Rapid and Facile Preparation of Giant Vesicles by the Droplet Transfer Method for Artificial Cell Construction
- 1Institute for Extra-cutting-edge Science and Technology Avant-garde Research (X-star), Japan Agency for Marine-Earth Science and Technology (JAMSTEC), Yokosuka, Japan
- 2Research Institute of Industrial Technology, Toyo University, Saitama, Japan
- 3PRESTO, Japan Science and Technology Agency (JST), Saitama, Japan
- 4Graduate School of Nanobioscience, Yokohama City University, Yokohama, Japan
Giant vesicles have been widely used for the bottom-up construction of artificial (or synthetic) cells and the physicochemical analysis of lipid membranes. Although methods for the formation of giant vesicles and the encapsulation of molecules within them have been established, a standardized protocol has not been shared among researchers including non-experts. Here we proposed a rapid and facile protocol that allows the formation of giant vesicles within 30 min. The quality of the giant vesicles encapsulating a cell-free protein expression system was comparable to that of the ones formed using a conventional method, in terms of the synthesis of both soluble and membrane proteins. We also performed protein synthesis in artificial cells using a lyophilized cell-free mixture and showed an equivalent level of protein synthesis. Our method could become a standard method for giant vesicle formation suited for artificial cell research.
Introduction
The droplet transfer method, which is also known as the inverted emulsion method, has been widely used for the formation of giant vesicles (GVs) with a diameter of tens of micrometers (Pautot et al., 2003; Walde et al., 2010). GVs have been applied as a model cell membrane for the investigation of the physical properties of lipid membranes in the field of soft-matter physics (Jimbo et al., 2016; Oda et al., 2020; Lowe et al., 2022), and for the construction of artificial cells in the field of synthetic biology (Lu et al., 2021). Although several methods have been developed to form GVs, such as electro formation (Angelova and Dimitrov, 1986) or film hydration (Reeves and Dowben, 1969; Tsumoto et al., 2009), the droplet transfer method (Moga et al., 2019) has advantages in the generation of single lamellar membrane vesicles and its application to biochemical experiments requiring physiological conditions. The principle of this method is as follows: the water-in-oil (W/O) droplets, which are stabilized by a monolayer of phospholipids, pass through another monolayer formed at the interface of a lipid-oil layer and an aqueous layer; as a consequence, lipid bilayer vesicles are formed in the aqueous solution (Noireaux and Libchaber, 2004).
Although GVs are highly versatile materials, it is not always easy to obtain quality GVs with high reproducibility, which often fails even if following the same experimental steps. Moreover, beginners who have no experience in preparing GVs may be more likely to have an experimental failure. This tendency increases as the composition of the internal solution become more complex. To reduce these difficulties, some tailor-made devices have been created and applied in the droplet transfer method (Morita et al., 2018; Bashirzadeh et al., 2021). In addition to the droplet transfer method, more advanced methods using microfluidic devices have recently been developed and will become the main technology in this field (Robinson, 2019; Kamiya, 2020). However, designing the devices, creating the template mold, and the relatively high cost for the system set up hesitate us to choose this method. Therefore, establishing a concise protocol that allows the easy and rapid preparation of GVs with high reproducibility is important to reduce experimental errors.
In this report, we present a handy protocol that allows the completion of all experimental steps, from the lipid-oil preparation to the GV formation, within 30 min without the use of specific devices. The reproducibility of the method is high and a sufficient population of GVs can be produced. We show that we have successfully prepared artificial cells by this method using a cell-free protein expression system (cell-free system) that has been lyophilized and rehydrated. Because the technique of GV formation is the basis of artificial cell experiments, we aim to standardize our method for the development of artificial (synthetic) cell research.
Overview of the Experimental Approach Used for GV Formation
As shown in Figure 1, GV formation is initiated by preparing a lipid-oil mixture containing the desired lipid composition. The prepared lipid-oil mixture is used to form W/O droplets by mixing with the inner solution, which is composed of the desired reaction mixture, e.g., a cell-free system. The prepared droplets are layered on the outer solution. Giving a force by centrifugation induces the passage of the droplets through the interface between the oil and aqueous layers, thus forming lipid bilayer GVs in the aqueous solution. The formed GVs are collected and used for a subsequent experiment. If needed, the GVs can be washed with the outer solutions to remove the components that leaked from the broken droplets.
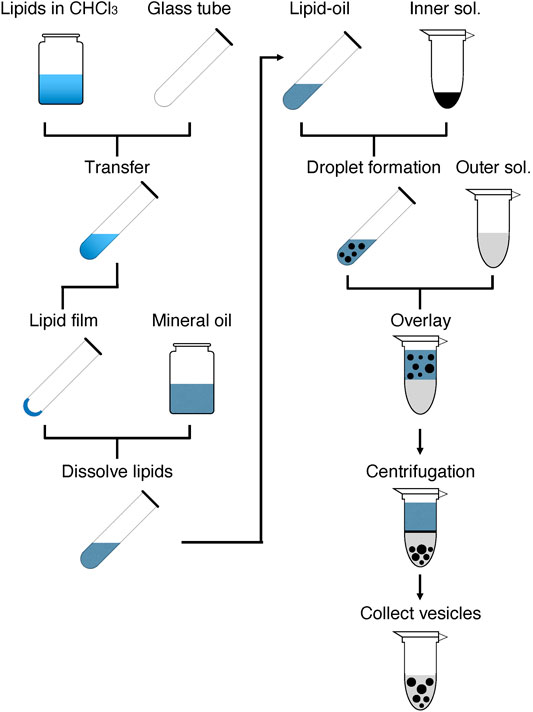
FIGURE 1. Overview of the rapid and facile preparation of giant vesicles. The left half shows the steps for the preparation of lipid-oil mixture, and the right half shows the steps for the formation of GVs. The details are described in the Stepwise Procedures.
Lipid Composition of GV Membranes
Although the type of lipids used for GV formation depends on the purpose of each experiment, 1-palmitoyl-2-oleoyl-sn-glycero-3-phosphocholine (POPC) or 1,2-dioleoyl-sn-glycero-3-phosphocholine (DOPC) is generally used as a basic lipid, because of its high stability. When preparing GV using several types of lipids, the lipids dissolved in an organic solvent such as chloroform at the defined concentration are mixed to obtain the desired composition. When synthesizing membrane proteins inside GVs, an acidic phospholipid such as phosphatidylglycerol (PG) or phosphatidylserine (PS) is added to the lipid composition—for example, 10–30 mol% acidic phospholipids are used—because the negative charge on the membrane surface is important for maintaining the correct structure of membrane protein and affects to its function (Pöyry and Vattulainen, 2016). The charge is also important for the localization of peripheral membrane proteins onto the membrane surface (Furusato et al., 2018). The use of 30 mol% cholesterol makes the membrane rigid (Gracià et al., 2010; Chakraborty et al., 2020). However, it should be noted that a certain amount of cholesterol inhibits the spontaneous membrane insertion when integral membrane proteins were synthesized inside GVs or outside of liposomes (Nakamura et al., 2018; Berhanu et al., 2019). Besides cholesterol, a physiological concentration of diacylglycerol also inhibits the spontaneous insertion (Nakamura et al., 2018). Fluorescent lipids or hydrophobic dyes, such as rhodamine-phosphatidylethanolamine (PE), NBD-PE, or Nile Red, are used for labeling GV membranes. Moreover, polyethylene glycol (PEG)-lipids (2–5 KDa in size) are used to avoid the adhesion of GVs. Chemically modified unnatural lipids are also used for experimental purposes (Kurihara et al., 2011; Bhattacharya et al., 2019). Conversely, natural lipids extracted from the membranes of various organisms, such as soybean or egg yolk, are not suitable for the formation of stable GV by this method, whereas they are applicable for the electroformation method (Méléard et al., 2009) or for preparation of small-size liposomes (<200 nm in diameter). Examples of lipid compositions reported in recent artificial cell studies are shown in Table 1.
Inner Solution
The inner solution corresponds to the internal aqueous phase of GVs. Regarding the inner solution, any component can be encapsulated in GVs, except hydrophobic molecules and detergents. For example, by adding purified proteins into the inner solution, various enzymatic reactions or structural formations in the GVs can be generated, e.g., polymerase chain reaction (Oberholzer et al., 1995), transcription (Altamura et al., 2021), actin polymerization (Lee et al., 2018; Litschel et al., 2021), Min oscillation (Litschel et al., 2018; Yoshida et al., 2019), etc. In more advanced applications, the encapsulation of a cell-free system reconstituted with purified multi-components involved in transcription and translation (Shimizu et al., 2001) or a cell extract from a certain organism such as Escherichia coli (Noireaux and Liu, 2020) allows the synthesis of desired proteins from the genes of interest. This technology has an advantage, particularly for the synthesis of membrane proteins (Furusato et al., 2018; Berhanu et al., 2019). In general, the purification of membrane proteins requires the use of a detergent that dissolves the cell membrane to isolate the proteins. The purified membrane protein sample contains a detergent, therefore it cannot be encapsulated inside GVs, although there are some exceptions (Yanagisawa et al., 2011; Altamura et al., 2017). To solve this problem, synthesizing membrane proteins inside GVs is a rational approach and allows the expression of biochemical functions on the GV membranes. This approach is depending on the phenomenon of spontaneous membrane insertion of nascent membrane proteins (Nishiyama et al., 2006). It should be noted that, when a certain amount of cholesterol or diacylglycerol is included in the composition of the GV membrane, this spontaneous insertion does not occur. The limitation of this approach is that not all membrane proteins can be integrated into the lipid membrane in a native form (Berhanu et al., 2019). For example, if a membrane protein has a large hydrophilic domain at the outside of the GV membrane (the opposite side of the translating ribosome), the spontaneous insertion with the correct membrane orientation of the protein does not occur.
In addition to purified proteins and cell-free systems, small-sized liposomes with a diameter of <200 nm can also be encapsulated, presenting the vesicle-in-vesicle structure (Lee et al., 2018; Berhanu et al., 2019; Altamura et al., 2021). This technique can mimic intracellular organelles. Interestingly, by coupling with a cell-free system that synthesizes a membrane protein, it is possible to localize the synthesized membrane proteins onto the liposome membrane (Berhanu et al., 2019). The membrane localization of the protein can be oriented using cholesterol (Nakamura et al., 2018), i.e., when cholesterol is used in the GV membrane but not in the liposome membrane, a large part of the synthesized membrane proteins are localized to the internal liposomes. The encapsulated liposome organelle is also useful for generating a proton gradient between the GV lumen and the liposome inside (Berhanu et al., 2019).
To mimic intracellular molecular crowding, Ficoll PM70 (Furusato et al., 2018; Berhanu et al., 2019) or bovine serum albumin (Fujiwara and Yanagisawa, 2014) is often encapsulated within GVs. This molecular crowding effect is essential for various types of molecular assembly and, especially, for the membrane localization of peripheral membrane proteins in association with a negative charge on the membrane surface.
In any case, the droplet transfer method generally uses sucrose in the inner solution to make it heavier than the glucose-containing outer solution, while maintaining equal osmotic pressure. In some experiments, sucrose may have a side effect on the internal reaction. In such a case, OptiPrep, a density gradient medium, is often used instead of sucrose (Van de Cauter et al., 2021).
An example of the inner solution used for the encapsulation of a commercial cell-free kit (PURE system) in GVs is shown in Table 2.
Outer Solution
The outer solution should be made when preparing the inner solution or in advance. The composition of the outer solution should be same or very similar to that of the inner solution, except reactive molecules and genes, i.e., enzymes and DNAs. The osmolality of the outer solution should be adjusted by changing the concentration of glucose, or alternative material, to be the same as the inner osmotic pressure. For the use of cell-free systems, the basic buffer composition and amino acids should be maintained, whereas a tRNA mixture and NTPs are removed. Note that the outer solution should be prepared from a double-concentrated solution, as the final solution can be diluted with the glucose solution (see an example in Table 3). The prepared outer solution can also be used for washing GVs after their formation.
Formation of GVs
The prepared W/O droplets are transferred to the aqueous phase by giving a force from the top to the bottom of the microtube by centrifugation (Figure 1). Although a large number of the droplets are broken when they pass through the interface, successful droplets form a lipid bilayer membrane, resulting in the generation of GVs. Usually, after centrifugation, white debris appears at the interface. This has to be removed entirely together with the oil phase. The formed GVs appear at the bottom of the tube because those are containing sucrose, which is heavier than glucose in the outer solution. A portion of the bottom fraction is collected carefully and transferred into a fresh tube for further experimentation. GVs can be washed with the same outer solution. Additional reagents can be supplied to the outer solution as needed while paying attention to the change in osmotic pressure. For example, ATP can be supplied to the exterior of the GVs at the same concentration as in the internal cell-free system, considering the possible leakage of ATP from the inside.
The Storable Artificial Cell Mixture
To make the component solutions of artificial cells portable and easy to store, the mixed inner cell-free reaction solution (without DNA) and the outer solution were lyophilized. These are used to make a ready-to-use kit together with the dried lipid film and mineral oil. This enabled us to generate artificial cells within 30 min starting from the preparation of the lipid-oil to the formation of artificial cells. The successful protein synthesis using the lyophilized and rehydrated cell-free components has been reported previously (Hunt et al., 2017; Lee et al., 2020; Yang et al., 2021). Therefore, it is possible to ship the samples at ambient temperature while avoiding moisture. This has a great advantage for working outside the laboratory or exchanging materials between laboratories.
Materials and Equipment
Materials
• Glass tube: diameter, 10 mm; length, 50 mm (Maruemu Corp., cat. #0407-03)
• Microtube, 1.5 ml (Eppendorf, 3810X)
• PCR tube, 0.2 ml (Nippon Genetics, Co., Ltd., cat. # FG-021D)
• Parafilm (Bemis™, PM996)
Reagents
• Chloroform (CHCl3) (Fujifilm, cat. #038-02606). CAUTION: the vapor and liquid forms of chloroform are toxic
• Mineral oil (MP Biomedicals, Inc., cat. #194836)
• 1-palmitoyl-2-oleoyl-glycero-3-phosphocholine (sodium salt), POPC (Avanti Polar, cat # 850457P)
• 1-palmitoyl-2-oleoyl-sn-glycero-3-phospho-(1′-rac-glycerol) (sodium salt), POPG (Avanti Polar, cat # 840457P)
• Sucrose (Fujifilm, cat. # 196-00015)
• d(+)-Glucose (Fujifilm, cat. # 049-31165)
• Cell-free system, e.g., PUREfrex2.0 (GeneFrontier, cat. # PF201-0.25-5-EX)
• Genes of interest (under the control of the T7 promoter and ribosome-binding site)
• (Optional) Ficoll PM70 (Sigma-Aldrich, cat. #F2878)
Reagent Preparation
• Phospholipids (e.g., POPC or POPG) are dissolved in CHCl3 at 100 mM in a glass vial and briefly processed with bath sonication.
• Sucrose and glucose solutions are prepared at 2 M with MilliQ water.
• Sol. I (Buffer) of PUREfrex2.0 is preheated at 37°C for 5 min before mixing and placed on ice until use, as per the manufacturer’s guidelines.
• Sol. II (enzymes) and III (ribosome) of PUREfrex2.0 are placed on ice until use, as per the manufacturer’s guidelines.
• Genes of interest are used as plasmids or linear DNAs.
Equipment
• Aluminum block heating bath (e.g., TITEC Corp., cat. #DTU-2BN)
• Vortex (e.g., Scientific Industries, Inc., cat. #SI-T236)
• Nitrogen (N2) gas
• Centrifuge (e.g., TOMY Digital Biology, cat. #MX-307)
• Ultrasonic bath (e.g., Elma Schmidbauer GmbH, cat #S15H)
• Inverted fluorescence microscope (e.g., Olympus Life Science IX73) or confocal microscopy system (e.g., Nikon AIR)
Anticipated Results
GVs encapsulating a cell-free system (PURE system) and DNA of gfp were prepared by following the protocol described herein (Figure 2), using the outer solution described in Table 3. The resulting GVs were incubated at 37°C for 1–3 h to perform protein synthesis inside. Before incubation, 20 ng/μL of RNaseA were added to the exterior of the GVs to eliminate possible protein synthesis outside the GVs. ATP was also added to the outside of the GVs to prevent the leakage of the encapsulated ATP. The reacted GVs were observed by a confocal microscope equipped with a differential interference contrast unit. Using a 488 nm laser, we observed the synthesized green fluorescent protein (GFP) within the GVs consist of 50 mol% POPC and 50 mol% POPG (Figure 3A).
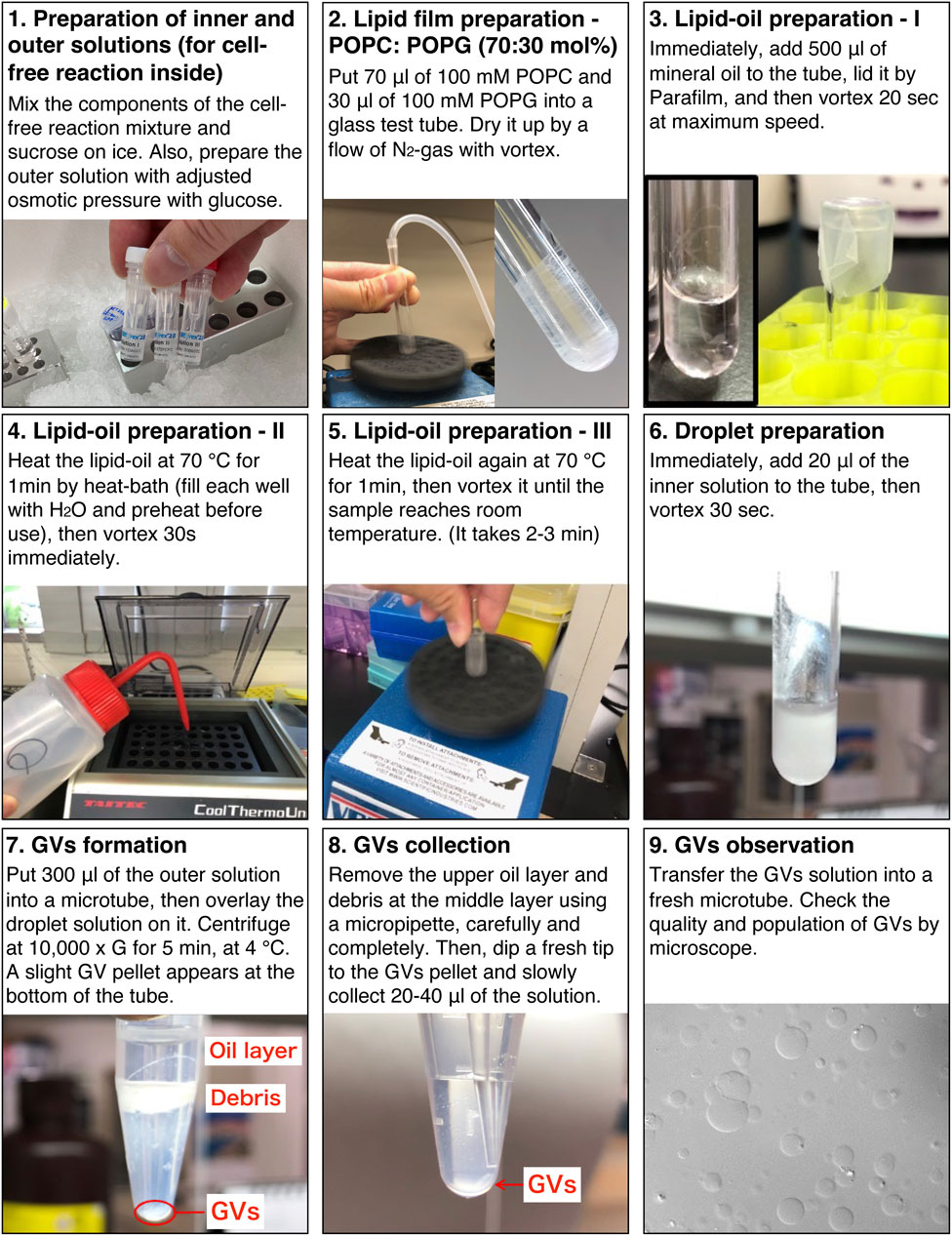
FIGURE 2. Stepwise protocol for the preparation of artificial cells. The details of the inner and outer solutions are provided in Tables 2, 3, respectively.
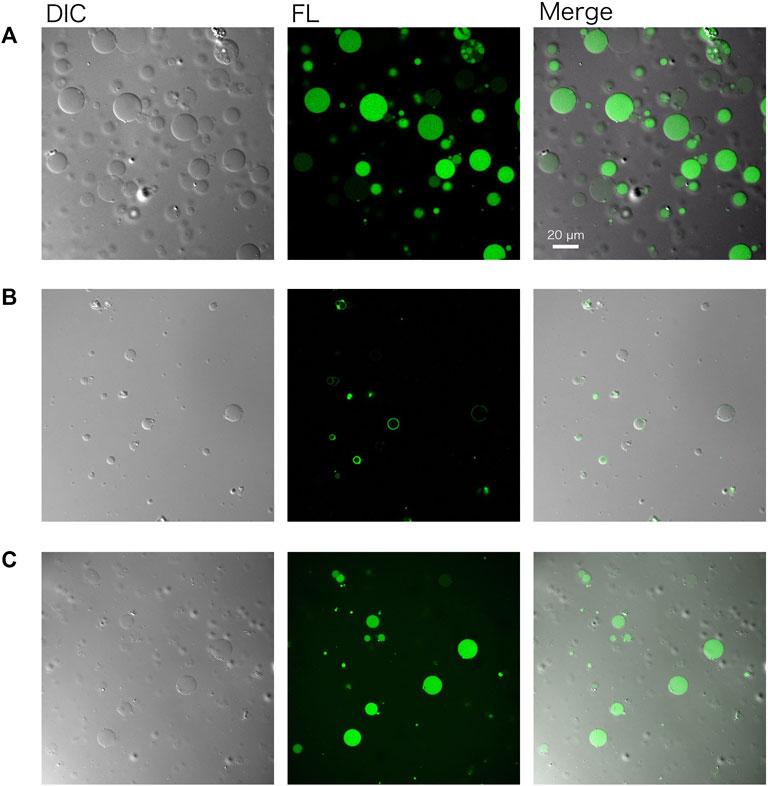
FIGURE 3. Confocal microscopy images of artificial cells. (A) Green fluorescent protein (GFP) and (B) a fusion protein of PlsY-GFP were synthesized in GVs. (C) GFP was synthesized using the lyophilized and rehydrated PURE system inside GVs. In any case, the GV membrane was composed of POPC: POPG (50:50 mol%). Images were obtained using a Nikon confocal microscopy A1R system. DIC: differential interference contrast, FL: fluorescence image.
A membrane protein was also synthesized using the same method by simply replacing the DNA. PlsY (glycerol-3-phosphate acyltransferase) is an integral membrane protein and was synthesized inside GVs. The gene encoding PlsY was fused to the GFP gene at the C terminus of the plsY DNA to visualize the product by microscopy. Note that modification of the N terminus of membrane proteins should be avoided because it may affect the co-translational spontaneous membrane localization of the proteins. Figure 3B shows successful protein synthesis in GVs and their localization onto the GV membrane.
Protein synthesis inside GVs was again performed using the lyophilized and rehydrated PURE system. All of the components of the reaction mixture including sucrose were mixed and lyophilized. After the rehydration of the PURE system, DNA encoding GFP was added and encapsulated inside GVs. The observed result was the same as that observed using the normal PURE system (Figure 3C).
Discussion
Although the construction of artificial cells will provide a deepened understanding of the life system of cells, technical restrictions are preventing the development of this research field. Many protocols for GV formation have been published to date, but, in many cases, those are optimized within individual laboratories in detail and often contain unpublished tips and knowledge. This fact sometimes impedes the reproducibility of the results when other researchers work for GV formation by following the method reported in the article.
According to one of the standard methods, a mixture of lipid and oil is prepared by adding the lipids dissolved in chloroform directly to the mineral oil and evaporating the chloroform by heating and stirring (Fujii et al., 2014; Uyeda et al., 2021). However, in this method, we found that a certain amount of chloroform remains in the oil solution even after evaporating the solvent for 90 min at 60°C with stirring (Figure 4). Although the effect of the residual solvent on the cell-free system might be negligible, there is a possibility that the reproducibility of the obtained GV quality may not be stable depending on the degree of residual solvent. Our proposed method can circumvent this risk, as the solvent is evaporated before mixing with the oil. As the other possibility, moisture in the oil or oxidation of the lipids may reduce the quality of the formation of GVs, as suggested by Robinson’s group (Moga et al., 2019) and Dekker’s group (Van de Cauter et al., 2021). To avoid these problems, the dried lipid film should be stored in a desiccator with low pressure under a stable temperature.
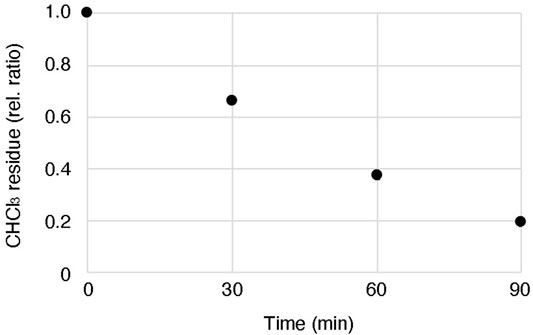
FIGURE 4. Residual chloroform in oil after heating and stirring. 200 µL chloroform containing 100 mM lipid (POPC 50 mol%: POPG 50 mol%) was added into 1 ml mineral oil, and then the lipid-oil mixture was heated at 60°C with stirring. The weight of the mixture was measured every 30 min until 90 min. The data were based on three independent replicates of the experiments.
The use of lyophilized cell-free mixture and dried lipid films not only enables further reduction of the preparation time but also has the potential to expand the versatility of artificial cell technology. This is extremely useful for working outside the laboratory and for shipping samples. Moreover, it may be applied as a ready-to-use biosensor kit for the detection of DNA sequences derived from specific viruses without the use of special equipment. For example, by combining with a signal amplification system (Sato et al., 2019), the presence of pathogenic viruses in an environment or biological samples can be detected outside the laboratory. We believe our method will be the basis for the development of artificial cell engineering.
Data Availability Statement
The raw data supporting the conclusions of this article will be made available by the authors, without undue reservation.
Author Contributions
Experiments were designed by YS and YK. Data were generated by YS and analyzed by YS and YK. The manuscript was written by YK.
Funding
This work was supported by the Human Frontier Science Program (RPG0029/2020 to YK), JST PRESTO (JPMJPR18K5 to YK), JSPS KAKENHI (16H06156, 16KK0161, 16H00797, 21H05156 to YK).
Conflict of Interest
The authors declare that the research was conducted in the absence of any commercial or financial relationships that could be construed as a potential conflict of interest.
Publisher’s Note
All claims expressed in this article are solely those of the authors and do not necessarily represent those of their affiliated organizations, or those of the publisher, the editors, and the reviewers. Any product that may be evaluated in this article, or claim that may be made by its manufacturer, is not guaranteed or endorsed by the publisher.
Acknowledgments
We thank Rumie Matsumura (JAMSTEC) and Yuuki Haruyama (Masason Foundation) for assisting the experiments, and Dr. Takashi Kanamori (GeneFrontier) for valuable discussion.
References
Altamura, E., Albanese, P., Marotta, R., Milano, F., Fiore, M., Trotta, M., et al. (2021). Chromatophores Efficiently Promote Light-Driven ATP Synthesis and DNA Transcription inside Hybrid Multicompartment Artificial Cells. Proc. Natl. Acad. Sci. USA 118 (7), e2012170118. doi:10.1073/pnas.2012170118
Altamura, E., Milano, F., Tangorra, R. R., Trotta, M., Omar, O. H., Stano, P., et al. (2017). Highly Oriented Photosynthetic Reaction Centers Generate a Proton Gradient in Synthetic Protocells. Proc. Natl. Acad. Sci. USA 114(15), 3837–3842. doi:10.1073/pnas.1617593114
Angelova, M. I., and Dimitrov, D. S. (1986). Liposome Electroformation. Faraday Discuss. Chem. Soc. 81 (0), 303–311. doi:10.1039/DC9868100303
Bashirzadeh, Y., Wubshet, N., Litschel, T., Schwille, P., and Liu, A. P. (2021). Rapid Encapsulation of Reconstituted Cytoskeleton inside Giant Unilamellar Vesicles. JoVE 177. doi:10.3791/63332
Berhanu, S., Ueda, T., and Kuruma, Y. (2019). Artificial Photosynthetic Cell Producing Energy for Protein Synthesis. Nat. Commun. 10 (1), 1325. doi:10.1038/s41467-019-09147-4
Bhattacharya, A., Brea, R. J., Niederholtmeyer, H., and Devaraj, N. K. (2019). A Minimal Biochemical Route towards De Novo Formation of Synthetic Phospholipid Membranes. Nat. Commun. 10 (1), 300. doi:10.1038/s41467-018-08174-x
Blanken, D., Foschepoth, D., Serrão, A. C., and Danelon, C. (2020). Genetically Controlled Membrane Synthesis in Liposomes. Nat. Commun. 11 (1), 4317. doi:10.1038/s41467-020-17863-5
Chakraborty, S., Doktorova, M., Molugu, T. R., Heberle, F. A., Scott, H. L., Dzikovski, B., et al. (2020). How Cholesterol Stiffens Unsaturated Lipid Membranes. Proc. Natl. Acad. Sci. USA 117 (36), 21896–21905. doi:10.1073/pnas.2004807117
Fujii, S., Matsuura, T., Sunami, T., Nishikawa, T., Kazuta, Y., and Yomo, T. (2014). Liposome Display for In Vitro Selection and Evolution of Membrane Proteins. Nat. Protoc. 9 (7), 1578–1591. doi:10.1038/nprot.2014.107
Fujiwara, K., and Yanagisawa, M. (2014). Generation of Giant Unilamellar Liposomes Containing Biomacromolecules at Physiological Intracellular Concentrations Using Hypertonic Conditions. ACS Synth. Biol. 3 (12), 870–874. doi:10.1021/sb4001917
Furusato, T., Horie, F., Matsubayashi, H. T., Amikura, K., Kuruma, Y., and Ueda, T. (2018). De Novo Synthesis of Basal Bacterial Cell Division Proteins FtsZ, FtsA, and ZipA inside Giant Vesicles. ACS Synth. Biol. 7 (4), 953–961. doi:10.1021/acssynbio.7b00350
Gracià, R. S., Bezlyepkina, N., Knorr, R. L., Lipowsky, R., and Dimova, R. (2010). Effect of Cholesterol on the Rigidity of Saturated and Unsaturated Membranes: Fluctuation and Electrodeformation Analysis of Giant Vesicles. Soft Matter 6 (7), 1472–1482. doi:10.1039/B920629A
Hunt, J. P., Yang, S. O., Wilding, K. M., and Bundy, B. C. (2017). The Growing Impact of Lyophilized Cell-free Protein Expression Systems. Bioengineered 8 (4), 325–330. doi:10.1080/21655979.2016.1241925
Jimbo, T., Sakuma, Y., Urakami, N., Ziherl, P., and Imai, M. (2016). Role of Inverse-Cone-Shape Lipids in Temperature-Controlled Self-Reproduction of Binary Vesicles. Biophysical J. 110 (7), 1551–1562. doi:10.1016/j.bpj.2016.02.028
Kamiya, K. (2020). Development of Artificial Cell Models Using Microfluidic Technology and Synthetic Biology. Micromachines 11 (6), 559. doi:10.3390/mi11060559
Kurihara, K., Tamura, M., Shohda, K.-i., Toyota, T., Suzuki, K., and Sugawara, T. (2011). Self-reproduction of Supramolecular Giant Vesicles Combined with the Amplification of Encapsulated DNA. Nat. Chem 3 (10), 775–781. doi:10.1038/nchem.1127
Lee, K. Y., Park, S.-J., Lee, K. A., Kim, S.-H., Kim, H., Meroz, Y., et al. (2018). Photosynthetic Artificial Organelles Sustain and Control ATP-dependent Reactions in a Protocellular System. Nat. Biotechnol. 36 (6), 530–535. doi:10.1038/nbt.4140
Lee, M. S., Raig, R. M., Gupta, M. K., and Lux, M. W. (2020). Lyophilized Cell-free Systems Display Tolerance to Organic Solvent Exposure. ACS Synth. Biol. 9 (8), 1951–1957. doi:10.1021/acssynbio.0c00267
Litschel, T., Kelley, C. F., Holz, D., Adeli Koudehi, M., Vogel, S. K., Burbaum, L., et al. (2021). Reconstitution of Contractile Actomyosin Rings in Vesicles. Nat. Commun. 12 (1), 2254. doi:10.1038/s41467-021-22422-7
Litschel, T., Ramm, B., Maas, R., Heymann, M., and Schwille, P. (2018). Beating Vesicles: Encapsulated Protein Oscillations Cause Dynamic Membrane Deformations. Angew. Chem. Int. Ed. 57 (50), 16286–16290. doi:10.1002/anie.201808750
Lowe, L. A., Loo, D. W. K., and Wang, A. (2022). Methods for Forming Giant Unilamellar Fatty Acid Vesicles. Methods Mol. Biol. 2402, 1–12. doi:10.1007/978-1-0716-1843-1_1
Lu, Y., Allegri, G., and Huskens, J. (2021). Vesicle-based Artificial Cells: Materials, Construction Methods and Applications. Mater. Horiz. 9, 892–907. doi:10.1039/d1mh01431e
Méléard, P., Bagatolli, L. A., and Pott, T. (2009). Giant Unilamellar Vesicle Electroformation from Lipid Mixtures to Native Membranes under Physiological Conditions. Methods Enzymol. 465, 161–176. doi:10.1016/s0076-6879(09)65009-6
Moga, A., Yandrapalli, N., Dimova, R., and Robinson, T. (2019). Optimization of the Inverted Emulsion Method for High‐Yield Production of Biomimetic Giant Unilamellar Vesicles. Chembiochem 20 (20), 2674–2682. doi:10.1002/cbic.201900529
Morita, M., Katoh, K., and Noda, N. (2018). Direct Observation of Bacterial Growth in Giant Unilamellar Vesicles: A Novel Tool for Bacterial Cultures. ChemistryOpen 7 (11), 845–849. doi:10.1002/open.201800126
Nakamura, S., Suzuki, S., Saito, H., and Nishiyama, K.-i. (2018). Cholesterol Blocks Spontaneous Insertion of Membrane Proteins into Liposomes of Phosphatidylcholine. J. Biochem. 163 (4), 313–319. doi:10.1093/jb/mvx083
Nishiyama, K.-i., Ikegami, A., Moser, M., Schiltz, E., Tokuda, H., and Müller, M. (2006). A Derivative of Lipid A Is Involved in Signal Recognition particle/SecYEG-dependent and -independent Membrane Integrations. J. Biol. Chem. 281 (47), 35667–35676. doi:10.1074/jbc.M608228200
Noireaux, V., and Libchaber, A. (2004). A Vesicle Bioreactor as a Step toward an Artificial Cell Assembly. Proc. Natl. Acad. Sci. U.S.A. 101 (51), 17669–17674. doi:10.1073/pnas.0408236101
Noireaux, V., and Liu, A. P. (2020). The New Age of Cell-free Biology. Annu. Rev. Biomed. Eng. 22, 51–77. doi:10.1146/annurev-bioeng-092019-111110
Oberholzer, T., Albrizio, M., and Luisi, P. L. (1995). Polymerase Chain Reaction in Liposomes. Chem. Biol. 2 (10), 677–682. doi:10.1016/1074-5521(95)90031-4
Oda, A., Watanabe, C., Aoki, N., and Yanagisawa, M. (2020). Liposomal Adhesion via Electrostatic Interactions and Osmotic Deflation Increase Membrane Tension and Lipid Diffusion Coefficient. Soft Matter 16 (18), 4549–4554. doi:10.1039/d0sm00416b
Pautot, S., Frisken, B. J., and Weitz, D. A. (2003). Engineering Asymmetric Vesicles. Proc. Natl. Acad. Sci. 100(19), 10718–10721. doi:10.1073/pnas.1931005100
Pöyry, S., and Vattulainen, I. (2016). Role of Charged Lipids in Membrane Structures - Insight Given by Simulations. Biochim. Biophys. Acta (Bba) - Biomembranes 1858 (10), 2322–2333. doi:10.1016/j.bbamem.2016.03.016
Reeves, J. P., and Dowben, R. M. (1969). Formation and Properties of Thin-Walled Phospholipid Vesicles. J. Cell. Physiol. 73 (1), 49–60. doi:10.1002/jcp.1040730108
Robinson, T. (2019). Microfluidic Handling and Analysis of Giant Vesicles for Use as Artificial Cells: A Review. Adv. Biosys. 3 (6), 1800318. doi:10.1002/adbi.201800318
Sato, Y., Komiya, K., Kawamata, I., Murata, S., and Nomura, S.-i. M. (2019). Isothermal Amplification of Specific DNA Molecules inside Giant Unilamellar Vesicles. Chem. Commun. 55 (62), 9084–9087. doi:10.1039/C9CC03277K
Shimizu, Y., Inoue, A., Tomari, Y., Suzuki, T., Yokogawa, T., Nishikawa, K., et al. (2001). Cell-free Translation Reconstituted with Purified Components. Nat. Biotechnol. 19 (8), 751–755. doi:10.1038/90802
Toparlak, Ö. D., Zasso, J., Bridi, S., Serra, M. D., Macchi, P., Conti, L., et al. (2020). Artificial Cells Drive Neural Differentiation. Sci. Adv. 6(38), eabb4920. doi:10.1126/sciadv.abb4920
Tsumoto, K., Matsuo, H., Tomita, M., and Yoshimura, T. (2009). Efficient Formation of Giant Liposomes through the Gentle Hydration of Phosphatidylcholine Films Doped with Sugar. Colloids Surf. B: Biointerfaces 68 (1), 98–105. doi:10.1016/j.colsurfb.2008.09.023
Uyeda, A., Reyes, S. G., Kanamori, T., and Matsuura, T. (2022). Identification of Conditions for Efficient Cell-Sized Liposome Preparation Using Commercially Available Reconstituted In Vitro Transcription-Translation System. J. Biosci. Bioeng. 133 (2), 181–186. doi:10.1016/j.jbiosc.2021.10.008
Van de Cauter, L., Fanalista, F., van Buren, L., De Franceschi, N., Godino, E., Bouw, S., et al. (2021). Optimized cDICE for Efficient Reconstitution of Biological Systems in Giant Unilamellar Vesicles. ACS Synth. Biol. 10 (7), 1690–1702. doi:10.1021/acssynbio.1c00068
Walde, P., Cosentino, K., Engel, H., and Stano, P. (2010). Giant Vesicles: Preparations and Applications. Chem. Eur. J. Chem. Bio. 11 (7), 848–865. doi:10.1002/cbic.201000010
Yanagisawa, M., Iwamoto, M., Kato, A., Yoshikawa, K., and Oiki, S. (2011). Oriented Reconstitution of a Membrane Protein in a Giant Unilamellar Vesicle: Experimental Verification with the Potassium Channel KcsA. J. Am. Chem. Soc. 133 (30), 11774–11779. doi:10.1021/ja2040859
Yang, J., Cui, Y., Cao, Z., Ma, S., and Lu, Y. (2021). Strategy Exploration for Developing Robust Lyophilized Cell-free Systems. Biotechnol. Notes 2, 44–50. doi:10.1016/j.biotno.2021.08.004
Keywords: giant vesicle, artificial cells, protocells, droplet transfer, cell-free synthetic biology, cell-free protein synthesis, liposome, lyophilization
Citation: Shimane Y and Kuruma Y (2022) Rapid and Facile Preparation of Giant Vesicles by the Droplet Transfer Method for Artificial Cell Construction. Front. Bioeng. Biotechnol. 10:873854. doi: 10.3389/fbioe.2022.873854
Received: 11 February 2022; Accepted: 03 March 2022;
Published: 07 April 2022.
Edited by:
Jian Li, ShanghaiTech University, ChinaReviewed by:
Allen Liu, University of Michigan, United StatesEmiliano Altamura, University of Bari Aldo Moro, Italy
Copyright © 2022 Shimane and Kuruma. This is an open-access article distributed under the terms of the Creative Commons Attribution License (CC BY). The use, distribution or reproduction in other forums is permitted, provided the original author(s) and the copyright owner(s) are credited and that the original publication in this journal is cited, in accordance with accepted academic practice. No use, distribution or reproduction is permitted which does not comply with these terms.
*Correspondence: Yutetsu Kuruma, ykuruma@jamstec.go.jp