- 1David H. Koch Institute for Integrative Cancer Research, Massachusetts Institute of Technology, Cambridge, MA, United States
- 2Center for Precision Cancer Medicine, Massachusetts Institute of Technology, Cambridge, MA, United States
- 3Coulter Department of Biomedical Engineering, Georgia Institute of Technology and Emory University, Atlanta, GA, United States
- 4Department of Pediatrics, Emory School of Medicine, Atlanta, GA, United States
- 5Aflac Cancer and Blood Disorders Center, Children’s Healthcare of Atlanta, Atlanta, GA, United States
- 6Winship Cancer Institute of Emory University, Atlanta, GA, United States
- 7Petit Institute for Bioengineering and Bioscience, Georgia Institute of Technology, Atlanta, GA, United States
Introduction
Polymer-drug conjugation (Harris, 1992; Harris and Chess, 2003; Haag and Kratz, 2006; Pelegri-O’Day et al., 2014; Hoffman, 2016; Ekladious et al., 2019) was first described in the 1954 by German chemist, Horst Jatzkewitz, who demonstrated that covalent attachment of poly (vinyl pyrrolidone) to the psychoactive compound, mescaline, could be used to prolong its circulation and duration of action (Figure 1A) (Jatzkewitz, 1954; Jatzkewitz, 1955; Luxenhofer, 2020). Yet despite its novelty and utility, Jatzkewitz’s innovation went largely unnoticed until the mid 1970s when it was revived by Ringsdorf, Kopecek, and Duncan, among others, who championed the notion that these novel macromolecules could enhance the suboptimal activity of various pharmaceuticals (Ringsdorf, 1975). It wouldn’t be until 1990—nearly 36 years from the publication of Jatzkewitz’s initial work—that the first polymer-drug conjugate would receive market approval in the form of Adagen, adenosine deaminase protein conjugated with 5 kDa poly (ethylene glycol), or PEG, used to treat a rare and hereditary, pediatric metabolic disorder called adenosine deaminase severe combined immunodeficiency (Hershfield et al., 1987).
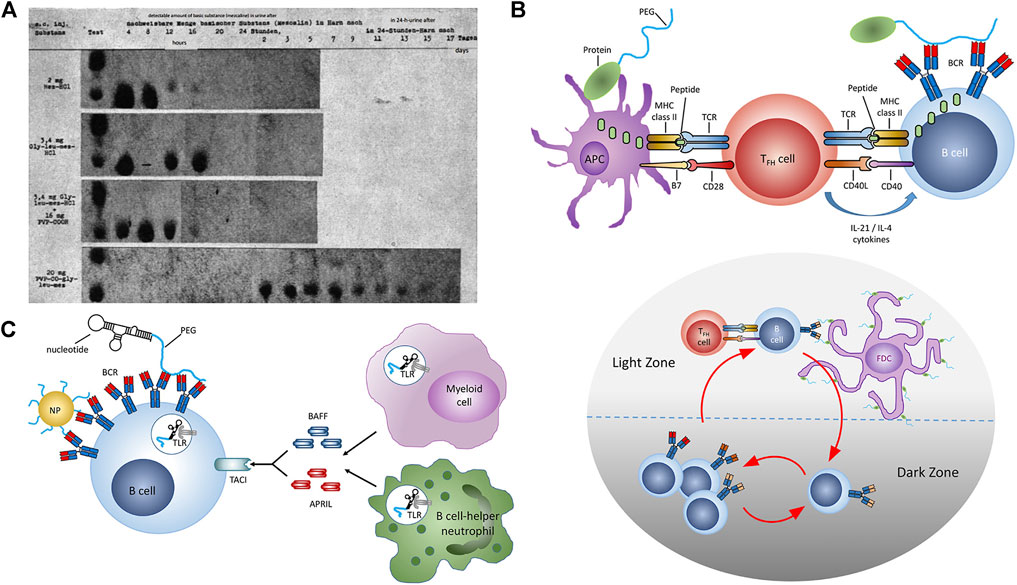
FIGURE 1. Polymer-Drug Conjugates: Inception to Immunology. (A) Renal excretion of mescaline and equimolar dosages of mescaline-PVP conjugate as measured by chromatography of urinary extracts obtained following s.c. adminisration in white mice circa 1955. (B) Thymus-dependent immune response against PEG. (upper panel) TFH activation following antigen presentation by APCs. Somatic hypermutation and class switching in B cells following antigen encounter and interaction with activated TFH cells. (lower panel) Affinity maturation of PEG-specific B cells in the spleen. (C) Thymus-independent immune response against PEG. Crosslinking of BCRs by PEG and coactivation of TLRs. Reproduced with permission from Reproduced with permission from (A) Luxenhofer, 2020 and (B,C) Chen et al., 2021. Copyright (A) 2020 de Gruyter GmbH and (B,C) 2021 American Chemical Society.
Polymer-drug conjugates have since gradually increased in their clinical application, now with more than 29 marketed products that vary widely in polymer architecture (linear and branched), molecular weight (0.3–60 kDa per polymer), and degree of conjugation (1–69-82 per drug) and nearly all of which employ the synthetic polymer, PEG, a polyether typically produced by the ring-opening polymerization of ethylene glycol (Alconcel et al., 2011; Ekladious et al., 2019; Xu et al., 2022). In addition to the diversity of their appended polymers, these therapeutics also vary widely in their drug partner, ranging from: 1) peptides (e.g. Somavert HGH receptor antagonist) to 2) small molecules (e.g. SMANCS neocarzinostatin chemotherapy and Movanik naloxone laxative) and 3) nucleic acids (e.g. Macugen anti-VEGF aptamer antiangiogenic) (Perdue et al., 2020). More recently, polymer conjugation has demonstrated further clinical utility in stabilizing lipid nanoparticles used to deliver small interfering RNA (siRNA, Onpattro) for the treatment of hereditary transthyretin-mediated (hATTR) amyloidosis (Zhang et al., 2020), as well as both current mRNA-based vaccines for SARS-CoV-2 (COVID-19), BNT162b2/Comirnaty and mRNA-1273/Spikevax (Schoenmaker et al., 2021). Interestingly, all three nanoparticle formulations share in their use of lipids tethered with 2 kDa linear, methoxy-terminal PEG (mPEG). While Phase III clinical trials for both mRNA vaccines demonstrated overwhelming safety and efficacy (e.g. 4.7 and 2.8 anaphylactic reactions cases per million registered during the first months both vaccination campaigns, respectively (CDC COVID-19 Response Team and Food and Drug Administration, 2021)), their widespread use has led to concerns from some that pre-existing anti-PEG antibodies may induce hypersensitivity reactions (de Vrieze, 2021) or that drug-induced PEG immunity may impact the efficacy or safety of subsequently administered PEGylated drugs or vaccines.
PEG’s remarkable hydrophilicity, flexibility, inertness, and relative biocompatibility have found the polymer numerous uses beyond modulating drug circulation or activity and today it can be found near ubiquitously in both consumer products such as detergents, cosmetics, and car wax, as well as in industrial applications including electroplating, historical artifact preservation, and molded product production (Harris, 1992; Prime and Whitesides, 1993; Harris and Chess, 2003; Li et al., 2005; Jokerst et al., 2011). PEGylating has also been used to improve stability of contrast agents for in vivo fluorescence imaging, photodynamic therapy, and sonodynamic therapy (Ding et al., 2018; Chen et al., 2021; Xu et al., 2022). Given PEG’s near exclusive utilization in polymer-drug conjugates, our rapidly increasing consumer use of the compound, and recent, prevalent, and systemic exposure to PEG in the form of mRNA vaccines and boosters for SARS-CoV-2 (currently >0.5 bn doses (C ovid-data-tracker, 2022) in the United States) (Polack et al., 2020; Baden et al., 2021), several obvious questions arise with relevance to both public awareness and public health: Is PEG immunogenetic? Does prior environmental exposure or PEG-drug conjugate therapy impact immune responses to PEG? Will PEG immunogenicity affect future vaccine efficacy? How can we minimize and hedge-against PEG immunogenicity in future polymer-drug formulations?
Immunity Towards PEG Is Pre-existing and Drug Exposure-inducible
PEG was classified as a GRAS (Generally Recognized as Safe) food ingredient by the FDA in 1973 and has a long history of safe use in humans. It is the most widely used stealth polymer in drug delivery and is typically regarded as a non-immunogenic polymer. Early studies by Richter and Akerblom in 1984 found that 0.2% of treatment-naïve individuals (individuals who have never received PEGylated biopharmaceuticals), had antibodies specific to PEG in their plasma (Richter and Åkerblom, 1984). Since then, the presence of pre-existing anti-PEG antibodies has been reported to range from 4.5 to 43.1% in treatment-naïve donors (Shpetner and Vallee, 1991; Garratty, 2004; Chen et al., 2016; Lubich et al., 2016; Yang et al., 2016), leading to the hypothesis that the frequency of pre-existing anti-PEG antibodies is increasing over time (Yang et al., 2016). Recent analysis of 79 historical (samples collected from the 1970s–1990s) and 377 contemporary human serum samples, indicate the presence of anti-PEG antibodies (IgG and IgM) in approximately 56 and 72% of samples respectively (Yang et al., 2016) with no significant difference in the measured concentrations of anti-PEG IgG and IgM, strongly suggesting that an apparent increase in pre-existing anti-PEG antibodies with time may in fact be a consequence of increased sensitivity in anti-PEG immunoassays developed in recent years (Yang et al., 2016; Chen et al., 2021). For example, direct binding assays using beads or ELISA plates are generally more sensitive compared to traditional bridging assays. Although these studies found that the prevalence of pre-existing anti-PEG antibodies was higher than was previously appreciated, the absolute concentrations of anti-PEG remain low in most positive individuals (Chen et al., 2021) and, as discussed later, drugs administered at different levels may be differentially impacted by pre-existing PEG immunity.
In addition to treatment-naïve immunity, anti-PEG antibodies are also drug-inducible and associated with systemic administration of PEGylated proteins (Chen et al., 2021), nucleic acids, liposomes, and nanoparticles (Judge and MacLachlan, 2008; Mima et al., 2015; Avci-Adali et al., 2013; Ishida et al., 2006a; Ishida et al., 2006b; Ishida et al., 2006c; Kozma et al., 2019). Drug-induced anti-PEG antibody responses occur via two principal mechanisms: T cell-dependent (TD) and T cell-independent (TI) pathways (Figures 1B,C). TD is typically associated with PEGylated proteins and peptides (Mima et al., 2015; Elsadek et al., 2020), while TI has been associated with systemic exposure to PEGylated nanoparticles (Freire Haddad et al., 2022). Anti-PEG antibodies induced by TD occur when peptides are presented by B cells to helper T cells, and is characterized by an initial peak of IgM, followed by class switching, and a larger peak in IgG (Freire Haddad et al., 2022). TI occurs when the antigen crosslinks receptors on IgM memory B cells and is characterized by high concentrations of IgM and low concentrations of IgG. Antibodies produced via the TI pathway have a weaker affinity for PEG compared to TD (Freire Haddad et al., 2022). While the basic underpinnings of anti-PEG immunity such as these are clear, 1) our understanding of how these processes vary with health or disease status, age, sex, or ethnicity and 2) our ability to predict the magnitude and functional impact of these responses on patients collectively remain unclear.
PEG Immunity can Induce Hypersensitivity Reactions and Alter Drug Transport/Efficacy but these Effects Vary Across Formulation Type and Mode of Administration
Hypersensitivity reactions, including anaphylaxis has been reported in association with many PEG-containing formulations including PEG-protein conjugates (pegloticase (Lipsky et al., 2014), pegvaliase (Gupta et al., 2018), pegaspargase (Hasan et al., 2017; Browne et al., 2018; Liu et al., 2019), pegcrisantaspase (Rau et al., 2018)), PEG excipients (polysorbate 80 (Pérez-Pérez et al., 2011)), contrast agents (SonoVue (de Groot et al., 2004; Geleijnse et al., 2009)), liposomes encapsulating oligonucleotides or plasmid DNA (Semple et al., 2005; Judge et al., 2006), and liposomal doxorubicin (Chanan-Khan et al., 2003; Szebeni, 2014). Pre-existing PEG antibodies, in contrast, have been implicated in hypersensitivity reactions to PEGylated medicines including pegaspargase (Liu et al., 2019) and the RNA aptamer, pegnivacogin (Povsic et al., 2013). Acute severe allergic reactions to pegnivacogin were observed only in patients with pre-existing anti-PEG antibodies, and the level of anti-PEG IgG antibodies correlated with adverse event severity (Povsic et al., 2016). In addition, 2 of 25 phenylketonuria patients treated with pegvaliase developed anaphylactic and hypersensitivity reactions to a PEGylated contraceptive (Longo et al., 2014) and 3 patients who developed allergies to pegaspargase also experienced hypersensitivity reactions when treated with pegcrisantaspase (Rau et al., 2018), indicating that anti-PEG antibodies induced by one PEGylated medicines can cross-react to other subsequently administered PEGylated medicines. The mechanism(s) by which anti-PEG antibodies induce hypersensitivity reactions is poorly understood; however, some possible mechanisms by which pegylated nanoparticles and pegylated nucleotides could induce hypersensitivity reactions include: 1) complement activation-related pseudoallergy (CARPA) (Szebeni et al., 2011; Dézsi et al., 2014; Mohamed et al., 2019), whereby anti-PEG antibodies bound to PEG on a nanoparticle or liposome surface can activate the complement cascade, liberating the anaphylatoxins C3a and C5a (Neun et al., 2018; Mohamed et al., 2019; Chen et al., 2020) and 2) Fc receptor activation of innate immune cells either by anti-PEG IgE antibodies (Shah et al., 2013; Stone et al., 2019; Zhou et al., 2021) or allergen-specific IgG that binds to Fc gamma receptors (FcγRs) expressed on platelets, macrophages, basophils, or neutrophils to release various mediators such as platelet-activating factor (PAF), cysteinyl leukotrienes (CysLTs), histamine, and serotonin (Finkelman, 2007; Reber et al., 2017; Beutier et al., 2018).
Accelerated blood clearance (ABC) of PEGylated compounds was identified in mice in 1999, and in patients treated with pegaspargase in 2007 (Cheng et al., 1999; Cheng et al., 2000; Armstrong et al., 2007) and is caused by an immune reaction associated with repeat exposure to PEG. The first injection of PEGylated drugs induces anti-PEG antibodies, which then bind and form an immune complex with the second dose of the PEGylated compound to activate the complement system. This results in the opsonization of PEG with C3 fragments and enhanced uptake by Kupffer cells in the liver and can result in altered drug pharmacokinetics and biodistribution (PK, BD) and reduced drug efficacy in subsequent doses (Dams et al., 2000; Ishida et al., 2006a; Ishida et al., 2008; Ishida and Kiwada, 2008; Hashimoto et al., 2014). Rapid drug clearance and loss of drug efficacy have been reported following treatment with PEG-uricase, pegvaliase (Gupta et al., 2018), PEGylated liposomes (Dams et al., 2000; Laverman et al., 2001; Ishida et al., 2003), and PEGylated liposomal doxorubicin. ABC has also been observed in animal models treated with empty PEGylated liposomes (Dams et al., 2000; Semple et al., 2005; Ishida et al., 2006a; Ishida et al., 2006b), poly(lactic acid) (PLA) nanoparticles, microbubbles, and lipoplexes (Ishihara et al., 2009; Fix et al., 2018). In addition, anti-PEG antibodies can hinder the distribution of PEGylated nanoparticles to target tissues. For example, N-linked glycans present on anti-PEG antibodies bound to PEGylated nanoparticles can interact with mucin in the mucosal layer and prevent passage to epithelial surfaces (Henry et al., 2016).
Some PEGylated nanomaterials and proteins do not display ABC in animal models (Koide et al., 2008; Kaminskas et al., 2011; Koide et al., 2012; Grenier et al., 2018) and one explanation for this phenomenon is that in order for ABC to occur, a threshold molar ratio of anti-PEG antibodies to PEG compound is required for efficient clearance (Shiraishi et al., 2016; McSweeney et al., 2018). For example, the molar concentration of PEG-proteins in circulation is typically lower than that of PEG-liposomes (Grenier et al., 2018) at therapeutic dosing levels; thus, nanoparticles are thought to be less vulnerable to anti-PEG antibody-associated clearance than proteins. Indeed, prior studies show that strong ABC is observed when the number of antibodies in circulation exceeds the number of PEGylated compounds (Xu et al., 2022). This trend holds across most PEGylated compounds including proteins, liposomes, micelles, and polymeric nanoparticles and agrees with previous studies showing that three anti-PEG antibodies per PEGylated protein or about 10 anti-PEG antibodies per pegylated liposome are required for ABC (Shiraishi et al., 2016; McSweeney et al., 2018; Chang et al., 2019). These findings suggest that only compounds dosed at very low molar concentrations (e.g. PEG-IFNα) may be susceptible to polymer-specific ABC whereas the estimated threshold concentration of anti-PEG antibodies needed to accelerate the clearance of nucleic acid drug carriers (e.g. Patrisan) overwhelmingly exceed those observed in patient blood (Xu et al., 2022).
In addition to formulation-dependent susceptibility to polymer immunogenicity, mode of administration can also modulate the impact of antibody recognition. Most clinically approved polymer-drug conjugates are intravenously administered and thus their interaction with plasma IgG and IgM is higher than may be expected following intramuscular or intratumoral injection, as is common among many mRNA indications including both BNT162b2/Comirnaty and mRNA-1273/Spikevax (Schoenmaker et al., 2021). Thus, the strikingly low rates of anaphylaxis observed following SARS-CoV-2 mRNA vaccination (CDC COVID-19 Response Team and Food and Drug Administration, 2021) may be attributable in part to its intramuscular administration. Future studies focusing on the impact of polymer type/architecture/density and corresponding immunogenicity on drug efficacy and transport (e.g. lymphatic) following local administration are therefore warranted.
PEG Immunogenicity can be Minimized but Alternative Polymers in Clinical Use are Lacking
Having established that PEG immunogenicity can limit the clinical utility of PEG-drug conjugates and that nanoparticle-based formulations may be less vulnerable to some of these effects relative to polymer-protein drug conjugates, how can one minimize the impact and risk of immunogenicity-diminished efficacy from future polymer-conjugated drugs and vaccines? As discussed above, PEG immunogenicity can arise through a variety of mechanisms (Xu et al., 2022) and includes antibody recognition associated with hypersensitivity reactions (e.g. anaphylaxis), accelerated blood clearance, premature drug release, or cross-reaction to other PEGylated therapies, among others. While limited in number, prior studies suggest that PEG antibody recognition is strongly dependent on polymer molecular weight (Xu et al., 2022), architecture, and end-functional group (Saifer et al., 2014). For example, antibodies with affinity towards backbone ethylene oxide units recognize immobilized PEG that is 2 kDa and larger with a minimum epitope subunit of approx. 16 repeats (700 Da) (Lee et al., 2020). Given that nearly all systemically administered polymer-drug conjugates are 2 kDa and above—per linear chain—the utilization of higher densities of lower molecular weight PEG may diminish the therapeutic impact of these backbone-specific antibodies. Such an approach is conceptually illustrated by branched PEG-drug conjugates (e.g. peginterferon alfa-2a, certolizumabpegol, and pegaptanib); however, those in clinical use (and which are systemically administrable) are limited to single site-modified, di-branched PEGs with per-arm molecular weight of approx. 10–30 kDa and with methoxy terminal groups; thus, the use of increasingly branched PEGs (i.e. hyperbranched, star, dendritic, bottlebrush) of lower per-branch molecular weight may diminish recognition by backbone-specific antibodies while maintaining favorable drug circulation, solubility, stability, activity profiles.
Polymer end-terminal groups can also play an important role in engineering future, less immunologically vulnerable PEG-drug conjugates as antibodies that recognize end-groups represent the other primary class of PEG-specific antibodies detected in vivo. While all clinical PEG-drug conjugates are chain-terminated by methoxy groups, recent preclinical studies suggest that hydroxy-terminal PEG conjugates generate lower amounts of backbone-specific anti-PEG IgM (Shimizu et al., 2018) and, while this improved immunogenicity comes with the tradeoff of higher complement activation and second-dose ABC (and typically, slightly shorter circulation half-life (Arvizo et al., 2011)), these findings may lead to the development of future polymer-drug conjugates with less propensity for immune activation. Other polymer end-group engineering strategies include the utilization of zwitterionic (Arvizo et al., 2011), ethoxy, and n-butyl ether (Saifer et al., 2014) moieties.
In addition to direct modifications of the polymer, corresponding drugs themselves can also modulate PEG immunogenicity. The introduction of 2’-fluro-modified pyrimidines and 2′-O-methyl-modifed purines has been shown to reduce the immunogenicity of PEGylated nucleic acids (Judge et al., 2005; Wang et al., 2009; Yu et al., 2009; Lee et al., 2016) while chemotherapeutics cytotoxic to B cells such as doxorubicin, mitoxantrone or oxaliplatin (Laverman et al., 2001; Ishida et al., 2006c; Cui et al., 2008; Abu Lila et al., 2012; Nagao et al., 2013) have been shown to mitigate anti-PEG IgM induced via PEGylated liposomal drug carriers often used to deliver these compounds in vivo (Cui et al., 2008; Mohamed et al., 2019).
Pharmacologic approaches have been further employed to diminish the impact of polymer immunogenicity including conjugation to or pre-treatment with immunosupressants, as well as the pre-treatment or co-infusion of tolerogenic compounds. Khanna et al. for example recently reported that pretreatment with the B/T cell immunosuppressant, mycophenolate mofetil, significantly improved treatment outcomes in a Phase I trial of patients with gout receiving pegloticase (Khanna et al., 2021). Other immunosuppressives under investigation to mitigate pegloticase immunogenicity include methotrexate, azathioprine, and leflunomide, while those used in conjunction with other ADA-prone therapies include rapamycin and anti-CD20. Likewise, pre-treatment or co-treatment with polymer, in particular high molecular weight (i.e. 40 kDa) PEG, has also been shown to reduce liposome-induced anti-PEG antibodies in preclinical studies (McSweeney et al., 2021). Taken together, these pharmacologic approaches are viewed by some to obviate the need PEG alternatives or derivatives; however, the deployment of immunosuppressives in combination with polymer-based vaccines and immunostimulatory therapies presents significant tradeoffs to drug efficacy, while PEG-based tolerogenics remain to be tested in patients.
Given 1) the therapeutic impact of PEG on drug immunogenicity, 2) the possible increasing prevalence of pre-existing and drug-induced PEG immunity, 3) the growing public need for safe and effective mRNA vaccines, and 4) our prevailing reliance on PEG for use in clinically approved nucleic acid and polymer-drug conjugate therapies (Schoenmaker et al., 2021), it is clear that the development and clinical validation of alternatives to (or derivatives of) PEG represents not only an unmet clinical need but also one with broad public health and national strategic interest. Indeed, the need for alternatives to PEG is a common refrain among those in the field (Harris, 1992), one as old as the first polymer-drug conjugate, Adagen; however, given the wide variety of potential candidate macromolecules such as polysaccharides, polyglycerols, and glycopolymers, (reviewed in detail elsewhere (Knop et al., 2010; Pelegri-O’Day et al., 2014; Bludau et al., 2017; Ekladious et al., 2019; Xu et al., 2022)), it begs the question as to why alternatives have yet to be approved (and studied post-approval) beyond poly(styrene co-maleic acid) (1993, Japan). Concerns over PEG immunogenicity have led some pharmaceutical companies to shy-away from or drop PEGylated products from their pipelines entirely (de Vrieze, 2020), thus the prospect of biopharma advancing clinically untested polymers through lengthy and expensive clinical trials is a difficult ask in the absence of a thoughtful incentive structure.
Given the challenging risk-reward of advancing non-PEG-based polymer-drug conjugates towards clinical translation, what can governments and funding agencies do to facilitate continued innovation in polymer-drug conjugate development and ensure the capacity for safe and effective vaccination at-scale? 1) Biosimilar-like regulatory guidelines for conjugable polymers (i.e. polysimilars) may be one approach to formalize and streamline the approval of new polymer-drug conjugates, albeit one likely requiring increased rigor given the wide structural diversity and potential health hazards of various polymer subunits relative to proteins. 2) Funding or federal lab support to perform large-scale longitudinal studies of immunogenicity towards polymers and other drug conjugates/excipients (lipids, polysaccharides, polypeptides, etc) would elucidate current (and potentially dynamic or age-, race-, and sex-specific) risks of polymer immunogenicity to human health, drug conjugate efficacy, and the strategic national need for mRNA vaccine-stabilizing polymers. 3) Federally subsidized R&D to offset the risks taken-on by companies exploring PEG- and other polymer-conjugates would greatly incentivize further innovation in this space. 4) Funding to improve our poor mechanistic understanding of polymer-induced immunogenicity and associated short- and long-term health risks would accelerate the discovery of new PEG derivatives and alternatives or propel historically utilized polymers through clinical translation. 5) Federal partnerships to ensure the financial viability of domestically manufactured, pharmaceutical-grade PEG and other polymers, as a matter of national interest, would ensure our readiness for future pandemics (and supply chain challenges) surely yet-to-come. In closing, while it is tempting to suggest a singular direction for polymer-drug conjugate development in the future, we also acknowledge that the ideal properties for a conjugation partner vary substantially with drug class, mode of administration, dosing frequency, and disease indication as discussed above; thus, with proper incentives, funding, and tools we anticipate that future conjugates will not only increase in diversity but also diverge based upon drug type and/or indication.
Author Contributions
YWK and ECD wrote and edited the manuscript.
Funding
This work was supported in part by the American Association of Cancer Research UK, the Coulter Department of Biomedical Engineering, and the Aflac Cancer and Blood Disorders Center of Children’s Healthcare of Atlanta.
Conflict of Interest
The authors declare that the research was conducted in the absence of any commercial or financial relationships that could be construed as a potential conflict of interest.
Publisher’s Note
All claims expressed in this article are solely those of the authors and do not necessarily represent those of their affiliated organizations, or those of the publisher, the editors and the reviewers. Any product that may be evaluated in this article, or claim that may be made by its manufacturer, is not guaranteed or endorsed by the publisher.
Acknowledgments
The authors wish to thank Steeley Dan for inspiring the title to this work. The content here is solely the responsibility of the authors and does not necessarily represent the official views of the organizations acknowledged herein.
References
Abu Lila, A. S., Eldin, N. E., Ichihara, M., Ishida, T., and Kiwada, H. (2012). Multiple Administration of PEG-Coated Liposomal Oxaliplatin Enhances its Therapeutic Efficacy: a Possible Mechanism and the Potential for Clinical Application. Int. J. Pharmaceutics 438, 176–183. doi:10.1016/j.ijpharm.2012.08.030
Alconcel, S. N. S., Baas, A. S., and Maynard, H. D. (2011). FDA-approved Poly(ethylene Glycol)-Protein Conjugate Drugs. Polym. Chem. 2, 1442–1448. doi:10.1039/c1py00034a
Armstrong, J. K., Hempel, G., Koling, S., Chan, L. S., Fisher, T., Meiselman, H. J., et al. (2007). Antibody against Poly(ethylene Glycol) Adversely Affects PEG-Asparaginase Therapy in Acute Lymphoblastic Leukemia Patients. Cancer 110, 103–111. doi:10.1002/cncr.22739
Arvizo, R. R., Miranda, O. R., Moyano, D. F., Walden, C. A., Giri, K., Bhattacharya, R., et al. (2011). Modulating Pharmacokinetics, Tumor Uptake and Biodistribution by Engineered Nanoparticles. PLOS ONE 6, e24374. doi:10.1371/journal.pone.0024374
Avci-Adali, M., Steinle, H., Michel, T., Schlensak, C., and Wendel, H. P. (2013). Potential Capacity of Aptamers to Trigger Immune Activation in Human Blood. PLoS One 8, e68810. doi:10.1371/journal.pone.0068810
Baden, L. R., El Sahly, H. M., Essink, B., Kotloff, K., Frey, S., Novak, R., et al. (2021). Efficacy and Safety of the mRNA-1273 SARS-CoV-2 Vaccine. N. Engl. J. Med. 384, 403–416. doi:10.1056/NEJMoa2035389
Beutier, H., Hechler, B., Godon, O., Wang, Y., Gillis, C. M., de Chaisemartin, L., et al. (2018). Platelets Expressing IgG Receptor FcγRIIA/CD32A Determine the Severity of Experimental Anaphylaxis. Sci. Immunol. 3, 5997. doi:10.1126/sciimmunol.aan5997
Bludau, H., Czapar, A. E., Pitek, A. S., Shukla, S., Jordan, R., and Steinmetz, N. F. (2017). POxylation as an Alternative Stealth Coating for Biomedical Applications. Eur. Polym. J. 88, 679–688. doi:10.1016/j.eurpolymj.2016.10.041
Browne, E. K., Moore, C., Sykes, A., Lu, Z., Jeha, S., and Mandrell, B. N. (2018). Clinical Characteristics of Intravenous PEG-Asparaginase Hypersensitivity Reactions in Patients Undergoing Treatment for Acute Lymphoblastic Leukemia. J. Pediatr. Oncol. Nurs. 35, 103–109. doi:10.1177/1043454217741868
CDC COVID-19 Response Team and Food and Drug Administration (2021). Allergic Reactions Including Anaphylaxis after Receipt of the First Dose of Pfizer-BioNTech COVID-19 Vaccine — United States, December 14-23, 2020. MMWR Morb. Mortal Wkly. Rep. 70, 46–51. doi:10.15585/mmwr.mm7002e1externalicon
Covid-data-tracker (2022). Covid-data-tracker CDC Available at: https://covid.cdc.gov/covid-data-tracker (Accessed 01 25, 2022).
Chanan-Khan, A., Szebeni, J., Savay, S., Liebes, L., Rafique, N. M., Alving, C. R., et al. (2003). Complement Activation Following First Exposure to Pegylated Liposomal Doxorubicin (Doxil): Possible Role in Hypersensitivity Reactions. Ann. Oncol. 14, 1430–1437. doi:10.1093/annonc/mdg374
Chang, T. C., Chen, B. M., Lin, W. W., Yu, P. H., Chiu, Y. W., Chen, Y. T., et al. (2019). Both IgM and IgG Antibodies against Polyethylene Glycol Can Alter the Biological Activity of Methoxy Polyethylene Glycol-Epoetin Beta in Mice. Pharmaceutics 12. doi:10.3390/pharmaceutics12010015
Chen, B.-M., Cheng, T.-L., and Roffler, S. R. (2021). Polyethylene Glycol Immunogenicity: Theoretical, Clinical, and Practical Aspects of Anti-polyethylene Glycol Antibodies. ACS Nano 15, 14022–14048. doi:10.1021/acsnano.1c05922
Chen, B.-M., Su, Y.-C., Chang, C.-J., Burnouf, P.-A., Chuang, K.-H., Chen, C.-H., et al. (2016). Measurement of Pre-existing IgG and IgM Antibodies against Polyethylene Glycol in Healthy Individuals. Anal. Chem. 88, 10661–10666. doi:10.1021/acs.analchem.6b03109
Chen, E., Chen, B.-M., Su, Y.-C., Chang, Y.-C., Cheng, T.-L., Barenholz, Y., et al. (2020). Premature Drug Release from Polyethylene Glycol (PEG)-Coated Liposomal Doxorubicin via Formation of the Membrane Attack Complex. ACS Nano 14, 7808–7822. doi:10.1021/acsnano.9b07218
Cheng, T.-L., Chen, B.-M., Chern, J.-W., Wu, M.-F., and Roffler, S. R. (2000). Efficient Clearance of Poly(ethylene Glycol)-Modified Immunoenzyme with Anti-PEG Monoclonal Antibody for Prodrug Cancer Therapy. Bioconjug. Chem. 11, 258–266. doi:10.1021/bc990147j
Cheng, T.-L., Wu, P.-Y., Wu, M.-F., Chern, J.-W., and Roffler, S. R. (1999). Accelerated Clearance of Polyethylene Glycol-Modified Proteins by Anti-polyethylene Glycol IgM. Bioconjug. Chem. 10, 520–528. doi:10.1021/bc980143z
Cui, J., Li, C., Wang, C., Li, Y., Zhang, L., Zhang, L., et al. (2008). Repeated Injection of Pegylated Liposomal Antitumour Drugs Induces the Disappearance of the Rapid Distribution Phase. J. Pharm. Pharmacol. 60, 1651–1657. doi:10.1211/jpp/60.12.0011
Dams, E. T., Laverman, P., Oyen, W. J., Storm, G., Scherphof, G. L., van Der Meer, J. W., et al. (2000). Accelerated Blood Clearance and Altered Biodistribution of Repeated Injections of Sterically Stabilized Liposomes. J. Pharmacol. Exp. Ther. 292, 1071–1079.
de Groot, M. C., van Zwieten-Boot, B. J., and van Grootheest, A. C. (2004). Severe Adverse Reactions after the Use of sulphur Hexafluoride (SonoVue) as an Ultrasonographic Contrast Agent. Ned Tijdschr Geneeskd 148, 1887–1888.
de Vrieze, J. (2021). Pfizer's Vaccine Raises Allergy Concerns. Science 371, 10–11. doi:10.1126/science.371.6524.10
de Vrieze, J. (2020). Suspicions Grow that Nanoparticles in Pfizer’s COVID-19 Vaccine Trigger Rare Allergic Reactions. Science 10. doi:10.1126/science.abg2359
Dézsi, L., Fülöp, T., Mészáros, T., Szénási, G., Urbanics, R., Vázsonyi, C., et al. (2014). Features of Complement Activation-Related Pseudoallergy to Liposomes with Different Surface Charge and PEGylation: Comparison of the Porcine and Rat Responses. J. Controlled Release 195, 2–10. doi:10.1016/j.jconrel.2014.08.009
Ding, F., Li, C., Xu, Y., Li, J., Li, H., Yang, G., et al. (2018). PEGylation Regulates Self-Assembled Small-Molecule Dye-Based Probes from Single Molecule to Nanoparticle Size for Multifunctional NIR-II Bioimaging. Adv. Healthc. Mater. 7, e1800973. doi:10.1002/adhm.201800973
Ekladious, I., Colson, Y. L., and Grinstaff, M. W. (2019). Polymer-drug Conjugate Therapeutics: Advances, Insights and Prospects. Nat. Rev. Drug Discov. 18, 273–294. doi:10.1038/s41573-018-0005-0
Elsadek, N. E., Emam, S. E., Abu Lila, A. S., Shimizu, T., Ando, H., Ishima, Y., et al. (2020). Pegfilgrastim (PEG-G-CSF) Induces Anti-polyethylene Glycol (PEG) IgM via a T Cell-dependent Mechanism. Biol. Pharm. Bull. 43, 1393–1397. doi:10.1248/bpb.b20-00345
Finkelman, F. D. (2007). Anaphylaxis: Lessons from Mouse Models. J. Allergy Clin. Immunol. 120, 506–515. doi:10.1016/j.jaci.2007.07.033
Fix, S. M., Nyankima, A. G., McSweeney, M. D., Tsuruta, J. K., Lai, S. K., and Dayton, P. A. (2018). Accelerated Clearance of Ultrasound Contrast Agents Containing Polyethylene Glycol Is Associated with the Generation of Anti-polyethylene Glycol Antibodies. Ultrasound Med. Biol. 44, 1266–1280. doi:10.1016/j.ultrasmedbio.2018.02.006
Freire Haddad, H., Burke, J. A., Scott, E. A., and Ameer, G. A. (2022). Clinical Relevance of Pre-existing and Treatment-Induced Anti-Poly(Ethylene Glycol) Antibodies. Regen. Eng. Transl Med. 8, 32–42. doi:10.1007/s40883-021-00198-y
Garratty, G. (2004). Progress in Modulating the RBC Membrane to Produce Transfusable Universal/stealth Donor RBCs. Transfus. Med. Rev. 18, 245–256. doi:10.1016/j.tmrv.2004.06.005
Geleijnse, M. L., Nemes, A., Vletter, W. B., Michels, M., Soliman, O. I., Caliskan, K., et al. (2009). Adverse Reactions after the Use of sulphur Hexafluoride (SonoVue) echo Contrast Agent. J. Cardiovasc. Med. 10, 75–77. doi:10.2459/jcm.0b013e328319bfba
Grenier, P., Viana, I. M. d. O., Lima, E. M., and Bertrand, N. (2018). Anti-polyethylene Glycol Antibodies Alter the Protein corona Deposited on Nanoparticles and the Physiological Pathways Regulating Their Fate In Vivo. J. Controlled Release 287, 121–131. doi:10.1016/j.jconrel.2018.08.022
Gupta, S., Lau, K., Harding, C. O., Shepherd, G., Boyer, R., Atkinson, J. P., et al. (2018). Association of Immune Response with Efficacy and Safety Outcomes in Adults with Phenylketonuria Administered Pegvaliase in Phase 3 Clinical Trials. EBioMedicine 37, 366–373. doi:10.1016/j.ebiom.2018.10.038
Haag, R., and Kratz, F. (2006). Polymer Therapeutics: Concepts and Applications. Angew. Chem. Int. Ed. 45, 1198–1215. doi:10.1002/anie.200502113
Harris, J. M., and Chess, R. B. (2003). Effect of Pegylation on Pharmaceuticals. Nat. Rev. Drug Discov. 2, 214–221. doi:10.1038/nrd1033
Harris, J. M. (1992). Poly (Ethylene Glycol) Chemistry: Biotechnical and Biomedical Applications. Springer Science & Business Media.
Hasan, H., Shaikh, O. M., Rassekh, S. R., Howard, A. F., and Goddard, K. (2017). Comparison of Hypersensitivity Rates to Intravenous and Intramuscular PEG-Asparaginase in Children with Acute Lymphoblastic Leukemia: A Meta-Analysis and Systematic Review. Pediatr. Blood Cancer 64, 81–88. doi:10.1002/pbc.26200
Hashimoto, Y., Uehara, Y., Abu Lila, A. S., Ishida, T., and Kiwada, H. (2014). Activation of TLR9 by Incorporated pDNA within PEG-Coated Lipoplex Enhances Anti-PEG IgM Production. Gene Ther. 21, 593–598. doi:10.1038/gt.2014.32
Henry, C. E., Wang, Y.-Y., Yang, Q., Hoang, T., Chattopadhyay, S., Hoen, T., et al. (2016). Anti-PEG Antibodies Alter the Mobility and Biodistribution of Densely PEGylated Nanoparticles in Mucus. Acta Biomater. 43, 61–70. doi:10.1016/j.actbio.2016.07.019
Hershfield, M. S., Buckley, R. H., Greenberg, M. L., Melton, A. L., Schiff, R., Hatem, C., et al. (1987). Treatment of Adenosine Deaminase Deficiency with Polyethylene Glycol-Modified Adenosine Deaminase. N. Engl. J. Med. 316, 589–596. doi:10.1056/nejm198703053161005
Hoffman, A. S. (2016). The Early Days of PEG and PEGylation (1970s-1990s). Acta Biomater. 40, 1–5. doi:10.1016/j.actbio.2016.05.029
Ishida, T., Atobe, K., Wang, X., and Kiwada, H. (2006). Accelerated Blood Clearance of PEGylated Liposomes upon Repeated Injections: Effect of Doxorubicin-Encapsulation and High-Dose First Injection. J. Controlled Release 115, 251–258. doi:10.1016/j.jconrel.2006.08.017
Ishida, T., Ichihara, M., Wang, X., and Kiwada, H. (2006). Spleen Plays an Important Role in the Induction of Accelerated Blood Clearance of PEGylated Liposomes. J. Controlled Release 115, 243–250. doi:10.1016/j.jconrel.2006.08.001
Ishida, T., Ichihara, M., Wang, X., Yamamoto, K., Kimura, J., Majima, E., et al. (2006). Injection of PEGylated Liposomes in Rats Elicits PEG-specific IgM, Which Is Responsible for Rapid Elimination of a Second Dose of PEGylated Liposomes. J. Controlled Release 112, 15–25. doi:10.1016/j.jconrel.2006.01.005
Ishida, T., Kashima, S., and Kiwada, H. (2008). The Contribution of Phagocytic Activity of Liver Macrophages to the Accelerated Blood Clearance (ABC) Phenomenon of PEGylated Liposomes in Rats. J. Controlled Release 126, 162–165. doi:10.1016/j.jconrel.2007.11.009
Ishida, T., and Kiwada, H. (2008). Accelerated Blood Clearance (ABC) Phenomenon upon Repeated Injection of PEGylated Liposomes. Int. J. Pharmaceutics 354, 56–62. doi:10.1016/j.ijpharm.2007.11.005
Ishida, T., Maeda, R., Ichihara, M., Irimura, K., and Kiwada, H. (2003). Accelerated Clearance of PEGylated Liposomes in Rats after Repeated Injections. J. Controlled Release 88, 35–42. doi:10.1016/s0168-3659(02)00462-5
Ishihara, T., Takeda, M., Sakamoto, H., Kimoto, A., Kobayashi, C., Takasaki, N., et al. (2009). Accelerated Blood Clearance Phenomenon upon Repeated Injection of PEG-Modified PLA-Nanoparticles. Pharm. Res. 26, 2270–2279. doi:10.1007/s11095-009-9943-x
Jatzkewitz, H. (1955). An ein kolloidales Blutplasma-Ersatzmittel (Polyvinylpyrrolidon) gebundenes Peptamin (Glycyl-L-leucyl-mezcalin) als neuartige Depotform für biologisch aktive primäre Amine (Mezcalin). Z. Naturforsch. B 10, 27–31. doi:10.1515/znb-1955-0106
Jatzkewitz, H. (1954). Über den Einbau physiologisch wirksamer Substanzen in ein kollodiales Blutplasma-Ersatzmittel. Hoppe-Seyler´s Z. für physiologische Chem. 297, 149–156. doi:10.1515/bchm2.1954.297.1.149
Jokerst, J. V., Lobovkina, T., Zare, R. N., and Gambhir, S. S. (2011). Nanoparticle PEGylation for Imaging and Therapy. Nanomedicine 6, 715–728. doi:10.2217/nnm.11.19
Judge, A. D., Sood, V., Shaw, J. R., Fang, D., McClintock, K., and MacLachlan, I. (2005). Sequence-dependent Stimulation of the Mammalian Innate Immune Response by Synthetic siRNA. Nat. Biotechnol. 23, 457–462. doi:10.1038/nbt1081
Judge, A., and MacLachlan, I. (2008). Overcoming the Innate Immune Response to Small Interfering RNA. Hum. Gene Ther. 19, 111–124. doi:10.1089/hum.2007.179
Judge, A., McClintock, K., Phelps, J. R., and Maclachlan, I. (2006). Hypersensitivity and Loss of Disease Site Targeting Caused by Antibody Responses to PEGylated Liposomes. Mol. Ther. 13, 328–337. doi:10.1016/j.ymthe.2005.09.014
Kaminskas, L. M., McLeod, V. M., Porter, C. J. H., and Boyd, B. J. (2011). Differences in Colloidal Structure of PEGylated Nanomaterials Dictate the Likelihood of Accelerated Blood Clearance. J. Pharm. Sci. 100, 5069–5077. doi:10.1002/jps.22682
Khanna, P. P., Khanna, D., Cutter, G., Foster, J., Melnick, J., Jaafar, S., et al. (2021). Reducing Immunogenicity of Pegloticase with Concomitant Use of Mycophenolate Mofetil in Patients with Refractory Gout: A Phase II, Randomized, Double‐Blind, Placebo‐Controlled Trial. Arthritis Rheumatol. 73, 1523–1532. doi:10.1002/art.41731
Knop, K., Hoogenboom, R., Fischer, D., and Schubert, U. S. (2010). Poly(ethylene Glycol) in Drug Delivery: Pros and Cons as Well as Potential Alternatives. Angew. Chem. Int. Edition 49, 6288–6308. doi:10.1002/anie.200902672
Koide, H., Asai, T., Hatanaka, K., Urakami, T., Ishii, T., Kenjo, E., et al. (2008). Particle Size-dependent Triggering of Accelerated Blood Clearance Phenomenon. Int. J. Pharmaceutics 362, 197–200. doi:10.1016/j.ijpharm.2008.06.004
Koide, H., Asai, T., Kato, H., Ando, H., Shiraishi, K., Yokoyama, M., et al. (2012). Size-dependent Induction of Accelerated Blood Clearance Phenomenon by Repeated Injections of Polymeric Micelles. Int. J. Pharmaceutics 432, 75–79. doi:10.1016/j.ijpharm.2012.04.049
Kozma, G. T., Mészáros, T., Vashegyi, I., Fülöp, T., Örfi, E., Dézsi, L., et al. (2019). Pseudo-anaphylaxis to Polyethylene Glycol (PEG)-Coated Liposomes: Roles of Anti-PEG IgM and Complement Activation in a Porcine Model of Human Infusion Reactions. ACS Nano 13, 9315–9324. doi:10.1021/acsnano.9b03942
Laverman, P., Carstens, M. G., Boerman, O. C., Dams, E. T., Oyen, W. J., van Rooijen, N., et al. (2001). Factors Affecting the Accelerated Blood Clearance of Polyethylene Glycol-Liposomes upon Repeated Injection. J. Pharmacol. Exp. Ther. 298, 607–612.
Lee, C.-C., Su, Y.-C., Ko, T.-P., Lin, L.-L., Yang, C.-Y., Chang, S. S.-C., et al. (2020). Structural Basis of Polyethylene Glycol Recognition by Antibody. J. Biomed. Sci. 27, 12. doi:10.1186/s12929-019-0589-7
Lee, Y., Urban, J. H., Xu, L., Sullenger, B. A., and Lee, J. (2016). 2′Fluoro Modification Differentially Modulates the Ability of RNAs to Activate Pattern Recognition Receptors. Nucleic Acid Ther. 26, 173–182. doi:10.1089/nat.2015.0575
Li, L., Chen, S., Zheng, J., Ratner, B. D., and Jiang, S. (2005). Protein Adsorption on Oligo(ethylene Glycol)-Terminated Alkanethiolate Self-Assembled Monolayers: The Molecular Basis for Nonfouling Behavior. J. Phys. Chem. B 109, 2934–2941. doi:10.1021/jp0473321
Lipsky, P. E., Calabrese, L. H., Kavanaugh, A., Sundy, J. S., Wright, D., Wolfson, M., et al. (2014). Pegloticase Immunogenicity: the Relationship between Efficacy and Antibody Development in Patients Treated for Refractory Chronic Gout. Arthritis Res. Ther. 16, R60. doi:10.1186/ar4497
Liu, Y., Smith, C. A., Panetta, J. C., Yang, W., Thompson, L. E., Counts, J. P., et al. (2019). Antibodies Predict Pegaspargase Allergic Reactions and Failure of Rechallenge. Jco 37, 2051–2061. doi:10.1200/jco.18.02439
Longo, N., Harding, C. O., Burton, B. K., Grange, D. K., Vockley, J., Wasserstein, M., et al. (2014). Single-dose, Subcutaneous Recombinant Phenylalanine Ammonia Lyase Conjugated with Polyethylene Glycol in Adult Patients with Phenylketonuria: an Open-Label, Multicentre, Phase 1 Dose-Escalation Trial. The Lancet 384, 37–44. doi:10.1016/s0140-6736(13)61841-3
Lubich, C., Allacher, P., de la Rosa, M., Bauer, A., Prenninger, T., Horling, F. M., et al. (2016). The Mystery of Antibodies against Polyethylene Glycol (PEG) - what Do We Know? Pharm. Res. 33, 2239–2249. doi:10.1007/s11095-016-1961-x
Luxenhofer, R. (2020). A Tutorial Translation of the Description of the Historically First Polymer Drug Conjugate and its In Vivo Evaluation. Z. Naturforsch. C 75, 303–311. doi:10.1515/znc-2020-0077
McSweeney, M. D., Shen, L., DeWalle, A. C., Joiner, J. B., Ciociola, E. C., Raghuwanshi, D., et al. (2021). Pre-treatment with High Molecular Weight Free PEG Effectively Suppresses Anti-PEG Antibody Induction by PEG-Liposomes in Mice. J. Controlled Release 329, 774–781. doi:10.1016/j.jconrel.2020.10.011
McSweeney, M. D., Wessler, T., Price, L. S. L., Ciociola, E. C., Herity, L. B., Piscitelli, J. A., et al. (2018). A Minimal Physiologically Based Pharmacokinetic Model that Predicts Anti-PEG IgG-Mediated Clearance of PEGylated Drugs in Human and Mouse. J. Controlled Release 284, 171–178. doi:10.1016/j.jconrel.2018.06.002
Mima, Y., Hashimoto, Y., Shimizu, T., Kiwada, H., and Ishida, T. (2015). Anti-PEG IgM Is a Major Contributor to the Accelerated Blood Clearance of Polyethylene Glycol-Conjugated Protein. Mol. Pharmaceutics 12, 2429–2435. doi:10.1021/acs.molpharmaceut.5b00144
Mohamed, M., Abu Lila, A. S., Shimizu, T., Alaaeldin, E., Hussein, A., Sarhan, H. A., et al. (2019). PEGylated Liposomes: Immunological Responses. Sci. Techn. Adv. Mater. 20, 710–724. doi:10.1080/14686996.2019.1627174
Nagao, A., Abu Lila, A. S., Ishida, T., and Kiwada, H. (2013). Abrogation of the Accelerated Blood Clearance Phenomenon by SOXL Regimen: Promise for Clinical Application. Int. J. Pharmaceutics 441, 395–401. doi:10.1016/j.ijpharm.2012.11.015
Neun, B. W., Barenholz, Y., Szebeni, J., and Dobrovolskaia, M. A. (2018). Understanding the Role of Anti-PEG Antibodies in the Complement Activation by Doxil In Vitro. Molecules 23, 71700. doi:10.3390/molecules23071700
Pelegri-O’Day, E. M., Lin, E.-W., and Maynard, H. D. (2014). Therapeutic Protein–Polymer Conjugates: Advancing beyond PEGylation. J. Am. Chem. Soc. 136, 14323–14332.
Perdue, L. A., Do, P., David, C., Chyong, A., Kellner, A. V., Ruggieri, A., et al. (2020). Optical Control of Cytokine Signaling via Bioinspired, Polymer-Induced Latency. Biomacromolecules 21, 2635–2644. doi:10.1021/acs.biomac.0c00264
Pérez-Pérez, L., García-Gavín, J., Piñeiro, B., and Zulaica, A. (2011). Biologic-induced Urticaria Due to Polysorbate 80: Usefulness of Prick Test. Br. J. Dermatol. 164, 1119–1120. doi:10.1111/j.1365-2133.2011.10220.x
Polack, F. P., Thomas, S. J., Kitchin, N., Absalon, J., Gurtman, A., Lockhart, S., et al. (2020). Safety and Efficacy of the BNT162b2 mRNA Covid-19 Vaccine. N. Engl. J. Med. 383, 2603–2615. doi:10.1056/nejmoa2034577
Povsic, T. J., Lawrence, M. G., Lincoff, A. M., Mehran, R., Rusconi, C. P., Zelenkofske, S. L., et al. (2016). Pre-existing Anti-PEG Antibodies Are Associated with Severe Immediate Allergic Reactions to Pegnivacogin, a PEGylated Aptamer. J. Allergy Clin. Immunol. 138, 1712–1715. doi:10.1016/j.jaci.2016.04.058
Povsic, T. J., Vavalle, J. P., Aberle, L. H., Kasprzak, J. D., Cohen, M. G., Mehran, R., et al. (2013). A Phase 2, Randomized, Partially Blinded, Active-Controlled Study Assessing the Efficacy and Safety of Variable Anticoagulation Reversal Using the REG1 System in Patients with Acute Coronary Syndromes: Results of the RADAR Trial. Eur. Heart J. 34, 2481–2489. doi:10.1093/eurheartj/ehs232
Prime, K. L., and Whitesides, G. M. (1993). Adsorption of Proteins onto Surfaces Containing End-Attached Oligo(ethylene Oxide): a Model System Using Self-Assembled Monolayers. J. Am. Chem. Soc. 115, 10714–10721. doi:10.1021/ja00076a032
Rau, R. E., Dreyer, Z., Choi, M. R., Liang, W., Skowronski, R., Allamneni, K. P., et al. (2018). Outcome of Pediatric Patients with Acute Lymphoblastic Leukemia/lymphoblastic Lymphoma with Hypersensitivity to Pegaspargase Treated with PEGylated Erwinia Asparaginase, Pegcrisantaspase: A Report from the Children's Oncology Group. Pediatr. Blood Cancer 65. doi:10.1002/pbc.26873
Reber, L. L., Hernandez, J. D., and Galli, S. J. (2017). The Pathophysiology of Anaphylaxis. J. Allergy Clin. Immunol. 140, 335–348. doi:10.1016/j.jaci.2017.06.003
Richter, A. W., and Åkerblom, E. (1984). Polyethylene Glycol Reactive Antibodies in Man: Titer Distribution in Allergic Patients Treated with Monomethoxy Polyethylene Glycol Modified Allergens or Placebo, and in Healthy Blood Donors. Int. Arch. Allergy Immunol. 74, 36–39. doi:10.1159/000233512
Ringsdorf, H. (1975). Structure and Properties of Pharmacologically Active Polymers. J. Polym. Sci. C Polym. Symp. 51, 135–153. doi:10.1002/polc.5070510111
Saifer, M. G. P., Williams, L. D., Sobczyk, M. A., Michaels, S. J., and Sherman, M. R. (2014). Selectivity of Binding of PEGs and PEG-like Oligomers to Anti-PEG Antibodies Induced by methoxyPEG-Proteins. Mol. Immunol. 57, 236–246. doi:10.1016/j.molimm.2013.07.014
Schoenmaker, L., Witzigmann, D., Kulkarni, J. A., Verbeke, R., Kersten, G., Jiskoot, W., et al. (2021). mRNA-Lipid Nanoparticle COVID-19 Vaccines: Structure and Stability. Int. J. Pharmaceutics 601, 120586. doi:10.1016/j.ijpharm.2021.120586
Semple, S. C., Harasym, T. O., Clow, K. A., Ansell, S. M., Klimuk, S. K., and Hope, M. J. (2005). Immunogenicity and Rapid Blood Clearance of Liposomes Containing Polyethylene Glycol-Lipid Conjugates and Nucleic Acid. J. Pharmacol. Exp. Ther. 312, 1020–1026. doi:10.1124/jpet.104.078113
Shah, S., Prematta, T., Adkinson, N. F., and Ishmael, F. T. (2013). Hypersensitivity to Polyethylene Glycols. J. Clin. Pharmacol. 53, 352–355. doi:10.1177/0091270012447122
Shimizu, T., Abu Lila, A. S., Fujita, R., Awata, M., Kawanishi, M., Hashimoto, Y., et al. (2018). A Hydroxyl PEG Version of PEGylated Liposomes and its Impact on Anti-PEG IgM Induction and on the Accelerated Clearance of PEGylated Liposomes. Eur. J. Pharmaceutics Biopharmaceutics 127, 142–149. doi:10.1016/j.ejpb.2018.02.019
Shiraishi, K., Kawano, K., Maitani, Y., Aoshi, T., Ishii, K. J., Sanada, Y., et al. (2016). Exploring the Relationship between Anti-PEG IgM Behaviors and PEGylated Nanoparticles and its Significance for Accelerated Blood Clearance. J. Controlled Release 234, 59–67. doi:10.1016/j.jconrel.2016.05.010
Shpetner, H. S., and Vallee, R. B. (1991). [17] Purification and Characterization of Dynamin. Methods Enzymol. 196, 192–201. doi:10.1016/0076-6879(91)96019-n
Stone, C. A., Liu, Y., Relling, M. V., Krantz, M. S., Pratt, A. L., Abreo, A., et al. (2019). Immediate Hypersensitivity to Polyethylene Glycols and Polysorbates: More Common Than We Have Recognized. J. Allergy Clin. Immunol. Pract. 7, 1533–1540. doi:10.1016/j.jaip.2018.12.003
Szebeni, J. (2014). Complement Activation-Related Pseudoallergy: a Stress Reaction in Blood Triggered by Nanomedicines and Biologicals. Mol. Immunol. 61, 163–173. doi:10.1016/j.molimm.2014.06.038
Szebeni, J., Muggia, F., Gabizon, A., and Barenholz, Y. (2011). Activation of Complement by Therapeutic Liposomes and Other Lipid Excipient-Based Therapeutic Products: Prediction and Prevention. Adv. Drug Deliv. Rev. 63, 1020–1030. doi:10.1016/j.addr.2011.06.017
Wang, D., Bhagat, L., Yu, D., Zhu, F.-G., Tang, J. X., Kandimalla, E. R., et al. (2009). Oligodeoxyribonucleotide-based Antagonists for Toll-like Receptors 7 and 9. J. Med. Chem. 52, 551–558. doi:10.1021/jm8014316
Xu, Y., Tuo, W., Yang, L., Sun, Y., Li, C., Chen, X., et al. (2022). Design of a Metallacycle-Based Supramolecular Photosensitizer for In Vivo Image-Guided Photodynamic Inactivation of Bacteria. Angew. Chem. Int. Ed. Engl. 61, e202110048. doi:10.1002/anie.202110048
Yang, Q., Jacobs, T. M., McCallen, J. D., Moore, D. T., Huckaby, J. T., Edelstein, J. N., et al. (2016). Analysis of Pre-existing IgG and IgM Antibodies against Polyethylene Glycol (PEG) in the General Population. Anal. Chem. 88, 11804–11812. doi:10.1021/acs.analchem.6b03437
Yu, D., Wang, D., Zhu, F.-G., Bhagat, L., Dai, M., Kandimalla, E. R., et al. (2009). Modifications Incorporated in CpG Motifs of Oligodeoxynucleotides lead to Antagonist Activity of Toll-like Receptors 7 and 9. J. Med. Chem. 52, 5108–5114. doi:10.1021/jm900730r
Zhang, X., Goel, V., and Robbie, G. J. (2020). Pharmacokinetics of Patisiran, the First Approved RNA Interference Therapy in Patients with Hereditary Transthyretin-Mediated Amyloidosis. J. Clin. Pharm. 60, 573–585. doi:10.1002/jcph.1480
Keywords: polymer chemistry, drug delivery, nanotechnology, nanomaterials, PEG
Citation: Kong YW and Dreaden EC (2022) PEG: Will It Come Back to You? Polyethelyne Glycol Immunogenicity, COVID Vaccines, and the Case for New PEG Derivatives and Alternatives. Front. Bioeng. Biotechnol. 10:879988. doi: 10.3389/fbioe.2022.879988
Received: 20 February 2022; Accepted: 11 April 2022;
Published: 27 April 2022.
Edited by:
Zhimin Tao, Jiangsu University, ChinaReviewed by:
Thomas Anchordoquy, University of Colorado Anschutz Medical Campus, United StatesYao Sun, Central China Normal University, China
Yang Qinglai, University of South China, China
Copyright © 2022 Kong and Dreaden. This is an open-access article distributed under the terms of the Creative Commons Attribution License (CC BY). The use, distribution or reproduction in other forums is permitted, provided the original author(s) and the copyright owner(s) are credited and that the original publication in this journal is cited, in accordance with accepted academic practice. No use, distribution or reproduction is permitted which does not comply with these terms.
*Correspondence: Yi Wen Kong, eXdrb25nQG1pdC5lZHU=; Erik C Dreaden, ZS5kcmVhZGVuQGVtb3J5LmVkdQ==, ZS5kcmVhZGVuQGdhdGVjaC5lZHU=