Microalgae-based wastewater treatment for developing economic and environmental sustainability: Current status and future prospects
- 1Center of Excellence in Bioconversion and Bioseparation for Platform Chemical Production, Institute of Biotechnology and Genetic Engineering, Chulalongkorn University, Pathumwan, Bangkok, Thailand
- 2Panyapiwat Institute of Management Demonstration School, Pakkred, Nonthaburi, Thailand
Over the last several decades, concerns about climate change and pollution due to human activity has gained widespread attention. Microalgae have been proposed as a suitable biological platform to reduce carbon dioxide, a major greenhouse gas, while also creating commercial sources of high-value compounds such as medicines, cosmetics, food, feed, and biofuel. Industrialization of microalgae culture and valorization is still limited by significant challenges in scaling up the production processes due to economic constraints and productivity capacities. Therefore, a boost in resource usage efficiency is required. This enhancement not only lowers manufacturing costs but also enhancing the long-term viability of microalgae-based products. Using wastewater as a nutrient source is a great way to reduce manufacturing costs. Furthermore, water scarcity is one of the most important global challenges. In recent decades, industrialization, globalization, and population growth have all impacted freshwater resources. Moreover, high amounts of organic and inorganic toxins in the water due to the disposal of waste into rivers can have severe impacts on human and animal health. Microalgae cultures are a sustainable solution to tertiary and quaternary treatments since they have the ability to digest complex contaminants. This review presents biorefineries based on microalgae from all angles, including the potential for environmental pollution remediation as well as applications for bioenergy and value-added biomolecule production. An overview of current information about microalgae-based technology and a discussion of the associated hazards and opportunities for the bioeconomy are highlighted.
1 Introduction
At present, global warming issues are escalating at an alarming degree and are becoming increasingly interconnected with others. Extreme weather events such as heat waves, droughts, and floods are becoming more frequent and intense as a result of climate change and putting human security at risk. Meanwhile, global warming exacerbates issues such as soil degradation, biodiversity loss, disease transmission, as well as water shortages. It has been speculated that these changes will have an impact on patterns of economic development, political stability, and the well-being of people. Carbon dioxide (CO2), a significant greenhouse gas (GHG) present in the Earth’s atmosphere that accounts for up to 60% of all greenhouse gases, has been increasing as a result of different human activities that contribute to global warming. CO2 emissions began to rapidly increase in the 1950s, reaching 25.23 billion metric tons of CO2 emission in 2000. Between 2000 and 2010, the emission of CO2 increased by 32%, reaching 34.81 billion metric tons in 2020. In 2020, Thailand accounted for 0.76 percent of world emissions (258 million tons of CO2) and was placed 24th in terms of CO2 emissions globally (Global Carbon Project, 2021). Moreover, Thailand is ranked by Germanwatch as one of the top ten countries at high risk of long-term climate change (Eckstein et al., 2021). Since Thailand is a developing country that depends on fossil fuel energy and has expanding metropolitan areas, the Thai government, like governments in many other countries around the world, has focused on how to reduce CO2 emissions by 7.6 percent per year for the next decade in order for Thailand to meet its commitment to prevent global warming from exceeding 1.5°C over pre-industrial levels. This goal, laid out in the 2015 Paris climate agreement, must be met in order to satisfy the demands of sustainable corporate practices in social and environmental responsibility, on which many nations have agreed (Tollefson, 2021).
There are two effective ways to reduce carbon dioxide emissions, including the use of alternative energy and expanding the techniques to capture and store CO2 to reduce emissions over the long term. Different biological, chemical, or physical processes can be used to capture and store CO2. Nonetheless, existing technologies have a number of technical and financial restrictions. Consequently, it is imperative that current technologies be upgraded and new ones be developed. As a comparison, biological CO2 fixation seems to be a more cost-effective and environmentally-friendly technology than physical or chemical methods. Biological CO2 fixation through photosynthesis is the process by which photosynthetic organisms absorb CO2, thus assisting in the control of atmospheric CO2 levels (Daneshvar et al., 2022). Compared to terrestrial crops, microalgae can grow faster, are more adaptable, and can fix CO2 at a rate that is 10–50 times greater than other land plants (Batista et al., 2015; Cuellar-Bermudez et al., 2015). Moreover, carbon sequestration using microalgae has been hailed as one of the most important and successful technologies in the world over the last few decades (Alami et al., 2021). Additionally, microalgae have the ability to survive in a wide variety of conditions without competing for food with humans and animals. Microalgae have a diverse range of functional elements, including peptides, carbohydrates, lipids, pigments, vitamins, and minerals, all of which have been shown to provide a variety of benefits. Microalgae have therefore generated widespread attention due to their potential use in various fields such as medicine, aquaculture, animal and human nutritional supplementation, agricultural production, and bioenergy.
Despite the enormous promise of microalgae in a variety of applications, their use is currently limited to laboratory settings. For a variety of reasons, industrial level applications have not been taken forward as much as they could, with the fundamental issue being the high economic costs associated with large-scale applications. The high cost of artificial medium and the poor biomass yield are two constraints connected with the generation of raw materials for diverse applications by microalgae (Pandey et al., 2019). To solve this problem, the use of effluents to culture microalgae is one of the most effective ways to emerge as a viable option to lower process costs and obtain microalgae biomass for a range of applications. Many studies reveal that microalgae can completely remove nitrogen, phosphorus, and hazardous components from various types of wastewater, resulting in biomass production, including from municipal, industrial, agro-industrial, and livestock wastewaters (Udaiyappan et al., 2017; Srinuanpan et al., 2020). Because certain microalgae species have evolved to survive in wastewater, this strategy can reduce manufacturing costs by combining wastewater treatment with the cultivation of microalgae. Thus, including microalgae-mediated CO2 bio-mitigation into a wastewater treatment infrastructure may be more affordable, cost-effective, and ecofriendly (Basu et al., 2014).
There have been recent advancements in the use of microalgae for environmental issues such as coupling with wastewater treatment or CO2 absorption. Only a limited number of studies have addressed the advantages and limitations of microalgal production in terms of green economy purposes. The composition of wastewater varies according to its source. It is critical to consider the ability of certain algae to grow in various types of contaminated wastewater. As a consequence, this review will involve wastewater from a variety of sources, such as municipal, agricultural, and industrial waste, that have been employed in algae growth experiments to create high-value bioactive chemicals in combination with wastewater treatment. The approach has the potential to incorporate CO2 reduction, bioenergy generation, and other high-value-added compounds produced by microalgae. The performance of several types of wastewater for microalgae culture is rigorously examined and assessed. This review also describes several microalgae potentials and future development trends for resource recovery. Furthermore, recent developments in the enhancement of CO2 collection by microalgae are reported in this review.
2 Green economy framework
Throughout the last several decades, the world economy has expanded at a dramatic pace. Extreme population expansion is a major issue, with the world’s population forecast to reach 8.5 billion in 2030, 9.7 billion in 2050, and 10.9 billion by 2100 (United Nations, 2019). As a consequence of the growing global population, large amounts of energy and resources have been consumed and pollution levels are high. The necessity of anticipating and preparing for these crises has been recognized and appreciated by many international organizations. Promoting a “green economy” is one such plan. The green economy concept was developed during the 2012 United Nations Conference on Sustainable Development in Rio de Janeiro, and it is based on the idea that environmental protection helps both the economy and society. The goal of this concept is to empower farmers and manufacturers to create more environmentally friendly production and consumption systems based on reuse and recycling for sustainable development (Loiseau et al., 2016).
3 Wastewater integrated algae-biorefinery for high-value compounds production
Because of the efficiency of being a bio-refinery of algae, interest in wastewater integrated algal-biorefinery has recently attracted considerable attention. Many previous studies indicate that several wastewaters (e.g., domestic, agricultural, and industrial wastewater) are rich in appropriate nutrients which could serve as inexpensive alternative raw nutrients source to cultivate microalgae using CO2 from atmospheric and flue gases (Wollmann et al., 2019; Chew et al., 2021; Li et al., 2022). The considerable advantage of wastewater integrated microalgae-based biorefinery is that it both solves environmental problems and also produces biofuel as well as other value-added compounds such as pigments, microelements, omega fatty acids, antioxidants, and animal feed (Kadir et al., 2018; Xiaogang et al., 2020; Chew et al., 2021).
The importance of microalgae as a rich source of novel bioactive compounds is quickly becoming recognized, as evidenced by the number of research studies on the topic. A significant number of vital compounds such as fatty acids, pigments, and other biochemicals are found in the composition of microalgae, resulting in microalgae becoming increasingly important (Bhattacharya and Goswami, 2020). A lot of biomolecules produced by microalgae have a diverse range of applications. Many different types of molecules can be utilized for a variety of different purposes, ranging from the use of lipids, proteins, and carbohydrates in food and nutraceutical applications to the use of pigments and sterols in cosmetic and pharmaceutical applications. To facilitate this review, the compounds isolated from microalgae are illustrated in Figure 1 with respect to four different domains of nutrition: carbohydrate, protein, lipid and pigment. According to global microalgae market data, microalgae pigments and lipid are two of the most prominent microalgae products on the market. The green alga Dunaliella salina, which thrives in open ponds with high salinity and light, is the source of the pigment β-carotene, which was created in massive quantities in 2010 (Enzing et al., 2014). The freshwater green alga Haematococcus pluvialis produces the commercially important pigment astaxanthin (Shah et al., 2016). Astaxanthin has a larger market value than β-carotene, despite its lower output. However, astaxanthin’s commercialization has been slow due to high production costs and the lack of a recognized market for human usage (Borowitzka, 2013). In the last decade, microalgae have become a third-generation biofuel feedstock due to their high triglyceride (TAG). However, the majority of energy is derived from nonrenewable fossil fuels such as coal, petroleum oil, and natural gas. The demand for energy is always increasing because of rapid population growth and industrialization. Consequently, the search for alternate energy sources has piqued the public’s interest. The potential use of algae as a source of renewable energy has inspired considerable interest due to a number of key advantages, including a rapid growth rate, high lipid content, and the capacity to grow without the use of arable land. Furthermore, algae can efficiently take CO2 during photosynthesis and create polysaccharides and triacylglycerol (TAG) (Wijffels and Barbosa, 2010). These compounds are the starting materials for the synthesis of bioethanol and biodiesel, both of which may be used in existing engines without major changes. Details of these products are discussed in the next topic.
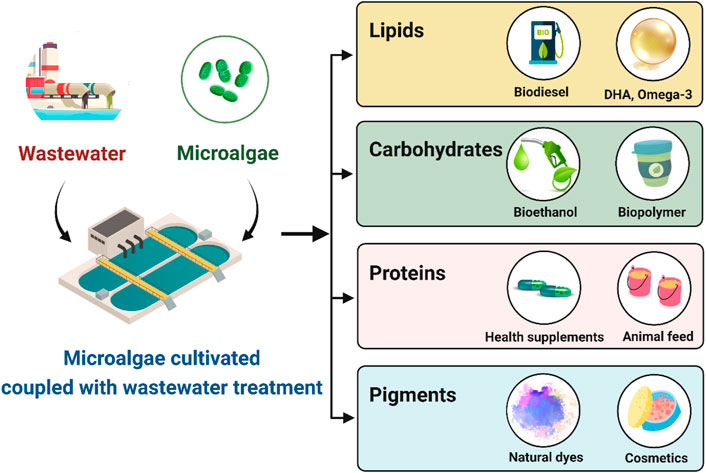
FIGURE 1. Wastewater integrated algal-biorefinery for biofuel and other value-added compound productions (created with BioRender.com).
3.1 Biofuel production
Microalgae have a capacity as a producer comparable to that of a land plant, which has been critical to human survival for a long time in human history in terms of significant sources of food, medicine, building materials, and energy supply. Diverse photosynthetic unicellular microalgae are emerging as novel sources of renewable energy that can fulfill the demands of the human activities. Microalgae lipids can be utilized as a raw material for biodiesel synthesis, and remaining biomass rich in carbohydrates can be used to produce bioethanol or biogas. Furthermore, using various thermochemical conversion processes, the whole biomass may be turned straight into crude bio-oil (Wang et al., 2022). Microalgae typically accumulate lipids between 20–50% of their dry weight. Some species can be as high as 80% under certain condition (Chisti, 2007). These neutral lipids, mainly in the form of triacylglycerols (TAGs) (up to 90–95%), which can be transformed to fatty acid methyl esters (FAMEs) and converted biodiesel (MacDougall et al., 2011). Algae have advantages over first generation biofuels made from sugar, starch, and vegetable oil because of their high growth rates and productivity, ability to grow on non-arable land using wastewater, ability to use water contaminants and CO2, and ability to produce a variety of high-value biological compounds (Cheng and Luo, 2022). In 2016, 135 billion gallons of biofuels (biodiesel and ethanol) were produced globally, accounting for 4% of total transportation fuel use. Biodiesel and ethanol are alternatives for petroleum diesel and gasoline, respectively, and have many advantages over petroleum, including lower CO2 emissions, lower manufacturing costs, and more sustainability (Kushwaha et al., 2022). In the global scenario, unpredictable energy prices are projected to persist due to rising demand from fast growing global economies, limited crude oil reserves, and political upheaval in crude oil producing countries, which can create significant supply disruption, speculation, and so on. This insecurity has the potential to affect the entire economy of the country and the world. As a result, liquid biofuels are now seen as an unavoidable development in the field of renewable energy. Table 1 shows several studies of microalgal growth coupled with wastewater treatment for biodiesel, bioethanol, biogas, biohydrogen and other valuable compounds production.
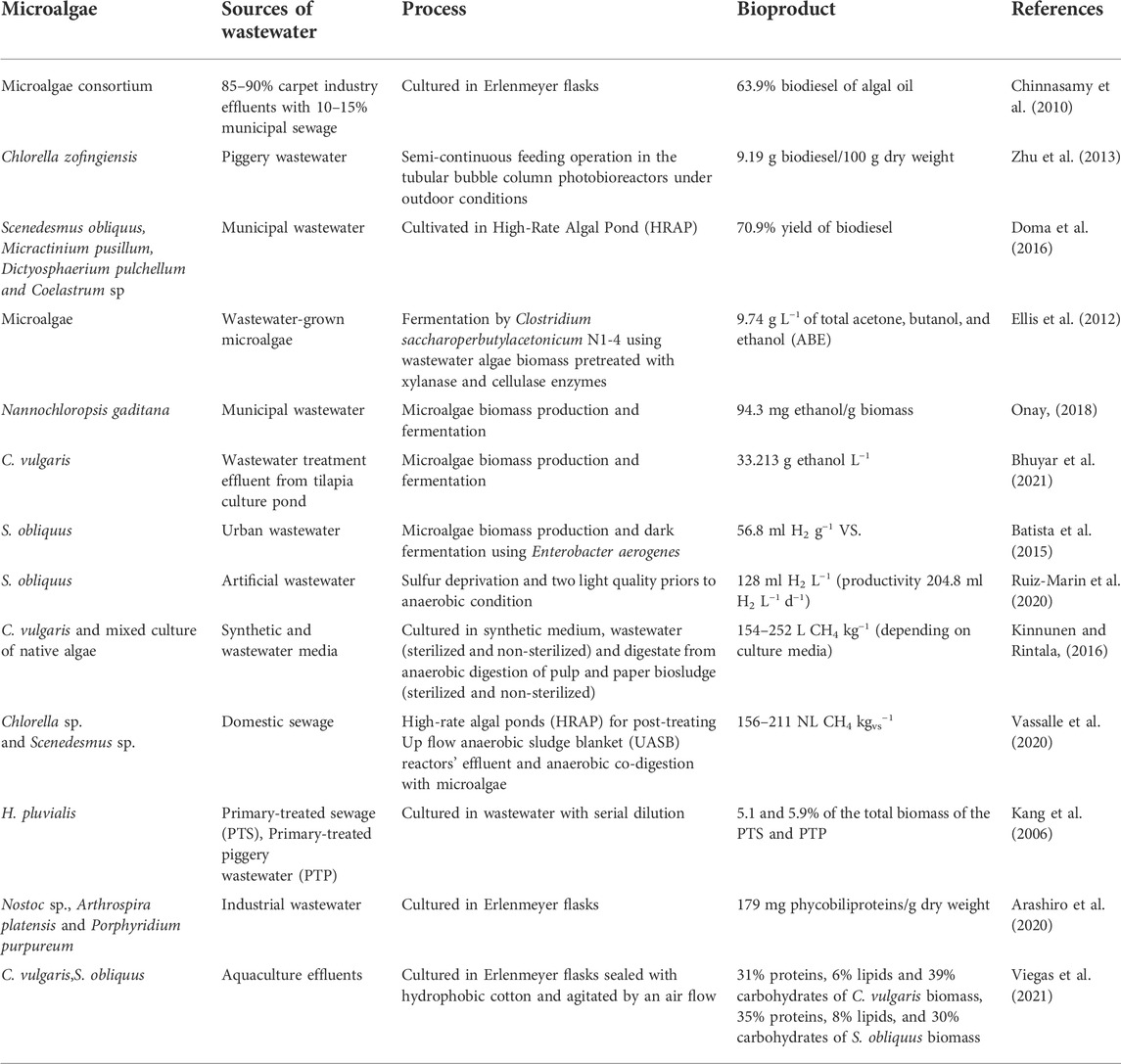
TABLE 1. Several studies of microalgae growth coupled with wastewater treatment for biofuel and other value-added compounds production.
3.2 Biodiesel
The production of biodiesel from microalgae comprises two different steps: 1) lipid extraction from microalgal cells and 2) transesterification of lipids using alcohol and a catalyst (Mondal et al., 2017). In a preliminary study of biomass production and ammonium removal in Synechococcus sp. VDW (accession number MH393765) isolated from natural seawater in Thailand (Tinpranee et al., 2018), we discovered that at optimum conditions (initial pH 7.4, inoculum size of 0.17 (OD730), and ammonium concentration of 10.5 mg L−1), maximum ammonium removal and biomass productivity were 95% and 34 mg L−1d−1, respectively. Furthermore, fatty acid methyl ester (FAME) analysis revealed that the major fatty acids were palmitic acid (C16:0), linoleic acid (C18:2 n6 cis), palmitoleic acid (C16:1), and oleic acid (C18:1 n9 cis), accounting for more than 80% of total fatty acids, indicating that this strain has potential for simultaneous water treatment and biomass production for biofuel feedstock (Srimongkol et al., 2019b). A review study by Pancha et al. (2019) showed various microalgae lipid content cultivated in various wastewaters ranging from 18–79% w/w of biomass. Meanwhile, Chinnasamy et al. (2010) found that >96% nutrient in treated wastewater containing 85–90% carpet industry effluents with 10–15% municipal sewage could be removed by a range of native algal isolates. Biomass production potential and lipid content were ∼9.2–17.8 tons ha−1 year−1 and 6.82%, respectively. In addition, around 63.9% of algal oil could be converted into biodiesel. In addition, Zhu et al. (2013) reported the FAME yield of C. zofingiensis in piggery wastewater for outdoor simultaneous wastewater treatment was 9.19% of dw. Doma et al. (2016) showed the oil content of biomass collected from High-Rate Algal Pond (HRAP) constructed to treat municipal wastewater was 5% and the biodiesel yield was 70.9%.
3.3 Bioethanol
Microalgae can produce and accumulate an abundance of carbohydrates that are useful for bioethanol production (Maia et al., 2020). Generally, wastewater-grown microalgae require pretreatment to hydrolyze the complex sugars into simple and readily metabolizable carbon source by fermentative microorganisms (Pancha et al., 2019). Ellis et al. (2012) showed that 9.74 g L−1 acetone-butanol-ethanol (ABE) was produced by Clostridium spp. Using wastewater algae biomass as a carbon source. Onay (2018) demonstrated that bioethanol yields of Nannochloropsis gaditana in various concentrations of municipal wastewaters (0, 30, 60, and 100%) ranging from 70.3 ± 2.4 mg g biomass−1 to 94.3 ± 5.5 mg g biomass−1 and 30% of wastewater showed the highest bioethanol yield (94.3 ± 5.5 mg g−1 biomass). A recent study by Bhuyar et al., 2021 showed that C. vulgaris cultivation in wastewater effluent from tilapia culture pond produced biomass of 0.376 ± 94.21 mg L−1 after cultivation and produced the highest ethanol concentration of 33.213 g L−1 after 96 h of fermentation.
3.4 Biohydrogen
Biohydrogen production from microalgae can take place through in different routes but generally involves fermentation biohydrogen production (e.g., dark fermentation biohydrogen production, photo fermentation biohydrogen production, and photo-dark combined fermentation biohydrogen production) and photosynthesis biohydrogen production (e.g., direct biological photolysis biohydrogen production, indirect biological photolysis biohydrogen production) (Wang et al., 2021). Batista et al. (2015) reported that S. obliquus can grow in urban wastewater and then the biomass can be converted into biohydrogen through dark fermentation by Enterobacter aerogenes producing 56.8 ml H2 gvs−1. Ruiz-Marin et al. (2020) demonstrated that microalgae C. vulgaris and S. obliquus immobilized cells grown in urban wastewater can produce biohydrogen in sulfur deprivation with a maximum hydrogen production of 128 ml H2 L−1 (productivity 204.8 ml H2 L−1 day−1) and 60.4 ml H2 L−1 (productivity 39.18 ml H2 L−1 day−1) for S. obliquus and C. vulgaris, respectively.
3.5 Biogas
Biogas is the end product of anaerobic digestion, which produces gas composed of 50–70% of methane, 30–45% of carbon dioxide, < 2% of hydrogen, and <3.5% of hydrogen sulfide (Vanegas and Bartlett, 2013; Milledge et al., 2019). Generally, anaerobic digestion is conducted by two processes: 1) simple sugar is fermented by fermentative bacteria and converted into alcohols through anaerobic digest and 2) metanogenic microorganisms use these compounds and synthesize biomethane (Danquah et al., 2011). Choudhary et al. (2016) revealed that native consortia PA6 has good nutrient removal ability from rural wastewaters with a theoretical methane potential of up to 0.79 m3 kg−1 VS. Kinnunen and Rintala (2016) showed that the biomethane potential of C. vulgaris and mixed culture of native algae species (dominating by Scenedesmus sp.) varied between 154 and 252 L CH4 kg−1 VS. depending on culture media including synthetic medium, wastewater (sterilized and non-sterilized), and digestate from anaerobic digestion of pulp and paper biosludge (sterilized and non-sterilized). A recent study by Vassalle et al. (2020) evaluated sewage treatment efficiency and biogas production of an up flow anaerobic sludge blanket (UASB) reactor followed by a high-rate algal ponds (HRAP) during a 1 year at demonstration–scale. Their result indicates that 65% COD and 61% N-NH4 were overall removed from the system. In addition, methane yield increased by 25% after anaerobic co-digestion with microalgae (156–211 NL CH4 kgVS−1).
3.6 Other valuable compounds
Despite intensive studies and several scientific initiatives, commercialization of microalgal biofuels has not yet been achieved. Since it is not economically feasible to devote resources to the production of a single microalgal product, researchers have found that combining the development of numerous products from a single batch of biomass is more efficient. Therefore, to produce biofuels, microalgal biomass can also be used for other purposes to produce other value-added compounds (Xiaogang et al., 2020). Several bioactive compounds have been discovered and purified from marine microalgae, including polysaccharides, pigments, and fatty acids (Ryckebosch et al., 2014). Some of these metabolites have demonstrated biological activities, including anti-oxidant, anti-inflammatory, anti-cancer, and anti-viral properties (Table 2). Among them, the most abundant components are proteins that play an important role in the structure and metabolism of microalgal cells. They are an essential component of the membrane and light-harvesting complex, which contains a large number of catalytic enzymes that are involved in the photosynthesis process. In terms of quantity, several species of microalgae have been reported to contain extremely high concentrations of protein; on a dry weight basis, these concentrations can range from 42% to over 70% in certain cyanobacteria and up to 58% in C. vulgaris, according to the literature (Hachicha et al., 2022). Microalgae are high in nutritional value because they contain all of the essential amino acids that mammals are unable to produce. Recently, peptides derived from the enzymatic hydrolysis of various dietary proteins have been shown to have a wide range of bioactivities, and there have been numerous papers on anti-oxidants and anti-cancer properties (Sheih et al., 2010; Ko et al., 2012; Zhu et al., 2017; Suttisuwan et al., 2019a; Suttisuwan et al., 2019b; Moaveni et al., 2022). For example, Angiotensin-converting enzyme (ACE) inhibitory and antioxidant properties from microalgae C. vulgaris were reported by Sheih et al., 2010) and they then discovered that the peptide fraction isolated from pepsin hydrolyzed algae protein waste had strong dose-dependent antiproliferation and induced post-G1 cell cycle arrest in human gastric cancer cell lines AGS. Carbohydrates, which include monosaccharides, oligosaccharides, and polysaccharides, perform structural and metabolic activities. In particular, an exopolysaccharide derived from microalgae secretions has attracted considerable attention due to its ease of extraction and isolation from the medium, which saves considerable time and energy. Exopolysaccharides are a highly valuable class of chemicals which have been demonstrated to possess immunomodulatory, anticoagulant, antimutagenic, antibacterial, radioprotective, anticancer, and anti-inflammatory bioactivities (Yim et al., 2003; Kanekiyo et al., 2005; Ngatu et al., 2012; Bafana, 2013). Glycosyllipids and triacylglycerols are two examples of microalgal lipids that can be classified as either polar or neutral. Cell membranes and organelles mostly contain polar lipids, while glycerol and unsaturated fatty acids (UFAs) are energy-storing molecules (Lupette and Benning, 2020). The amount of lipids in microalgae is affected by the type of microalgae, the amount of light, the growing environment, and the temperature. Despite these differences, microalgae are a significant source of polyunsaturated fatty acids (PUFAs), including omega-3 fatty acids, docosahexaenoic (DHA), and eicosapentaenoic (EPA). These UFAs have also been shown to have antioxidant capabilities, to reduce hypertension and to have immune-regulating qualities (Zhou et al., 2022). As a result, a number of microalgae, such as Schizochytrium (for DHA), Nannochloropsis (for EPA), Isochrysis, Nannochloropsis, Phaeodactylum, Pavlova. and Thalassiosira (for omega-3), have potential as fish oil substitutes for vegetarians, vegans, and those who dislike to the taste of fish. Furthermore, pigments found in microalgae such as astaxanthin, β-carotene, phycocyanin, lutein, and violaxanthin have anti-oxidant, anti-cancer, and anti-inflammatory properties (Dufosse et al., 2005; Talero et al., 2015; Chen et al., 2016; Khoo et al., 2019; Prabakaran et al., 2020). Kang et al. (2006) reported that astaxanthin content accounted for about 5.1 and 5.9% of the total biomass of H. pluvialis cultivation in primary-treated sewage (PTS) and primary-treated piggery wastewater (PTP), respectively.
4 Wastewater in an alternative growth media
Microalgal-based wastewater treatment is possible by using wastewater as a source of nutrients for microalgae growth, which promotes the concept of a circular economy and increases the sustainability of the process. In recent years, many types of wastewater have been employed to develop algal biomass for phytoremediation purposes. Wastewater is classified into different categories based on its source: municipal wastewater (produced by rural and urban households), agricultural wastewater (produced by crop cultivation, livestock breeding, agricultural product processing, and so on), and industrial wastewater (produced by various industries), as discussed in the subsection below. Table 3 summarizes the wastewater supply, nutrient removal potential, eventual biomass concentration, growing system, and production rate for each study.
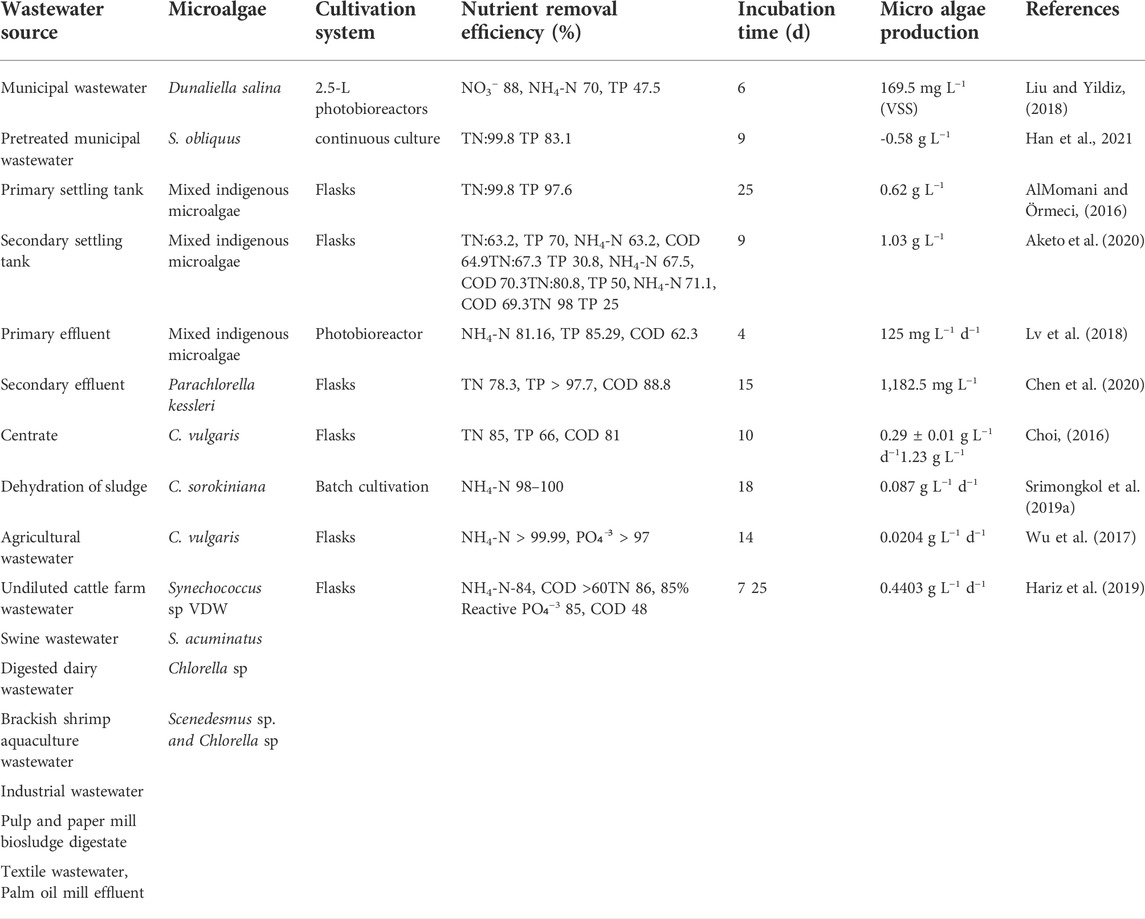
TABLE 3. Role of microalgae in different types of wastewaters in terms of nutrient removal efficiency, incubation time, and microalgae production.
4.1 Composition of wastewater for microalgae cultivation
For more than 30 years, environmental concerns regarding biological and chemical water pollution have been a major focus for society, business, and government (Crini and Lichtfouse, 2019). Vast amounts of wastewater are produced which can contain harmful contaminants. Wastewater often contains substantial amounts of organic and inorganic nutrients, which generate ecological imbalances owing to their high biological oxygen demand (BOD) and chemical oxygen demand (COD). Excess nutrients, particularly nitrogen (N) and phosphorus (P), create water eutrophication, which is one of the world’s most difficult environmental concerns (Yang et al., 2008). This phenomenon causes environmental concerns such as solid waste and by-product generation, undesirable product emissions into the air, excessive growth of undesirable microbes endangering aquatic life forms, and groundwater contamination, which contribute to widespread health-related problems in areas near the discharge range (Amenorfenyo et al., 2019). It is vital to treat wastewater to reduce environmental contaminants (Rasoul-Amini et al., 2014). Wastewater treatment provides necessary protection for the sustainability of urban environment because it is a key part of global water circulation. The major objective of wastewater treatment is to markedly remove contaminating that are implanted in the water such as carbonaceous (organic; predominantly determined as biological oxygen demand (BOD)) materials, nitrogen (N), and phosphorus (P) compounds prior to being discharged into receiving systems (Grady et al., 2011; Peter et al., 2021).
Using microalgae in wastewater treatment is a sustainable option that has been widely studied for over 50 years in terms of microalgal production of useful chemical compounds, such as biofuels, as well as wastewater treatment, because it can efficiently convert carbon dioxide (CO2) into biofuel products and chemical substances without generating pollution and can lead to a reduction in greenhouse gas emissions. Furthermore, these procedures outweigh the disadvantages of traditional wastewater treatment, such as high operational costs and the generation of secondary waste from chemical operations (Rasoul-Amini et al., 2014; Srimongkol et al., 2019a; Aketo et al., 2020; Chai et al., 2021). It is critical to treat all forms of wastewaters in order to decrease pollutants in the environment (Rasoul-Amini et al., 2014). Wastewater composition can be greatly influenced by different wastewater generating methods and disposal systems (Bhatia et al., 2021). The composition of wastewater has a significant impact on the development of microalgae, the rate of pollutant clearance, and the creation of various intracellular compounds (carbohydrate, protein, and lipid). The carbon source, organic or inorganic carbon, macronutrients, nitrogen, phosphorus, micronutrients, vitamins, and trace elements in wastewater all have an effect on the capacity of microalgae to remove pollutants and thrive (Ahmad et al., 2022; You et al., 2022). The wastewater widely utilized for microalgae production documented in the literature may be classified according to its source, which includes municipal, agricultural, and industrial wastewater (Chiu et al., 2008; Liu and Hong, 2021).
4.2 Municipal wastewater
Municipal wastewater or domestic wastewater is defined as wastewater discharged from houses, kitchens, bathrooms, and laundry rooms (REF). In comparison to several types of wastewater, municipal wastewater composes of lower levels of N (15–90 mg L−1), P (5–20 mg L−1) and typically has a low level of COD concentration (less than 300 mg L−1) (Scott et al., 2012; You et al., 2022) and is often suitable for microalgae-based wastewater processes. Municipal wastewaters generally have a low COD concentration (less than 300 mg L−1) which why municipal wastewater has been the more commonly used and studied in recent decades (Li et al., 2008). There are four categories of municipal wastewater used for cultivation of microalgae, including raw sewage, which is municipal wastewater prior primary settling, primary sewage, which is wastewater after primary settling, secondary sewage, which is wastewater after treating with activated sludge in the aeration tank, and centrate, which is the by-product of sludge dewatering containing high amounts of nutrients (Li et al., 2008; Liu and Hong, 2021; You et al., 2022).
The growth and purification capabilities of C. vulgaris, Neochloris oleoabundans, and the mixed microalgal in primary sewage, secondary sewage, and centrate were investigated by AlMomani and Örmeci (2016). The results demonstrated that their growth rates differed in different types of wastewater, and the mixed indigenous microalgae showed better wastewater purification capability than C. vulgaris, N. oleoabundans. Moreover, Han et al. (2021) demonstrated that S. obliquus showed a vital role in removing nitrogen and phosphorus from the wastewater from primary and secondary settling tanks. The results showed that total nitrogen (TN) from primary and settling tank wastewater were 99.8 and 98.9%, respectively. Meanwhile, total phosphorus (TP) from primary and settling tank wastewater were 83.1 and 97.6%, respectively. Moreover, the total lipid yields of S. obliquus for 10 days cultivation in wastewater from the primary and secondary settling tanks were 0.38 and 0.33 g L−1, respectively which were higher than those found in the other literature. In addition, to improve the removal efficiency of nutrients in municipal wastewater using micro algae, many studies found that adding high concentration of CO2 (5–15%) can induce the nutrient removal and improve growth and lipid production by microalgae (Li et al., 2008; Lima et al., 2020; Liu and Hong, 2021). The nutrient composition in different municipal wastewaters should be considered as an important factor for microalgae treatment. The ratio and the nutrients concentration should be balanced by mixing different categories of wastewater to meet wastewater discharge standards. The influence of emerging pollutants on the performance of microalgae treatment should also be considered (You et al., 2022).
4.3 Agricultural wastewater
Agricultural wastewater is wastewater discharged from the process of crop cultivation, livestock breeding, and agricultural products processing, including farmland drainage wastewater and animal manure wastewater, (Liu and Hong, 2021; You et al., 2022). Several studies have presented the benefits of agricultural product processing wastewater as a medium for microalgae cultivation, such as potato processing wastewater, palm oil mill effluent, starch processing wastewater, and swine wastewater (You et al., 2022). Agricultural wastewaters, especially animal manure wastewaters, have high nutrient concentrations and so are great sources for nutrient recovery (Li et al., 2019). Agricultural wastewaters from animal manure wastewater have high nutrient concentrations, high turbidity, and high insoluble organic compound concentrations, and there are very limited algae species used in the animal wastewater treatment (Li et al., 2019; Liu and Hong, 2021). For example, piggery wastewater generally has N/P ratio of 12–17, a total nitrogen level of 800–2300 mg L−1, and total phosphorus levels of 50–230 mg L−1 (Beuckels, et al., 2015). However, contaminants with high turbidity can block light and reduce photosynthetic efficiency. Meanwhile, high amounts of ammonia nitrogen concentration can impede the growth of microalgae involving the electron transfer of photosystem II, making it unsuitable for microalgae cultivation. In addition, there are very limited algae strains used in the animal wastewater treatment (Zhou et al., 2012). Consequently, Therefore, agriculture wastewater is commonly diluted before algal-based treatments in order to reduce the turbidity and nutrient concentration. From Chen et al. (2020), the TN, TP and COD removal efficacies for C. sorokiniana strain AK-1 were 78.3 ± 1.4%, >97.7% and 88.8 ± 0.9%, respectively after 15 days of cultivation in 10% diluted swine wastewater augmented with BG11 medium.
4.4 Industrial wastewater
Industrial wastewater includes pulp and paper industry effluent, petroleum industrial wastewater, sugar mill effluent, coal-fired metal-contaminated wastewater, pharmaceutical industry wastewater, textile dye industry effluent, palm oil mill effluent (POME), electroplating industry wastewater, and agricultural machinery manufacturing industry wastewater (Wang et al., 2016; Udaiyappan et al., 2017). Wastewater generated from various industrial sections are composed of many types of contaminants such as heavy metals, antibiotics, oil and grease, and some other chemicals (Udaiyappan et al., 2017; Goswami et al., 2021; Liu and Hong, 2021). For example, Thailand is a major manufacturer and exporter of palm oil products. POME is wastewater generated from palm oil industry and is a known contaminant discharged into rivers in Southeast Asia. The concentrations of TN, NH3-N, BOD, and COD in POME are in the ranges of 180–1,400 mg L−1, 4–80 mg L−1, 10,250–43,750 mg L−1, and 15,000–100,000 mg L−1, respectively, with pH values in the range of 3.5–5.2 (Udaiyappan et al., 2017). Similarly, food processing industries which belonged to the industry section also contain high levels of TN, TP, BOD, COD, BOD, TN, and TP (Goswami et al., 2021).
Due to the aforementioned reasons, industrial wastewater is not appropriate for microalgae-based treatment based on the characteristics and properties of industrial wastewater reported by many previous studies. Only specific species of microalgae could be used to treat toxic heavy metals in wastewater through absorption and adsorption (Li et al., 2019; You et al., 2022). Microalgae, namely Cholorella and Scenedesmus have been proven as an effective species for treating olive oil and industry wastewater (Tao et al., 2017; Wu et al., 2017). Tao et al. (2017) conducted an experiment using pulp and paper mill biosludge digestate for cultivation of S. acuminatus. The results showed that both micro algae can remove more than 99.99% of NH4-N and more than 96.9% of PO₄−3-P, with a biomass concentration of 2.9 g L−1. Moreover, Chlorella sp. was reported to be able to tolerate a high concentration of CO2 and convert it into biomass (Chiu et al., 2008). Meanwhile, Wu et al. (2017) demonstrated that Chlorella sp. Could grow using raw textile wastewater (Wu et al., 2017) and NH4+-N removal efficiency with aeration at 10% dilution rate and COD removal efficiency with aeration at 0% were 84% and >60%, respectively.
Industrial wastewaters are usually combined with anaerobic pretreatment or diluted suitably in order to avert the inhibition of algal growth caused by high COD concentrations since this type of wastewater contains some nutrient and high-COD as mentioned before (Wang et al., 2016). Hariz et al. (2019) used an integrated system of effluent treatment and CO2 fixation by Scenedesmus sp. UKM9 and Chlorella sp. UKM2. In this study, Scenedesmus sp. was used to treat POME in the first step. Then Chlorella sp. was used to treat the treated POME from the first step and capture carbon dioxide gas (CO2) in the second step. The results show that this system can remove 86% TN, 85% Reactive Phosphate (PO₄−3), and 48% COD, respectively, indicating a higher nutrient reduction in POME and greater CO2 fixed when compared to the individual treatment operation. Moreover, using molecular biology techniques is another way to enhance the expression of related enzymes in microalgae cells which can improve the effectiveness of wastewater treatment as well as biomass accumulation of microalgae (You et al., 2022).
As previously mentioned, heavy metals are a contaminant type present in industrial wastewater. Heavy metals are natural components of the Earth’s soil and crust that can be found in every ecosystem on Earth with a density greater than 5 g cm−3. Heavy metals are important for basic physiological and chemical for organisms such as plants and animals. However, some heavy metals can be poisonous to organisms. Heavy metals that are usually found in wastewater include arsenic (As), cadmium (Cd), copper (Cu), chromium (Cr), lead (Pb), manganese (Mn), Mercury (Hg), nickel (Ni), and zinc (Zn) (Ahmed et al., 2022). Microalgae show high capacities for metal biosorption. The advantages include quick uptake capability of metal, highly environmentally friendly, energy and time saving, low-cost reusable, reusable, faster growth rate compared with terrestrial plants, and nonhazardous waste generation (Kumar et al., 2015; Leong and Chang, 2020).
Heavy metals ions from wastewater can be taken up by microalgae cells through two processes, biosorption and bioaccumulation (Ahmed et al., 2022). The first mode of heavy metals uptake is referred as bioaccumulation, in which heavy metal ions are transported across the cell membrane by different systems including active and passive transport systems to be accumulated in the cells, with this process only occurring in living cells. Bioaccumulation is the slow intracellular positive diffusion and accumulation which is the mode by which heavy metals can pass to the cell of microalgae and across the cell membrane through the cell metabolic cycle. The major limitation of the process is the containment of organic carbon sources in nutritive medium for growth of the microorganism (Kumar et al., 2015; Jais et al., 2017; Kanamarlapudi et al., 2018).
The process of biosorption or rapid passive adsorption is called the independent metabolic process and occurs in living or dead cells. Heavy metal ions are attached in the cellular structure and absorbed onto the binding sites present in the cellular structure. The heavy metal ions are trapped and attached to the functional groups on the cell surface as a result of ion exchange, complexation, chelation, and micro-precipitation. The metal ions are trapped to the functional groups on the cell surface due to ion exchange, complexation, chelation, and micro-precipitation (Salam, 2019). This process is called the independent metabolic process and takes place in live or dead cells. Through ion exchange, the metal ions in the surrounding wastewater are exchanged with element ions held on the cell surface such as Ca, K and Na (Jais et al., 2017). During the process of passive biosorption, metal ions in the cationic form are physically adsorbed over the microalgal cell surface that contains functional groups like amino (-NH2), carboxyl (-COOH), hydroxyl (-OH), and sulfhydryl (-SH) (Ayele and Godeto, 2021). Several factors affect the biosorption process, including biomass concentration, initial metal concentration, and operational factors such as pH, temperature, concentration of the biosorbent, and concentration of the initial metal ion (Ayele and Godeto, 2021). However, the use of freely suspended microalgae as biosorbent has some limitations such as their small size, low mechanical strength, and the difficulty to separate the biomass and effluent (Salam, 2019).
5 Microalgae to couple wastewater treatment with microalgae for coupling CO2 fixation
According to the Global Energy Review 2021 by the International Energy Agency (IEA), global energy CO2 emissions are projected to rebound and increase by 4.8%, approaching the 2019 peak as fossil fuel demand rebounds (IEA, 2021). Global CO2 emissions is a critical global issue which is a primary driver of global warming through increased concentrations of greenhouse gases. Microalgae-mediated CO2 sequestration has been the subject of numerous research projects and has become one of the most promising strategies to reduce CO2 emissions. Microalgae can recycle CO2 into bioenergy through photosynthesis. Interestingly, CO2 sequestration by microalgae is an environmentally friendly and sustainable method (Brilman et al., 2013). Photosynthetic CO2 assimilation in microalgae consists of light-dependent and light-independent reactions. Through photosynthesis, microalgae can absorb CO2 emitted from various sources which is then converted into biomass and precursors of carbohydrates. These carbohydrates are then used to synthesize different biomolecules including lipids, proteins, and nucleic acids (Zeng et al., 2011; Zhang and Liu, 2021). A previous report stated that the cost of manufacturing Chlorella biomass is $4.87 kg−1, with an energy consumption of 0.96 kWh kg−1 of biomass (Valdovinos-García et al., 2020). Furthermore, 4,000 m3 of algae growth ponds might sequester up to 2.2 k tones of CO2 per year under natural daylight conditions (Stewart and Hessami, 2005). Another study found that 50 MW power plants might emit about 414 k tones of CO2 year−1, while a 1000-ha open raceway pond could absorb about 250 k tones of CO2 year−1. According to this study, algae might reduce CO2 emissions by 50% (Stepan et al., 2002).
5.1 Advantages of using microalgae for CO2 fixation
Compared to other photosynthetic organisms, microalgae have a large number of advantages for atmospheric CO2 capture. Microalgae cultivation systems can be tightly controlled and optimized, while its byproducts can be used to generate high value-added products due to their small size which allows them to be controlled in a closed system. They also have a higher growth rate and CO2 fixation capacity which results in a very high energy conservation efficiency (Paul et al., 2021). Based on their simple cell structure and high volume-to-area ratio, microalgae have a greater growth rate and CO2 fixation capacity than terrestrial plants. It has been reported that microalgae can grow 100 times faster and are able to fix CO2 while capturing solar energy with an efficiency that is 10–50 times greater than that of terrestrial plants (Singh and Ahluwalia, 2013; Zhang and Liu, 2021). Tsai et al. (2017) measured the maximum photosynthetic CO2 uptake rates in natural algae species, which had microalgae and green algae as the dominant species cultivated in two high-rate ponds (HRP). The maximum CO2 uptake rates of reactor 1 and reactor 2 were 36,299 mg m−2 d−1 and 48,829 mg m−2 d−1, respectively. Moreover, they found that the CO2 uptake rate of algae in reactor 1 was potentially higher than those of terrestrial plants which were calculated from the literature data. e.g., oak afforestation in Denmark, 31 years (2,371 mg m−2 d−1), Norway spruce afforestation in Germany and Italy, 93–112 years (2,763 mg m−2 d−1), and white pine afforestation in Rhode Island, United States, 115 years (2,110 mg m−2 d−1).
Another point of interest for CO2 sequestration using microalgae is their powerful environmental flexibility since they can tolerate and adapt to various extreme environmental conditions (Moreira and Pires, 2016; Onyeaka et al., 2021). Consequently, microalgae can play an important role in removing contaminants from wastewater from industries and agricultural activities that simultaneously produce high value-added products resulting in economic feasibility (Razzak et al., 2017; Paul et al., 2021). For instance, Yang et al. (2018) stably cultivated Monoraphidium dybowskii LB50 under semi-continuous culture with open raceway ponds in a desert area for 3 years, which corresponded to 30% of lipid content, 18,000 mg m−2 d−1 of biomass productivity, and a 33,000 mg m−2 d−1 CO2 fixation rate.
5.2 Development and current CO2 sequestration using microalgae
During the 1970’s, the U.S. Department of Energy (DOE) began research on microalgal wastewater treatment, and the recovered microalgal biomass was used for methane production. Next, a program named the “Aquatic Species Program” (ASP) funded by the U.S. Department of Energy’s Office of Fuels Development (DOE-OFD) was initiated to evaluate the potential of biodiesel production from high lipid-content algae grown in ponds, utilizing waste CO2 from coal fired power plants (Sheehan et al., 1998). This program achieved two major successes, including the institution of a microalgae culture collection center and a pilot-scale microalgae cultivation of two raceway ponds in New Mexico. During the 1990s, a research and development program in Japan costing over $250 million was implemented. This project was also related to bio fixation of CO2 and greenhouse gas emission abatement using closed microalgae photobioreactors (PBRs). However, it was later discontinued owing to the high costs associated with the reactors. In addition, the US DOE-NETL promoted microalgae research and development using closed PBRs. Moreover, other international participants consisting of Arizona Public Services, ENEL ProduzioneRicerca, EniTecnologie, ExxonMobil, and Rio Tinto have also participated in microalgae-based CO2 mitigation research (Bhola et al., 2014; Prasad et al., 2021). Furthermore, several other microalgae-based CO2 sequestration research projects are operating worldwide. The projects are mainly based on reducing the operational costs of carbon sequestration by using waste resources for algae biomass and a variety of value-added bioproducts production. Table 4 illustrates microalgae-based CO2 fixation research under various cultivation systems. However, most research and developmental activities on CO2 sequestration using microalgae is currently at the laboratory phase. Techniques to enhance microalgae cultivation for pilot-scale carbon capture are required which should focus on achieving higher biomass productivities, culture stability over long periods of time, economical harvesting methods, and improved biomass-to-fuels transformation technologies (Prasad et al., 2021).
6 Challenges and future perspective
Microalgae are a third-generation feedstock and a promising starting material for bio-renewables production that can compensate for fossil fuel and the first generation of biofuel that comes from corn, soy, and sugar cane. Nowadays, the combination of microalgae and clean technology such as biorefineries and biofuel production can release almost zero waste into the environment (Wang et al., 2022). Although CO2 sequestration using microalgae has an environmental benefit, it is limited by the high expense of CO2 capture and transport, as well as significant CO2 losses during microalgae culture. This constraint may result in high manufacturing costs. The normal compression process flow through the pipeline requires a lot of energy and raises transport expenses. Economic analyses revealed that combining the supplementation of the two carbon sources significantly reduced the cost of carbon procurement, dropping from $1.37 kg−1 when using 1% (v/v) CO2 alone as the carbon source to $0.86 kg−1 when using 1% (v/v) CO2 together with NaHCO3 (0.5 g L−1) while increasing the yield of FAME by nearly 80%. These results suggest that mixing CO2 with NaHCO3 is a more cost-effective way to supply carbon to microalgae for biodiesel production (Nayak et al., 2018). In addition, there are some gaps that must be considered as an environmental point for the further progress of larger-scale production. For instance, ammonia (NH3) is an inorganic fertilizer that is used to attain an appropriate algal biomass growth rate and productivity. For every 1 kg of NH3 generated, 1.2 kg of CO2 is released, resulting in environmental deterioration and the discharge of chemical reagents into the environment during the process of microalgae production. This issue must be considered as an important point to manage the whole microalgal production factory with clean energy to achieve environmental sustainability (Wang et al., 2022). The life cycle assessment (LCA) can be used to evaluate the environmental effects and energy concerns, which is an appropriate choice for future improvements in the environmental field (De Souza et al., 2019). According to a previous study, LCAs can be used to show potential ways to prove that applying microalgae contributes to positive feedback to the environment (Peter et al., 2021).
With regard to the economic concerns, using a microalgae system to remove nitrogen, phosphorus, and dissolved organic carbon from several types of wastewaters is far more sustainable than conventional systems because a microalgae system can operate outside in sunlight which serves to reduce costs (Mohsenpour et al., 2021). However, due to the open nature of open systems, additional bacteria may contaminate the culture. Contamination with other microorganisms could be a limitation since this process is conducted in non-sterile conditions, leaving only a few strains that are sufficiently resistant, fast growing, and tolerant of extreme conditions that can be grown in open reactors. A potential solution to this problem is using co-culture between microalgae and other microorganisms i.e., bacteria, yeast, and fungi to accelerate wastewater treatment (Mohsenpour et al., 2021). Consequently, the role of co-culture requires further exploration in wastewater scales with synthetic and real wastewater (Fazal et al., 2018). Further studies should investigate the interaction between microalgae and other microorganisms and study gene and transcription factors involved in the mechanism of microorganisms in wastewater. Omics approaches using targeted genome editing such as clustered regularly interspaced short palindromic repeats (CRISPR) to develop an effective solution to improve the future of microalgae for value added bioproducts in more commercial and potential is highly recommended (Kumar et al., 2021).
Another important challenge is that the composition of nutrients included in various municipal wastewaters should be taken into account when treating microalgae. The ratio and concentration of nutrients should be balanced by mixing different types of wastewaters in order to meet wastewater discharge guidelines (Ahmad et al., 2022). Besides the cost of algal biomass generation, post-cultivation biomass recovery can account for up to 30% of the total production expenses. Microalgae cells are tiny (2–20 µm) and have a density similar to water, which is one of the primary reasons for this. There are many challenges in large-scale agriculture, including efficient biomass recovery. Due to the large amount of energy that is needed, another problem that arises is the removal of microalgae from wastewater produced by the many different industrial wastewater treatment procedures (Amenorfenyo et al., 2019). Recent research indicates that the concentration of microalgal biomass will require a two-stage process: the utilization of flocculation in combination with sedimentation. Bio-flocculation is the better choice between these two methods because it cuts down on the costs of chemicals while keeping the efficiency of the biomass (Barros et al., 2015; Alam and Wang 2019). However, there are very limited research focusing on economic evaluation. The economical practicability of the process in real wastewater treatment under operation are still required for further study.
Additional carbon sequestration research on microalgae should be focused on the factors that influence the growth of microalgae, such as their wide range of tolerance and sensitivity to temperature, pH, irradiance and nutritional conditions, where even minor changes in cultivation conditions can have a significant impact on product yields. Additionally, carbon sequestration research on microalgae should focus on aspects affecting their growth, such as their wide range of tolerance and sensitivity to temperature, pH, irradiance, and nutritional circumstances, where even minor changes in production conditions affect product output (Molazadeh et al., 2019). Using modeling to assist the optimization of in the further studies is an alternative choice. Finally, the durability and economic viability of large-scale microalgae carbon sequestration are contingent upon a thorough knowledge of the photosynthetic process, the improvement of growth factors, and the development of technical infrastructure.
7 Conclusion
In summary, microalgae are photosynthetic microorganisms that play a vital role in the bioremediation of several types of wastewaters, including removal of N, P, and C, the reduction of BOD, as well as heavy metal removal. The integration of microalgae into several types of wastewaters can decrease the cost of wastewater treatment, obtain a lower footprint in terms of energy consumption, and provide environmental sustainability compared to existing conventional wastewater treatment processes. It is highly vital to evaluate the environmental effects of large-scale use of microalgae bioenergy if it is to be developed into an alternative energy to reduce fossil fuel consumption. Moreover, integrated microalgal biorefinery not only solves environmental problems, but also acts as a producer which can produce high added-value bio compounds such as biofuel, biodiesel, and other valuable compounds.
Author contributions
PSr and AK, designed the concept for the review article and further interpret the literature in the relevant field. PSr, PSa, PSo and WW searched the database and wrote and finalized the manuscript. AK supervised the writing process. All authors have read and agreed the manuscript.
Funding
This review is supported by Ratchadapisek Somphot Fund for Postdoctoral Fellowship, Chulalongkorn University. The authors wish to express their sincere gratitude to the Thailand Science Research and Innovation (TSRI) Fund (CU_FRB640001_01_61_2), the Thailand Science Research and Innovation Fund Chulalongkorn University (CU_FRB65_bcg (34)_210_61_02), and the Center of Excellence on Medical Biotechnology (CEMB), S&T Postgraduate Education and Research Development Office (PERDO), Office of Higher Education Commission (OHEC), Thailand (SN-63–009–01) for their financial assistance, without which the completion of this study would not have been feasible.
Acknowledgments
The authors wish to express their gratitude to the Institute of Biotechnology and Genetic Engineering at Chulalongkorn University for supporting this work.
Conflict of interest
The authors declare that the research was conducted in the absence of any commercial or financial relationships that could be construed as a potential conflict of interest.
Publisher’s note
All claims expressed in this article are solely those of the authors and do not necessarily represent those of their affiliated organizations, or those of the publisher, the editors and the reviewers. Any product that may be evaluated in this article, or claim that may be made by its manufacturer, is not guaranteed or endorsed by the publisher.
References
Ahmad, S. F., Mofijur, M., Parisa, T. A., Islam, N., Kusumo, F., Inayat, A., et al. (2022). Progress and challenges of contaminate removal from wastewater using microalgae biomass. Chemosphere 286, 131656. doi:10.1016/j.chemosphere.2021.131656
Aketo, T., Hoshikawa, Y., Nojima, D., Yabu, Y., Maeda, Y., Yoshino, T., et al. (2020). Selection and characterization of microalgae with potential for nutrient removal from municipal wastewater and simultaneous lipid production. J. Biosci. Bioeng. 129 (5), 565–572. doi:10.1016/j.jbiosc.2019.12.004
Alam, M. A., and Wang, Z. (2019). Microalgae biotechnology for development of biofuel and wastewater treatment. Singapore: Springer Singapore.
Alami, A. H., Alasad, S., Ali, M., and Alshamsi, M. (2021). Investigating algae for CO2 capture and accumulation and simultaneous production of biomass for biodiesel production. Sci. Total Environ. 759, 143529. doi:10.1016/j.scitotenv.2020.143529
AlMomani, F. A., and Örmeci, B. (2016). Performance of Chlorella Vulgaris, Neochloris Oleoabundans, and mixed indigenous microalgae for treatment of primary effluent, secondary effluent and centrate. Ecol. Eng. 95, 280–289. doi:10.1016/j.ecoleng.2016.06.038
Amenorfenyo, D. K., Huang, X., Zhang, Y., Zeng, Q., Zhang, N., Ren, J., et al. (2019). Microalgae brewery wastewater treatment: Potentials, benefits and the challenges. Int. J. Environ. Res. Public Health 16, 1910. doi:10.3390/ijerph16111910
Arashiro, L. T., Boto-Ordóñez, M., Van, S. W. H., Ferrer, I., Garfí, M., and Rousseau, D. P. L. (2020). Natural pigments from microalgae grown in industrial wastewater. Bioresour. Technol. 303, 122894. doi:10.1016/j.biortech.2020.122894
Ayele, A., and Godeto, Y. G. (2021). Bioremediation of chromium by microorganisms and its mechanisms related to functional groups. J. Chem. 2021, 1–21. doi:10.1155/2021/7694157
Bafana, A. (2013). Characterization and optimization of production of exopolysaccharide from Chlamydomonas reinhardtii. Carbohydr. Polym. 95 (2), 746–752. doi:10.1016/j.carbpol.2013.02.016
Barahoei, M., Hatamipour, M. S., and Afsharzadeh, S. (2020). CO2 capturing by Chlorella vulgaris in a bubble column photo-bioreactor; Effect of bubble size on CO2 removal and growth rate. J. CO2 Util. 37, 9–19. doi:10.1016/j.jcou.2019.11.023
Barros, A. I., Gonçalves, A. L., Simões, M., and Pires, J. C. (2015). Harvesting techniques applied to microalgae: A review. Renew. Sustain. Energy Rev. 41, 1489–1500. doi:10.1016/j.rser.2014.09.037
Basu, S., Roy, A. S., Mohanty, K., and Ghoshal, A. K. (2014). CO2 biofixation and carbonic anhydrase activity in Scenedesmus obliquus SA1 cultivated in large scale open system. Bioresour. Technol. 164, 323–330. doi:10.1016/j.biortech.2014.05.017
Batista, A. P., Ambrosano, L., Graça, S., Sousa, C., Marques, P. A., Ribeiro, B., et al. (2015). Combining urban wastewater treatment with biohydrogen production-an integrated microalgae-based approach. Bioresour. Technol. 184, 230–235. doi:10.1016/j.biortech.2014.10.064
Beuckels, A., Smolders, E., and Muylaert, K. (2015). Nitrogen availability influences phosphorus removal in microalgae-based wastewater treatment. Water Res. 77, 98–106. doi:10.1016/j.watres.2015.03.018
Bhatia, S. K., Otari, S. V., Jeon, J. M., Gurav, R., Choi, Y. K., Bhatia, R. K., et al. (2021). Biowaste-to-bioplastic (polyhydroxyalkanoates): Conversion technologies, strategies, challenges, and perspective. Bioresour. Technol. 326, 124733. doi:10.1016/j.biortech.2021.124733
Bhattacharya, M., and Goswami, S. (2020). Microalgae–A green multi-product biorefinery for future industrial prospects. Biocatal. Agric. Biotechnol. 25, 101580. doi:10.1016/j.bcab.2020.101580
Bhola, V., Swalaha, F. M., Kumar, R. R., Singh, M., and Bux, F. (2014). Overview of the potential of microalgae for CO2 sequestration. Int. J. Environ. Sci. Technol. 11, 2103–2118. doi:10.1007/s13762-013-0487-6
Bhuyar, P., Trejo, M., Dussadee, N., Unpaprom, Y., Ramaraj, R., and Whangchai, K. (2021). Microalgae cultivation in wastewater effluent from tilapia culture pond for enhanced bioethanol production. Water Sci. Technol. 84 (10-11), 2686–2694. doi:10.2166/wst.2021.194
Borowitzka, M. A. (2013). High-value products from microalgae-their development and commercialisation. J. Appl. Phycol. 25, 743–756. doi:10.1007/s10811-013-9983-9
Brilman, W., Alba, L. G., and Veneman, R. (2013). Capturing atmospheric CO2 using supported amine sorbents for microalgae cultivation. Biomass Bioenergy 53, 39–47. doi:10.1016/j.biombioe.2013.02.042
Chai, W. S., Tan, W. G., Munawaroh, H. S. H., Gupta, V. K., Ho, S. H., and Show, P. L. (2021). Multifaceted roles of microalgae in the application of wastewater biotreatment: A review. Environ. Pollut. 269, 116236. doi:10.1016/j.envpol.2020.116236
Chen, C. Y., Hsieh, C., Lee, D. J., Chang, C. H., and Chang, J. S. (2016). Production, extraction and stabilization of lutein from microalga Chlorella sorokiniana MB-1. Bioresour. Technol. 200, 500–505. doi:10.1016/j.biortech.2015.10.071
Chen, C. Y., Kuo, E. W., Nagarajan, D., Ho, S. H., Dong, C. D., Lee, D. J., et al. (2020). Cultivating Chlorella sorokiniana AK-1 with swine wastewater for simultaneous wastewater treatment and algal biomass production. Bioresour. Technol. 302, 122814. doi:10.1016/j.biortech.2020.122814
Cheng, F., and Luo, H. (2022). Evaluating the minimum fuel selling price of algae-derived biofuel from hydrothermal liquefaction. Bioresour. Technol. Rep. 17, 100901. doi:10.1016/j.biteb.2021.100901
Chew, K. W., Khoo, K. S., Foo, H. T., Chia, S. R., Walvekar, R., and Lim, S. S. (2021). Algae utilization and its role in the development of green cities. Chemosphere 268, 129322. doi:10.1016/j.chemosphere.2020.129322
Chinnasamy, S., Bhatnagar, A., Hunt, R. W., and Das, K. C. (2010). Microalgae cultivation in a wastewater dominated by carpet mill effluents for biofuel applications. Bioresour. Technol. 101 (9), 3097–3105. doi:10.1016/j.biortech.2009.12.026
Chisti, Y. (2007). Biodiesel from microalgae. Biotechnol. Adv. 25, 294–306. doi:10.1016/j.biotechadv.2007.02.001
Chiu, S. Y., Kao, C. Y., Chen, C. H., Kuan, T. C., Ong, S. C., and Lin, C. S. (2008). Reduction of CO2 by a high-density culture of Chlorella sp. in a semicontinuous photobioreactor. Bioresour. Technol. 99 (9), 3389–3396. doi:10.1016/j.biortech.2007.08.013
Choi, H. J. (2016). Dairy wastewater treatment using microalgae for potential biodiesel application. Environ. Eng. Res. 21, 393–400. doi:10.4491/eer.2015.151
Choudhary, P., Prajapati, S. K., and Malik, A. (2016). Screening native microalgal consortia for biomass production and nutrient removal from rural wastewaters for bioenergy applications. Ecol. Eng. 91, 221–230. doi:10.1016/j.ecoleng.2015.11.056
Crini, G., and Lichtfouse, E. (2019). Advantages and disadvantages of techniques used for wastewater treatment. Environ. Chem. Lett. 17, 145–155. doi:10.1007/s10311-018-0785-9
Cuellar-Bermudez, S. P., Garcia-Perez, J. S., Rittmann, B. E., and Parra-Saldivar, R. (2015). Photosynthetic bioenergy utilizing CO2: An approach on flue gases utilization for third generation biofuels. J. Clean. Prod. 98, 53–65. doi:10.1016/j.jclepro.2014.03.034
Daneshvar, E., Wicker, R. J., Show, P. L., and Bhatnagar, A. (2022). Biologically-mediated carbon capture and utilization by microalgae towards sustainable CO2 biofixation and biomass valorization–A review. Chem. Eng. J. 427, 130884. doi:10.1016/j.cej.2021.130884
Danquah, M., Liu, B., and Harun, R. (2011). “Analysis of process configurations for bioethanol production from microalgal biomass,” in Progress in biomass and bioenergy production. Editor S. S. Shaukat (London: IntechOpen).
Davoodbasha, M., Edachery, B., Nooruddin, T., Lee, S., and Kim, J. W. (2018). An evidence of C16 fatty acid methyl esters extracted from microalga for effective antimicrobial and antioxidant property. Microb. Pathog. 115, 233–238. doi:10.1016/j.micpath.2017.12.049
De Souza, M. P., Hoeltz, M., Brittes Benitez, L., Machado, Ê. L., and de Souza Schneider, R. D. C. (2019). Microalgae and clean technologies: A review. Clean. –. Soil Air Water 47 (11), 1800380. doi:10.1002/clen.201800380
Desbois, A. P., Mearns-spragg, A., and Smith, V. J. (2009). A fatty acid from the diatom Phaeodactylum tricornutum is antibacterial against diverse bacteria including multi-resistant Staphylococcus aureus (MRSA). Mar. Biotechnol. 11, 45–52. doi:10.1007/s10126-008-9118-5
Doma, H. S., Abdo, S. M., Mahmoud, R. H., Enin, S. A. El., and Diwani, G. El. (2016). Production and characterization of biodiesel from microalgae cultivated in municipal wastewater treatment plant. Res. J. Pharm. Biol. Chem. Sci. 7 (2), 1912–1919.
Dufossé, L., Galaup, P., Yaron, A., Arad, S. M., Blanc, P., Murthy, K. N. C., et al. (2005). Microorganisms and microalgae as sources of pigments for food use: A scientific oddity or an industrial reality? Trends Food Sci. Technol. 16 (9), 389–406. doi:10.1016/j.tifs.2005.02.006
Eckstein, D., Künzel, V., and Schäfer, L. (2021). Global climate risk index 2021. Who suffers most from extreme weather events? Weather-related loss events in 2019 and 2000-2019. Bonn, Germany: Germanwatch.
Ellis, J. T., Hengge, N. N., Sims, R. C., and Miller, C. D. (2012). Acetone, butanol, and ethanol production from wastewater algae. Bioresour. Technol. 111, 491–495. doi:10.1016/j.biortech.2012.02.002
Enzing, C., Ploeg, M., Barbosa, M., and Sijtsma, L. (2014). Microalgae-based products for the food and feed sector: An outlook for Europe. JRC Sci. Policy Rep. 19–37. doi:10.2791/3339
Fazal, T., Mushtaq, A., Rehman, F., Khan, A. U., Rashid, N., Farooq, W., et al. (2018). Bioremediation of textile wastewater and successive biodiesel production using microalgae. Renew. Sustain. Energy Rev. 82, 3107–3126. doi:10.1016/j.rser.2017.10.029
Global Carbon Project 2020 (2021). Global carbon atlas. Availableat: http://www.globalcarbonatlas.org/en/CO2-emissions (Accessed November 15, 2021).
Goswami, R. K., Mehariya, S., Verma, P., Lavecchia, R., and Zuorro, A. (2021). Microalgae-based biorefineries for sustainable resource recovery from wastewater. J. Water Process Eng. 40, 101747. doi:10.1016/j.jwpe.2020.101747
Grady, C. P. L., Daigger, G. T., Love, N. G., and Filipe, C. D. M. (2011). Biological wastewater treatment. Florida: CRC Press.
Hachicha, R., Elleuch, F., Ben Hlima, H., Dubessay, P., de Baynast, H., Delattre, C., et al. (2022). Biomolecules from microalgae and Cyanobacteria: Applications and market survey. Appl. Sci. (Basel). 12, 1924. doi:10.3390/app12041924
Han, W., Jin, W., Li, Z., Wei, Y., He, Z., Chen, C., et al. (2021). Cultivation of microalgae for lipid production using municipal wastewater. Process Saf. Environ. Prot. 155, 155-165. doi:10.1016/j.psep.2021.09.014
Hariz, H. B., Takriff, M. S., Yasin, N. H. M., Ba-Abbad, M. M., and Hakimi, N. I. N. M. (2019). Potential of the microalgae-based integrated wastewater treatment and CO2 fixation system to treat Palm Oil Mill Effluent (POME) by indigenous microalgae; Scenedesmus sp. and Chlorella sp. J. Water Process Eng. 32, 100907. doi:10.1016/j.jwpe.2019.100907
International Energy Agency (IEA) (2021). Global energy review 2021. Availableat: https://www.iea.org/reports/global-energy-review-2021 (Accessed February 28, 2022).
Jais, N. M., Mohamed, R. M. S. R., Al-Gheethi, A. A., and Hashim, M. K. (2017). The dual roles of phycoremediation of wet market wastewater for nutrients and heavy metals removal and microalgae biomass production. Clean. Technol. Environ. Policy 19 (1), 37–52. doi:10.1007/s10098-016-1235-7
Jin, X., Gong, S., Chen, Z., Xia, J., and Xiang, W. (2021). Potential microalgal strains for converting flue gas CO2 into biomass. J. Appl. Phycol. 33, 47–55. doi:10.1007/s10811-020-02147-8
Kadir, W. N. A., Lam, M. K., Uemura, Y., Lim, J. W., and Lee, K. T. (2018). Harvesting and pre-treatment of microalgae cultivated in wastewater for biodiesel production: A review. Energy Convers. Manag. 171, 1416–1429. doi:10.1016/j.enconman.2018.06.074
Kanamarlapudi, S. L. R. K., Chintalpudi, V. K., and Muddada, S. (2018). Application of biosorption for removal of heavy metals from wastewater. Biosorption 18 (69), 70–116. doi:10.5772/intechopen.77315
Kanekiyo, K., Lee, J. B., Hayashi, K., Takenaka, H., Hayakawa, Y., Endo, S., et al. (2005). Isolation of an antiviral polysaccharide, nostoflan, from a terrestrial cyanobacterium, Nostoc flagelliforme. J. Nat. Prod. (Gorakhpur). 68 (7), 1037–1041. doi:10.1021/np050056c
Kang, C. D., An, J. Y., Park, T. H., and Sim, S. J. (2006). Astaxanthin biosynthesis from simultaneous N and P uptake by the green alga Haematococcus pluvialis in primary-treated wastewater. Biochem. Eng. J. 31 (3), 234–238. doi:10.1016/j.bej.2006.08.002
Kassim, M. A., and Meng, T. K. (2017). Carbon dioxide (CO2) biofixation by microalgae and its potential for biorefinery and biofuel production. Sci. Total Environ. 585, 1121–1129. doi:10.1016/j.scitotenv.2017.01.172
Khoo, K. S., Lee, S. Y., Ooi, C. W., Fu, X., Miao, X., Ling, T. C., et al. (2019). Recent advances in biorefinery of astaxanthin from Haematococcus pluvialis. Bioresour. Technol. 288, 121606. doi:10.1016/j.biortech.2019.121606
Kinnunen, V., and Rintala, J. (2016). The effect of low-temperature pretreatment on the solubilization and biomethane potential of microalgae biomass grown in synthetic and wastewater media. Bioresour. Technol. 221, 78–84. doi:10.1016/j.biortech.2016.09.017
Ko, S. C., Kang, N., Kim, E. A., Kang, M. C., Lee, S. H., Kang, S. M., et al. (2012). A novel angiotensin I-converting enzyme (ACE) inhibitory peptide from a marine Chlorella ellipsoidea and its antihypertensive effect in spontaneously hypertensive rats. Process Biochem. 47 (12), 2005–2011. doi:10.1016/j.procbio.2012.07.015
Kumar, B. R., Mathimani, T., Sudhakar, M. P., Rajendran, K., Nizami, A. S., Brindhadevi, K., et al. (2021). A state of the art review on the cultivation of algae for energy and other valuable products: Application, challenges, and opportunities. Renew. Sustain. Energy Rev. 138, 110649. doi:10.1016/j.rser.2020.110649
Kumar, K. S., Dahms, H. U., Won, E. J., Lee, J. S., and Shin, K. H. (2015). Microalgae–a promising tool for heavy metal remediation. Ecotoxicol. Environ. Saf. 113, 329–352. doi:10.1016/j.ecoenv.2014.12.019
Kushwaha, A., Yadav, A. N., Singh, B., Dwivedi, V., Kumar, S., Goswami, L., et al. (2022). “Life cycle assessment and techno-economic analysis of algae-derived biodiesel: Current challenges and future prospects,” in Waste-to-Energy approaches towards zero waste. Editors C. M. Hussain, S. Singh, and L. Goswami (Cambridge, Massachusetts: Elsevier Academic Press), 343–372.
Leong, Y. K., and Chang, J. S. (2020). Bioremediation of heavy metals using microalgae: Recent advances and mechanisms. Bioresour. Technol. 303, 122886. doi:10.1016/j.biortech.2020.122886
Li, K., Liu, Q., Fang, F., Luo, R., Lu, Q., Zhou, W., et al. (2019). Microalgae-based wastewater treatment for nutrients recovery: A review. Bioresour. Technol. 291, 121934. doi:10.1016/j.biortech.2019.121934
Li, S., Show, P. L., Ngo, H. H., and Ho, S. H. (2022). Algae-mediated antibiotic wastewater treatment: A critical review. Environ. Sci. Ecotechnology 9, 100145. doi:10.1016/j.ese.2022.100145
Li, Y., Horsman, M., Wu, N., Lan, C. Q., and Dubois-Calero, N. (2008). Biofuels from microalgae. Biotechnol. Prog. 24, 0–820. doi:10.1021/bp070371k
Lima, S., Villanova, V., Grisafi, F., Caputo, G., Brucato, A., and Scargiali, F. (2020). Autochthonous microalgae grown in municipal wastewaters as a tool for effectively removing nitrogen and phosphorous. J. Water Process Eng. 38, 101647. doi:10.1016/j.jwpe.2020.101647
Liu, X. Y., and Hong, Y. (2021). Microalgae-based wastewater treatment and recovery with biomass and value-added products: A brief review. Curr. Pollut. Rep. 7, 227–245. doi:10.1007/s40726-021-00184-6
Liu, Y., and Yildiz, I. (2018). The effect of salinity concentration on algal biomass production and nutrient removal from municipal wastewater by Dunaliella salina. Int. J. Energy Res. 42, 2997–3006. doi:10.1002/er.3967
Loiseau, E., Saikku, L., Antikainen, R., Droste, N., Hansjürgens, B., Pitkänen, K., et al. (2016). Green economy and related concepts: An overview. J. Clean. Prod. 139, 361–371. doi:10.1016/j.jclepro.2016.08.024
Lopes, P. A., Bandarra, N. M., Martins, S. V., Martinho, J., Alfaia, C. M., Madeira, M. S., et al. (2017). Markers of neuroprotection of combined EPA and DHA provided by fish oil are higher than those of EPA (Nannochloropsis) and DHA (Schizochytrium) from microalgae oils in Wistar rats. Nutr. Metab. 14 (1), 62–17. doi:10.1186/s12986-017-0218-y
Lupette, J., and Benning, C. (2020). Human health benefits of very-long-chain polyunsaturated fatty acids from microalgae. Biochimie 178, 15–25. doi:10.1016/j.biochi.2020.04.022
Lv, J., Liu, Y., Feng, J., Liu, Q., Nan, F., and Xie, S. (2018). Nutrients removal from undiluted cattle farm wastewater by the two-stage process of microalgae-based wastewater treatment. Bioresour. Technol. 264, 311–318. doi:10.1016/j.biortech.2018.05.085
MacDougall, K. M., McNichol, J., McGinn, P. J., O’Leary, S. J. B., and Melanson, J. E. (2011). Triacylglycerol profiling of microalgae strains for biofuel feedstock by liquid chromatography–high-resolution mass spectrometry. Anal. Bioanal. Chem. 401, 2609–2616. doi:10.1007/s00216-011-5376-6
Maia, J. L. D., Cardoso, J. S., Mastrantonio, D. J. D. S., Bierhals, C. K., Moreira, J. B., Costa, J. A. V., et al. (2020). Microalgae starch: A promising raw material for the bioethanol production. Int. J. Biol. Macromol. 165 (2), 2739–2749. doi:10.1016/j.ijbiomac.2020.10.159
Milledge, J. J., Nielsen, B. V., Maneein, S., and Harvey, P. J. (2019). A brief review of anaerobic digestion of algae for bioenergy. Energies 12 (6), 1166. doi:10.3390/en12061166
Moaveni, S., Salami, M., Khodadadi, M., McDougall, M., and Emam-Djomeh, Z. (2022). Investigation of S. limacinum microalgae digestibility and production of antioxidant bioactive peptides. LWT 154, 112468. doi:10.1016/j.lwt.2021.112468
Mohsenpour, S. F., Hennige, S., Willoughby, N., Adeloye, A., and Gutierrez, T. (2021). Integrating micro-algae into wastewater treatment: A review. Sci. Total Environ. 752, 142168. doi:10.1016/j.scitotenv.2020.142168
Molazadeh, M., Ahmadzadeh, H., Pourianfar, H. R., Lyon, S., and Rampelotto, P. H. (2019). The use of microalgae for coupling wastewater treatment with CO2 biofixation. Front. Bioeng. Biotechnol. 7, 42. doi:10.3389/fbioe.2019.00042
Molino, A., Mehariya, S., Karatza, D., Chianese, S., Iovine, A., Casella, P., et al. (2019). Bench-scale cultivation of microalgae Scenedesmus almeriensis for CO2 capture and lutein production. Energies 12, 2806. doi:10.3390/en12142806
Mondal, M., Goswami, S., Ghosh, A., Oinam, G., Tiwari, O. N., Das, P., et al. (2017). Production of biodiesel from microalgae through biological carbon capture: A review. 3 Biotech. 7 (2), 99. doi:10.1007/s13205-017-0727-4
Moreira, D., and Pires, J. C. M. (2016). Atmospheric CO2 capture by algae: Negative carbon dioxide emission path. Bioresour. Technol. 215, 371–379. doi:10.1016/j.biortech.2016.03.060
Nayak, M., Suh, W. I., Lee, B., and Chang, Y. K. (2018). Enhanced carbon utilization efficiency and FAME production of Chlorella sp. HS2 through combined supplementation of bicarbonate and carbon dioxide. Energy Convers. Manag. 156, 45–52. doi:10.1016/j.enconman.2017.11.002
Ngatu, N. R., Okajima, M. K., Yokogawa, M., Hirota, R., Eitoku, M., Muzembo, B. A., et al. (2012). Anti-inflammatory effects of sacran, a novel polysaccharide from Aphanothece sacrum, on 2, 4, 6-trinitrochlorobenzene–induced allergic dermatitis in vivo. Ann. Allergy Asthma Immunol. 108 (2), 117–122.e2. doi:10.1016/j.anai.2011.10.013
Onay, M. (2018). Bioethanol production from Nannochloropsis gaditana in municipal wastewater. Energy Procedia 153, 253–257. doi:10.1016/j.egypro.2018.10.032
Onyeaka, H., Miri, T., Obileke, K., Hart, A., Anumudu, C., and Al-Sharify, Z. T. (2021). Minimizing carbon footprint via microalgae as a biological capture. Carbon Capture Sci. Technol. 1, 100007. doi:10.1016/j.ccst.2021.100007
Pancha, I., Chokshi, K., and Mishra, S. (2019). “Industrial wastewater-based microalgal biorefinery: A dual strategy to remediate waste and produce microalgal bioproducts,” in Application of microalgae in wastewater treatment. Editors S. Gupta,, and F. Bux (Cham: Springer), 173–193.
Pandey, A., Srivastava, S., and Kumar, S. (2019). Isolation, screening and comprehensive characterization of candidate microalgae for biofuel feedstock production and dairy effluent treatment: A sustainable approach. Bioresour. Technol. 293, 121998. doi:10.1016/j.biortech.2019.121998
Paul, S., Bera, S., Dasgupta, R., Mondal, S., and Roy, S. (2021). Review on the recent structural advances in open and closed systems for carbon capture through algae. Energy Nexus 4, 100032. doi:10.1016/j.nexus.2021.100032
Peter, A. P., Khoo, K. S., Chew, K. W., Ling, T. C., Ho, S. H., Chang, J. S., et al. (2021). Microalgae for biofuels, wastewater treatment and environmental monitoring. Environ. Chem. Lett. 19 (4), 2891–2904. doi:10.1007/s10311-021-01219-6
Prabakaran, G., Sampathkumar, P., Kavisri, M., and Moovendhan, M. (2020). Extraction and characterization of phycocyanin from Spirulina platensis and evaluation of its anticancer, antidiabetic and antiinflammatory effect. Int. J. Biol. Macromol. 153, 256–263. doi:10.1016/j.ijbiomac.2020.03.009
Prasad, R., Gupta, S. K., Shabnam, N., Oliveira, C. Y. B., Nema, A. K., Ansari, F. A., et al. (2021). Role of microalgae in global CO2 sequestration: Physiological mechanism, recent development, challenges, and future prospective. Sustainability 13, 13061. doi:10.3390/su132313061
Rasoul-Amini, S., Montazeri-Najafabady, N., Shaker, S., Safari, A., Kazemi, A., Mousavi, P., et al. (2014). Removal of nitrogen and phosphorus from wastewater using microalgae free cells in bath culture system. Biocatal. Agric. Biotechnol. 3 (2), 126–131. doi:10.1016/j.bcab.2013.09.003
Razzak, S. A., Ali, S. A. M., Hossain, M. M., and deLasa, H. (2017). Biological CO2 fixation with production of microalgae in wastewater – a review. Renew. Sustain. Energy Rev. 76, 379–390. doi:10.1016/j.rser.2017.02.038
Rodas-Zuluaga, L. I., Castañeda-Hernández, L., Castillo-Vacas, E. I., Gradiz-Menjivar, A., López-Pacheco, I. Y., Castillo-Zacarías, C., et al. (2020). Bio-capture and influence of CO2 on the growth rate and biomass composition of the microalgae Botryococcus braunii and Scenedesmus sp. J. CO2 Util. 43, 101371. doi:10.1016/j.jcou.2020.101371
Ruiz-Marin, A., Canedo-López, Y., and Chávez-Fuentes, P. (2020). Biohydrogen production by Chlorella vulgaris and Scenedesmus obliquus immobilized cultivated in artificial wastewater under different light quality. Amb. Express 10, 191. doi:10.1186/s13568-020-01129-w
Ryckebosch, E., Bruneel, C., Termote-Verhalle, R., Goiris, K., Muylaert, K., and Foubert, I. (2014). Nutritional evaluation of microalgae oils rich in omega-3 long chain polyunsaturated fatty acids as an alternative for fish oil. Food Chem. x. 160, 393–400. doi:10.1016/j.foodchem.2014.03.087
Salam, K. A. (2019). https://www.biofueljournal.com/article_88261.html. Biofuel Res. J. 6, 948–961. doi:10.18331/BRJ2019.6.2.2
Scott, K., Yu, E. H., Ghangrekar, M. M., Erable, B., and Duţeanu, N. M. (2012). “Biological and microbial fuel cells,” in Comprehensive renewable energy. Editor A. Sayigh (Amsterdam: Elsevier), 277–300.
Shah, M. M. R., Liang, Y., Cheng, J. J., and Daroch, M. (2016). Astaxanthin-producing green microalga Haematococcus pluvialis: From single cell to high value commercial products. Front. Plant Sci. 7, 531. doi:10.3389/fpls.2016.00531
Sheehan, J., Dunahay, T., Benemann, J., and Roessler, P. (1998). A look back at the U. S. Department of Energy’s aquatic species program: Biodiesel from algae; close-out report. NREL Report No. TP-580-24190.
Sheih, I. C., Fang, T. J., Wu, T. K., and Lin, P. H. (2010). Anticancer and antioxidant activities of the peptide fraction from algae protein waste. J. Agric. Food Chem. 58 (2), 1202–1207. doi:10.1021/jf903089m
Singh, U. B., and Ahluwalia, A. S. (2013). Microalgae: A promising tool for carbon sequestration. Mitig. Adapt. Strateg. Glob. Chang. 18, 73–95. doi:10.1007/s11027-012-9393-3
Srimongkol, P., Thongchul, N., Phunpruch, S., and Karnchanatat, A. (2019a). Ability of marine cyanobacterium Synechococcus sp. VDW to remove ammonium from brackish aquaculture wastewater. Agric. Water Manag. 212, 155–161. doi:10.1016/j.agwat.2018.09.006
Srimongkol, P., Thongchul, N., Phunpruch, S., and Karnchanatat, A. (2019b). Optimization of Synechococcus sp. VDW cultivation with artificially prepared shrimp wastewater for ammonium removal and its potential for use as a biofuel feedstock. J. Oleo Sci. 68, 233–243. doi:10.5650/jos.ess18203
Srinuanpan, S., Cheirsilp, B., and Kassim, M. A. (2020). “Oleaginous microalgae cultivation for biogas upgrading and phytoremediation of wastewater,” in Microalgae cultivation for biofuels production. Editor A. Yousuf (Cambridge, Massachusetts: Elsevier Academic Press), 69–82.
Stepan, D. J., Shockey, R. E., Moe, T. A., and Dorn, R. (2002). Carbon dioxide sequestering using microalgal systems. Pittsburgh, PA: U.S: Department of Energy.
Stewart, C., and Hessami, M. A. (2005). A study of methods of carbon dioxide capture and sequestration-the sustainability of a photosynthetic bioreactor approach. Energy Convers. Manag. 46, 403–420. doi:10.1016/j.enconman.2004.03.009
Suttisuwan, R., Phunpruch, S., Saisavoey, T., Sangtanoo, P., Thongchul, N., and Karnchanatat, A. (2019b). Free radical scavenging properties and induction of apoptotic effects of Fa fraction obtained after proteolysis of bioactive peptides from microalgae Synechococcus sp. VDW. Food Technol. Biotechnol. 57 (3), 358–368. doi:10.17113/ftb.57.03.19.6028
Suttisuwan, R., Phunpruch, S., Saisavoey, T., Sangtanoo, P., Thongchul, N., and Karnchanatat, A. (2019a). Isolation and characterization of anti-inflammatory peptides derived from trypsin hydrolysis of microalgae protein (Synechococcus sp. VDW). Food Biotechnol. 33 (4), 303–324. doi:10.1080/08905436.2019.1673171
Talero, E., García-Mauriño, S., Ávila-Román, J., Rodríguez-Luna, A., Alcaide, A., and Motilva, V. (2015). Bioactive compounds isolated from microalgae in chronic inflammation and cancer. Mar. Drugs 13 (10), 6152–6209. doi:10.3390/md13106152
Tao, R., Kinnunen, V., Praveenkumar, R., Lakaniemi, A. M., and Rintala, J. A. (2017). Comparison of Scenedesmus acuminatus and Chlorella vulgaris cultivation in liquid digestates from anaerobic digestion of pulp and paper industry and municipal wastewater treatment sludge. J. Appl. Phycol. 29 (6), 2845–2856. doi:10.1007/s10811-017-1175-6
Tinpranee, N., Incharoensakdi, A., and Phunpruch, S. (2018). Screening cyanobacteria from marine coastal waters of Thailand for biohydrogen production. J. Appl. Phycol. 30, 3471–3481. doi:10.1007/s10811-018-1490-6
Tollefson, J. (2021). COVID curbed carbon emissions in 2020-but not by much. Nature 589, 343. doi:10.1038/d41586-021-00090-3
Tsai, D. D. W., Chen, P. H., and Ramaraj, R. (2017). The potential of carbon dioxide capture and sequestration with algae. Ecol. Eng. 98, 17–23. doi:10.1016/j.ecoleng.2016.10.049
Udaiyappan, A. F. M., Hasan, H. A., Takriff, M. S., and Abdullah, S. R. S. (2017). A review of the potentials, challenges and current status of microalgae biomass applications in industrial wastewater treatment. J. Water Process Eng. 20, 8–21. doi:10.1016/j.jwpe.2017.09.006
United Nations, (2019). World population prospects 2019. Available at: https://population.un.org/wpp. (Accessed June 17, 2019).
Valdovinos-García, E. M., Barajas-Fernández, J., Olán-Acosta, M. D. L. Á., Petriz-Prieto, M. A., Guzmán-López, A., and Bravo-Sánchez, M. G. (2020). Techno-economic study of CO2 capture of a thermoelectric plant using microalgae (Chlorella vulgaris) for production of feedstock for bioenergy. Energies 13 (2), 413. doi:10.3390/en13020413
Vanegas, C. H., and Bartlett, J. (2013). Green energy from marine algae: Biogas production and composition from the anaerobic digestion of Irish seaweed species. Environ. Technol. 34 (15), 2277–2283. doi:10.1080/09593330.2013.765922
Vassalle, L., Díez-Montero, R., Machado, A. T. R., Moreira, C., Ferrer, I., Mota, C. R., et al. (2020). Upflow anaerobic sludge blanket in microalgae-based sewage treatment: Co-Digestion for improving biogas production. Bioresour. Technol. 300, 122677. doi:10.1016/j.biortech.2019.122677
Viegas, C., Gouveia, L., and Gonçalves, M. (2021). Aquaculture wastewater treatment through microalgal. Biomass potential applications on animal feed, agriculture, and energy. J. Environ. Manage. 286, 112187. doi:10.1016/j.jenvman.2021.112187
Wang, K., Khoo, S. K., Kit, W. C., Anurita, S., Wei-Hsin, C., Jo-Shu, C., et al. (2021). Microalgae: The future supply house of biohydrogen and biogas. Front. Energy Res. 9. doi:10.3389/fenrg.2021.660399
Wang, S., Mukhambet, Y., Esakkimuthu, S., and Abomohra, A. (2022). Integrated microalgal biorefinery–Routes, energy, economic and environmental perspectives. J. Clean. Prod., 131245. doi:10.1016/j.jclepro.2022.131245
Wang, Y., Ho, S. H., Cheng, C. L., Guo, W. Q., Nagarajan, D., Ren, N. Q., et al. (2016). Perspectives on the feasibility of using microalgae for industrial wastewater treatment. Bioresour. Technol. 222, 485–497. doi:10.1016/j.biortech.2016.09.106
Wijffels, R. H., and Barbosa, M. J. (2010). An outlook on microalgal biofuels. Science 329, 796–799. doi:10.1126/science.1189003
Wollmann, F., Dietze, S., Ackermann, J. U., Bley, T., Walther, T., Steingroewer, J., et al. (2019). Microalgae wastewater treatment: Biological and technological approaches. Eng. Life Sci. 19 (12), 860–871. doi:10.1002/elsc.201900071
Wu, J. Y., Lay, C. H., Chen, C. C., and Wu, S. Y. (2017). Lipid accumulating microalgae cultivation in textile wastewater: Environmental parameters optimization. J. Taiwan Inst. Chem. Eng. 79, 1–6. doi:10.1016/j.jtice.2017.02.017
Xiaogang, H., Jalalah, M., Jingyuan, W., Zheng, Y., Li, X., and Salama, E-S. (2020). Microalgal growth coupled with wastewater treatment in open and closed systems for advanced biofuel generation. Biomass Convers. biorefin. 12, 1939–1958. doi:10.1007/s13399-020-01061-w
Yadav, G., Panda, S. P., and Sen, R. (2020). Strategies for the effective solid, liquid and gaseous waste valorization by microalgae: A circular bioeconomy perspective. J. Environ. Chem. Eng. 8 (6), 104518. doi:10.1016/j.jece:2020.104518
Yang, H., He, Q., and Hu, C. (2018). Feasibility of biodiesel production and CO2 emission reduction by Monoraphidium dybowskii LB50 under semi-continuous culture with open raceway ponds in the desert area. Biotechnol. Biofuels 11, 82. doi:10.1186/s13068-018-1068-1
Yang, X. E., Wu, X., Hao, H. L., and He, Z. L. (2008). Mechanisms and assessment of water eutrophication. J. Zhejiang Univ. Sci. B 9, 197–209. doi:10.1631/jzus.B0710626
Yim, J. H., Kim, S. J., Ahn, S. H., and Lee, H. K. (2003). Optimal conditions for the production of sulfated polysaccharide by marine microalga Gyrodinium impudicum strain KG03. Biomol. Eng. 20 (4-6), 273–280. doi:10.1016/s1389-0344(03)00070-4
You, X., Yang, L., Zhou, X., and Zhang, Y. (2022). Sustainability and carbon neutrality trends for microalgae-based wastewater treatment: A review. Environ. Res. 112860. doi:10.1016/j.envres.2022.112860
Yu, Q., Wang, H., Li, X., Yin, Y., Qin, S., and Ge, B. (2020). Enhanced biomass and CO2 sequestration of Chlorella vulgaris using a new mixotrophic cultivation method. Process Biochem. 90, 168–176. doi:10.1016/j.procbio.2019.11.022
Zeng, X., Danquah, M. K., Chen, X. D., and Yinghua, L. (2011). Microalgae bioengineering: From CO2 fixation to biofuel production. Renew. Sustain. Energy Rev. 15, 3252–3260. doi:10.1016/j.rser.2011.04.014
Zhang, S., and Liu, Z. (2021). Advances in the biological fixation of carbon dioxide by microalgae. J. Chem. Technol. Biotechnol. 96 (6), 1475–1495. doi:10.1002/jctb.6714
Zhou, J., Wang, M., Saraiva, J. A., Martins, A. P., Pinto, C. A., Prieto, M. A., et al. (2022). Extraction of lipids from microalgae using classical and innovative approaches. Food Chem. x. 384, 132236. doi:10.1016/j.foodchem.2022.132236
Zhou, W., Hu, B., Li, Y., Min, M., Mohr, M., Du, Z., et al. (2012). Mass cultivation of microalgae on animal wastewater: A sequential two-stage cultivation process for energy crop and omega-3-rich animal feed production. Appl. Biochem. Biotechnol. 168 (2), 348–363. doi:10.1007/s12010-012-9779-4
Zhu, L., Wang, Z., Takala, J., Hiltunen, E., Qin, L., Xu, Z., et al. (2013). Scale-up potential of cultivating Chlorella zofingiensis in piggery wastewater for biodiesel production. Bioresour. Technol. 137, 318–325. doi:10.1016/j.biortech.2013.03.144
Keywords: microalgae, wastewater treatment, biomolecule production, biorefineries, bioenergy
Citation: Srimongkol P, Sangtanoo P, Songserm P, Watsuntorn W and Karnchanatat A (2022) Microalgae-based wastewater treatment for developing economic and environmental sustainability: Current status and future prospects. Front. Bioeng. Biotechnol. 10:904046. doi: 10.3389/fbioe.2022.904046
Received: 25 March 2022; Accepted: 18 July 2022;
Published: 07 September 2022.
Edited by:
Aran Incharoensakdi, Chulalongkorn University, ThailandReviewed by:
Kuan Shiong Khoo, UCSI University, MalaysiaJun Wei Lim, University of Technology Petronas, Malaysia
Copyright © 2022 Srimongkol, Sangtanoo, Songserm, Watsuntorn and Karnchanatat. This is an open-access article distributed under the terms of the Creative Commons Attribution License (CC BY). The use, distribution or reproduction in other forums is permitted, provided the original author(s) and the copyright owner(s) are credited and that the original publication in this journal is cited, in accordance with accepted academic practice. No use, distribution or reproduction is permitted which does not comply with these terms.
*Correspondence: Aphichart Karnchanatat, Aphichart.K@chula.ac.th