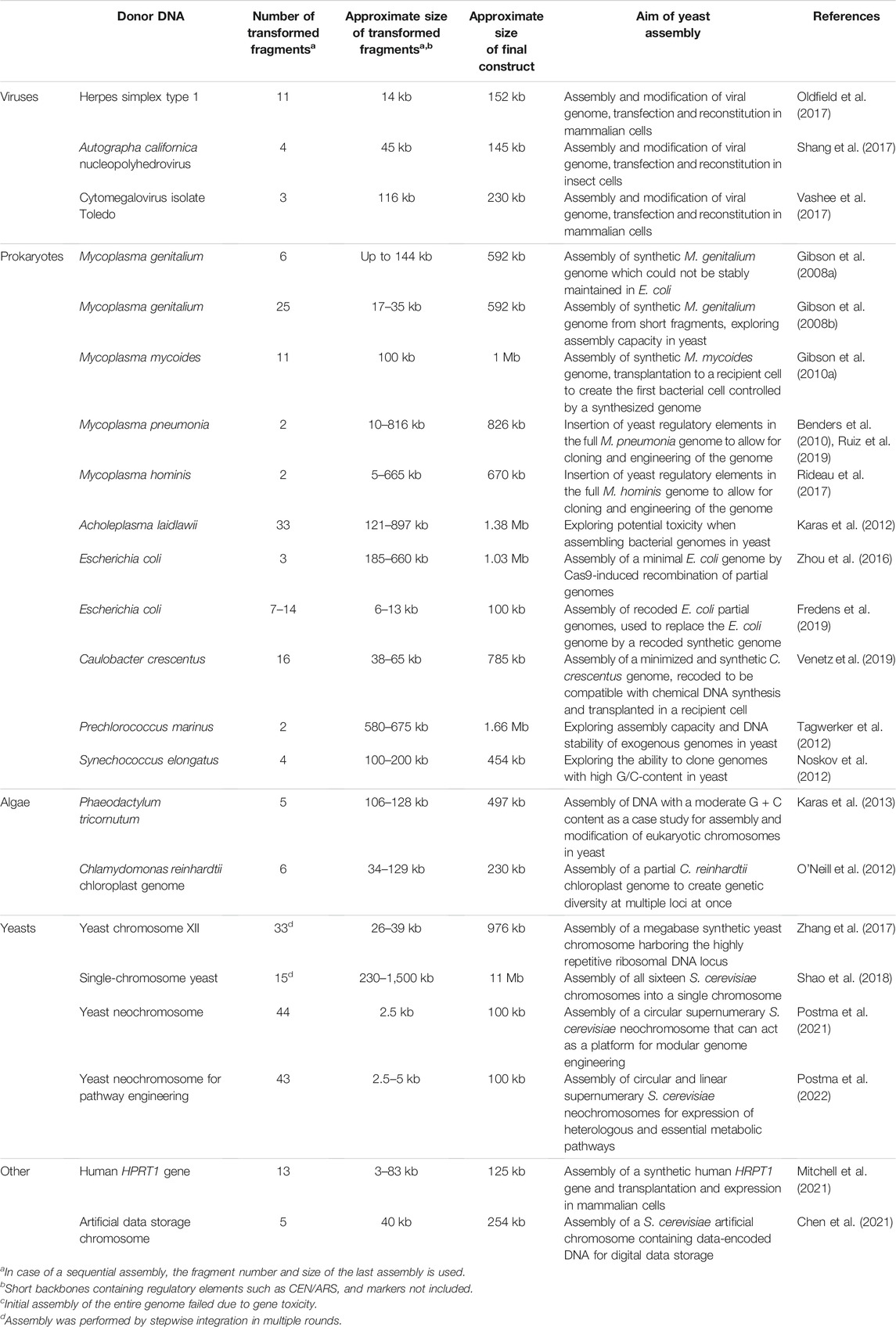
TABLE 1. Overview of the contribution of S. cerevisiae in synthetic genomics by the assembly of large (>100 kb) DNA constructs.
While S. cerevisiae is not the only microbial host available for the construction of (neo)chromosomes (Figure 1), several key features make it superior to its bacterial alternatives Bacillus subtilis and E. coli as genome foundry: 1) S. cerevisiae has the natural ability to carry large amounts of DNA and therefore to host multiple exogenous bacterial genomes (Benders et al., 2010); 2) E. coli frequently struggles with toxicity caused by the expression of exogenous bacterial sequences (Sorek et al., 2007; Gibson et al., 2008b; Karas et al., 2015), while S. cerevisiae is very robust to the presence of heterologous DNA from prokaryotic or eukaryotic origin (Tagwerker et al., 2012); 3) S. cerevisiae can, in a single transformation, assemble many DNA oligonucleotides into (partial) genomes. B. subtilis can also maintain large exogenous DNA constructs, but requires a stepwise method for DNA assembly, in which each DNA part is integrated sequentially into B. subtilis genome (Itaya et al., 2018). This approach is intrinsically more labor-intensive and time-consuming than S. cerevisiae single transformation assembly.
Surprised by S. cerevisiae genetic tractability, Gibson and others wondered “how many pieces can be assembled in yeast in a single step?” (Gibson et al., 2008a). Pioneering a SG approach for metabolic engineering based on modular, specialized synthetic chromosomes, Postma et al. probed this limit recently in our lab by constructing 100 kb artificial linear and circular neochromosomes from 44 DNA parts in a single transformation (Postma et al., 2021; Postma et al., 2022). The remarkable efficiency of in vivo assembly (36% of assemblies faithful to design) revealed that its limit has clearly not been reached yet, and that future systematic studies are required to evaluate the true potential of S. cerevisiae as a genome foundry. The supernumerary chromosomes were shown to stably maintain complete heterologous pathways as well as the yeast’s central carbon metabolism, underlining the potential of yeast synthetic genomics in the development of optimized cell-factories. Once assembled, synthetic chromosomes could be easily edited in S. cerevisiae thanks to its efficient HR and rich molecular toolbox.
Challenges in Genome Assembly Using Yeast
While S. cerevisiae is natively proficient for SG, several aspects of in vivo assembly in yeast are still far from optimal. Firstly, compared to bacterial alternatives, S. cerevisiae cells grow slowly with a maximum specific growth rate around 0.4–0.5 h−1 and are hard to disrupt due to their sturdy cell wall. Considering that large DNA constructs above a few hundred kilobases are sensitive to shear stress, chromosome extraction and purification from S. cerevisiae is possible, but remains tenuous and inefficient, leading to low DNA yields and potentially damaged chromosomes (Blount et al., 2016). Secondly, the strength of S. cerevisiae can become its weakness, as the HR machinery can be overzealous and recombine any (short) DNA sequence with homology within or between the (neo)chromosomes, which may lead to misassemblies. Lastly, non-homologous end joining and microhomology-mediated end joining, DNA repair mechanisms that assemble pieces of DNA with no or minimal homology, are present in S. cerevisiae with low activity (Ranjha et al., 2018; Lee K. et al., 2019), and can also cause misassemblies. Similar to how E. coli was engineered to become a lab tool for DNA amplification, these shortcomings could be alleviated by engineering S. cerevisiae into a more powerful genome foundry.
Are there future alternatives to S. cerevisiae? Naturally, B. subtilis and E. coli could also be engineered. However, considering the minute fraction of the vast microbial biodiversity that has been tested for genetic accessibility and DNA assembly, it is likely that microbes yet to be discovered are even better genome foundries. Environments causing extreme DNA damage (high radiation, toxic chemicals, etc.) might be a source of HR-proficient organisms (e.g. (Albarracín et al., 2012; Sato et al., 2020)) better suited for SG.
In a more distant future, in vitro alternatives might replace the need for live DNA foundries altogether, thereby accelerating and simplifying genome construction. However, this will require major technological advances in in vitro DNA assembly and amplification. Already substantial efforts have led to the development of methods for DNA amplification, such as rolling circle amplification by the phage φ29 DNA polymerase (Dean, 2001; Lau et al., 2017), recently implemented for the amplification of a 116 kb multipartite genome (Libicher et al., 2020) and the in vitro amplification of synthetic genomes using the E. coli replisome, which already demonstrated to be capable of amplification of 1 Mb synthetic genomes (Mukai et al., 2020). Targets for improvement of these methods are the maximal length of amplified DNA fragments, the yield of amplification, the need for restriction of the amplified, concatenated molecules or the formation of non-specifically amplified products. The development of an in vitro approach that can parallel S. cerevisiae in vivo assembly capability seems even more challenging. While an interesting avenue might be to transplant S. cerevisiae HR DNA repair in vitro, it presents a daunting task considering that all players and their respective role have not been fully elucidated yet (Kwon et al., 2017; Ranjha et al., 2018). Still, considering that highly complex systems such as the transcription and translation machineries have been successfully implemented in vitro and are commercially available (Shimizu et al., 2001), cell-free S. cerevisiae HR might become a reality in the coming years.
Outlook
Since the first genome synthesis in 2008, relatively few genomes have been synthetized. Low-cost, customizable construction of designer genomes, currently accessible for small viral, organellar or bacterial constructs, is still out of reach for large (eukaryotic) genomes. There are still numerous technical, financial, and computational hurdles that must be overcome on the road to microbial designer genomes, tailored to applications in bio-based industry. Here we reviewed why the yeast S. cerevisiae is a key organism in the field of SG, however, the spectrum of available hosts is expected to increase as research in SG advances. For example, a recent study shows improving the HR capacity of the industrially relevant yeast Yarrowia lipolytica could greatly expand the potential applications of SG in bio-based processes (Guo et al., 2020).
In the near future, SG is anticipated to contribute to various fields, such as a platform technology for industrial biotechnological processes (Schindler, 2020; Postma et al., 2022), as a new means for data storage (Chen et al., 2021) and for the development of new cell therapies and other medical applications, which is the ambition of the Genome Project-Write (Boeke et al., 2016). In parallel, worldwide bottom-up approaches endeavor to construct synthetic cells from scratch, such as the European consortia BaSyC (http://www.basyc.nl), MaxSynBio (https://www.maxsynbio.mpg.de) and the Synthetic cell initiative (http://www.syntheticcell.eu) and the US-based Build-a-cell initiative (http://buildacell.io) (reviewed in Mutschler et al., 2019). Looking further ahead, SG may even assist in understanding and engineering entire ecosystems by assembly of a metagenomes in a single cell (Belda et al., 2021). SG, albeit still in its infancy and mostly limited to academic research, has bright days ahead, and S. cerevisiae is foreseen to remain a valuable, if not indispensable, SG tool for the coming decade.
Author Contributions
All authors contributed to the work presented in this article. CK, EP, EK and PD-L conceived and drafted the manuscript, CK, EP, EK, and CC performed literature research and wrote sections of the manuscript, PD-L edited and revised the manuscript. All authors read and agreed to the final manuscript.
Funding
This work was funded by AdLibYeast ERC consolidator grant (648141 to PD-L.); European Union’s Horizon 2020 Framework Programme for Research and Innovation and “BaSyC—Building a Synthetic Cell” Gravitation grant (024.003.019).
Conflict of Interest
The authors declare that the research was conducted in the absence of any commercial or financial relationships that could be construed as a potential conflict of interest.
Publisher’s Note
All claims expressed in this article are solely those of the authors and do not necessarily represent those of their affiliated organizations, or those of the publisher, the editors and the reviewers. Any product that may be evaluated in this article, or claim that may be made by its manufacturer, is not guaranteed or endorsed by the publisher.
References
Albarracín, V. H., Pathak, G. P., Douki, T., Cadet, J., Borsarelli, C. D., Gärtner, W., et al. (2012). Extremophilic Acinetobacter Strains from High-Altitude Lakes in Argentinean Puna: Remarkable UV-B Resistance and Efficient DNA Damage Repair. Orig Life Evol. Biosph. 42 (2-3), 201–221. doi:10.1007/s11084-012-9276-3
Beaucage, S. L., and Caruthers, M. H. (1981). Deoxynucleoside Phosphoramidites-A New Class of Key Intermediates for Deoxypolynucleotide Synthesis. Tetrahedron Lett. 22 (20), 1859–1862. doi:10.1016/s0040-4039(01)90461-7
Belda, I., Williams, T. C., de Celis, M., Paulsen, I. T., and Pretorius, I. S. (2021). Seeding the Idea of Encapsulating a Representative Synthetic Metagenome in a Single Yeast Cell. Nat. Commun. 12 (1), 1599–1608. doi:10.1038/s41467-021-21877-y
Benders, G. A., Noskov, V. N., Denisova, E. A., Lartigue, C., Gibson, D. G., Assad-Garcia, N., et al. (2010). Cloning Whole Bacterial Genomes in Yeast. Nucleic Acids Res. 38 (8), 2558–2569. doi:10.1093/nar/gkq119
Blount, B. A., Driessen, M. R. M., and Ellis, T. (2016). GC Preps: Fast and Easy Extraction of Stable Yeast Genomic DNA. Sci. Rep. 6 (1), 26863. doi:10.1038/srep26863
Boeke, J. D., Church, G., Hessel, A., Kelley, N. J., Arkin, A., Cai, Y., et al. (2016). The Genome Project-Write. Science 353 (6295), 126–127. doi:10.1126/science.aaf6850
Burke, D. T., Carle, G. F., and Olson, M. V. (1987). Cloning of Large Segments of Exogenous DNA into Yeast by Means of Artificial Chromosome Vectors. Science 236 (4803), 806–812. doi:10.1126/science.3033825
Casini, A., Storch, M., Baldwin, G. S., and Ellis, T. (2015). Bricks and Blueprints: Methods and Standards for DNA Assembly. Nat. Rev. Mol. Cell Biol 16 (9), 568–576. doi:10.1038/nrm4014
Chao, R., Yuan, Y., and Zhao, H. (2015). Recent Advances in DNA Assembly Technologies. FEMS Yeast Res. 15 (1), 1–9. doi:10.1111/1567-1364.12171
Chen, W., Han, M., Zhou, J., Ge, Q., Wang, P., Zhang, X., et al. (2021). An Artificial Chromosome for Data Storage. Natl. Sci. Rev. 8 (5), nwab028. doi:10.1093/nsr/nwab028
Coradini, A. L. V., Hull, C. B., and Ehrenreich, I. M. (2020). Building Genomes to Understand Biology. Nat. Commun. 11 (1), 6177. doi:10.1038/s41467-020-19753-2
Dai, J., Boeke, J. D., Luo, Z., Jiang, S., and Cai, Y. (2020). Sc3.0: Revamping and Minimizing the Yeast Genome. Genome Biol. 21 (1), 205. doi:10.1186/s13059-020-02130-z
Dean, F. B., Nelson, J. R., Giesler, T. L., and Lasken, R. S. (2001). Rapid Amplification of Plasmid and Phage DNA Using Phi29 DNA Polymerase and Multiply-Primed Rolling Circle Amplification. Genome Res. 11 (6), 1095–1099. doi:10.1101/gr.180501
Eisenstein, M. (2020a). Enzymatic DNA Synthesis Enters New Phase. Nat. Biotechnol. 38 (10), 1113–1115. doi:10.1038/s41587-020-0695-9
Eisenstein, M. (2020b). How to Build a Genome. Nature 578 (7796), 633–635. doi:10.1038/d41586-020-00511-9
Forster, A. C., and Church, G. M. (2006). Towards Synthesis of a Minimal Cell. Mol. Syst. Biol. 2, 45. doi:10.1038/msb4100090
Fredens, J., Wang, K., de la Torre, D., Funke, L. F. H., Robertson, W. E., Christova, Y., et al. (2019). Total Synthesis of Escherichia coli with a Recoded Genome. Nature 569 (7757), 514–518. doi:10.1038/s41586-019-1192-5
Gibson, D. G., Benders, G. A., Andrews-Pfannkoch, C., Denisova, E. A., Baden-Tillson, H., Zaveri, J., et al. (2008a). Complete Chemical Synthesis, Assembly, and Cloning of a Mycoplasma Genitalium Genome. science 319 (5867), 1215–1220. doi:10.1126/science.1151721
Gibson, D. G., Benders, G. A., Axelrod, K. C., Zaveri, J., Algire, M. A., Moodie, M., et al. (2008b). One-step Assembly in Yeast of 25 Overlapping DNA Fragments to Form a Complete Synthetic Mycoplasma Genitalium Genome. Proc. Natl. Acad. Sci. 105 (51), 20404–20409. doi:10.1073/pnas.0811011106
Gibson, D. G., Glass, J. I., Lartigue, C., Noskov, V. N., Chuang, R.-Y., Algire, M. A., et al. (2010a). Creation of a Bacterial Cell Controlled by a Chemically Synthesized Genome. science 329 (5987), 52–56. doi:10.1126/science.1190719
Gibson, D. G., Smith, H. O., Hutchison, C. A., Venter, J. C., and Merryman, C. (2010b). Chemical Synthesis of the Mouse Mitochondrial Genome. Nat. Methods 7 (11), 901–903. doi:10.1038/nmeth.1515
Gibson, D. G., Young, L., Chuang, R.-Y., Venter, J. C., Hutchison, C. A., and Smith, H. O. (2009). Enzymatic Assembly of DNA Molecules up to Several Hundred Kilobases. Nat. Methods 6 (5), 343–345. doi:10.1038/nmeth.1318
Guo, Z. P., Borsenberger, V., Croux, C., Duquesne, S., Truan, G., Marty, A., et al. (2020). An Artificial Chromosome ylAC Enables Efficient Assembly of Multiple Genes in Yarrowia Lipolytica for Biomanufacturing. Commun. Biol. 3 (1), 199–210. doi:10.1038/s42003-020-0936-y
Hao, M., Qiao, J., and Qi, H. (2020). Current and Emerging Methods for the Synthesis of Single-Stranded DNA. Genes 11 (2), 116. doi:10.3390/genes11020116
Hughes, R. A., and Ellington, A. D. (2017). Synthetic DNA Synthesis and Assembly: Putting the Synthetic in Synthetic Biology. Cold Spring Harb Perspect. Biol. 9 (1), a023812. doi:10.1101/cshperspect.a023812
Itaya, M., Sato, M., Hasegawa, M., Kono, N., Tomita, M., and Kaneko, S. (2018). Far Rapid Synthesis of Giant DNA in the Bacillus Subtilis Genome by a Conjugation Transfer System. Sci. Rep. 8 (1), 8792. doi:10.1038/s41598-018-26987-0
Karas, B. J., Molparia, B., Jablanovic, J., Hermann, W. J., Lin, Y.-C., Dupont, C. L., et al. (2013). Assembly of Eukaryotic Algal Chromosomes in Yeast. J. Biol. Eng. 7 (1), 30. doi:10.1186/1754-1611-7-30
Karas, B. J., Suzuki, Y., and Weyman, P. D. (2015). Strategies for Cloning and Manipulating Natural and Synthetic Chromosomes. Chromosome Res. 23 (1), 57–68. doi:10.1007/s10577-014-9455-3
Karas, B. J., Tagwerker, C., Yonemoto, I. T., Hutchison, C. A., and Smith, H. O. (2012). Cloning the Acholeplasma Laidlawii PG-8A Genome in Saccharomyces cerevisiae as a Yeast Centromeric Plasmid. ACS Synth. Biol. 1 (1), 22–28. doi:10.1021/sb200013j
Kunes, S., Botstein, D., and Fox, M. S. (1985). Transformation of Yeast with Linearized Plasmid DNA. J. Mol. Biol. 184 (3), 375–387. doi:10.1016/0022-2836(85)90288-8
Kwon, Y., Daley, J. M., and Sung, P. (2017). Reconstituted System for the Examination of Repair DNA Synthesis in Homologous Recombination. Methods Enzymol. 591, 307–325. doi:10.1016/bs.mie.2017.03.021
Larionov, V., Kouprina, N., Eldarov, M., Perkins, E., Porter, G., and Resnick, M. A. (1994). Transformation-associated Recombination between Diverged and Homologous DNA Repeats Is Induced by Strand Breaks. Yeast 10 (1), 93–104. doi:10.1002/yea.320100109
Larionov, V., Kouprina, N., Graves, J., Chen, X. N., Korenberg, J. R., and Resnick, M. A. (1996). Specific Cloning of Human DNA as Yeast Artificial Chromosomes by Transformation-Associated Recombination. Proc. Natl. Acad. Sci. 93 (1), 491–496. doi:10.1073/pnas.93.1.491
Lau, Y. H., Stirling, F., Kuo, J., Karrenbelt, M. A. P., Chan, Y. A., Riesselman, A., et al. (2017). Large-scale Recoding of a Bacterial Genome by Iterative Recombineering of Synthetic DNA. Nucleic Acids Res. 45 (11), 6971–6980. doi:10.1093/nar/gkx415
Lee, H., Wiegand, D. J., Griswold, K., Punthambaker, S., Chun, H., Kohman, R. E., et al. (2020). Photon-directed Multiplexed Enzymatic DNA Synthesis for Molecular Digital Data Storage. Nat. Commun. 11 (1), 5246–5249. doi:10.1038/s41467-020-18681-5
Lee, H. H., Kalhor, R., Goela, N., Bolot, J., and Church, G. M. (2019). Terminator-free Template-independent Enzymatic DNA Synthesis for Digital Information Storage. Nat. Commun. 10 (1), 2383–2412. doi:10.1038/s41467-019-10258-1
Lee, K., Ji, J.-H., Yoon, K., Che, J., Seol, J.-H., Lee, S. E., et al. (2019). Microhomology Selection for Microhomology Mediated End Joining in Saccharomyces cerevisiae. Genes 10 (4), 284. doi:10.3390/genes10040284
Libicher, K., Hornberger, R., Heymann, M., and Mutschler, H. (2020). In Vitro self-replication and Multicistronic Expression of Large Synthetic Genomes. Nat. Commun. 11 (1), 904–908. doi:10.1038/s41467-020-14694-2
Mitchell, L. A., McCulloch, L. H., Pinglay, S., Berger, H., Bosco, N., Brosh, R., et al. (2021). De Novo assembly and Delivery to Mouse Cells of a 101 Kb Functional Human Gene. Genetics 218 (1), iyab038. doi:10.1093/genetics/iyab038
Mukai, T., Yoneji, T., Yamada, K., Fujita, H., Nara, S., and Su’etsugu, M. (2020). Overcoming the Challenges of Megabase-Sized Plasmid Construction in Escherichia coli. ACS Synth. Biol. 9 (6), 1315–1327. doi:10.1021/acssynbio.0c00008
Mutschler, H., Robinson, T., Tang, T. Y. D., and Wegner, S. (2019). Special Issue on Bottom‐Up Synthetic Biology. ChemBioChem 20 (20), 2533–2534. doi:10.1002/cbic.201900507
Noskov, V. N., Koriabine, M., Solomon, G., Randolph, M., Barrett, J. C., Leem, S. H., et al. (2001). Defining the Minimal Length of Sequence Homology Required for Selective Gene Isolation by TAR Cloning. Nucleic Acids Res. 29 (6), e32. doi:10.1093/nar/29.6.e32
Noskov, V. N., Karas, B. J., Young, L., Chuang, R.-Y., Gibson, D. G., Lin, Y.-C., et al. (2012). Assembly of Large, High G+C Bacterial DNA Fragments in Yeast. ACS Synth. Biol. 1 (7), 267–273. doi:10.1021/sb3000194
O'Neill, B. M., Mikkelson, K. L., Gutierrez, N. M., Cunningham, J. L., Wolff, K. L., Szyjka, S. J., et al. (2012). An Exogenous Chloroplast Genome for Complex Sequence Manipulation in Algae. Nucleic Acids Res. 40 (6), 2782–2792. doi:10.1093/nar/gkr1008
Oldfield, L. M., Grzesik, P., Voorhies, A. A., Alperovich, N., Macmath, D., Najera, C. D., et al. (2017). Genome-wide Engineering of an Infectious Clone of Herpes Simplex Virus Type 1 Using Synthetic Genomics Assembly Methods. Proc. Natl. Acad. Sci. USA 114 (42), E8885–E8894. doi:10.1073/pnas.1700534114
Ostrov, N., Beal, J., Ellis, T., Gordon, D. B., Karas, B. J., Lee, H. H., et al. (2019). Technological Challenges and Milestones for Writing Genomes. Science 366 (6463), 310–312. doi:10.1126/science.aay0339
Paul, S. S., Trabelsi, H., Yaseen, Y., Basu, U., Altaii, H. A., and Dhali, D. (2021). “Advances in Long DNA Synthesis,” in Microbial Cell Factories Engineering for Production of Biomolecules (Elsevier), 21–36. doi:10.1016/b978-0-12-821477-0.00014-3
Postma, E. D., Dashko, S., van Breemen, L., Taylor Parkins, S. K., Van Den Broek, M., Daran, J.-M., et al. (2021). A Supernumerary Designer Chromosome for Modular In Vivo Pathway Assembly in Saccharomyces cerevisiae. Nucleic Acids Res. 49 (3), 1769–1783. doi:10.1093/nar/gkaa1167
Postma, E. D., Hassing, E.-J., Mangkusaputra, V., Geelhoed, J., de la Torre, P., van den Broek, M., et al. (2022). Modular, Synthetic Chromosomes as New Tools for Large Scale Engineering of Metabolism. Metabol. Eng. 72, 1–13. doi:10.1016/j.ymben.2021.12.013
Pretorius, I. S., and Boeke, J. D. (2018). Yeast 2.0-connecting the Dots in the Construction of the World's First Functional Synthetic Eukaryotic Genome. FEMS Yeast Res. 18 (4), foy032. doi:10.1093/femsyr/foy032
Ranjha, L., Howard, S. M., and Cejka, P. (2018). Main Steps in DNA Double-Strand Break Repair: an Introduction to Homologous Recombination and Related Processes. Chromosoma 127 (2), 187–214. doi:10.1007/s00412-017-0658-1
Rideau, F., Le Roy, C., Descamps, E. C. T., Renaudin, H., Lartigue, C., and Bébéar, C. (2017). Cloning, Stability, and Modification of Mycoplasma Hominis Genome in Yeast. ACS Synth. Biol. 6 (5), 891–901. doi:10.1021/acssynbio.6b00379
Ruiz, E., Talenton, V., Dubrana, M.-P., Guesdon, G., Lluch-Senar, M., Salin, F., et al. (2019). CReasPy-cloning: a Method for Simultaneous Cloning and Engineering of Megabase-Sized Genomes in Yeast Using the CRISPR-Cas9 System. ACS Synth. Biol. 8 (11), 2547–2557. doi:10.1021/acssynbio.9b00224
Sato, T., Takada, D., Itoh, T., Ohkuma, M., and Atomi, H. (2020). Integration of Large Heterologous DNA Fragments into the Genome of Thermococcus Kodakarensis. Extremophiles 24 (3), 339–353. doi:10.1007/s00792-020-01159-z
Schindler, D. (2020). Genetic Engineering and Synthetic Genomics in Yeast to Understand Life and Boost Biotechnology. Bioengineering 7 (4), 137. doi:10.3390/bioengineering7040137
Shang, Y., Wang, M., Xiao, G., Wang, X., Hou, D., Pan, K., et al. (2017). Construction and rescue of a Functional Synthetic Baculovirus. ACS Synth. Biol. 6 (7), 1393–1402. doi:10.1021/acssynbio.7b00028
Shao, Y., Lu, N., Wu, Z., Cai, C., Wang, S., Zhang, L.-L., et al. (2018). Creating a Functional Single-Chromosome Yeast. Nature 560 (7718), 331–335. doi:10.1038/s41586-018-0382-x
Shimizu, Y., Inoue, A., Tomari, Y., Suzuki, T., Yokogawa, T., Nishikawa, K., et al. (2001). Cell-free Translation Reconstituted with Purified Components. Nat. Biotechnol. 19 (8), 751–755. doi:10.1038/90802
Sorek, R., Zhu, Y., Creevey, C. J., Francino, M. P., Bork, P., and Rubin, E. M. (2007). Genome-Wide Experimental Determination of Barriers to Horizontal Gene Transfer. Science 318 (5855), 1449–1452. doi:10.1126/science.1147112
Su’etsugu, M., Takada, H., Katayama, T., and Tsujimoto, H. (2017). Exponential Propagation of Large Circular DNA by Reconstitution of a Chromosome-Replication Cycle. Nucleic Acids Res. 45 (20), 11525–11534. doi:10.1093/nar/gkx822
Tagwerker, C., Dupont, C. L., Karas, B. J., Ma, L., Chuang, R.-Y., Benders, G. A., et al. (2012). Sequence Analysis of a Complete 1.66 Mb Prochlorococcus Marinus MED4 Genome Cloned in Yeast. Nucleic Acids Res. 40 (20), 10375–10383. doi:10.1093/nar/gks823
Thi Nhu Thao, T., Labroussaa, F., Ebert, N., V’kovski, P., Stalder, H., Portmann, J., et al. (2020). Rapid Reconstruction of SARS-CoV-2 Using a Synthetic Genomics Platform. Nature 582 (7813), 561–565. doi:10.1038/s41586-020-2294-9
van Nies, P., Westerlaken, I., Blanken, D., Salas, M., Mencía, M., and Danelon, C. (2018). Self-replication of DNA by its Encoded Proteins in Liposome-Based Synthetic Cells. Nat. Commun. 9 (1), 1583. doi:10.1038/s41467-018-03926-1
Vashee, S., Stockwell, T. B., Alperovich, N., Denisova, E. A., Gibson, D. G., Cady, K. C., et al. (2017). Cloning, Assembly, and Modification of the Primary Human Cytomegalovirus Isolate Toledo by Yeast-Based Transformation-Associated Recombination. Msphere 2 (5), e00331–00317. doi:10.1128/mSphereDirect.00331-17
Vashee, S., Arfi, Y., and Lartigue, C. (2020). Budding Yeast as a Factory to Engineer Partial and Complete Microbial Genomes. Curr. Opin. Syst. Biol. 24, 1–8. doi:10.1016/j.coisb.2020.09.003
Venetz, J. E., Del Medico, L., Wölfle, A., Schächle, P., Bucher, Y., Appert, D., et al. (2019). Chemical Synthesis Rewriting of a Bacterial Genome to Achieve Design Flexibility and Biological Functionality. Proc. Natl. Acad. Sci. USA 116 (16), 8070–8079. doi:10.1073/pnas.1818259116
Zhang, W., Zhao, G., Luo, Z., Lin, Y., Wang, L., Guo, Y., et al. (2017). Engineering the Ribosomal DNA in a Megabase Synthetic Chromosome. Science 355 (6329), eaaf3981. doi:10.1126/science.aaf3981
Zhang, W., Mitchell, L. A., Bader, J. S., and Boeke, J. D. (2020). Synthetic Genomes. Annu. Rev. Biochem. 89, 77–101. doi:10.1146/annurev-biochem-013118-110704
Keywords: synthetic genomics, yeast, Saccharomyces cerevisiae (Baker’s yeast), genome foundry, DNA assembly, synthetic cells
Citation: Koster CC, Postma ED, Knibbe E, Cleij C and Daran-Lapujade P (2022) Synthetic Genomics From a Yeast Perspective. Front. Bioeng. Biotechnol. 10:869486. doi: 10.3389/fbioe.2022.869486
Received: 04 February 2022; Accepted: 28 February 2022;
Published: 21 March 2022.
Edited by:
Ralf Takors, University of Stuttgart, GermanyReviewed by:
Mario Andrea Marchisio, Tianjin University, ChinaCopyright © 2022 Koster, Postma, Knibbe, Cleij and Daran-Lapujade. This is an open-access article distributed under the terms of the Creative Commons Attribution License (CC BY). The use, distribution or reproduction in other forums is permitted, provided the original author(s) and the copyright owner(s) are credited and that the original publication in this journal is cited, in accordance with accepted academic practice. No use, distribution or reproduction is permitted which does not comply with these terms.
*Correspondence: Pascale Daran-Lapujade, cC5hLnMuZGFyYW4tbGFwdWphZGVAdHVkZWxmdC5ubA==
†These authors have contributed equally to this work and share first authorship