- 1Biotechnology and Food Engineering, Guangdong Technion—Israel Institute of Technology, Shantou, China
- 2Guangdong Technion Department of Chemistry—Israel Institute of Technology, Shantou, China
- 3Polymer Physics Laboratory, Department of Chemical Engineering, Guangdong Technion–Israel Institute of Technology (GTIIT), Shantou, China
- 4Faculty of Biotechnology and Food Engineering, Technion—Israel Institute of Technology, Haifa, Israel
- 5Guangdong Provincial Key Laboratory of Materials and Technologies for Energy Conversion, Guangdong Technion—Israel Institute of Technology, Shantou, China
Plasticulture, the practice of using plastic materials in agricultural applications, consumes about 6.7 million tons of plastics every year, which is about 2% of the overall global annual plastics production. For different reasons, plastic material used for agriculture is difficult to recycle. Therefore, most of it is either buried in fertile soils, thereby significantly causing deterioration of their properties, or, at best case, end in landfills where its half-life is measured in decades and even centuries. Hence, developing biodegradable plastic materials that are suitable for agricultural applications is a vital and inevitable need for the global human society. In our labs, two types of potentially biodegradable plastic polymer films were prepared and characterized imidazolium in terms of their bio-degradability. In the first approach, polymers made of ionic liquid monomers were prepared using photo radical induced polymerization. The second approach relies on formation of polyethylene-like n-alkane disulfide polymers from 1,ω-di-thiols through thermally activated air oxidation. These two families of materials were tested for their biodegradability in soils by using a simulation system that combines a controlled environment chamber equipped with a respirometer and a proton-transfer-reaction time of flight mass spectrometer (PTR-TOF-MS) system. This system provides a time-dependent and comprehensive fingerprint of volatiles emitted in the degradation process. The results obtained thus far indicate that whereas the ionic-liquid based polymer does not show significant bio-degradability under the test conditions, the building block monomer, 1,10-n-decane dithiol, as well as its disulfide-based polymer, are bio-degradable. The latter reaching, under basic soil conditions and in room temperature, ∼20% degradation within three months. These results suggest that by introduction of disulfide groups into the polyethylene backbone one may be able to render it biodegradable, thus considerably shortening its half-life in soils. Principal component analysis, PCA, of the data about the total volatiles produced during the degradation in soil indicates a distinctive volatile “fingerprint” of the disulfide-based bio-degradable products which comes from the volatile organic compounds portfolio as recorded by the PTR-TOF-MS. The biodegradation volatile fingerprint of this kind of film was different from the “fingerprint” of the soil background which served as a control. These results can help us to better understand and design biodegradable films for agricultural mulching practices.
Introduction
Plastic waste is an increasing concern around the world. The use of plastics in agricultural practices such as mulching is on the rise as well (Huang et al., 2020). Plasticulture, i.e. agricultural practices that include the use of plastic materials, is essential for food security in most parts of the world. The usage of plastic polymers in agriculture is quite diverse, including for example: greenhouse covers, trailing, packaging, irrigation systems, silage, soil mulching and more (Briassoulis and Dejean, 2010; Zumstein et al., 2019; Zurier and Goddard, 2021). Although necessary, the use of plastics in agriculture can lead to wide environmental adverse effects, such as contamination of ground water, disturbance of the ecosystems of terrestrial and aquatic fauna and flora (Zhou et al., 2020), deterioration of soil properties of agricultural lands (for example their gas exchange and water retention capacity), spread of toxic microplastics particles (Sander, 2019; Huang et al., 2020; Zurier and Goddard, 2021) etc. Plastic mulching is probably the most abundant plasticulture practice. Agricultural plastic mulching is done for various reasons, including water preservation, crop protection, soil remediation, treatments against weeds and soilborne pathogens (such as solarization and fumigation (Achmon et al., 2017)) and other important practices used to increase the yields of crops. Mulching is estimated to cover an agricultural area of more than 128,500 km2 around the world (Zhou et al., 2020) and China is the global leader in terms of plasticulture mulching practices (Huang et al., 2020). For these reasons, the need for biodegradable plastics for agricultural mulching is critical from the environmental point of view. Although it is an obvious need, not many commercial solutions are currently available and most of the agricultural mulching done today utilizes non-degradable plastic polymers, mainly polyethylene (PE), (Briassoulis et al., 2015; Ahmed et al., 2018; Zhang et al., 2019). Unlike the slow implementation of biodegradable plastic polymers in commercial agricultural mulching systems, research in this field receives increased attention and has a fast progress pace (Kyrikou and Briassoulis, 2007; Sander, 2019; Huang et al., 2020). There are several important characteristics that plastic mulching materials must possess along their entire service life in order to be widely applied in the field: 1) durability (mechanical, chemical, resistance to water and solvents, etc.), 2) mechanical flexibility (ability to be stretched over various shapes of field plots), 3) light weight, 4) modularity (can have variable thickness, color and gas permeability), 5) non-toxicity, and most important, 6) affordability (being inexpensive and easy to produce in very large quantities). The combination of these characteristics makes it hard to find alternative biodegradable plastic polymers to replace the current non-degradable ones. Moreover, it is challenging to define what can be considered as a biodegradable plastic in soil and what is only bio-available (a material that judging from its chemical formula looks biodegradable, but does not actually degrade in a relevant pace (Zumstein et al., 2019)). Some recent progress was done in this area by introducing the European Standard EN 17033: Plastics–Biodegradable mulch films (EN17033 Plastics, 2018). Additionally, most degradation processes are complex and are not sufficiently characterized, and hence are less understood from microbiological and chemical perspectives (Sander, 2019). Yet there are some examples of biodegradable mulching materials that are available, such as cellulose, starch, poly-b-hydroxybutyrate and alike (Kyrikou and Briassoulis, 2007; Sander, 2019). To date, most polymer soil biodegradability studies have been using standard methods such as respiration or polymer size reduction (Achmon et al., 2019) to assess the biodegradability rate. Some recent studies used 13C-labeled polymers to closely monitor the degradation of the carbon skeleton of the polymer (Thomas et al., 2021). The analysis of biodegradability is still a debatable issue and although standards are available and are being updated (EN17033 Plastics, 2018), there is still a lot of room for improvement. Generally, the biodegradability can be looked upon through the carbon conversion by Equation 1.
Equation 1:
Where Cpolymer is the polymers’ carbon backbone, Cbiomass is the utilization of the carbon to build live biomass (mainly microbial) and Cresidues represents the carbon remaining in polymer residues as long as biodegradation is not completed (Sander, 2019). However, a more precise equation is presented in Equation 2.
Equation 2:
Where Csecondary products are additional secondary metabolites produced by those microbes that are not converting all of the polymer into biomass and emit secondary products back to the environment. In most of the studies on biodegradability of polymers the focus is on the CO2 and Cpolymer, and sometimes on Cresidues, but rarely on Csecondary products. Csecondary products can also be divided according to Equation 3.
Equation 3:
Only few studies have looked at the Cnon-volatile products (Zumstein et al., 2019; Thomas et al., 2021) which are mainly soluble residues secreted by the microbial activity, and as far as the authors know, no study to date has looked at the Cvoltile products emanating by the polymer’s biodegradation process in the soil.
In this study we tested two new synthetic materials as potential candidates to be used as biodegradable plastic polymers for agricultural mulching. We also report here a first insight into Cvoltile products (Volatile Organic Compounds (VOCs)) emitted during the biodegradation process of those two new types of polymers. This study is opening a hatch to a new way of looking at the complex mechanisms underlying the biodegradation of polymers in the soil through VOCs production during the process.
Materials and methods
Apparatus
Respirometery experiments were performed using a Micro-Oxymax Respirometer (Columbus Instruments, United States). Detectors of CO2 Carbon Dioxide Sensor 0–10% CH4 Methane Measuring (0–10%) and O2 Paramagnetic Oxygen Sensor 0–100% - (Columbus Instruments, United States) where used to monitor the simulated process of the biodegradation of plastics in soil. Mass spectrometry was performed using a PTR-TOF-MS 1000 (Ionicon Analytik Ges.m.b.H., Innsbruck, Austria).
Materials
Ethyl bromoacetate, 1-vinyl imidazole, butylated hydroxyl toluene (BHT), 1,10-decanedithiol, sodium hydroxide (NaOH), phenylbis (2,4,6-triethylbenzoyl) phosphine oxide were purchased from Sigma-Aldrich (Merck) and used as received. Microcrystalline cellulose was purchased from Alfa Aesar Co. and used as received. All solvents, such as ethyl acetate, methanol, THF and acetone, as well as other materials used in the present research were purchased from Shanghai Macklin Biochemical Co., and were of the highest purity available. All commercial materials were used as received unless specifically noted. Tryptic Soy Agar (TSA) was purchased from BD, MD, United States. Oxytetracycline-Glucose Yeast Extract (OGYE) Agar Base was purchased from Solarbio, Beijing, China. Oxytetracycline hydrochloride was purchased from Solarbio, Beijing China. Bacillus cereus (BC) Agar Base was purchased from OXOID, Basingstoke, United Kingdom. Eosin Methylene Blue Agar (EMBA) was purchased from HuanKai Microbial, Guangdong, China.
1-vinyl-3-(2-ethoxy-2-oxoethyl) imidazolium bromide
Ethyl bromoacetate (10.0 ml, 225 mmol) and 50.0 ml of ethyl acetate were added to a 100 ml round bottom flask equipped with a magnetic stirrer. 1-vinyl imidazole (8.17 ml, 90.2 mmol) containing 100 ppm butylated hydroxyl toluene (BHT) was added dropwise to the flask while stirring, and the mixture was left to stir for 24 h at room temperature. The precipitate was filtered and washed with three portions of ethyl acetate, then dried in a vacuum oven at 40°C for 24 h. The product, 1-vinyl-3-(2-ethoxy-2-oxoethyl) imidazolium bromide, in the form of an off-white solid, was obtained with an 81% yield.
Off white solid, M.P. = 95°C; 81% yield; 1H NMR (400 MHz, D2O, δ): 9.10 (s, 1H, 2-Im), 7.80 (s, 1H, 4-Im), 7.57 (s, 1H, 5-Im)), 7.11 (dd, J = 16.0, 8.0 Hz, 1H, N-CH = CH2), 5.78 (dd, J = 16.0, 4.0 Hz, 1H, CH = CH2), 5.42 (dd, J = 8.0, 8.0 Hz, 1H, CH = CH2), 5.21 (s, 2H, -N+C-CH2-C=O-), 4.23 (q, J = 6.0 Hz, 2H, CH3-CH2-O), 1.20 (t, J = 8.0 Hz, 3H, -CH2-CH3); 13C NMR (400 MHz, D2O, δ): 167.86, 135.95, 128.18, 124.23, 119.47, 110.30, 63.74, 50.35, 13.32; HRMS (MALDI-TOF) (ESI) m/z: [M-Br]+calc. for C9H13N2O2, 181.216; found, 181.052.
Poly-(1-vinyl-3-(2-ethoxy-2-oxoethyl) imidazolium bromide)
15 uL of photoinitiator stock solution (0.3 g of phenylbis (2,4,6-triethylbenzoyl) phosphine oxide in 1 g of THF) was added to 1 g of a 1:1 w/v liquid mixture of 1-vinyl-3-(2-ethoxy-2-oxoethyl) imidazolium bromide and 1-vinyl imidazole. The combined solution was spread over glass slides and exposed to UV-VIS light to induce polymerization. The product is obtained in the form of a yellowish-brown film (Sevilia et al., 2022; Zertal et al., 2022).
Poly 1,10-disulfanyl-n-decane
1,10-decanedithiol (9.5 ml, 43.7 mmol) was added to a solution of NaOH (18 g, 450 mmol) in methanol (300 ml) using a 500 ml round bottom flask equipped with a compressed air inlet and an outlet. The reaction mixture was stirred vigorously for 5 days with the addition of 250 ml of methanol each day to compensate for evaporation. The crude, in the form of white color suspension with some lumps, was dried and washed with several portions of water until it became neutral, then with several portions of methanol and acetone, and then dried in a vacuum oven at 45 °C for 48 h. The product, in the form of a white solid, was obtained with a 54% yield (Tada et al., 2012).
White solid, 54% yield; 1H NMR (400 MHz, CDCl3, δ): 2.66–2.49 (t, q, J = 4 Hz, J = 8 Hz 8H, -H2C-S-S-CH2-), 1.60–1.67 (complex multiplet, 8H, -CH2-CH2-S-S-CH2-CH2-), 1.28–1.39 (complex multiplet, 24H, central alkyl chain); 13C NMR (400 MHz, CDCl3, δ): 39.17, 34.03, 29.4–29.43, 28.36–28.52, 24.65.
Determination of inherent biodegradability
The biodegradability of polymer poly-(disulfanyl-n-decane)1,10 decanedithiol (carbon content: 58.8%wt.) and of monomer 1,10-decane dithiol (carbon content: 58.2%wt.) in soil under basic conditions and unadjusted conditions was investigated in this preliminary experiment. The biodegradability of plastic materials was determined according to ISO 17556:2019 (ISO, 2019) by measuring the amounts of evolved CO2. Soil samples were collected from the field experiment station of the GTIIT, in Shantou, Guangdong, China (23°31′4″N, 116°45′6″E). After collection, the soil was sieved to give particles smaller than 2 mm in size and obvious plant materials, stones and other inert materials were removed. The sieved soil was then air-dried under the sun for three days. In the test, a biodegradable reference material, microcrystalline cellulose (Alfa Aesar Co. Inc.), was used as a positive control. Samples were prepared by thoroughly mixing 60 g of dry soil with 0.6 g polymer/monomer/reference material. 24 g of distilled water were added to each sample to reach approximately 50% total water-holding capacity. To adjust the sample to the basic environment, 4.2 ml NaOH solution (1 M) was added to the samples at the beginning of the experiment. Throughout the experiment, samples’ pH was maintained at 8.5–8.8 for basic groups and at 5.0–5.5 for unadjusted groups. Soil with only water addition served as the blank group of the test. The soil mixtures were placed inside 100 ml flasks which were connected to a Micro-Oxymax Respirometer (Columbus Instruments, United States). The system was set in aerobic mode and was capable of measuring CO2, O2 and CH4 quantities (CO2 Sensor 0–10% CH4 Measuring (0–10%) and O2 Paramagnetic Sensor 0–100% - Columbus Instruments, United States) with a cycle of approximately 4 h for each individual flask. All flasks were incubated in triplicates at 25°C. Every 10 days, water was added to keep the water content between 40 and 60%wt. of the total water-holding capacity and the soil was gently re-mixed.
The amount of CO2 evolved was measured by the Micro-Oxymax Respirometer and the data was collected with the help of the Micro-Oxymax Windows software. Since the amount of CO2 was recorded in volume units, the measured mass of CO2 (m) was calculate by using the ideal gas law, Equation 4, and Equation 5.
Equation 4:
Equation 5:
where V is the volume, P is the pressure in the respirometer (811 mmHg), R is the ideal gas constant, T is the system temperature (298°K), V is the measured volume of CO2, and Mw is the molecular weight of CO2 (44.01 g/mol).
In accordance with ISO 17556:2019, the percentage biodegradation (Dt) was calculated from Equation 6 and Equation 7:
Equation 6:
Equation 7:
where ThCO2 is the theoretical amount of CO2 that can be evolved by the test material, MT is the mass of test material introduced into each flask, wc is the carbon content of the test material determined from the chemical formula, ΣmT is the cumulative amount of CO2 evolved in the flask containing test material and soil mixture, ΣmB is the cumulative amount of CO2 evolved in the flask containing mere soil (blank). The test was done until 60% of the reference material, microcrystalline cellulose, was degraded given what can be named “inherent biodegradability” that indicates the potential of the materials to be bioavailable in a relatively short period of time.
PTR-TOF-MS analysis
Samples were prepared in 100 ml flasks as done for the biodegradability test at 25°C. An additional abiotic group was prepared by placing 0.9 g of plastics inside an empty flask. The flasks were capped with screw caps having two channels, one for aeration and one for injection into the apparatus. The headspace composition of the samples was measured using a PTR-TOF 1000 (Ionicon Analytik Ges.m.b.H., Innsbruck, Austria) under the following working conditions: 630 V drift voltage, 2.30 mbar drift pressure, 6.5 sccm inlet flow, 80°C drift tube and inlet temperature. H3O+ was employed as the primary ion and the instrument was operated at an E/N ratio (E corresponds to the electric field strength and N to buffer gas number density within the drift tube) of 142 Td (1 Td = 10–17 Vcm2). For each flask, more than 120 cycles (2 min each) were recorded, and a stable stage (generally 90 to 120 cycles) was used for the analysis. All the samples were measured once a day.
Microbial cultures of soil samples
A microbial test was done for microbial load in the tested samples. Four kinds of culture media were prepared for testing the microbial culture growth: Tryptic Soy Agar (TSA) (BD, MD, United States), Oxytetracycline-Glucose Yeast Extract (OGYE) Agar Base (Solarbio, Beijing, China)) with the supplement of Oxytetracycline hydrochloride (Solarbio, Beijing China), Bacillus cereus (BC) Agar Base (OXOID, Basingstoke, United Kingdom) and Eosin Methylene Blue Agar (EMBA) (HuanKai Microbial, Guangdong, China). All media were prepared and sterilized according to their corresponding specifications. For each soil sample, 2 g of soil mixtures were transferred to 20 ml sterilized saline, followed by mixing using a vortex. After the dirt grains settled down, the supernatant (addressed as a 10–1 dilution) was collected and diluted to 10–2. For each medium, 0.1 ml dilution was used for plating. TSA and EMBA were incubated at 37°C for 24 h, while OGYE and BC were incubated at 30°C for 24 h.
Data analysis
Statistical ANOVA tests were performed using the R 4.1.1 software (R Foundation for Statistical Computing, Vienna, Austria) to evaluate the differences in the CO2 evolution rate between plastic/reference material degradation processes and their corresponding blanks. The PTR data were pre-processed and analyzed using PTR-MS Viewer 3.2 (Ionicon Analytik Ges.m.b.H., Innsbruck, Austria). Principal component analysis (PCA) was carried out using R 4.1.1, and 3D plots of the PTR spectra were generated using MATLAB R2021b (The MathWorks, Inc., Natick, MA, United States), based on the exported PTR data.
Results and discussion
Polymers
Two prototypes of polymer backbones were investigated, with the aim of developing novel biodegradable polymers, based on their biodegradability properties. A first prototype, poly-VI-VIM, is composed of a mixture of 1-vinylimidazole (VI) and 1-vinyl-3-(2-ethoxy-2-oxoethyl) imidazolium bromide (VIM), and was prepared by photoinduced radical polymerization, according to Figure 1, where n/m = 3.75. A 1:1 wt. mixture of VI and VIM was prepared, then 0.0045 mg/ml of photo initiator (Irgacure 819) was added, and the mixture was well mixed, spreaded atop a glass plate and cured using a 100 W white LED. Curing was monitored by FT-IR, and irradiation proceeded untill the complete disappearance of the vinyl absorption bands. The resulting films were used as prepared for the biodegradation experiments.
The second prototype material, poly-(disulfanyl-n-decane), was prepared in a single step from commercially available 1,10-decanedithiol through air oxidation under basic conditions, (Figure 2). 1,10-decanedithiol was dissolved in methanol containing NaOH and exposed to air flow for 5 days at room temperature. A white color thick melt was obtained, which was washed with several portions of distilled water until it became neutral, then washed with several portions of methanol and acetone, and then it was filtered and dried in a vacuum oven at 45°C for 48 h. The bright white powder, poly-(disulfanyl-n-decane), was used for the preparation of disk samples, Φ = 25 mm, thickness = 2 mm, through compression molding, done for 1 h at 125°C under 13 Mpa pressure between two smooth PTFE sheets.

FIGURE 2. Air oxidation of 1,10-decanedithiol to poly-(disulfanyl-n-decane). Measuring the biodegradation of the polymers in the soil.
Biodegradability of plastic polymers in the soil is usually measured by monitoring the emission of CO2 during the process (Briassoulis et al., 2020; Huang et al., 2020; Zurier and Goddard, 2021). In this study the Micro-Oxymax respirometer system was used to monitor the CO2, O2 and CH4 evolution caused by the biodegradation of the two polymers poly-VI-VIM and poly-(disulfanyl-n-decane), in the soil (Figure 3).

FIGURE 3. Flow chart of the biodegradability test and volatile compounds analysis. Test plastic (or reference material) and dried soil were mixed homogeneously with the supplement of distilled water. The soil mixture was incubated in a low temperature incubator with a constant temperature of 25 °C. The changes of CO2, O2 and CH4 concentrations were measured by a Micro-Oxymax Respirometer System in real time and the volatile compounds were analyzed using a PTR-TOF-MS.
The system allowed a real-time and continuous measurement of the three relevant gases. The results showed that poly-VI-VIM exhibited very low biodegradability under the tested experimental conditions and it did not show any significant difference from the control soil system (data not shown). In contrast, poly-(disulfanyl-n-decane) showed significant biodegradability in the soil (Figures 4–6 and Table 1). A preliminary experiment was done to evaluate the optimal soil biodegradation conditions in terms of pH (Figure 4). In this experiment both poly-(disulfanyl-n-decane) and its monomer were tested for their bioavailability. The biodegradation assessment was done by monitoring the accumulation and evolution rate of CO2 and O2 respiration of the soil (Figure 4A,B,C,E). The accumulation and evolution rate of CH4 was also monitored to see that no release of this potent greenhouse gas (GHG) takes place as part of the biodegradation process (Figure 4C,F). The results indicate a significantly more efficient degradation under basic conditions (pH∼8.5) of the soil than under neutral conditions (pH∼6) (Figure 4). The pH level is known to be an important factor that may have a significant impact on plastic biodegradation in the soil (Emadian et al., 2017; Fernandes et al., 2020; Liu et al., 2021). A recent study found that residues of plastic mulches have a lower negative impact on rice growth under basic conditions (pH 8.5) (Liu et al., 2021). In this study the “inherent” biodegradability was measured rather than a complete “ultimate biodegradability” which is a complete mineralization of the plastic polymer. The “inherent” biodegradability is a sufficient measurement to indicate how much of the plastic polymer is available for a relatively short period of biodegradation.
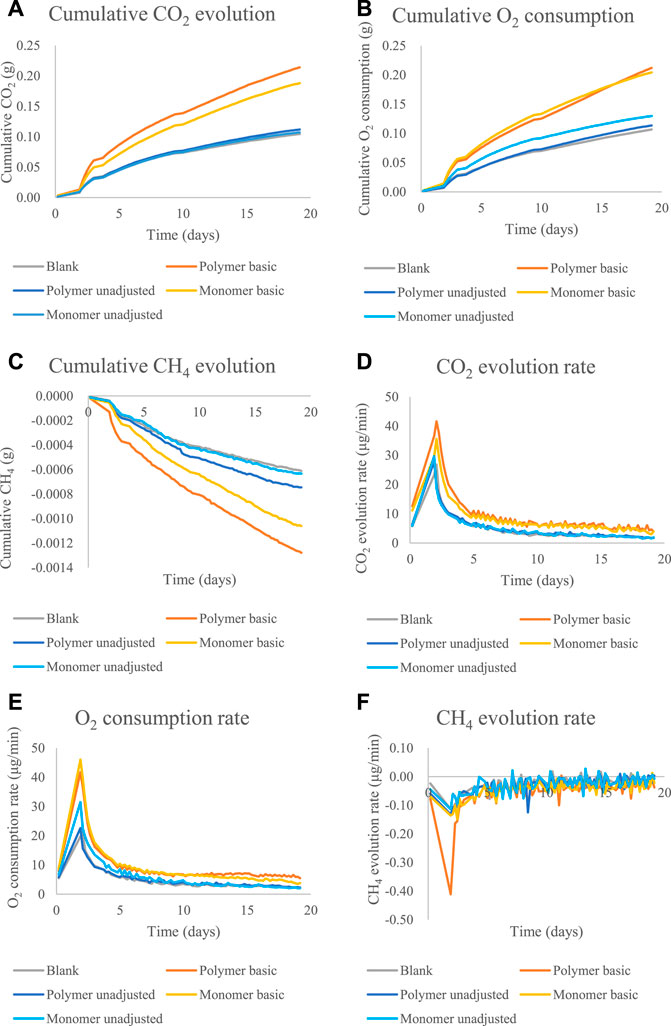
FIGURE 4. The cumulative amounts and rate of CO2, O2 and CH4 emission for the biodegradability test of poly-(disulfanyl-n-decane) and monomer 1,10-decane dithiol under basic conditions (pH∼8.5) and unadjusted conditions (pH∼5.3): (A) the cumulative CO2 evolution. (B) The cumulative O2 consumption. (C) The cumulative CH4 evolution. (D) The CO2 evolution rate. (E) The O2 consumption rate. (F) The CH4 evolution rate.
To get a better understanding of the biodegradation process of poly-(disulfanyl-n-decane) an additional study was performed under optimal pH conditions (pH ∼8.5) and for a longer period (Figure 5). Poly-(disulfanyl-n-decane) showed significantly higher accumulation and evolution rate of CO2 and O2 respiration than those measured in the control soil respiration (Figure 5).
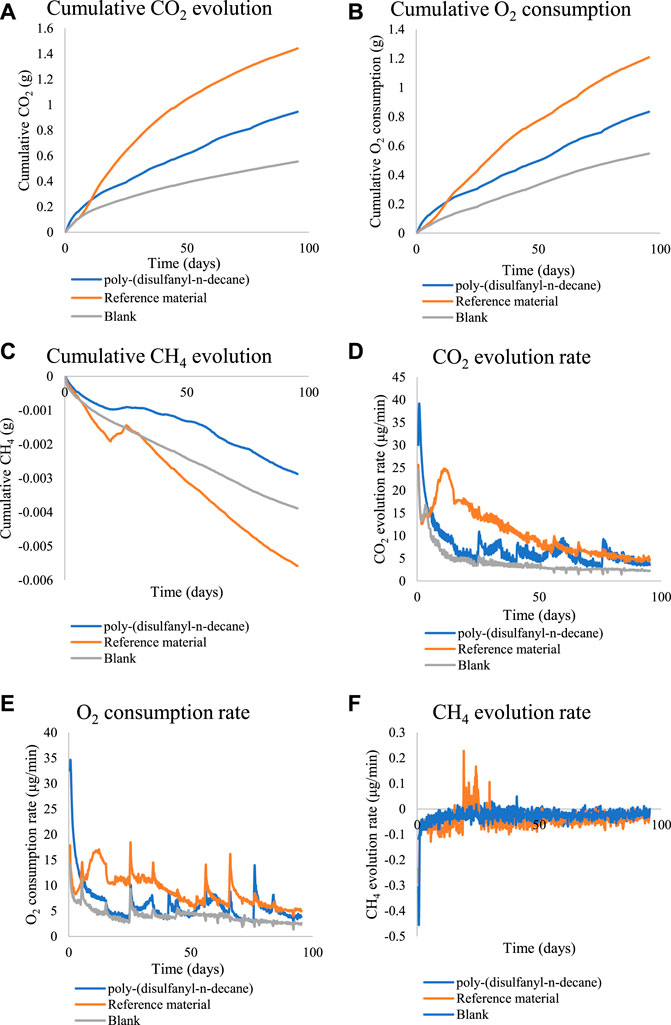
FIGURE 5. The cumulative amounts and rate of CO2, O2 and CH4 for the biodegradability test of poly-(disulfanyl-n-decane). (A) The cumulative CO2 evolution. (B) The cumulative O2 consumption. (C) The cumulative CH4 evolution. (D) The CO2 evolution rate. (E) The O2 consumption rate. (F) The CH4 evolution rate.
Both CO2 and O2 respiration patterns were similar during the three months of the degradation in soil experiment. Around 0.8 and 0.4 g of CO2 were produced during the three months from the reference material (cellulose microcrystalline) and the tested poly-(disulfanyl-n-decane) polymer respectively (after subtraction of the results from the soil control). A similar trend was observed for the oxygen consumption with close to 0.6 and 0.2 g of O2 consumed during the three months more than the reference material (microcrystalline cellulose) and the tested poly-(disulfanyl-n-decane) polymer respectively (after subtraction of the results from the soil control). It is worth noticing that oxygen consumption is a relative measure of the reduction of oxygen below the atmospheric levels (this is why the results are lower than the CO2 production results). The known biodegrading reference material showed as expected the highest biodegradability and reached a 60% degradation in three months (Figure 6), while at the same time the tested poly-(disulfanyl-n-decane) polymer reached only 20% degradation. It is also interesting to note that for an unknown reason the reference material had a certain “lag” phase in the degradation process which was different from the tested material. While at a first glance the observed degradation rate of poly-(disulfanyl-n-decane) seems slow, for many plasticulture applications, including mulching films, it is too fast. Mulching films are used under harsh conditions and their service life ranges from 1 month for short soil treatments to 6 months in some crop protection techniques (Kasirajan and Ngouajio, 2012; Achmon et al., 2018). Along this period of time, these polymer sheets are required to perform with no significant loss of their chemical and physical properties. In order to achieve this, the bio-degradation of the polymer should be by far slower than that of the reference material. The exact optimal degradation conditions test should be done in the specific needed field conditions in the future.
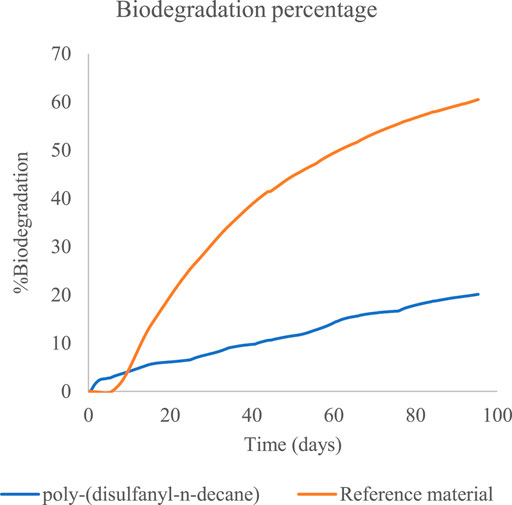
FIGURE 6. The percentage of biodegradation of poly-(disulfanyl-n-decane) and of the reference material.
These results are encouraging as the biodegradability of poly-(disulfanyl-n-decane) suggests that it is bioavailable to the soil microbial population in the tested conditions. It was even shown that a significantly maximal rate of respiration was observed with poly-(disulfanyl-n-decane) than with the negative soil control as well as with the positive reference material (Table 1). As this study focuses on the potential of biodegradability rather than on looking at the complete mineralization of the polymer, the test was conducted until 60% degradation of the reference material was reached (Briassoulis and Dejean, 2010). Additionally, all the tested polymers did not show any significant methane production (Figures 4, 5). Methane production from plastic degradation is attracting increasing attention as methane is a potent GHG and it was shown that plastic degradation may have an impact on the overall release of methane into the atmosphere (Royer et al., 2018). The fact that the tested polymer degraded in the soil without any significant production of methane is encouraging in terms of the environmental impact of the biodegradation in the soil of future mulching applications with such polymers.
Volatiles profile of polymer biodegradation
Many studies about biodegradation of plastic polymers in soil have overlooked the characterization of non-CO2 volatile products that are being produced during the process. Previous studies recognized the risks of formation of carcinogenic and toxic VOC materialss by plastic degradation (He et al., 2015; Kuchmenko et al., 2020). The present study is among the first to study the profile of VOCs emitted during the biodegradation of plastic polymers in the soil. Cvolatile products of the poly-(disulfanyl-n-decane) shows a distinct profile of volatiles that is markedly different from the reference material and from the control soil, as can be seen from the PCA plot (Figure 7). Additionally, by using the ability of the PTR-TOF-MS to constantly monitor VOCs emission during the entire biodegradation in soil processes, a plot of the time dependent VOCs emission was created (Figure 8). Interesting phenomena were observed in the production of VOCs over time during the biodegradation in soil process.
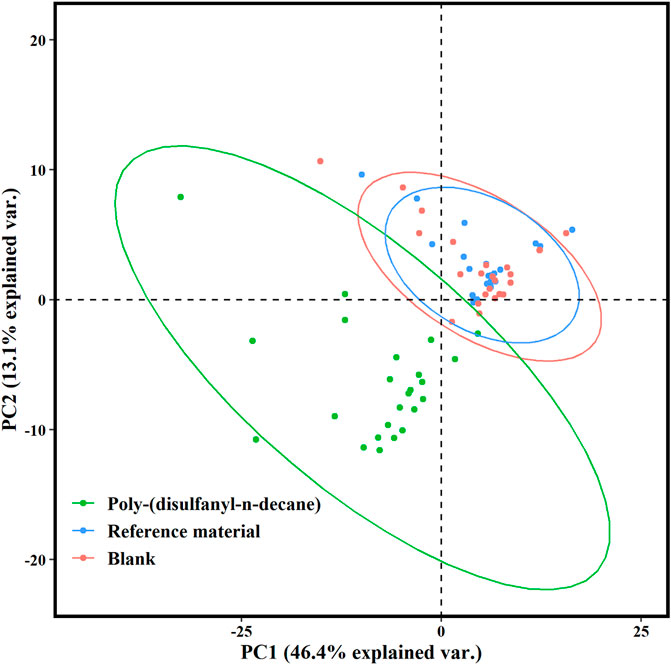
FIGURE 7. Score plot of the principal component analysis (PCA) for PTR-TOF-MS spectral data of the poly-(disulfanyl-n-decane) polymer, the reference material and the blank after 25 days. The first principal component (PC1) and the second principal component (PC2) described 46.4 and 13.1% of the sample variability, respectively.
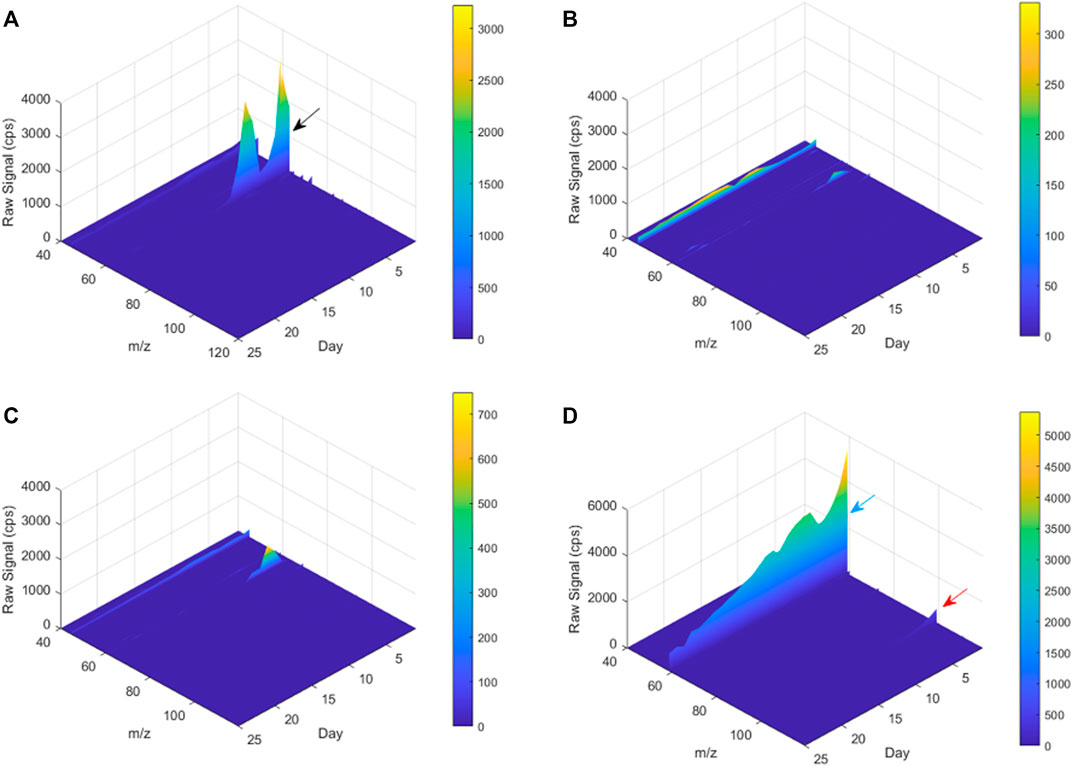
FIGURE 8. A 3D plot of PTR-TOF-MS spectra for m/z 40–120 from Day 1 to Day 25. (A) poly-(disulfanyl-n-decane) and soil mixture, (B) reference material and soil mixture, (C) only soil (blank), (D) only poly-(disulfanyl-n-decane). The black, blue and red arrows mark the peaks of m/z 63, 59 and 99, respectively.
The tested poly-(disulfanyl-n-decane) polymer showed a high concentration of a molecule with an m/z ratio of 63 (Figure 8A) mainly in the initial first 15 days (this molecule is probably ethanethiol), whereas the reference material showed significantly lower amounts of VOCs production during the tested period and also in the blank soil control (Figures 8B,C). When testing the polymer under basic conditions a high emission with an m/z ratio of 59 was observed which might be an indicator for these conditions as it was not observed under natural conditions. The PTR-MS Viewer 3.2 program was used to assess the chemical composition of the VOCs, based on a library of recognized materials (Table 2). Among these recognized molecules, detailed in Table 2, those which could have a special interest are the ones that showed a significantly higher signal coming from the tested poly-(disulfanyl-n-decane) polymer. The major VOCs originating from poly-(disulfanyl-n-decane) polymer were methanethiol, ethanethiol, penta-1,3-diene and 2-pentanone. The presence of these molecules (especially the thiols) might be used as indicators for the biodegradability of poly-(disulfanyl-n-decane) in soil and, as shown in the 3D plot, was predominant at the beginning of the process (Figure 8A). Additional future studies may be able to correlate between the presence and amounts of these molecules and the rate of degradation, as well as to unveil the mechanism of the biodegradation process, in order to see how well the presence and amounts of these molecules can be used to assess the degradation pace of the specific polymer.
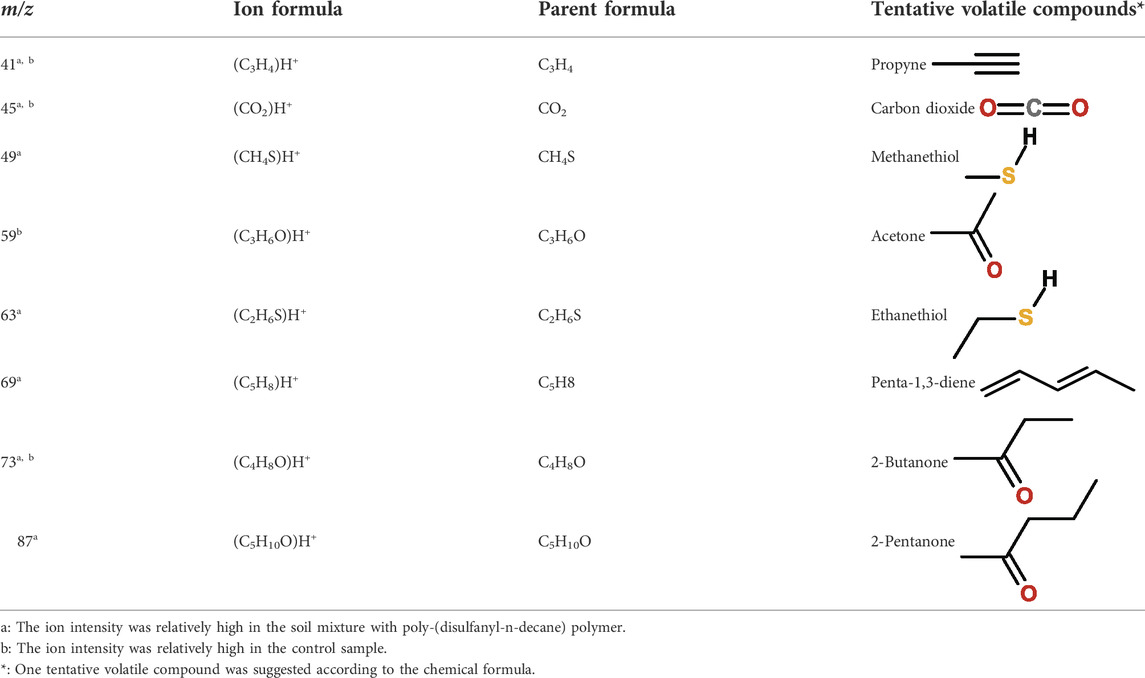
TABLE 2. Tentative volatile compounds detected during the biodegradation of 1,10-decanedithiol polymer.
Culturable microbial profile of polymer biodegradation
Even though the soil microbial population responsible for the degradation of the polymer was not the focus of the current study, a general plating test was performed to see if any changes in this population can be observed (Figure 9). The results clearly indicate that the tested polymer had an impact on the soil microbial population expressed by higher numbers observed in the general count of microbes in the TSA plats. While no major differences were observed in the fungal population in the OGYE media, a distinctive difference was observed in both the Bacillus cereus agar and the gram-negative Eosin Methylene Blue Agar (EMBA) between the soil with the tested polymer and the positive and negative control soils (Figure 9). It should be noted that these are merely initial results that may suggest that a certain shift in the soil microbial population did occur and that a further study, including next generation DNA sequencing, should be carried out in order to better elucidate these changes.
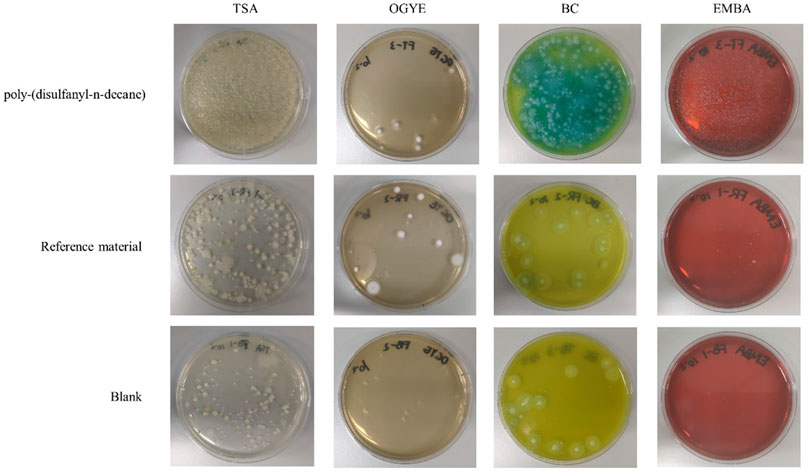
FIGURE 9. Plating of soil mixtures with a dilution of 102 in Tryptic Soy Agar (TSA), Oxytetracycline Glucose Yeast Extract (OGYE), Bacillus cereus Agar (BC) and Eosin Methylene Blue Agar (EMBA) in the end of the biodegradability test.
Conclusions
The current study looked at two new plastic synthetic polymers which are prototype candidates for future biodegradable plastic mulching films. The initial results showed that one of the tested polymers, poly-(disulfanyl-n-decane), may be bio-available and degrade in the soil under standard conditions, especially under slightly basic soil conditions. Poly-(disulfanyl-n-decane) degrades at a rate of losing ∼20% %wt. of the total polymer mass in three months, which might be too fast for mulching films that must retain their properties for periods of up to 6 months. It is important to note that the tests were done in laboratory conditions and the materials were introduced into the soil which means that a longer period in field conditions should also be tested in the future. Poly-(disulfanyl-n-decane) is essentially a polyethylene into which disulfide bridges were inserted in order to allow biological entities, such as microbes, to break the undigestable long alkane chain into segments that are bioavailable and, as the present work shows, also biodegradable. As the results indicate that poly-(disulfanyl-n-decane) has high bioavailability, the next step will be to further dilute the disulfide bridges in the polyethylene skeleton, thereby creating polymers with mechanical and physical properties that are closer to those of ordinary polyethylene, while achieving at the same time also the desired biodegradability rate for mulching films.
A unique simulation system of biodegradation in field conditions allowed monitoring of the VOCs emitted by the degradation of the poly-(disulfanyl-n-decane) polymer. The system showed a specific volatile “fingerprint” containing methanethiol and ethanethiol which suggest degradation of the polymer through a combination of reduction of the disulfide bridges and cleavage of carbon-carbon bonds in positions αcarbon-βcarbon and βcarbon-χcarbon relative to the sulfur atom. Monitoring of these materials might serve as markers for determining the rate of biodegradation of this polymer in the future. The microbial population of the soil appears to be impacted by the incorporation of the poly-(disulfanyl-n-decane) polymer into the soil. Future tests will be needed to further tailor this polymer for agricultural usage and to further understand its impact on the soil biological, chemical and physical properties as well as for looking at the fate of the degradation products in real agricultural systems.
Data availability statement
The raw data supporting the conclusions of this article will be made available by the authors, without undue reservation.
Author contributions
All authors listed have made a substantial, direct, and intellectual contribution to the work and approved it for publication.
Funding
This research was partially supported by Li Ka Shing Foundation (LKSF) Cross-Disciplinary Research Grant (#2020LKSFG07D) and “Climbing Plan” project to be funded by the Guangdong Provincial Science and Technology Innovation Strategy Special Fund. The authors acknowledge the support of the Guangdong Provincial Key Laboratory of Materials and Technologies for Energy Conversion. Supported by the 2021 Guangdong Special Fund for Science and Technology, Multi-effect valorization of tea waste by soil biosolarization and restoration of farmland soil ecosystem/土壤生物日晒对茶渣的多效循环利用及农田土壤生态系统的修复, (#STKJ2021128) and Special funds for higher education development of Guangdong Province.
Acknowledgments
The authors acknowledge the support of the Guangdong Provincial Key Laboratory of Materials and Technologies for Energy Conversion.
Conflict of interest
The authors declare that the research was conducted in the absence of any commercial or financial relationships that could be construed as a potential conflict of interest.
Publisher’s note
All claims expressed in this article are solely those of the authors and do not necessarily represent those of their affiliated organizations, or those of the publisher, the editors and the reviewers. Any product that may be evaluated in this article, or claim that may be made by its manufacturer, is not guaranteed or endorsed by the publisher.
References
Achmon, Y., Dowdy, F. R., Simmons, C. W., Zohar-Perez, C., Rabinovitz, Z., Nussinovitch, A., et al. (2019). Degradation and bioavailability of dried alginate hydrocolloid capsules in simulated soil system. J. Appl. Polym. Sci. 136, 48142. doi:10.1002/app.48142
Achmon, Y., Fernández-Bayo, J. D., Hernandez, K., McCurry, D. G., Harrold, D. R., Su, J., et al. (2017). Weed seed inactivation in soil mesocosms via biosolarization with mature compost and tomato processing waste amendments. Pest Manag. Sci. 73, 862–873. doi:10.1002/ps.4354
Achmon, Y., Sade, N., Wilhelmi, M., del, M. R., Fernández-Bayo, J. D., Harrold, D. R., et al. (2018). Effects of short-term biosolarization using mature compost and industrial tomato waste amendments on the generation and persistence of biocidal soil conditions and subsequent tomato growth. J. Agric. Food Chem. 66, 5451–5461. doi:10.1021/acs.jafc.8b00424
Ahmed, T., Shahid, M., Azeem, F., Rasul, I., Shah, A. A., Noman, M., et al. (2018). Biodegradation of plastics: Current scenario and future prospects for environmental safety. Environ. Sci. Pollut. Res. 25, 7287–7298. doi:10.1007/s11356-018-1234-9
Briassoulis, D., Babou, E., Hiskakis, M., and Kyrikou, I. (2015). Analysis of long-term degradation behaviour of polyethylene mulching films with pro-oxidants under real cultivation and soil burial conditions. Environ. Sci. Pollut. Res. 22, 2584–2598. doi:10.1007/s11356-014-3464-9
Briassoulis, D., and Dejean, C. (2010). Critical review of norms and standards for biodegradable agricultural plastics part Ι. Biodegradation in soil. J. Polym. Environ. 18, 384–400. doi:10.1007/s10924-010-0168-1
Briassoulis, D., Mistriotis, A., Mortier, N., and Tosin, M. (2020). A horizontal test method for biodegradation in soil of bio-based and conventional plastics and lubricants. J. Clean. Prod. 242, 118392. doi:10.1016/j.jclepro.2019.118392
Emadian, S. M., Onay, T. T., and Demirel, B. (2017). Biodegradation of bioplastics in natural environments. Waste Manag. 59, 526–536. doi:10.1016/j.wasman.2016.10.006
EN17033 Plastics (2018). EN17033 Plastics - biodegradable mulch films for use in agriculture and horticulture - requirements and test methods. Available at: https://www.en-standard.eu/din-en-17033-plastics-biodegradable-mulch-films-for-use-in-agriculture-and-horticulture-requirements-and-test-methods.
Fernandes, M., Salvador, A., Alves, M. M., and Vicente, A. A. (2020). Factors affecting polyhydroxyalkanoates biodegradation in soil. Polym. Degrad. Stab. 182, 109408. doi:10.1016/j.polymdegradstab.2020.109408
He, Z., Li, G., Chen, J., Huang, Y., An, T., Zhang, C., et al. (2015). Pollution characteristics and health risk assessment of volatile organic compounds emitted from different plastic solid waste recycling workshops. Environ. Int. 77, 85–94. doi:10.1016/j.envint.2015.01.004
Huang, Y., Liu, Q., Jia, W., Yan, C., and Wang, J. (2020). Agricultural plastic mulching as a source of microplastics in the terrestrial environment. Environ. Pollut. 260, 114096. doi:10.1016/j.envpol.2020.114096
ISO (2019). Plastics — determination of the ultimate aerobic biodegradability of plastic materials in soil by measuring the oxygen demand in a respirometer or the amount of carbon dioxide evolved. Available at: https://www.iso.org/standard/74993.html.26
Kasirajan, S., and Ngouajio, M. (2012). Polyethylene and biodegradable mulches for agricultural applications: A review. Agron. Sustain. Dev. 32, 501–529. doi:10.1007/s13593-011-0068-3
Kuchmenko, T., Umarkhanov, R., and Lvova, L. (2020). E-nose for the monitoring of plastics catalytic degradation through the released Volatile Organic Compounds (VOCs) detection. Sensors Actuators B Chem. 322, 128585. doi:10.1016/j.snb.2020.128585
Kyrikou, I., and Briassoulis, D. (2007). Biodegradation of agricultural plastic films: A critical review. J. Polym. Environ. 15, 125–150. doi:10.1007/s10924-007-0053-8
Liu, Y., Huang, Q., Hu, W., Qin, J., Zheng, Y., Wang, J., et al. (2021). Effects of plastic mulch film residues on soil-microbe-plant systems under different soil pH conditions. Chemosphere 267, 128901.
Royer, S.-J., Ferrón, S., Wilson, S. T., and Karl, D. M. (2018). Production of methane and ethylene from plastic in the environment. PLoS One 13, e0200574. Available at: doi:10.1371/journal.pone.0200574
Sander, M. (2019). Biodegradation of polymeric mulch films in agricultural soils: Concepts, knowledge gaps, and future research directions. Environ. Sci. Technol. 53, 2304–2315. doi:10.1021/acs.est.8b05208
Sevilia, S., Parvari, G., Bernstein, J., Fridman, N., Grinstein, D., Gottlieb, L., et al. (2022). Imidazolium based energetic materials. Chem. Select 7, e202200322. doi:10.1002/slct.202200322
Tada, Y., Yamamoto, T., Tezuka, Y., Kawamoto, T., and Mori, T. (2012). Effective synthesis and crystal structure of a 24-membered cyclic decanedisulfide dimer. Chem. Letters 41 (12), 1678–1680. doi:10.1246/cl.2012.1678
Thomas, Z. M., Arno, S., Frederick, N. T., Rebekka, B., Dagmar, W., Michael, W., et al. (2021). Biodegradation of synthetic polymers in soils: Tracking carbon into CO2 and microbial biomass. Sci. Adv. 4, eaas9024. doi:10.1126/sciadv.aas9024
Zertal, Y., Yong, M., Levi, A., Sevilia, S., Tsoglin, A., Parvari, G., et al. (2022). Alkyl vinyl imidazolium ionic liquids as fuel-binders for photo-curable energetic propellants. Adv. Funct. Mat.. In press. doi:10.1021/acsapm.2c00499
Zhang, H., Miles, C., Ghimire, S., Benedict, C., Zasada, I., DeVetter, L., et al. (2019). Polyethylene and biodegradable plastic mulches improve growth, yield, and weed management in floricane red raspberry. Sci. Hortic. 250, 371–379. doi:10.1016/j.scienta.2019.02.067
Zhou, B., Wang, J., Zhang, H., Shi, H., Fei, Y., Huang, S., et al. (2020). Microplastics in agricultural soils on the coastal plain of Hangzhou Bay, east China: Multiple sources other than plastic mulching film. J. Hazard. Mat. 388, 121814. doi:10.1016/j.jhazmat.2019.121814
Zumstein, M. T., Narayan, R., Kohler, H.-P. E., McNeill, K., and Sander, M. (2019). Dos and do nots when assessing the biodegradation of plastics.
Keywords: plastic biodegradation, soil respiration, microbial volatile organic compounds, PTR-ToF-MS (proton transfer reaction time-of-flight mass spectrometry), disulfide-based polymer binder
Citation: Dar SU, Wu Z, Zhang L, Yu P, Qin Y, Shen Y, Zou Y, Poh L, Eichen Y and Achmon Y (2022) On the quest for novel bio-degradable plastics for agricultural field mulching. Front. Bioeng. Biotechnol. 10:922974. doi: 10.3389/fbioe.2022.922974
Received: 18 April 2022; Accepted: 28 June 2022;
Published: 08 August 2022.
Edited by:
Zhanyong Wang, Shenyang Agricultural University, ChinaReviewed by:
Antonis Mistriotis, Agricultural University of Athens, GreecePaola Rizzarelli, National Research Council (IPCB CNR), Italy
Copyright © 2022 Dar, Wu, Zhang, Yu, Qin, Shen, Zou, Poh, Eichen and Achmon. This is an open-access article distributed under the terms of the Creative Commons Attribution License (CC BY). The use, distribution or reproduction in other forums is permitted, provided the original author(s) and the copyright owner(s) are credited and that the original publication in this journal is cited, in accordance with accepted academic practice. No use, distribution or reproduction is permitted which does not comply with these terms.
*Correspondence: Yigal Achmon, eWlnYWwuYWNobW9uQGd0aWl0LmVkdS5jbg==
†These authors have contributed equally to this work
‡Present address: Permanent Address, Schulich Faculty of Chemistry, Technion—Israel Institute of Technology, Technion City, Israel
§ORCID: Sami Ullah Dar, orcid.org/0000-0003-0131-6376; Yigal Achmon, orcid.org/0000-0003-2120-3096