- 1Department of Life Science and Institute of Biotechnology, National Dong Hwa University, Hualien, Taiwan
- 2Department of Chemistry, National Dong Hwa University, Hualien, Taiwan
- 3Medical Imaging Research Center, Institute for Radiological Research, Chang Gung University, Taoyuan, Taiwan
- 4Department of Neurology, Chang Gung Memorial Hospital, College of Medicine, Chang Gung University, Taoyuan, Taiwan
Peptide conformational imprints (PCIs) offer a promising perspective to directly generate binding sites for preserving enzymes with high catalytic activity and stability. In this study, we synthesized a new chiral cross-linker cost-effectively for controlling the matrix morphology of PCIs on magnetic particles (PCIMPs) to stabilize their recognition capability. Meanwhile, based on the flank part of the sequences on papain (PAP), three epitope peptides were selected and synthesized. Molecularly imprinted polymers (MIPs) were then fabricated in the presence of the epitope peptide using our new cross-linker on magnetic particles (MPs) to generate PCIMPs. PCIMPs were formed with helical cavities that complement the PAP structure to adsorb specifically at the targeted position of PAP. PCIMPs65–79 were found to have the best binding parameters to the PAP with Kd = 0.087 μM and Bmax = 4.56 μM. Upon esterification of N-Boc-His-OH, proton nuclear magnetic resonance (1H-NMR) was used to monitor the yield of the reaction and evaluate the activity of PAP/PCIMPs. The kinetic parameters of PAP/PCIMPs65–79 were calculated as Vmax = 3.0 μM s−1, Km = 5 × 10−2 M, kcat = 1.1 × 10–1 s−1, and kcat/Km = 2.2 M−1 s−1. In addition, PAP is bound tightly to PCIMPs to sustain its activity after four consecutive cycles.
Introduction
Papain (PAP) is a cysteine protease (EC 3.4.22.2) found in papaya tease. Its substrate contains arginine or lysine residue and is commonly used in the food industry. PAP acts as a highly specific and effective biocatalyst and has been reported to catalyze carbon–carbon formation in organic synthesis (Cajnko et al., 2020; Cajnko et al., 2021; Bjelić et al., 2022). It operated under mild reaction conditions and separated easily from the reaction mixture (Morcelle et al., 2006; Jeong et al., 2011; Llerena-Suster et al., 2012; Cao et al., 2015). PAP-mediated esterification has been studied previously. It decreases the environmental impact of chemical alterations that typically require acyl chlorides or toxic coupling agents. In fact, PAP is usually utilized for trans-esterification of alkyl or vinyl esters in a medium with low water content (Prabhakar et al., 2017; Marathe et al., 2020). Moreover, PAP was also found to possess esterase activity in a biphasic system or an aqueous solution, and N-Boc amino acid esters were synthesized (Cantacuzène et al., 1987; de Beer et al., 2012). PAP shows remarkable catalytic performance in esterification, transesterification, and hydrolysis (Jeong et al., 2011; Anwar et al., 2017), but some of its properties may not fit industrial requirements due to inherent limitations such as lack of reusability, instability in organic solvents at high temperatures, and denaturing at different pH ranges (Homaei et al., 2010; Sheldon, 2011; Homaei, 2015).
Over the last five decades, numerous methods have been developed to immobilize all classes of enzymes. Enzyme immobilization technology was developed to reduce drawbacks and make them reusable at a commercial level (Homaei et al., 2013; Mohamad et al., 2015; Liang et al., 2021). Immobilized enzymes as catalysts were reviewed (Basso and Serban, 2019; Kankala et al., 2019). Previously, Tai and colleagues immobilized PAP on Sephadex G-50 to convert N-protected amino acids to their methyl esters (Tai et al., 1989). Since then, many efforts have been dedicated to utilizing PAP as a biocatalyst for diverse catalytic applications. For example, Storer and colleagues immobilized PAP on celite (PAP/celite) to catalyze the esterification of Cbz-glycine with methanol as a substrate in 12 different solvents of widely varying polarity (Stevenson and Storer, 1991). N-Cbz-L-alanine was converted to its corresponding esters with 2-phenethyl alcohol by using PAP/celite (Shih et al., 1997). Although these techniques can improve enzyme stability, they might also be accountable for restrictions in local and global protein flexibility. Inevitably, protein conformation was unstable under harsh synthesis conditions (Secundo, 2013; Hoarau et al., 2017). Enzymes lose their structure orientation during immobilization processes, which plays a crucial role in reducing enzymatic activity (Basso and Serban, 2019; Nguyen et al., 2019).
The fabrication of peptide conformational imprints (PCIs) on magnetic particles (PCIMPs) is a delicate process to immobilize enzymes (Chou et al., 2020; Kanubaddi et al., 2021). A helical peptide fragment of the enzyme was used as a template to form molecularly imprinted polymers (MIPs). As helical peptide segment-mediated PCIMPs were constructed, elegant helical cavities complementary to the enzyme structure can be achieved (Chou et al., 2020; Kanubaddi et al., 2021). By taking this dynamic property into account, the selection of the template (Figure 1) was crucial to generate helical cavities using PCIs. Binding enzymes tightly at the target position is the key issue in keeping enzymes flexible as well as stable. The advantage of this method is to create accessible binding sites on the surface of MPs and enable catalytic active sites with less interference during catalysis. The novelty of this work is to monitor the esterification yield of Boc-His-OH directly using 1H-NMR with great accuracy; demonstrate the activation effect of PCIMPs on the esterification activity of PAP; and sustain the esterification process of PAP/PCIMPs for four consecutive cycles. Finally, the immobilized enzyme is contemplated to further increase the efficiency and convenience in the catalysis of the reverse reaction of hydrolysis.
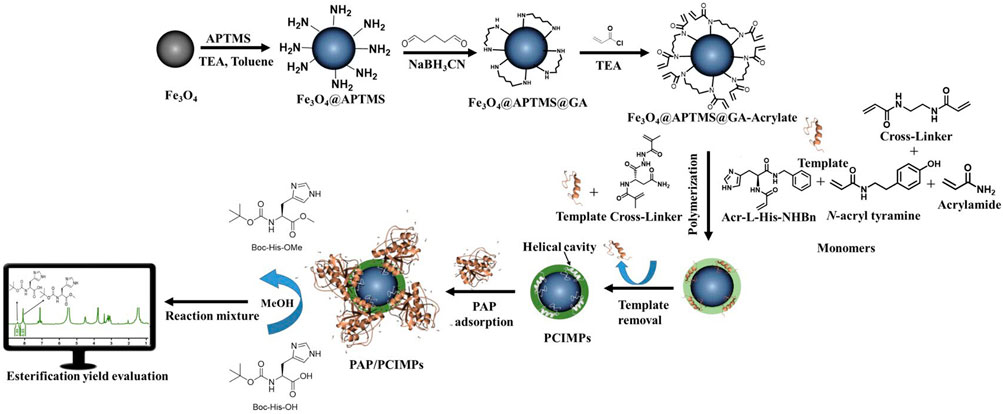
FIGURE 1. Schematic diagram of the (Top) fabrication of MPs to PCIMPs using cross-linker, monomers, and template (Bottom) application of PCIMPs using their binding mechanisms toward PAP and PAP/PCIMPs catalyzed esterification and yield evaluation under the monitor. APTMS, 3-aminopropyl trimethoxysilane; TEA, trimethylamine; GA, glutaraldehyde; MPs, magnetic particles; PAP, papain; PCIs, peptide conformational imprints; PCIMPs, peptide conformational imprint magnetic particles.
Our research methods can be divided into four main categories: 1) synthesis of a cross-linker and monomers from asparagine; 2) fabrication of PCIMPs using a single cross-linker; 3) adsorption of PAP to PCIMPs to obtain binding parameters; 4) operation of PAP/PCIMP-catalyzed esterification to observe enzyme kinetics.
Materials and methods
The synthesis of the monomers is described in our previous studies. Briefly, Acr-L-His-NHBn (Tai et al., 2011) was prepared using the following steps. Initially, Boc-L-His-OMe was synthesized using Boc-His-OH and methanol by adding PAP as a catalyst (Tai et al., 1989). Then, the obtained Boc-L-His-OMe was converted to Boc-L-His-NHBn using benzylamine in the presence of PAP. Next, trifluoroacetic acid was used to deprotect the Boc group to form L-His-NHBn (Tai, 2003); then, acrylation of L-His-NHBn was carried out to obtain Acr-L-His-NHBn. Finally, another monomer, N‐acryl tyramine, was prepared by acylation with acrylic chloride of tyramine hydrochloride (Singh et al., 2013).
Synthesis of a cross-linker (Metha-Asn-NHNH-Metha)
Scheme 1 shows the synthetic route of the cross-linker. Briefly, a mixture of Boc-L-Asn-OH (1 g, 1 eq) and Boc-NHNH2 (0.68 g, 1.2 eq) was dissolved in a water (H2O)/tetrahydrofuran (THF) mixture (1:1) and stirred for a few minutes. Later, 3-(ethyliminomethyleneamino)-N,N-dimethyl-propan-1-amine hydrochloride (EDC⋅HCl; 0.828 g, 1 equiv) was added portion-wise to the solution, stirred for 4 hours, and monitored by thin layer chromatography (TLC). The solution was extracted into ethyl acetate (EtOAc, 10 ml) and washed with 0.1 N hydrochloric acid (HCl), followed by H2O and saturated brine solution. The organic layer was passed through sodium sulfate (Na2SO4). The solvent was removed under a rotary evaporator to obtain 2 as a white solid (1.0 g, 67%). Boc-L-Asn-NHNH-Boc 2 (1 g) was dissolved in 10 ml of methanolic HCl (4 M). The mixture was stirred for 4 h at 0°C. After that, the solvent was evaporated under the rotary evaporator at room temperature (RT) and washed three times with diethyl ether (Et2O, 10 ml) to form a precipitate. The solid was dried under vacuum at 0°C to obtain 0.5 g of L-Asn-NHNH2 3. It was then dissolved in 10 ml of dry dichloromethane (DCM) and flushed with nitrogen (N2). Methacrylic anhydride (2 equiv.), followed by N,N-diisopropylethylamine (DIPEA; 5 equiv) were added dropwise and stirred overnight at 0°C under N2. Finally, the solution was extracted into DCM. The organic layer was washed with 0.1 N HCl, followed by H2O and saturated brine solution. The organic layer was mixed with anhydrous Na2SO4 to remove moisture and was purified by column chromatography. A solid was precipitated with a mixture of DCM and hexane solvents, which was further dried at 0°C and stored at −5°C. In this way, cross-linker 4 was obtained with a 48% yield (see the entire process in Supplementary Figure S2).
Synthesis of the template
The peptide segments, such as PAP65–79 (GGYPW SALQLVAQYG), PAP65-78 (GGYPWSALQLVAQY), PAP66-79 (GYPWSALQLVAQYG), and PAP66-78 (GYPWSALQLVAQY) were synthesized using solid-phase chemistry of the fluorenylmethoxycarbonyl (Fmoc) method (Collins et al., 2014). The template (peptide residues) was synthesized using a CEM Discover Microwave-assisted Peptide synthesizer (Kohan Co., Taipei, Taiwan) as described previously (Collins et al., 2014; Lin et al., 2019; Kanubaddi et al., 2021). Subsequently, the purity of these peptides was monitored by HPLC equipped with an RP-18, using a mobile phase of 75:25 v/v methanol/water at RT and at a flow rate of 1 ml/min. Sharp peaks were observed at a retention time of ∼19 min, known as a template (Supplementary Figure S1A); these peptides’ purity was observed to be ∼88%. The template samples such as PAP65–79, PAP65–78, and PAP66–79 were analyzed using a Shimadzu Matrix-Assisted Laser Desorption/Ionization-Time of Flight (MALDI/TOF) mass spectrometer (MS) (Kyoto, Japan), and PAP66-78 (GYPWSALQLVAQY) was analyzed using Bruker Autoflex MALDI/TOF mass spectrometry (Germany) with 2,5-dihydroxybenzoic acid (DHB) as the matrix. of The sharp peaks of PAP66-78 were observed in the HPLC chromatogram at a retention time of ∼3 min, and purity was observed to be ∼95% (Supplementary Figure S1C). The reported m/z values of PAP65–79, PAP65–78, PAP66–79, and PAP66–78 were observed, respectively, at 1610.78 [M + H]+, 1552.50, 1552.74 as shown in Supplementary Figure S1B, and 1517.951 [M + Na]+ (Supplementary Figure S1D).
Preparation of PCIMPs
The synthesis of Fe3O4, Fe3O4@APTMS, Fe3O4@APTMS-GA, and Fe3O4@APTMS-GA-acrylate was carried out as described previously (Ding et al., 2006; Yang et al., 2017; Kanubaddi et al., 2021). Furthermore, for the preparation of PCIMPs using a cross-linker, 5.6 mM of the cross-linker (Metha-Asn-NHNH-Metha) and 0.014 mM of the template (PAP65–79, PAP65-78, or PAP66-79) were dissolved in 12 ml of trifluoroethanol (TFE)/H2O = 7: 3. For fabrication of PCIMPs using monomers, acrylamide (AA) (0.24 mM), N-Acr-L-His-NHBn (0.24 mM), N-acryltyramine (0.48 mM), and the cross-linker N,N′-ethylene bisacrylamide (0.84 mM), and 0.014 mM of the template (PAP66-78) were dissolved in 6 ml of TFE/ H2O = 7: 3. The combination was stirred for 1 h to make a pre-self-assembled mixture. Then 100 mg of Fe3O4@APTMS-GA-acrylate was added to the mixture and stirred for another 1 h, followed by 500 μl (10%, W/W) of ammonium persulfate (initiator) and 250 μl (5%, W/V) of tetramethylethylenediamine (TEMED), stirred at RT for 24 h in the presence of N2. Next, the resultant mixture was washed four times with 5% acetic acid (aq), containing 0.5% tween@20 and rinsed with H2O. Finally, the pore structures formed from different template molecules and were denoted as PCIMPs65–79, PCIMPs65–78, and PCIMPs66–79 (Kanubaddi et al., 2021). The compositions of other PCIMPs are given in Supplementary Table S1;these were synthesized using the same procedure.
Physicochemical characterization of MPs and PCIMPs
The presence of PCIs fabricating on MPs was confirmed using Fourier transform infrared (FT-IR) spectroscopy (Bruker TENSOR 27, Ettlingen, Germany). The morphology of functionalized MPs and PCIMPs was observed under a Field Emission-Scanning Electron Microscope (FE-SEM, JOEL JSM-7000F/JEOL Ltd. Japan) equipped with an Oxford Instruments X-Max EDS system and operated at an acceleration voltage of 200 kV. The elemental analysis was performed by energy-dispersive X-ray spectroscopy (EDS). To demonstrate the amine groups on the surface of MPs, Ninhydrin reagent in the detection of grafted functional groups was assessed. For the Ninhydrin test (Albert Brown Ltd., Leicester, United Kingdom), the samples were placed into the Ninhydrin gel vials provided and incubated at 60°C for 30 min. The vials were then inspected and scored according to the following scale: 0, no color (negative); 1, slight purple color; and 2, dark purple color.
Determination of binding affinities of PCIMPs
To prevent the non-specific binding sites' adsorption on PCIMPs, can binding experiments were carried out for a few minutes. Briefly, 20 mg of PCIMPs was suspended in 1 ml of H2O containing specific initial PAP concentrations (0.125, 0.25, 0.5, 1.0, and 2.0 mg/ml). After being shaken at 25°C for 5 min, the mixture was separated using a magnet. Then, 200 µl of the supernatant was taken out and measured using a Fluorescence Microplate Reader at Eex/Eem = 290 nm/350 nm. The binding affinities of PCIMPs were evaluated using the Scatchard analysis equation (1) (Gerdon et al., 2005; Diltemiz et al., 2009; Tai et al., 2012; Kanubaddi et al., 2021),
where [L] is the concentration of PAP in the solution, [RL] is the concentration of the bound PAP from the solution, Bmax represents the maximum number of binding sites, and Kd is the ligand dissociation constant.
Immobilization of papain (PAP/PCIMPs)
Briefly, 10 mg of PAP was dissolved in 1 ml of H2O and 20 mg of PCIMPs was added to the solution to incubate for 4 h. The resulting PAP/PCIMPs were collected and washed with H2O. Finally, the PAP/PCIMPs obtained were dried at 0°C and stored in a sealed vial at 4°C until further use.
Determination of esterification activity of PAP and immobilization PAP (PAP/PCIMPs) by the 1H-NMR method
The esterification activity of PAP and PAP/PCIMPs was determined using the 1H-NMR method. The starting material was Boc-L-His-OH and the product was Boc-L-His-OMe, which was observed over time. The percentage of esterification rate was calculated by using the following equation (2):
To determine the esterification activity of PAP and PAP/PCIMPs, a solution of Boc-L-His-OH (0.1 M) was prepared in dry methanol (MeOH). Then, an enzymatic reaction in organic solvents was carried out, with modifications of earlier reported methods (Zaks and Klibanov, 1985; Belyaeva et al., 2002). First, 20 mg of PAP was added to Boc-L-His-OH (0.1 M) in 2 ml of MeOH, followed by 50 μl of water. Then, the reaction was carried out at 20°C for 48 h. For immobilized enzyme, 20 mg of PAP/PCIMPs was added to a Boc-L-His-OH (0.1 M) solution and incubated for 48 h, and the product concentration was monitored for 48 h with samples taken every 8 h by 1H-NMR. The same procedure was also used for the adsorption test. For de-adsorption, we used acetonitrilcan (ACN): H2O to remove the PAP, and repeated the process four times.
Determination of kinetic constants of PAP and PAP/PCIMPs
The kinetic parameters of PAP and PAP/PCIMPs were determined using varying concentrations of Boc-L-His-OH (0.06, 0.08, and 0.1 M) in 2 ml of dry MeOH. Then, 20 mg of PAP/PCIMPs was added to each concentration and incubated. For every 8 h interval, 100 μl of the aliquot was withdrawn, dried in a vacuum, and monitored by 1H-NMR. Finally, the kinetic parameters of PAP and PAP/PCIMPs were evaluated using the Michaelis–Menten kinetics plot obtained from the following equation 3:
where v is the velocity, Vmax is the maximum rate of enzyme activity, [S] is the substrate’s concentration, and Km is the Michaelis half-saturation constant.
The turnover number (kcat) was determined using the following equation 4:
where Vmax is the maximum rate of enzyme activity and [E] is the concentration of the enzyme (Bossi et al., 2012).
Reusability analysis
To demonstrate the reusability performance of the PCIMPs’ imprinted materials, stable catalytic activity was compared with the PAP-immobilized Sephadex G-25 (Tai et al., 1989) and PAP, respectively. The PAP/PCIMPs65–79 were also examined with the same catalytic activity at different times to determine reuse performance.
Results and Discussion
Rational selection of the template and its analysis
To obtain unique protein recognition on the surface of MPs, the helical peptide residues in the template are the critical parameters. The selection of template peptide fragments is based on the flank part of the spatial protein structure to limit their interference during catalysis. The length of the peptide segments such as 14–15-mer from the flexible structure on the surface of MPs can be helpful during the process of protein rebinding (Tai et al., 2011; Bossi et al., 2012; Kanubaddi et al., 2021). Therefore, the chosen 14–15-mer peptide sequences containing PAP65–79, PAP65–78, and PAP66–79 of the PAP were selected as a template. The location of the template is shown in Figure 2, and the list of the peptides is described in Table 1.
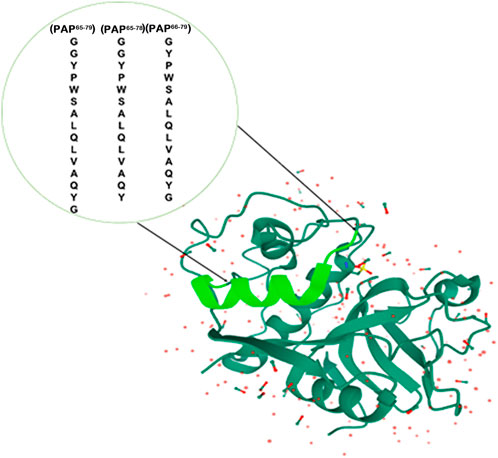
FIGURE 2. The selected sequence is in pale green. These segments consist of the series, i.e., PAP65–79, PAP65–78, and PAP66–79. (The crystal structure of PAP was reproduced from https://www.rcsb.org/3d-view/9PAP, and PDB ID: 9PAP.)
Helical conformational analysis
The helical structure of our designed peptides (PAP65–79, PAP65–78, and PAP66–79) were examined using a J-715 spectropolarimeter (Jasco Inc., Japan). The synthesized peptides gave a well-defined secondary conformation by circular dichroism (CD) of the peptide solution (20 mM; TFE/H2O = 7:3). As shown in Figure 3, the CD spectra exhibited positive peaks at around 193 nm and two minimum negative bands at 207 and 218 nm, indicating α-helix structures’ predominance. Accordingly, the peptides were stabilized as a helical conformation in a mixed solvent (TFE/H2O) to allow the formation of helical cavities with a PCIMPs-based method (Chou et al., 2020; Kanubaddi et al., 2021).
Preparation of a cross-linker (Metha-L-Asn-NHNH-Metha)
The synthesis of the cross-linker is straightforward, as outlined in Scheme 1. First, Boc-L-Asn-OH 1 was coupled with Boc-NHNH2 via EDC to generate 2. The Boc protecting group of 2 was then deprotected using MeOH⋅HCl to obtain 3. Finally, after acylating with twofold methacryloyl chloride, cross-linker 4 was obtained. The role of the synthesized cross-linker is also kind of a functional monomer. The cross-linker can form more rigid PCIMPs for improving the binding affinity and forming stable PCIMPs. It was attributed to cooperative hydrogen bonding or electrostatic interactions with the protein molecule. Moreover, it possesses an amino group that can easily interact with the substrate to enhance the productive catalytic activity of PAP/PCIMPs.
Proposed mechanism of formation of PCIMPs and their interaction with the template and PAP
Because of self-assembly, the amino group of the cross-linkers attached to the template with ionic bonding and hydrogen bonding. Meanwhile, the monomers, cross-linkers, and Fe3O4@APTMSGA-acrylate were attached to each other with the hydrophobic acryloyl group. All the monomers and cross-linkers were thus attached to the template and Fe3O4@APTMS-GA-acrylate in a preorganized manner. Afterward, the formulation of PCIMPs took place in an organized manner. The free-radical polymerization process of a cross-linker was initiated by ammonium persulfate and TEMED. TEMED accelerates the rate of formation of free radicals from persulfate. These, in turn, catalyze polymerization. The persulfate free radical converts the methacrylate group of the cross-linker to free radicals, which react with unreacted ones to begin the polymer chain reaction. The elongating polymer chain is randomly cross-linked with Fe3O4@APTMS-GA-acrylate, resulting in the production of 3D polymer networks; the interaction of the polymer with the template via ionic bonding and hydrogen bonding to produce a template–PCIMPs complex. This is formed in a large excess of a crosslinking agent to form a 3D polymer network. After the polymerization process, template molecules are removed using an amphiphilic solvent. The role of the synthesized cross-linker is also of a dual-function monomer. The cross-linker can form more rigid PCIMPs to improve the binding affinity and stabilize PCIMPs. It was attributed to cooperative hydrogen bonding, ionic bonding, and electrostatic interactions with the protein molecule. Moreover, it possesses an amino group that can easily interact with the substrate to enhance the catalytic activity of PAP/PCIMPs.
Physical characterization of MPs and PCIMPs
The fabrication of MPs is shown in Figure 4A, and is characterized by Fourier transform infrared (FT-IR) spectroscopy (Bruker TENSOR 27, Ettlingen, Germany). In the beginning, the bare Fe3O4 sample reflected only a few functional groups prominently, such as iron oxide and hydroxide groups. The broad peak at around 582 cm−1 was ascribed to the Fe-O, and a peak at 3,396 cm.−1 (Figure 4A,a). Later, APTMS immobilized on Fe3O4 resulted in additional peaks around 1,032 cm−1, 1,120 cm−1, and 1,560 cm.1 (C-N vibration), and a maximum at 3,420 cm−1, which could be ascribed to the silanol group (Si-O) and NH2 (Kurtan and Baykal, 2014; Farjadian et al., 2016; Yang Y. et al., 2018; Gao et al., 2018; Kanubaddi et al., 2021), respectively. These characteristic peaks of APTMS molecules indicate their successful coating on Fe3O4 (Figure 4A,b). In addition, the C-H stretching peaks at ∼ 2,900 cm−1 are due to the presence in the alkyl chain of APTMS43-44. Besides, no peak was observed at 1,739 cm−1, indicating that C=O at both ends of the glutaraldehyde functional groups reacted with NH2. This was attributed to the formation of a secondary amine. The unique peak at 3,414 cm−1 was ascribed to the N-H group (Figure 4A,c). Further, as seen in Figure 4A,d, the peak at 1,630 cm.−1, ascribed as the characteristic peak of C=C, suggests successfully conjugated acrylation on MPs (Secundo, 2013; Farjadian et al., 2015; Kanubaddi et al., 2021).
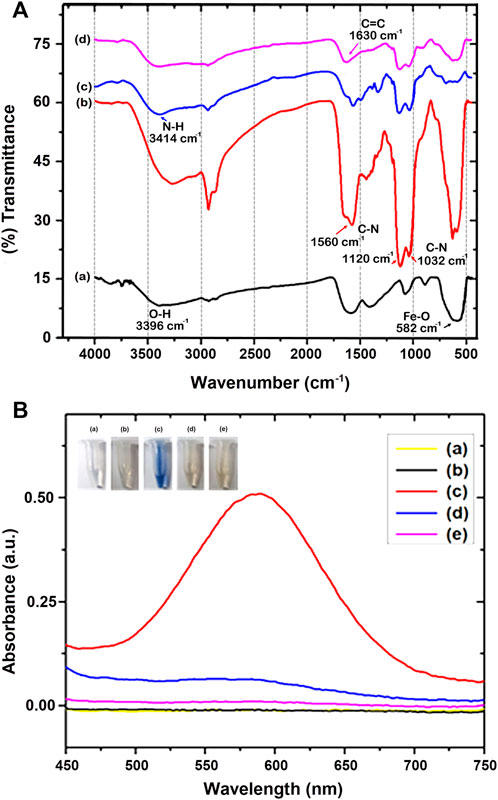
FIGURE 4. (A) FT-IR spectra of (a) Fe3O4, (b) Fe3O4@APTMS, (c) Fe3O4@APTMS-GA, and (d) Fe3O4@APTMS-GA-acrylate. (B) Ninhydrin assay (a) Ninhydrin, (b) Fe3O4, (c) Fe3O4-APTMS, (d) Fe3O4-APTMS@GA, and (e) Fe3O4-APTMS@GA-acrylate.
Furthermore, the successful modification of the amine group on the surface of Fe3O4 was confirmed by the Ninhydrin test (Figure 4B), using our established method (Kuo et al., 2015). We treated the modified Fe3O4 samples with a 1 ml Ninhydrin solution at 60°C for 30 min. After the reaction, the MPs were centrifuged. The solvent was measured in UV-Vis, and the characteristic absorption of Ruhemann’s purple was shown at 580 nm., which can be labeled as primary amine-modified on the surface of Fe3O4. However, when treated with secondary amine-modified Fe3O4 nanoparticles (Fe3O4@APTMS-GA), ninhydrin cannot produce Ruhemann’s purple color (Kuo et al., 2015). When we compared Ruhemann’s purple absorbent intensities, Fe3O4-APTMS showed high intensity, whereas Fe3O4@APTMS-GA and Fe3O4@APTMS-GA-acrylate samples showed low intensities. This shows that GA is successfully modified on the amine-modified Fe3O4 nanoparticles. The reactivity of ninhydrin with various surface-functionalized Fe3O4 samples provides further evidence to confirm successive chemical modifications on the Fe3O4 surfaces.
Eventually, the surface morphology of functionalized MPs and PCIMPs was subsequently characterized by Field Emission-Scanning Electron Microscopy (FE-SEM) observations (JEOL JSM-7000F/JEOL Ltd. Microscope from Tokyo, Japan). It was apparent from the FE-SEM images of Fe3O4 nanoparticles that their successively surface-functionalized samples were uniform, and their spherical shape can be seen in Figure 5. We determined the shape of MPs and PCIMPs based on the Feret diameter analysis measurement. The diameter of each particle was calculated on one axis of the particle. We also measured the size of the particle directly based on its FE-SEM image. The FE-SEM image shows that Fe3O4 is a spherical shape, with an average size of ∼142 nm, as shown in Figure 5A. Moreover, APTMS coated on the surface of Fe3O4. An increase in particle size was observed, the average size being ∼175 nm, indicating the successful immobilization of APTMS on Fe3O4, (Figure 5B). Further, immobilization of GA on modified MPs resulted in a uniform shape and size of ∼228 nm (Figure 5C). Moreover, acrylate functionalization on modified MPs shows that the average size was ∼260 nm (Figure 5D). The elemental analysis from the Energy-dispersive X-ray Spectroscopy (EDS) image (Figure 5E) showed that Fe3O4@APTMS-GA-acrylate possessed the highest content of the C element over Fe, Si, O, and N species. Successful modification of acrylate on modified MPs was also observed. Among all PCIMPs, as shown in Figures 5F–H, the 15-mer fabricated PCIMPs65–79 were comparatively larger, around 375 nm. Other 14-mer fabricated PCIMPs65-78 and PCIMPs66-79 showed similar sizes (∼360 nm.).
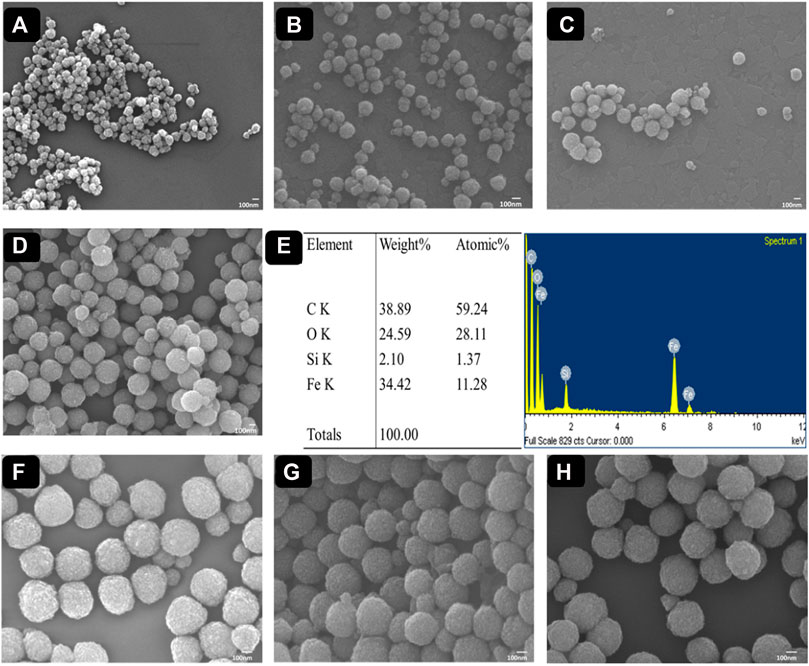
FIGURE 5. FE-SEM images of (A) Fe3O4, (B) Fe3O4@APTMS, (C) Fe3O4@APTMS-GA, and (D) Fe3O4@APTMS-GA-acrylate. The EDX spectrum image of (E) Fe3O4@APTMS-GA-acrylate. FE-SEM images of (F) PCIMPs65–79, (G) PCIMPs65–78, and (H) PCIMPs66–79.
Fabrication of PCIMPs to capture PAP
To further improve the PCIMPs’ fabrication, it was also necessary to create recognition sites that are complementary to protein recognition. The L-asparagine derivative (Metha-Asn-NHNH-Metha) was introduced as a cross-linker. The role of the cross-linker is to control the surface morphology of the polymer matrix and stabilize the imprinted binding sites, retaining their molecular recognition capability (Sellergren, 1999; Vasapollo et al., 2011). As shown in Supplementary Table S1, PCIMPs were fabricated with different concentrations of cross-linker and template. As the amount of the cross-linker and template increased, it helped to increase the binding affinity toward the mother protein. Meanwhile, adding a cross-linker formed more rigid PCIMPs and resulted in a more stable catalyst.
The PCIMPs-grafted 15- and 14-mer peptides were then tested for their ability to bind their mother protein, as described previously (Tai et al., 2012). As shown in Table 2, it was found that PCIMPs65–79 had more significant binding affinities, while the other two, PCIMPs65-78 and PCIMPs66-79, exhibited lower affinity. It was previously reported that the higher the number of residues in the template, the better binding affinity was observed (Tai et al., 2011; Kanubaddi et al., 2021). Accordingly, for PCIMP-grafted 15-mer peptides, the best Kd value was 0.087 μM, and it had a better affinity than the PCIMP-griated 14-mer peptides. Interestingly, among PCIMP-grafted 14-mer peptides, PCIMPs66-79 had the best Kd value (0.13 μM), whereas PCIMPs65-78 exhibited the lowest value (0.17 μM). Moreover, our developed PCIMPs generated binding sites that complemented protein recognition and consequently produced higher affinity toward targeted protein PAP. The elegant helical cavities stamping approach was compared with the other MIPs-grafted methods, based on the binding affinities and absorption time. Our results showed a higher affinity of PAP to PCIMPs65–79 than other observed MIPs-grafting approaches (Yang et al., 2016; Xu et al., 2018; Boitard et al., 2019).
The traditional synthetic method developed for 13-mer grafted PCIMPs66-78 was also compared with the fabricated PCIMPs with the cross-linker to detect PAP. In this preparation method, to improve the fabrication of PCIMPs66-78, we introduced two monomers, chiral histidine derivative (Acr-L-His-NHBn) and N-acryl tyramine. Interestingly, as displayed in Table 2, we observed that 13-mer-grafted PCIMPs66-78 led to higher affinity toward the analyte (Kd = 0.097 μM) when compared to fabricated 14-mer-grafted PCIMPs using the cross-linker (Metha-Asn-NHNH-Metha), i.e., PCIMPs65-78 (Kd = 0.17 μM) and PCIMPs66-79 (Kd = 0.13 μM). Moreover, the traditional formulated 13-mer-grafted PCIMPs had a better affinity toward PAP than the 14-mer-grafted PCIMPs using the cross-linker (Tai et al., 2011). Furthermore, monomers such as Acr-L-His-NHBn and N-acryl tyramine helped to strengthen affinity for PAP and helped harden the surface of the polymer matrix on PCIMPs,66-78 and reduced swelling while imprinting integrity (Yang et al., 2013). In addition, adding the cross-linker (EBBA) formed more rigid PCIMPs66-78 and produced a more stable catalytic activity (Tai et al., 2011). There was also a tendency for longer peptide residues to sustain a stable conformation throughout the polymerization process. For instance, 15 mer-grafted PCIMPs65–79 fabricated with Metha-Asn-NHNH-Metha showed a higher binding affinity toward PAP compared to the 13-mer and 14-mer PCIMPs. Therefore, fabricated PCIMPs65–79 using a cross-linker resulted in better protein binding and higher catalytic performance.
Evaluation of esterification activity of PAP and PAP/PCIMPs by using 1H-NMR
The maximum conversion of our PAP/PCIMPs was achieved in 24 hours (Figure 6A). Thus, the same duration (i.e., 24 hours) was chosen for the study of PAP/PCIMPs. The performance of the fabricated PAP/PCIMPs65–79 in catalyzing the esterification of N-protected amino acid was compared with the previous results. Accordingly, PAP/celite catalyzed esterification of N-protected amino acids in various organic solvents for 4 days. The desired product was obtained by silica gel chromatography, and purified esters were measured with 1H NMR. Their highest conversion was 71%, and reusability was not measured in this study (Shih et al., 1997). In another study, PAP was immobilized separately with eight kinds of adsorbents in a buffer solution. Among them, Sephadex G-50 was found to be the best adsorbent for immobilization of PAP. Accordingly, the PAP/Sephadex G-50 catalyzed esterification of N-substituted amino acids in MeOH for 2 days. Although this method shows a significant yield in esterification compared with other studies, PAP/Sephadex G-50 was difficult to reuse (Tai et al., 1989). Upon a comprehensive evaluation of N-protected amino acid esterification, the PCIMPs approach provided significant advantages over other methods. It was evident that the helical cavities created recognition sites on the MP surface and tightly bound the enzyme. As a result, it demonstrated better catalytic activity for esterification in comparison with previously conducted studies. The best catalytic performance of PAP/PCIMPs65–79 for the esterification of Boc-L-His-OH was 89% in 24 h.
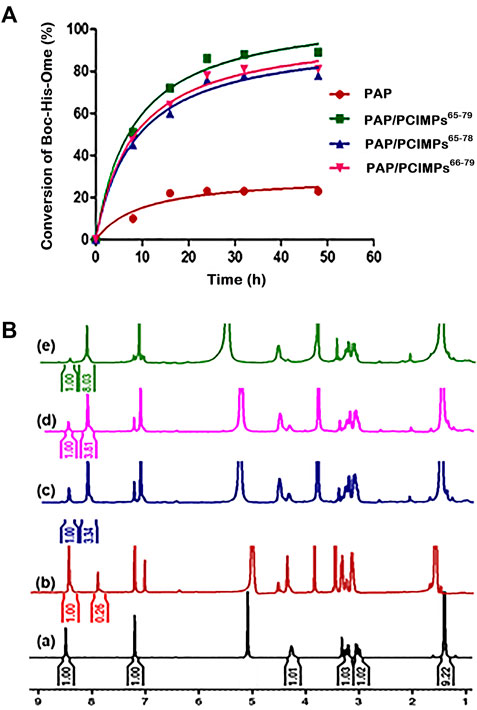
FIGURE 6. (A) Results of esterification of Boc-His-OH at 35°C at various intervals of reaction times. (B) 1H-NMR spectra of (a) the starting material (Boc-His-OH), and the reaction mixture of the starting material and ester formed by (b) PAP, (c) PAP/PCIMPs65–78, (d) PAP/PCIMPs66–79, and (e) PAP/PCIMPs65–79 (no of scans used = 32, solvent = CD3OD).
The data suggested a trend that initially crude PAP catalyzed hydrolysis of substrates rapidly. However, due to the amount of substrate decreasing, the rate of hydrolysis gradually decreased after 8 h; the reaction reached equilibrium after 48 h. The PAP reaction was found to produce the lowest final yield (20.6%), which can be attributed to certain protein instability features in organic solvents, thereby decreasing enzyme activity (Stepankova et al., 2013). As for PAP/PCIMPs, the highest yield (89%) was attained with PAP/PCIMPs65–79 in 24 h. The performances of PAP/PCIMPs66-79 and PAP/PCIMPs65-78 were lower, at 79.2% and ∼77%, respectively. This shows that PCIMPs’ imprinted materials are highly stable in organic solvents when compared to crude PAP, and enhance the yield of esterification for up to 48 h. In comparison, the yield catalyzed by traditional formulated PAP/PCIMPs66-78 was 84.5% (Supplementary Figure S3), higher than those of PAP/PCIMPs66-79 and PAP/PCIMPs65-78, but 4% lower than that of PAP/PCIMPs65–79.
To measure the reaction yield accurately, esterification yields were characterized using 1H-NMR. The spectra were acquired at a frequency of 300 MHz on a Bruker (from Billerica, Massachusetts, United States) and Ultrashield (9.4 T) spectrometer using a 5-mm BBo probe at 296.2 K. To calculate the conversion of Boc-L-His-OH to Boc-L-His-OMe, the ratio between the integral relevant to the hydrogen group of the imidazole ring of Boc-L-His-OH and corresponding to the hydrogen group of Boc-L-His-OMe was compared to calculate the yield of the reaction based on Equation 2. As shown in Figure 6B,a, the chemical displacement at 8.5 ppm was a hydrogen group of the imidazole of the original material (Boc-L-His-OH), while the proton value was equal to 1. The imidazole of the product (Boc-L-His-OMe) was similar, at 8 ppm. The finding of a peak at 3.8 ppm was representative of α proton.
Kinetic parameters of PAP and PAP/PCIMPs
The Michaelis–Menten equation was applied to determine the enzyme kinetic parameters for PAP catalyzed reaction. In the present study, kinetic parameters of PAP and PAP/PCIMPs were determined by changing the Boc-L-His-OH concentration from 0.06 to 0.1 M. It was then calculated using the Michaelis–Menten plot, as shown in Figure 7.
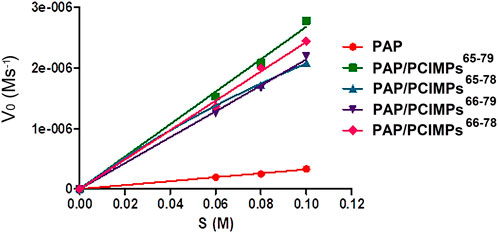
FIGURE 7. Michaelis–Menten plot of PAP and PAP/PCIMPs obtained with various concentrations of Boc-His-OH.
As shown in Table 3, the Vmax values were found to be 0.33 and 3.05 μM s−1 for PAP and PAP/PCIMPs65–79, while the performances of PAP/PCIMPs65-78 and PAP/PCIMPs66–79 were at 2.08 μM s−1 and 2.2 μM s−1, respectively. The Km of PAP was found to be the highest at 5.5 × 10−2 M. The lowest Km value was attained with PAP/PCIMPs65–79 as 5 × 10−2 M, whereas the Km value for PAP/PCIMPs65-78 and PAP/PCIMPs66–79 were 5.2 × 10−2 and 5.3 × 10−2 M, respectively. The Km value in PAP/PCIMPs was lower than that in the native enzyme. Consequently, PAP/PCIMPs have a greater affinity for the substrate than the PAP (Chen et al., 1998; Meridor and Gedanken, 2013). According to our calculation, PAP/PCIMPs possess the higher Vmax value and the lower Km value. Among all PAP/PCIMPs, PAP/PCIMPs65–79 have the best kinetic parameters to effectively promote the activity of PAP after immobilization (Sun et al., 2018).
As shown in Table 3, the turnover (kcat) value of PAP (7.8 × 10−4 s−1) was lower than those of PAP/PCIMPs65–79 (1.1 × 10−1 s−1), PAP/PCIMPs65-78 (6.8 × 10−2 s−1), and PAP/PCIMPs66–79 (7 × 10−2 s−1), which could be attributed to the higher catalytic efficiency of immobilized PAP than the free enzyme. The higher kcat value was possible due to the favorable interaction between the carrier (PCIMPs) and the enzyme (PAP), which addressed the PAP fold into the optimized conformation on the PCIMPs’ surface. Among all PAP/PCIMPs, the highest kcat value for PAP/PCIMPs65-79 was 1.1 × 10−1 s−1. ., and were 6.8 × 10−2, and 7 × 10−2 s−1 or PAP/PCIMPs65-78. and PAP/PCIMPs66-79, respectively. Similarly, the catalytic efficiency (kcat/Km) value of the PAP was 0.014 M−1 s−1, whereas it was 2 M−1 s−1 for PAP/PCIMPs65–79;PAP/PCIMPs65–78 and PAP/PCIMPs66-79 had similar values (∼1.3 M−1 s−1). The values of all PAP/PCIMPs were higher than that of the free PAP. This is probably because PAP/PCIMPs can maintain enzymatic activity and preserve favorable enzyme conformations (Wong et al., 2017; Yang L. et al., 2018; Li et al., 2019).
Moreover, we examined the kinetic parameters of traditionally formulated PAP/PCIMPs66-78 by increasing the Boc-L-His-OH concentration from 0.6, 0.8, and 0.1 M to 0.66, 0.88, and 0.11 M. As shown in Table 3, the Vmax value of PAP/PCIMPs66-78 was 2.4 μM s−1, which was higher than those of PAP/PCIMPs65-78 (2.08 μM s−1), and PAP/PCIMPs66-79 (2.2 μM s−1) fabricated with Metha-Asn-NHNH-Metha. Thus, evidence supporting higher substrate concentrations increased considerably, while reaction rate increased significantly, changing the kinetic parameters. Thus, we can conclude that it appears worthwhile to increase substrates for PAP/PCIMPs66–78.
Reusability
Another promising feature of PCIMPs is the rebinding of proteins and reusability. Once the PCIMPs are used for the application, the imprinted material used should be desorpted and reused. This will result in the sustainable repeated use of the material.
To demonstrate regeneration ability and reusability of imprinted materials of PCIMPs65–79, adsorption–desorption cycles were repeated four times using the same imprinted material, as shown in Figure 8. The partially dried imprinted materials of PCIMPs containing methanol were blow-dried in a fume hood, and the damp PCIMPs-imprinted material was used for subsequent runs. The resulting product was preserved at 0°C for future use.
The amount of Boc-L-His-OMe-produced yield in the first cycle was set as 100%. The yield of Boc-L-His-OMe slightly declined after the first reuse. However, the yield remained around 80% in the last two cycles, proving that imprinted polymer particles can be regenerated and reused. Additionally, PAP/PCIMPs exhibited stable catalytic activity in the reusability studies, compared with the PAP/Sephadex G-25 and PAP. PAP/Sephadex G-25 declined to a 20% yield after the fourth cycle, and PAP did not show any significant reusability. Long-term stability and reusability of PAP/PCIMPs were high compared to the PAP/Sephadex G-25 and PAP.
Conclusion
In summary, we succeeded in synthesizing a new chiral cross-linker, Metha-Asn-NHNH-Metha, from L-asparagine. Fabrication of the helical peptides using this cross-linker to form PCIMPs was accomplished by molecular imprinting technology. Among all the helical peptides used, 15-mer PCIMPs65–79 gained the highest binding affinity toward PAP and achieved the highest catalytic activity. Therefore, the fabrication of PCIMPs can provide an easy route to develop specific protein binding/adsorption and maintain enzyme catalytic activity.
Although trypsin flexibility interfered with the inhibitive effect on capturing the α-helix region during hydrolysis (Kanubaddi et al., 2021), PCIMPs were found to improve papain stability and robustness for esterification. This could be because the amount of water in esterification is much reduced in the reaction medium resulting in greater stability/activity of PAP/PCIMPs than PAP itself. The reuse displayed a small decrease in catalytic activity after four consecutive usages of the immobilized enzyme. Therefore, we conclude that our investigations will lead to more applications of these types of nanobiocatalysts in the future.
Data availability statement
The original contributions presented in the study are included in the article/Supplementary Material. Further inquiries can be directed to the corresponding authors.
Author contributions
KR, C-LY, and P-YH contributed to the execution of experiments, data analysis, and interpretation. KR, C-YL, D-FT, and C-HL contributed to the review and editing. C-YL, D-FT, and C-HL contributed to the study concept and design, data analysis and interpretation, securing of funding, and writing the manuscript. All authors have read and agreed to the published version of the draft of the manuscript.
Funding
This work is partially supported by the Taiwan Ministry of Science and Technology (MOST 106-2113-M-259-005 and 109-2221-E-182-008-MY3).
Conflict of interest
The authors declare that the research was conducted in the absence of any commercial or financial relationships that could be construed as a potential conflict of interest.
Publisher’s note
All claims expressed in this article are solely those of the authors and do not necessarily represent those of their affiliated organizations, or those of the publisher, the editors, and the reviewers. Any product that may be evaluated in this article, or claim that may be made by its manufacturer, is not guaranteed or endorsed by the publisher.
Supplementary material
The Supplementary Material for this article can be found online at: https://www.frontiersin.org/articles/10.3389/fbioe.2022.943751/full#supplementary-material
References
Anwar, M., Rasul, M. G., and Ashwath, N. (Editors) (2017). IEEE 7th International Conference on Power and Energy Systems (ICPES) - optimization of biodiesel production process from papaya (Carica papaya) seed oil. Toronto, Canada: IEEE. pp 131-134.
Basso, A., and Serban, S. (2019). Industrial applications of immobilized enzymes—a review. Mol. Catal. 479, 110607. doi:10.1016/j.mcat.2019.110607
Belyaeva, E. A., Gra, D. V., and Eremeev, N. L. (2002). On the mechanism of interaction of organic solvents with the active site of alpha-chymotrypsin. Biochemistry. 67 (9), 1032–1036. doi:10.1023/a:1020530220774
Bjelić, A., Hočevar, B., Grilc, M., Novak, U., and Likozar, B. (2022). A review of sustainable lignocellulose biorefining applying (natural) deep eutectic solvents (DESs) for separations, catalysis and enzymatic biotransformation processes. Rev. Chem. Eng. 38 (3), 243–272. doi:10.1515/revce-2019-0077
Boitard, C., Lamouri, A., Ménager, C., and Griffete, N. (2019). Whole protein imprinting over magnetic nanoparticles using photopolymerization. ACS Appl. Polym. Mat. 1 (5), 928–932. doi:10.1021/acsapm.9b00109
Bossi, A. M., Sharma, P. S., Montana, L., Zoccatelli, G., Laub, O., Levi, R., et al. (2012). Fingerprint-imprinted polymer: Rational selection of peptide epitope templates for the determination of proteins by molecularly imprinted polymers. Anal. Chem. 84 (9), 4036–4041. doi:10.1021/ac203422r
Cajnko, M. M., Novak, U., Grilc, M., and Likozar, B. (2020). Enzymatic conversion reactions of 5-hydroxymethylfurfural (HMF) to bio-based 2, 5-diformylfuran (DFF) and 2, 5-furandicarboxylic acid (FDCA) with air: Mechanisms, pathways and synthesis selectivity. Biotechnol. Biofuels 13, 66. doi:10.1186/s13068-020-01705-z
Cajnko, M. M., Oblak, J., Grilc, M., and Likozar, B. (2021). Enzymatic bioconversion process of lignin: Mechanisms, reactions and kinetics. Bioresour. Technol. 340, 125655. doi:10.1016/j.biortech.2021.125655
Cantacuzène, D., Pascal, F., and Guerreiro, C. (1987). Synthesis of amino acid esters by papain. Tetrahedron 43 (8), 1823–1826. doi:10.1016/s0040-4020(01)81493-9
Cao, S-L., Xu, H., Li, X-H., Lou, W-Y., and Zong, M-H. (2015). Papain@Magnetic nanocrystalline cellulose nanobiocatalyst: A highly efficient biocatalyst for dipeptide biosynthesis in deep eutectic solvents. ACS Sustain. Chem. Eng. 3 (7), 1589–1599. doi:10.1021/acssuschemeng.5b00290
Chen, J-P., Sun, Y-M., and Chu, D-H. (1998). Immobilization of alpha-amylase to a composite temperature-sensitive membrane for starch hydrolysis. Biotechnol. Prog. 14 (3), 473–478. doi:10.1021/bp9800384
Chou, C-Y., Lin, C-Y., Wu, C-H., and Tai, D-F. (2020). Sensing HIV protease and its inhibitor using "helical epitope"-imprinted polymers. Sensors (Basel) 20 (12), 3592. doi:10.3390/s20123592
Collins, J. M., Porter, K. A., Singh, S. K., and Vanier, G. S. (2014). High-efficiency solid phase peptide synthesis (HE-SPPS). Org. Lett. 16 (3), 940–943. doi:10.1021/ol4036825
de Beer, R. J., Zarzycka, B., Mariman, M., Amatdjais-Groenen, H. I., Mulders, M. J., Quaedflieg, P. J., et al. (2012). Papain-specific activating esters in aqueous dipeptide synthesis. ChemBioChem 13 (9), 1319–1326. doi:10.1002/cbic.201200017
Diltemiz, S. E., Hür, D., Ersöz, A., Denizli, A., and Say, R. (2009). Designing of MIP based QCM sensor having thymine recognition sites based on biomimicking DNA approach. Biosens. Bioelectron. X. 25 (3), 599–603. doi:10.1016/j.bios.2009.01.032
Ding, S., Xing, Y., Radosz, M., and Shen, Y. (2006). Magnetic nanoparticle supported catalyst for atom transfer radical polymerization. Macromolecules 39 (19), 6399–6405. doi:10.1021/ma061062y
Farjadian, F., Ghasemi, S., and Mohammadi-Samani, S. (2016). Hydroxyl-modified magnetite nanoparticles as novel carrier for delivery of methotrexate. Int. J. Pharm. X. 504 (1-2), 110–116. doi:10.1016/j.ijpharm.2016.03.022
Farjadian, F., Hosseini, M., Ghasemi, S., and Tamami, B. (2015). Phosphinite-functionalized silica and hexagonal mesoporous silica containing palladium nanoparticles in heck coupling reaction: Synthesis, characterization, and catalytic activity. RSC Adv. 5 (97), 79976–79987. doi:10.1039/c5ra16131b
Gao, Z., Yi, Y., Zhao, J., Xia, Y., Jiang, M., Cao, F., et al. (2018). Co-immobilization of laccase and TEMPO onto amino-functionalized magnetic Fe3O4 nanoparticles and its application in acid fuchsin decolorization. Bioresour. Bioprocess. 5 (1), 27. doi:10.1186/s40643-018-0215-7
Gerdon, A. E., Wright, D. W., and Cliffel, D. E. (2005). Quartz crystal microbalance detection of glutathione-protected nanoclusters using antibody recognition. Anal. Chem. 77 (1), 304–310. doi:10.1021/ac048953t
Hoarau, M., Badieyan, S., and Marsh, E. N. G. (2017). Immobilized enzymes: Understanding enzyme - surface interactions at the molecular level. Org. Biomol. Chem. 15 (45), 9539–9551. doi:10.1039/c7ob01880k
Homaei, A. (2015). Enhanced activity and stability of papain immobilized on CNBr-activated sepharose. Int. J. Biol. Macromol. 75, 373–377. doi:10.1016/j.ijbiomac.2015.01.055
Homaei, A. A., Sajedi, R. H., Sariri, R., Seyfzadeh, S., and Stevanato, R. (2010). Cysteine enhances activity and stability of immobilized papain. Amino Acids 38 (3), 937–942. doi:10.1007/s00726-009-0302-3
Homaei, A. A., Sariri, R., Vianello, F., and Stevanato, R. (2013). Enzyme immobilization: An update. J. Chem. Biol. 6 (4), 185–205. doi:10.1007/s12154-013-0102-9
Jeong, J., Lee, S-Y., and Hur, W. (2011). Composition variation of papain-catalyzed esterification of a fibroin peptide mixture. Biotechnol. Bioprocess Eng. 16 (4), 654–660. doi:10.1007/s12257-011-0076-9
Kankala, R. K., Zhang, H., Liu, C-G., Kanubaddi, K. R., Lee, C-H., Wang, S-B., et al. (2019). Metal species–encapsulated mesoporous silica nanoparticles: Current advancements and latest breakthroughs. Adv. Funct. Mat. 29 (43), 1902652. doi:10.1002/adfm.201902652
Kanubaddi, K. R., Huang, P-Y., Chang, Y-L., Wu, C-H., Li, W., Kankala, R. K., et al. (2021). Deviation of trypsin activity using peptide conformational imprints. Nanomater. (Basel) 11 (2), 334. doi:10.3390/nano11020334
Kuo, Y-M., Kuthati, Y., Kankala, R. K., Wei, P-R., Weng, C-F., Liu, C-L., et al. (2015). Layered double hydroxide nanoparticles to enhance organ-specific targeting and the anti-proliferative effect of cisplatin. J. Mat. Chem. B 3 (17), 3447–3458. doi:10.1039/c4tb01989j
Kurtan, U., and Baykal, A. (2014). Fabrication and characterization of Fe3O4@APTES@PAMAM-Ag highly active and recyclable magnetic nanocatalyst: Catalytic reduction of 4-nitrophenol. Mat. Res. Bull. 60, 79–87. doi:10.1016/j.materresbull.2014.08.016
Li, R., Yang, J., Xiao, Y., and Long, L. (2019). In vivo immobilization of an organophosphorus hydrolyzing enzyme on bacterial polyhydroxyalkanoate nano-granules. Microb. Cell Fact. 18 (1), 166. doi:10.1186/s12934-019-1201-2
Liang, X., Liu, Y., Wen, K., Jiang, W., and Li, Q. (2021). Immobilized enzymes in inorganic hybrid nanoflowers for biocatalytic and biosensing applications. J. Mat. Chem. B 9 (37), 7597–7607. doi:10.1039/d1tb01476e
Lin, C-Y., Tsai, S-H., and Tai, D-F. (2019). Detection of oxytocin, atrial natriuretic peptide, and brain natriuretic peptide using novel imprinted polymers produced with amphiphilic monomers. J. Pept. Sci. 25 (3), e3150. doi:10.1002/psc.3150
Llerena-Suster, C. R., José, C., Collins, S. E., Briand, L. E., and Morcelle, S. R. (2012). Investigation of the structure and proteolytic activity of papain in aqueous miscible organic media. Process Biochem. 47 (1), 47–56. doi:10.1016/j.procbio.2011.10.003
Marathe, S. J., Shah, N. N., and Singhal, R. S. (2020). Enzymatic synthesis of fatty acid esters of trehalose: Process optimization, characterization of the esters and evaluation of their bioactivities. Bioorg. Chem. 94, 103460. doi:10.1016/j.bioorg.2019.103460
Meridor, D., and Gedanken, A. (2013). Preparation of enzyme nanoparticles and studying the catalytic activity of the immobilized nanoparticles on polyethylene films. Ultrason. Sonochem. 20 (1), 425–431. doi:10.1016/j.ultsonch.2012.06.005
Mohamad, N. R., Marzuki, N. H. C., Buang, N. A., Huyop, F., and Wahab, R. A. (2015). An overview of technologies for immobilization of enzymes and surface analysis techniques for immobilized enzymes. Biotechnol. Biotechnol. Equip. 29 (2), 205–220. doi:10.1080/13102818.2015.1008192
Morcelle, S. R., Barberis, S., Priolo, N., Caffini, N. O., and Clapés, P. (2006). Comparative behaviour of proteinases from the latex of Carica papaya and Funastrum clausum as catalysts for the synthesis of Z-Ala-Phe-OMe. J. Mol. Catal. B Enzym. 41 (3), 117–124. doi:10.1016/j.molcatb.2006.05.007
Nguyen, H. H., Lee, S. H., Lee, U. J., Fermin, C. D., and Kim, M. (2019). Immobilized enzymes in biosensor applications. Mater. (Basel) 12 (1), 121. doi:10.3390/ma12010121
Prabhakar, S., Vivès, T., Ferrières, V., Benvegnu, T., Legentil, L., Lemiègre, L., et al. (2017). A fully enzymatic esterification/transesterification sequence for the preparation of symmetrical and unsymmetrical trehalose diacyl conjugates. Green Chem. 19 (4), 987–995. doi:10.1039/c6gc02680j
Secundo, F. (2013). Conformational changes of enzymes upon immobilisation. Chem. Soc. Rev. 42 (15), 6250. doi:10.1039/c3cs35495d
Sellergren, B. (1999). Polymer- and template-related factors influencing the efficiency in molecularly imprinted solid-phase extractions. TrAC Trends Anal. Chem. 18 (3), 164–174. doi:10.1016/s0165-9936(98)00117-4
Sheldon, R. A. (2011). Characteristic features and biotechnological applications of cross-linked enzyme aggregates (CLEAs). Appl. Microbiol. Biotechnol. 92 (3), 467–477. doi:10.1007/s00253-011-3554-2
Shih, I-L., Chiu, L-C., Lai, C-T., Liaw, W-C., and Tai, D-F. (1997). Enzymes catalyzed esterification of N-protected amino acids with secondary alcohols. Biotechnol. Lett. 19 (9), 857–859. doi:10.1023/a:1018329402408
Singh, R. K., Tiwari, M. K., Singh, R., and Lee, J. K. (2013). From protein engineering to immobilization: Promising strategies for the upgrade of industrial enzymes. Int. J. Mol. Sci. 14 (1), 1232–1277. doi:10.3390/ijms14011232
Stepankova, V., Bidmanova, S., Koudelakova, T., Prokop, Z., Chaloupkova, R., Damborsky, J., et al. (2013). Strategies for stabilization of enzymes in organic solvents. ACS Catal. 3 (12), 2823–2836. doi:10.1021/cs400684x
Stevenson, D. E., and Storer, A. C. (1991). Papain in organic solvents: Determination of conditions suitable for biocatalysis and the effect on substrate specificity and inhibition. Biotechnol. Bioeng. 37 (6), 519–527. doi:10.1002/bit.260370605
Sun, X., Zhu, W., and Matyjaszewski, K. (2018). Protection of opening lids: Very high catalytic activity of lipase immobilized on core-shell nanoparticles. Macromolecules 51 (2), 289–296. doi:10.1021/acs.macromol.7b02361
Tai, D-F., Fu, S-L., Chuang, S-F., and Tsai, H. (1989). Papain catalyzed esterification in polar organic solvents. Biotechnol. Lett. 11 (3), 173–176. doi:10.1007/bf01026051
Tai, D-F., Ho, Y-F., Wu, C-H., Lin, T-C., Lu, K-H., Lin, K-S., et al. (2011). Artificial-epitope mapping for CK-MB assay. Analyst 136 (11), 2230. doi:10.1039/c0an00919a
Tai, D-F., Lin, Y-F., Lu, K-H., Chen, G-Y., and Shu, H-C. (2012). A direct immersion system for peptide enrichment. J. Chin. Chem. Soc. 59 (3), 338–344. doi:10.1002/jccs.201100635
Tai, D-F. (2003). Enzymatic peptide synthesis: From the substrate point of view. Curr. Org. Chem. 7, 515–554. doi:10.2174/1385272033486819
Vasapollo, G., Sole, R. D., Mergola, L., Lazzoi, M. R., Scardino, A., Scorrano, S., et al. (2011). Molecularly imprinted polymers: Present and future prospective. Int. J. Mol. Sci. 12 (9), 5908–5945. doi:10.3390/ijms12095908
Wong, D-E., Senecal, K. J., and Goddard, J. M. (2017). Immobilization of chymotrypsin on hierarchical nylon 6, 6 nanofiber improves enzyme performance. Colloids Surfaces B Biointerfaces 154, 270–278. doi:10.1016/j.colsurfb.2017.03.033
Xu, J., Prost, E., Haupt, K., and Sum Bui, TseB. (2018). Direct and sensitive determination of trypsin in human urine using a water-soluble signaling fluorescent molecularly imprinted polymer nanoprobe. Sensors Actuators B Chem. 258, 10–17. doi:10.1016/j.snb.2017.11.077
Yang, C., Yan, X., Guo, H., and Fu, G. (2016). Synthesis of surface protein-imprinted nanoparticles endowed with reversible physical cross-links. Biosens. Bioelectron. X. 75, 129–135. doi:10.1016/j.bios.2015.08.033
Yang, H-H., Lu, K-H., Lin, Y-F., Tsai, S-H., Chakraborty, S., Zhai, W-J., et al. (2013). Depletion of albumin and immunoglobulin G from human serum using epitope-imprinted polymers as artificial antibodies. J. Biomed. Mat. Res. A 101 (7), 1935–1942. doi:10.1002/jbm.a.34491
Yang, L., Tian, J., Meng, J., Zhao, R., Li, C., Ma, J., et al. (2018). Modification and characterization of Fe3O4 nanoparticles for use in adsorption of alkaloids. Molecules 23 (3), 562. doi:10.3390/molecules23030562
Yang, Q., Zhu, Y., Luo, B., Lan, F., Wu, Y., Gu, Z., et al. (2017). pH-Responsive magnetic nanospheres for the reversibly selective capture and release of glycoproteins. J. Mat. Chem. B 5 (6), 1236–1245. doi:10.1039/c6tb02662a
Yang, Y., Wang, S., Zhou, Z., Zhang, R., Shen, H., Song, J., et al. (2018). Enhanced reusability and activity: DNA directed immobilization of enzyme on polydopamine modified magnetic nanoparticles. Biochem. Eng. J. 137, 108–115. doi:10.1016/j.bej.2018.05.019
Keywords: esterification, molecularly imprinted polymers, papain, peptide conformational imprints, enzyme immobilization
Citation: Kanubaddi KR, Yang C-L, Huang P-Y, Lin C-Y, Tai D-F and Lee C-H (2022) Peptide conformational imprints enhanced the catalytic activity of papain for esterification. Front. Bioeng. Biotechnol. 10:943751. doi: 10.3389/fbioe.2022.943751
Received: 14 May 2022; Accepted: 11 July 2022;
Published: 16 August 2022.
Edited by:
Lifeng Kang, The University of Sydney, AustraliaReviewed by:
Tushar Kumeria, University of New South Wales, AustraliaBlaž Likozar, National Institute of Chemistry, Slovenia
Kun Xue, Institute of Materials Research and Engineering (A∗STAR), Singapore
Copyright © 2022 Kanubaddi, Yang, Huang, Lin, Tai and Lee. This is an open-access article distributed under the terms of the Creative Commons Attribution License (CC BY). The use, distribution or reproduction in other forums is permitted, provided the original author(s) and the copyright owner(s) are credited and that the original publication in this journal is cited, in accordance with accepted academic practice. No use, distribution or reproduction is permitted which does not comply with these terms.
*Correspondence: Chung-Yin Lin, d2lud29vZDdAbWFpbC5jZ3UuZWR1LnR3; Dar-Fu Tai, ZGZ0YWlAZ21zLm5kaHUuZWR1LnR3