Biosynthetic approaches to efficient assimilation of CO2 via photorespiration modification in plant chassis
- 1Key Laboratory of Systems Microbial Biotechnology, Tianjin Institute of Industrial Biotechnology, Chinese Academy of Sciences, Tianjin, China
- 2National Center of Technology Innovation for Synthetic Biology, Tianjin, China
- 3College of Biotechnology, Tianjin University of Science and Technology, Tianjin, China
- 4China Tobacco Gene Research Center, Zhengzhou Tobacco Research Institute of CNTC, Zhengzhou, China
Plant chassis has emerged as the platform with great potential for bioproduction of high value-added products such as recombinant protein, vaccine and natural product. However, as the primary metabolic pathway, photorespiration results in the loss of photosynthetically fixed carbon compounds and limits the exploration of plant chassis. People are endeavored to reduce the photorespiration energy or carbon loss based on variation screening or genetic engineering. Insomuch as protein engineering of Rubisco has not resulted in the significant improvement of Rubisco specificity which is linked to the direct CO2 fixation, the biosynthetic approaches of photorespiration bypass are gaining much more attention and manifested great potentiality in conferring efficient assimilation of CO2 in plant chassis. In this review, we summarize the recent studies on the metabolic pathway design and implementation of photorespiration alternative pathway aiming to provide clues to efficiently enhance carbon fixation via the modification of photorespiration in plant chassis for bioproduction. These will benefit the development of plant synthetic metabolism for biorefineries via improvement of artificial carbon sequestration cycle, particularly for the mitigation of serious challenges such as extreme climate change, food and energy shortages in the future.
Introduction
As the global human population rapidly increases, new and efficient biological systems are urgent to be obtained to meet the growing demand for resources (Rai et al., 2019). Synthetic biology has been established to be one of the powerful platforms that focuses on the design of novel synthetic biological pathways or redesign of existing natural systems which could fulfill the requirement mentioned above (Holland and Jez, 2018). Although microbial chassises are widely used in present industrial bioproduction, their improvement is greatly challenged due to the lack of post-translational modifications, compartmentalization, non-functional nature and negligible activity of some proteins (Tiwari et al., 2021). Plant chassis, gradually emerging as the platform with great potential for bioproduction of high value-added products through manipulation of synthetic biology, is becoming the ideal and sustainable platform for their ability to directly use sunlight and CO2 to generate a variety of organic compounds (Fesenko and Edwards, 2014). Thus, plant synthetic biology is expected to present great potentiality in leading the development of molecular farming to benefit the production of food, fuels, fodder, therapeutics and environmental welfare to create totally synthetic life forms or components (Rai et al., 2019; Fausther-Bovendo and Kobinger, 2021; Cournoyer et al., 2022).
In optimizing the plant chassis which is suitable for the bioproduction of value-added metabolites, people are mostly focusing on the modification of carbon flux in C3 plants such as tobacco, rice and tomato. Ribulose-1,5-bisphosphate carboxylase oxygenase (Rubisco) is the first and important enzyme in C3 pathway to fix atmospheric CO2 (Sage et al., 2012). Both 3-PGA and 2-PG are generated due to the enzymatic activity of Rubisco (Lorimer, 1981; Betti et al., 2016). 2-PG, which could not be directly used for carbon fixation, inhibits the activity of chloroplastic enzymes (Anderson, 1971; Norman and Colman, 1991). To degrade 2-PG, a photorespiration pathway was developed to recycle 2-PG into 3-PGA that re-enter the Calvin-Benson cycle during evolution, through a serial of catalysis in chloroplast, peroxisome, mitochondrion and cytosol (Bauwe et al., 2010). During the process, two molecules of 2-PG are converted into one molecule of 3-PGA and one carbon atom is lost as CO2 in the mitochondria (Peterhansel et al., 2010), resulting in 25% of carbon loss (Walker et al., 2016). Furthermore, the photorespiratory effects could be enhanced by serious conditions such as high temperature and water shortage (Sharkey, 1988; Walker et al., 2016). Intensive studies are tentatively performed to decrease the carbon loss in photorespiration by genetic manipulation of Rubisco aiming to improve its selectivity and kinetic properties but without great effects (Bathellier et al., 2018; South et al., 2018). By contrast, the design of novel photorespiratory bypass by biosynthetic approaches has brought attention and is thought to play a major role in reducing carbon release of native photorespiration. To date, several novel photorespiratory bypasses have been implemented into plants and remarkably developed (Kebeish et al., 2007; Carvalho et al., 2011; South et al., 2019; Shen et al., 2019; Roell et al., 2021). The present review summarizes the novel biosynthetic pathways of reducing carbon release via the design of photorespiratory bypasses, and analyzes their potential effects. Then future perspective is suggested aiming to provide people with enlightenment to make progressive development in this field.
Biosynthetic photorespiratory bypasses implemented into plants
1. Among the reported photorespiratory bypass pathways implemented into plants, E. coli-originated glyoxylate oxidation catalysis has been extensively tested (Kebeish et al., 2007; Dalal et al., 2015; South et al., 2019; Chen et al., 2019; Wang et al., 2019; Nayak et al., 2022; Zhang et al., 2022). Glycolate is converted into glyoxylate by glycolate dehydrogenase (GDH) or glycolate oxidase (GLO), followed by the generation of tartronic semialdehyde and CO2 from two molecules of glyoxylate catalyzed by glyoxylate carboligase (GCL). Tartronic semialdehyde is then converted to glycerate by tartronic semialdehyde reductase (TSR) in the chloroplast, and all the catalytic steps are established in the chloroplast (Figure 1, fonts marked by red and orange color). H2O2, as the by-product of GLO-mediated catalysis, is decomposed via the introduction of catalase (CAT) (Wang et al., 2019).
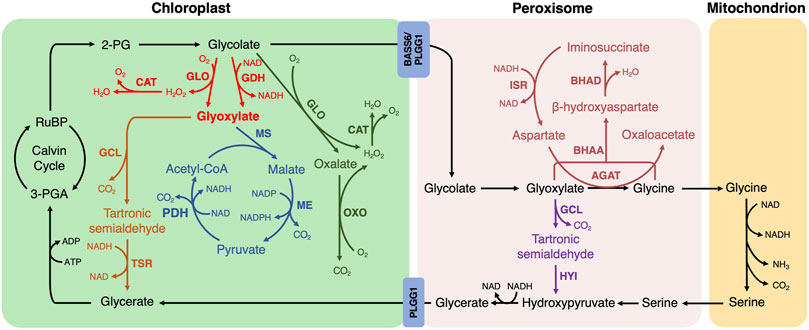
FIGURE 1. The photorespiratory bypasses implemented into plants. The natural photorespiratory pathway and biosynthetic bypasses to photorespiration are marked by defined color. BASS, Bile acid sodium symporter; PLGG, glycolate-glycerate transporter. The first bypass in the chloroplast (red and orange; Kebeish et al., 2007; Dalal et al., 2015; South et al., 2019; Chen et al., 2019; Wang et al., 2020; Nayak et al., 2022; Zhang et al., 2022): GLO, glycolate oxidase; CAT, catalase; GDH, glycolate dehydrogenase; GCL, glyoxylate carboligase; TSR, tartronic semialdehyde reductase. The second bypass in the chloroplast (red and blue; Maier et al., 2012; South et al., 2019; Cavanagh et al., 2022): MS, malate synthase; ME, malic enzyme; PDH, pyruvate dehydrogenase. The third bypass in the chloroplast (green; Shen et al., 2019): OXO, oxalate oxidase. The first bypass in the peroxisome (purple; Carvalho et al., 2011): HYI, hydroxypyruvate isomerase. The second bypass in the peroxisome (dark red; Roell et al., 2021): AGAT, aspartate:glyoxylate aminotransferase; BHAA, β-hydroxyaspartate aldolase; BHAD, β-hydroxyaspartate dehydratase; ISR, iminosuccinate reductase.
This bypass manifested several advantages compared to the natural photorespiration pathway. Firstly, CO2 is shifted and released into the chloroplast, where it could be re-cycled into Calvin-Bension metabolism. Secondly, the NH3 production is greatly avoided. Thirdly, the metabolite transport between organelles is bypassed, whereas between 14 and 18 transport processes are required in natural photorespiration (Reumann and Weber, 2006). Therefore, it saves more energy than the native photorespiratory. Expectedly, the reduced photorespiration, improved photosynthetic performance and enhanced biomass production were observed in Arabidopsis, camelina and cucumber (Kebeish et al., 2007; Dalal et al., 2015; Chen et al., 2019). In detail, CO2 compensation point was significantly decreased in transgenic Arabidopsis with a reduction of more than 10% (Kebeish et al., 2007). Transgenic camelina showed 14–28% increase in CO2 fixation (Dalal et al., 2015). The same effects on biomass and grain yield were obtained when it was introduced into rice (Wang et al., 2019; Nayak et al., 2022), however, the seeding rate is decreased and chalky rice rate is increased, which could be explained by the undelivered photosynthetic carbohydrates into grains in a timely or efficient way during the filling stage (Wang et al., 2019; Zhang et al., 2022).
2. In the second bypass, a cycle to completely decarboxylate glycolate is introduced into the chloroplast (Maier et al., 2012; South et al., 2019). Glycolate is oxidized to glyoxylate by GDH or GLO, which is the same as E. coli glyoxylate oxidation bypass. Glyoxylate and acetyl-CoA are then converted to malate using malate synthase (MS), and the resulting malate is decarboxylated into pyruvate by the malic enzyme (ME) with the first CO2 release. Pyruvate is oxidized into acetyl-CoA by pyruvate dehydrogenase (PDH) with the second CO2 release. The acetyl-CoA re-enters into the bypass by combining with glyoxylate (Figure 1, fonts marked by red and blue color).
This bypass shifts CO2 release to the chloroplast with no NH3 production as in the first bypass, but impedes the Calvin-Benson cycle due to no 3-PGA recovery (Peterhansel et al., 2013). It has already been successfully implemented into Arabidopsis and tobacco (Maier et al., 2012; South et al., 2019). The Arabidopsis transgenic lines exhibited various phenotypes of leaf color and oxidative lesions possibly due to their variation of CAT activity in chloroplasts, which remains to be explained (Maier et al., 2012). CO2 compensation point was not statistically different between genotypes in Arabidopsis (Maier et al., 2012). Consistent with the results of Arabidopsis, 24% of the transgenic tobacco with GLO and CAT presented stunted growth and yellow leaves. However, the transgenic tobacco, in which GDH is used instead of GLO to remove the need for CAT, showed a biomass increase of 18% and 24% and a CO2 compensation point decrease of 6.4% and 10% under wild type and PLGG1 RNAi background when compared with control, respectively (South et al., 2019). Meanwhile, the transgenic tobacco line with PLGG1 RNAi module sustained 19% less yield loss compared to wild type under high temperature conditions (Cavanagh et al., 2022). These results suggest that the introduction of GDH into plants without producing H2O2 is a valuable strategy to improve the agronomic trait or phenotype.
3. In the third bypass, glycolate is converted to CO2 completely by endogenous enzymes in the chloroplast (Shen et al., 2019). Glycolate is oxidized to oxalate and H2O2 by GLO in two steps, and oxalate is then oxidized to CO2 and H2O2 by oxalate oxidase (OXO). The by-product H2O2 is also scavenged by CAT (Figure 1, fonts marked by green color). The chloroplastic CO2 concentration is enhanced and NH3 release is bypassed in this design. Nevertheless, no additional reducing equivalent is generated and more ATP units are required (Shen et al., 2019). When the bypass is introduced into rice, CO2 compensation point is decreased and biomass production is enhanced but grain yield varied by sowing seasons and setting rate was decreased (Shen et al., 2019). In addition, the decreased head milled rice rate and increased chalky rice rate were observed in the transgenic rice lines, indicating that milling quality and appearance quality was reduced to some extent (Zhang et al., 2022). Furthermore, this bypass and the first bypass using the rice-self originating CAT in transgenic rice did not show oxidative damage compared to the second bypass using E. coli sourced CAT in transgenic Arabidopsis and tobacco (Maier et al., 2012; Shen et al., 2019; South et al., 2019; Wang et al., 2019), suggesting that endogenous enzyme may more efficient to scavenge H2O2.
4. The first bypass located in the peroxisome is a simplified version of E. coli glyoxylate oxidation catalysis (Carvalho et al., 2011). The decarboxylation reaction of glyoxylate is catalyzed by the same enzyme GCL. Tartronic semialdehyde is then converted to hydroxypyruvate fed back into photorespiration by hydroxypyruvate isomerase (HYI) (Figure 1, fonts marked by purple color). NH3 production is avoided but only three-quarters of carbon is converted to 3-PGA (Eisenhut et al., 2008). Since HYI protein was not detected in transgenic tobacco, this bypass has been partially established in tobacco (Carvalho et al., 2011). Leaves of transgenic tobacco exhibited growth retardation and lesions after exposure to ambient air but not at an increased concentration of CO2, indicating the presence of a metabolic defect associated with photorespiration nitrogen cycle. These results suggest that the metabolic flux through glyoxylate to tartronic semialdehyde directly or indirectly caused deleterious effects on plants and the impact of this bypass remains to be proven (Carvalho et al., 2011).
5. Another bypass located in the peroxisome is a β-hydroxyaspartate cycle also starts from glyoxylate (Schada von Borzyskowski et al., 2019). Glyoxylate and aspartate are first converted into glycine and oxaloacetate by aspartate:glyoxylate aminotransferase (AGAT). Then the resulting glycine and glyoxylate are condensed into β-hydroxyaspartate using β-hydroxyaspartate aldolase (BHAA). β-hydroxyaspartate is converted to iminosuccinate in the presence of β-hydroxyaspartate dehydratase (BHAD). Iminosuccinate is reduced to aspartate by iminosuccinate reductase (ISR) to regenerate the amino group donor was regenerated for the first step of this bypass (Figure 1, fonts marked by dark red color). Oxaloacetate, generated in this bypass, could directly enter the tricarboxylic acid cycle or be used as the substrate for anabolic reactions without carbon and nitrogen loss (Schada von Borzyskowski et al., 2019; Roell et al., 2021). Roell et al. (2021) further investigated this bypass by using Arabidopsis ggt1-1 mutant in which glutamate glyoxylate aminotransferase 1 is down-regulated, thereby directing metabolite flux toward this biosynthetic bypass. The transgenic plants under mutant background were increased in growth but did not significantly affect the CO2 compensation point compared with ggt1-1 mutant in ambient air (Roell et al., 2021). However, due to the multiple effects of oxaloacetate metabolism such as tricarboxylic acid cycle and amino acid biosynthesis, the full potential of this bypass may be masked (Roell et al., 2021).
Potentially achieved photorespiratory bypasses in plants
In addition to the implemented bypasses described above, there are some promising alternative bypasses that could be experimentally tested in plants. Such as similar to the third bypass in the chloroplast, glycolate is converted to two molecules of CO2 completely (Eisenhut et al., 2008; Claassens et al., 2020; Khurshid et al., 2020). In these bypasses, glycolate is first converted to formate with one molecule of CO2 release by three or four enzymes and then formate oxidizes to CO2 by formate dehydrogenase (FDH) (Eisenhut et al., 2008; Claassens et al., 2020; Khurshid et al., 2020) (Figure 2, fonts marked by orange color). In addition, it is also possible to design new bypasses. For example, glyoxylate can spontaneously convert to formate and CO2 in the presence of H2O2 (Wingler et al., 1999). Therefore, GLO and FDH could be combined with the above non-enzymatic reaction together to completely decarboxylate glycolate into two molecules of CO2.
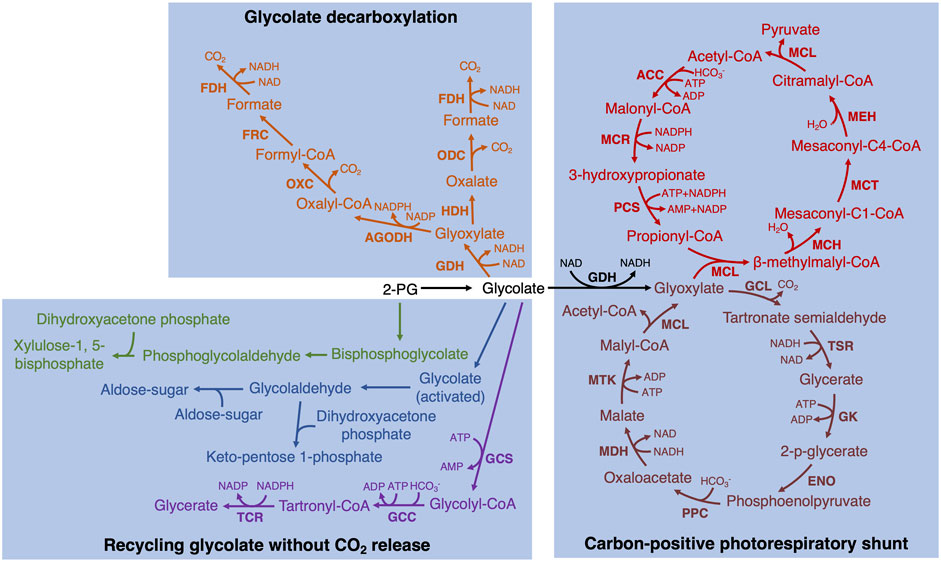
FIGURE 2. The potentially achieved biosynthetic bypasses of photorespiration in plants. Two glycolate decarboxylation bypasses are marked by orange (Eisenhut et al., 2008; Claassens et al., 2020; Khurshid et al., 2020). GDH, glycolate dehydrogenase; HDH, hydroxyacid dehydrogenase; ODC, oxalate decarboxylase; FDH, formate dehydrogenase; AGODH, CoA-acylating glyoxylate dehydrogenase; OXC, oxalyl-CoA decarboxylase; FRC, formyl-CoA transferase. Two different hypothetical bypasses to recycle glycolate without CO2 release are marked by green (Ort et al., 2015) and blue (Bar-Even, 2018), respectively. Tartronyl-CoA pathway (purple; Scheffen et al., 2021): GCS, glycolyl-CoA synthetase; GCC, glycolyl-CoA carboxylase; TCR, tartronyl-CoA reductase. The bypass converting glycolate to pyruvate (black and red; Shih et al., 2014): MCL, malyl-CoA lyase; MCH, mesaconyl-C1-CoA hydratase; MCT, mesaconyl-CoA C1:C4 CoA transferase; MEH, mesaconyl-C4-CoA hydratase; ACC, acetyl-CoA carboxylase; MCR, malonyl-CoA reductase; PCS, propionyl-CoA synthase. The bypass converting glycolate to acetyl-CoA (black and dark red; Yu et al., 2018): GCL, glyoxylate carboligase; TSR, tartronic semialdehyde reductase; GK, glycerate kinase; ENO, enolase; PPC, phosphoenolpyruvate carboxylase; MDH, malate dehydrogenase; MTK, malate thiokinase.
Furthermore, the carbon release is always detected in photorespiration and alternative pathways need to be tested in plants that could recycle glycolate without CO2 release. One hypothetical bypass is to reduce 2-PG to phosphoglycolaldehyde, which is then combined with dihydroxyacetone phosphate to produce xylulose bisphosphate. Then xylulose bisphosphate can be dephosphorylated to xylulose-5-phosphate back into Calvin-Benson cycle (Ort et al., 2015) (Figure 2, fonts marked by green color). Another hypothetical carbon re-cycle route is to reduce glycolate to glycolaldehyde via a glycolyl-phosphate or glycolyl-CoA intermediate, and then glycolaldehyde is as an acceptor or donor by an aldol reaction into the Calvin-Benson cycle (Bar-Even, 2018) (Figure 2, fonts marked by blue color). In addition, Trudeau et al. (2018) utilized the natural and artificially designed enzymes to identify some synthetic carbon-conserving bypasses and provide principles of the alternative biosynthesis. Recently, by applying rational design and high-throughput evolution of enzymes, one of the bypasses, tartronyl-CoA pathway, has been successfully reconstituted and implemented in vitro (Scheffen et al., 2021) (Figure 2, fonts marked by purple color). This bypass could directly convert glycolate to glycerate by only three enzymes and fix an additional carbon, increasing the carbon efficiency from 75% to 150%. The other approach is to engineer bypass involving intermediates not present in plants or design a single enzyme to convert glycolate to glycerate directly in the chloroplast.
A carbon-positive photorespiratory shunt for converting downstream products was suggested as a strategy beyond zero-carbon release bypasses. A promising alternative pathway that needs to be tested in plants is to convert glycolate to pyruvate, which requires introducing seven enzymes and fixing one HCO3− (Shih et al., 2014). This bypass prevented the loss of NH4+ while increasing the carbon fixation rate (Figure 2, fonts marked by black and red color). In addition, Yu et al. (2018) designed a synthetic malyl-CoA-glycerate pathway to assimilate glycolate to produce acetyl-CoA by releasing one molecule of CO2 and fixing one molecule of HCO3− to achieve no carbon loss (Figure 2, fonts marked by black and dark red color).
Perspective on the modification of photorespiration by synthetic biology
The reported bypasses may be limited by contingencies of evolutionary change and natural selection due to the attempts to reduce carbon release are inferred from biochemistry and theory (Peterhansel et al., 2013b). The substrate affinity, optimal pH and sensitivity of inhibitors need to be adjusted to support high fluxes in novel pathways considering that the introduced enzymes are not active enough when implemented into plant cells (Rademacher et al., 2002; Peterhansel et al., 2013b). In addition, since most of the bypasses are established in vitro, it is necessary to clone entire biosynthetic bypasses gradually into a single construct for transformation of a single plant to better assess these alternative approaches. Multiple designs need to be tested for combinations of promoter genes to optimize gene expression (South et al., 2018). Furthermore, it is possible to turn off the native photorespiratory pathway by using mutants or gene knockout approaches to maximize flux for testing alternative pathways (South et al., 2019; Roell et al., 2021).
Since most of the bypasses are targeted to the chloroplast, it is necessary to have efficient and precise chloroplast transit peptides (CTP) to target enzymes into the chloroplast. CTP recognition is governed by sequence-independent interactions and vectorial-specific recognition domains by a series of in vitro and in vivo experiments (Chotewutmontri et al., 2012). Shen et al. (2017) revealed that a 21 amino acid unfolded region in the N-terminus of CTP is important to efficiently import proteins into the chloroplast. In addition, Wimmer et al. (2017) found the specific but not conserved sequence elements of CTP could target proteins to the peripheral chloroplasts rather than central chloroplasts in a single-cell C4 plant. These results suggest that the optimization of CTP sequence is crucial to effectively target diverse enzymes into the chloroplasts.
The availability of both reducing equivalent and ATP is crucial to bioproduction. In plants, ATP and NADPH are generated in the process of photosynthesis in the chloroplast (Voon and Lim, 2019), and NADH is mainly generated in the mitochondria by the tricarboxylic acid cycle (Maynard and Kanarek, 2020). However, since most dehydrogenases function with NADH as a cofactor, the sufficient supply of NADH is essential for the production of dehydrogenase-derived chemicals (Meng et al., 2021). Therefore, ferredoxin-NAD+ reductase could be introduced into the chloroplast to generate NADH for increasing reducing equivalents. More photorespiratory bypasses that depend on NADH can also be achieved in the chloroplast in the future.
The pathway targeted to the mitochondrion has not been evaluated for the establishment of photorespiratory bypasses. Glycine and serine from photorespiration could serve as the substrates for bypass design in the mitochondrion. Glycine is important for synthesis of collagen, elastin and other protein in mammals (Meléndez-Hevia and de Paz-Lugo, 2008). Perhaps the new bypass introduction might convert glycine to collagen to reduce carbon release and increase target product. Serine could be converted to pyruvate (Yu and Liao, 2018) or cysteine (Busch, 2020) to participate in the tricarboxylic acid cycle or sulfate assimilation, respectively. However, the bypasses using serine as a substrate could only reduce the downstream reactions of photorespiration, but cannot reduce the CO2 and NH3 release.
In conclusion, novel and technological solutions must be obtained to further increase the productivity of crops because traditional genetic engineering may reach a plateau (Batista-Silva et al., 2020). Synthetic biology is opening up a new opportunity, that is, possible to conceptualize, design, build and test multiple approaches to redesign photorespiration to improve plant growth and yield (South et al., 2018). However, more fundamental researches and more advanced biosynthetic approaches are needed to effectively reduce carbon release in plant chassis. It is necessary to assess the pathways not only in model plants but also in food crops under a range of relevant agricultural environments to benefit the development of plant synthetic metabolism.
Author contributions
QW and LZ drafted the manuscript. QW, HY, PC, FC, and LZ reviewed and edited the manuscript. All the authors read and approved the final manuscript.
Funding
This work was supported by grants from Science and Technology Partnership Program, Ministry of Science and Technology of China (KY202001017), Tianjin Synthetic Biotechnology Innovation Capacity Improvement Project (TSBICIP-CXRC-027; TSBICIP-IJCP-001), TIB-VIB Joint Center of Synthetic Biology (TSBICIP-IJCP-002). PC is supported by the Science and Technology Project of CNTC (110202101008, JY-08).
Conflict of interest
The authors declare that the research was conducted in the absence of any commercial or financial relationships that could be construed as a potential conflict of interest.
Publisher’s note
All claims expressed in this article are solely those of the authors and do not necessarily represent those of their affiliated organizations, or those of the publisher, the editors and the reviewers. Any product that may be evaluated in this article, or claim that may be made by its manufacturer, is not guaranteed or endorsed by the publisher.
References
Anderson, L. E. (1971). Chloroplast and cytoplasmic enzymes. II. Pea leaf triose phosphate isomerases. Biochimica Biophysica Acta - Enzym. 235, 237–244. doi:10.1016/0005-2744(71)90051-9
Bar-Even, A. (2018). Daring metabolic designs for enhanced plant carbon fixation. Plant Sci. 273, 71–83. doi:10.1016/j.plantsci.2017.12.007
Bathellier, C., Tcherkez, G., Lorimer, G. H., and Farquhar, G. D. (2018). Rubisco is not really so bad. Plant Cell Environ. 41, 705–716. doi:10.1111/pce.13149
Batista-Silva, W., da Fonseca-Pereira, P., Martins, A. O., Zsögön, A., Nunes-Nesi, A., Araújo, W. L., et al. (2020). Engineering improved photosynthesis in the era of synthetic biology. Plant Commun. 1, 100032. doi:10.1016/j.xplc.2020.100032
Bauwe, H., Hagemann, M., and Fernie, A. R. (2010). Photorespiration: Players, partners and origin. Trends Plant Sci. 15, 330–336. doi:10.1016/j.tplants.2010.03.006
Betti, M., Bauwe, H., Busch, F. A., Fernie, A. R., Keech, O., Levey, M., et al. (2016). Manipulating photorespiration to increase plant productivity: Recent advances and perspectives for crop improvement. J. Exp. Bot. 67, 2977–2988. doi:10.1093/jxb/erw076
Busch, F. A. (2020). Photorespiration in the context of Rubisco biochemistry, CO2 diffusion and metabolism. Plant J. 101, 919–939. doi:10.1111/tpj.14674
Carvalho, J. F. C., Madgwick, P. J., Powers, S. J., Keys, A. J., Lea, P. J., Parry, M. A., et al. (2011). An engineered pathway for glyoxylate metabolism in tobacco plants aimed to avoid the release of ammonia in photorespiration. BMC Biotechnol. 11, 111. doi:10.1186/1472-6750-11-111
Cavanagh, A. P., South, P. F., Bernacchi, C. J., and Ort, D. R. (2022). Alternative pathway to photorespiration protects growth and productivity at elevated temperatures in a model crop. Plant Biotechnol. J. 20, 711–721. doi:10.1111/pbi.13750
Chen, Z. F., Kang, X. P., Nie, H. M., Zheng, S. W., Zhang, T. L., Zhou, D., et al. (2019). Introduction of exogenous glycolate catabolic pathway can strongly enhances photosynthesis and biomass yield of cucumber grown in a low-CO2 environment. Front. Plant Sci. 10, 702. doi:10.3389/fpls.2019.00702
Chotewutmontri, P., Reddick, L. E., McWilliams, D. R., Campbell, I. M., and Bruce, B. D. (2012). Differential transit peptide recognition during preprotein binding and translocation into flowering plant plastids. Plant Cell 24, 3040–3059. doi:10.1105/tpc.112.098327
Claassens, N. J., Scarinci, G., Fischer, A., Flamholz, A. I., Newell, W., Frielingsdorf, S., et al. (2020). Phosphoglycolate salvage in a chemolithoautotroph using the calvin cycle. Proc. Natl. Acad. Sci. U. S. A. 117, 22452–22461. doi:10.1073/pnas.2012288117
Cournoyer, J. E., Altman, S. D., Gao, Y., Wallace, C. L., Zhang, D., Lo, G. H., et al. (2022). Engineering artificial photosynthetic life-forms through endosymbiosis. Nat. Commun. 23, 2254. doi:10.1038/s41467-022-29961-7
Dalal, J., Lopez, H., Vasani, N. B., Hu, Z., Swift, J. E., Yalamanchili, R., et al. (2015). A photorespiratory bypass increases plant growth and seed yield in biofuel crop camelina sativa. Biotechnol. Biofuels 8, 175. doi:10.1186/s13068-015-0357-1
Eisenhut, M., Ruth, W., Haimovich, M., Bauwe, H., Kaplan, A., Hagemann, M., et al. (2008). The photorespiratory glycolate metabolism is essential for cyanobacteria and might have been conveyed endosymbiontically to plants. Proc. Natl. Acad. Sci. U. S. A. 105, 17199–17204. doi:10.1073/pnas.0807043105
Fausther-Bovendo, H., and Kobinger, G. (2021). Plant-made vaccines and therapeutics. Science 373, 740–741. doi:10.1126/science.abf5375
Fesenko, E., and Edwards, R. (2014). Plant synthetic biology: a new platform for industrial biotechnology. J. Exp. Bot. 65, 1927–1937. doi:10.1093/jxb/eru070
Holland, C. K., and Jez, J. M. (2018). Arabidopsis: the original plant chassis organism. Plant Cell Rep. 37, 1359–1366. doi:10.1007/s00299-018-2286-5
Kebeish, R., Niessen, M., Thiruveedhi, K., Bari, R., Hirsch, H. J., Rosenkranz, R., et al. (2007). Chloroplastic photorespiratory bypass increases photosynthesis and biomass production in Arabidopsis thaliana. Nat. Biotechnol. 25, 593–599. doi:10.1038/nbt1299
Khurshid, G., Abbassi, A. Z., Khalid, M. F., Gondal, M. N., Naqvi, T. A., Shah, M. M., et al. (2020). A cyanobacterial photorespiratory bypass model to enhance photosynthesis by rerouting photorespiratory pathway in C3 plants. Sci. Rep. 10, 20879. doi:10.1038/s41598-020-77894-2
Lorimer, G. H. (1981). The carboxylation and oxygenation of ribulose 1, 5-bisphosphate: the primary events in photosynthesis and photorespiration. Annu. Rev. Plant Physiol. 32, 349–382. doi:10.1146/annurev.pp.32.060181.002025
Maier, A., Fahnenstich, H., von Caemmerer, S., Engqvist, M. K. M., Weber, A. P. M., Flügge, U. I., et al. (2012). Transgenic introduction of a glycolate oxidative cycle into a. thaliana chloroplasts leads to growth improvement. Front. Plant Sci. 3, 38. doi:10.3389/fpls.2012.00038
Maynard, A. G., and Kanarek, N. (2020). NADH ties one-carbon metabolism to cellular respiration. Cell Metab. 31, 660–662. doi:10.1016/j.cmet.2020.03.012
Meléndez-Hevia, E., and de Paz-Lugo, P. (2008). Branch-point stoichiometry can generate weak links in metabolism: the case of glycine biosynthesis. J. Biosci. 33, 771–780. doi:10.1007/s12038-008-0097-5
Meng, H., Zhang, W., Zhu, H., Yang, F., Zhang, Y., Zhou, J., et al. (2021). Over-expression of an electron transport protein OmcS provides sufficient NADH for D-lactate production in cyanobacterium. Biotechnol. Biofuels 14, 109. doi:10.1186/s13068-021-01956-4
Nayak, L., Panda, D., Dash, G. K., Lal, M. K., Swain, P., Baig, M. J., et al. (2022). A chloroplast glycolate catabolic pathway bypassing the endogenous photorespiratory cycle enhances photosynthesis, biomass and yield in rice (Oryza sativa L.). Plant Sci. 314, 111103. doi:10.1016/j.plantsci.2021.111103
Norman, E. G., and Colman, B. (1991). Purification and characterization of phosphoglycolate phosphatase from the cyanobacterium Coccochloris peniocystis. Plant Physiol. 95, 693–698. doi:10.1104/pp.95.3.693
Ort, D. R., Merchant, S. S., Alric, J., Barkan, A., Blankenship, R. E., Bock, R., et al. (2015). Redesigning photosynthesis to sustainably meet global food and bioenergy demand. Proc. Natl. Acad. Sci. U. S. A. 112, 8529–8536. doi:10.1073/pnas.1424031112
Peterhansel, C., Blume, C., and Offermann, S. (2013). Photorespiratory bypasses: how can they work? J. Exp. Bot. 64, 709–715. doi:10.1093/jxb/ers247
Peterhansel, C., Horst, I., Niessen, M., Blume, C., Kebeish, R., Kürkcüoglu, S., et al. (2010). Photorespiration. Arabidopsis Book 8, e0130. doi:10.1199/tab.0130
Peterhansel, C., Krause, K., Braun, H. P., Espie, G. S., Fernie, A. R., Hanson, D. T., et al. (2013b). Engineering photorespiration: current state and future possibilities. Plant Biol. (Stuttg). 15, 754–758. doi:10.1111/j.1438-8677.2012.00681.x
Rademacher, T., Häusler, R. E., Hirsch, H. J., Zhang, L., Lipka, V., Weier, D., et al. (2002). An engineered phosphoenolpyruvate carboxylase redirects carbon and nitrogen flow in transgenic potato plants. Plant J. 32, 25–39. doi:10.1046/j.1365-313x.2002.01397.x
Rai, K. M., Ghose, K., Rai, A., Singh, H., Srivastava, R., and Mendu, V. (2019). “Genome engineering tools in plant synthetic biology,” in Current developments in Biotechnology and bioengineering. Editors S. P. Singh, A. Pandey, G. Du, and S. Kumar (Amsterdam, The Netherlands: Elsevier), 47–73.
Reumann, S., and Weber, A. P. M. (2006). Plant peroxisomes respire in the light: Some gaps of the photorespiratory C2 cycle have become filled-others remain. Biochimica Biophysica Acta - Mol. Cell Res. 1763, 1496–1510. doi:10.1016/j.bbamcr.2006.09.008
Roell, M. S., Schada von Borzykowski, L., Westhoff, P., Plett, A., Paczia, N., Claus, P., et al. (2021). A synthetic C4 shuttle via the β-hydroxyaspartate cycle in C3 plants. Proc. Natl. Acad. Sci. U. S. A. 118, e2022307118. doi:10.1073/pnas.2022307118
Sage, R. F., Sage, T. L., and Kocacinar, F. (2012). Photorespiration and the evolution of C4 photosynthesis. Annu. Rev. Plant Biol. 63, 19–47. doi:10.1146/annurev-arplant-042811-105511
Schada von Borzyskowski, L., Severi, F., Krüger, K., Hermann, L., Gilardet, A., Sippel, F., et al. (2019). Marine Proteobacteria metabolize glycolate via the β-hydroxyaspartate cycle. Nature 575, 500–504. doi:10.1038/s41586-019-1748-4
Scheffen, M., Marchal, D. G., Beneyton, T., Schuller, S. K., Klose, M., Diehl, C., et al. (2021). A new-to-nature carboxylation module to improve natural and synthetic CO2 fixation. Nat. Catal. 4, 105–115. doi:10.1038/s41929-020-00557-y
Sharkey, T. D. (1988). Estimating the rate of photorespiration in leaves. Physiol. Plant. 73, 147–152. doi:10.1111/j.1399-3054.1988.tb09205.x
Shen, B. R., Wang, L. M., Lin, X. L., Yao, Z., Xu, H. W., Zhu, C. H., et al. (2019). Engineering a new chloroplastic photorespiratory bypass to increase photosynthetic efficiency and productivity in rice. Mol. Plant 12, 199–214. doi:10.1016/j.molp.2018.11.013
Shen, B. R., Zhu, C. H., Yao, Z., Cui, L. L., Zhang, J. J., Yang, C. W., et al. (2017). An optimized transit peptide for effective targeting of diverse foreign proteins into chloroplasts in rice. Sci. Rep. 7, 46231. doi:10.1038/srep46231
Shih, P. M., Zarzycki, J., Niyogi, K. K., and Kerfeld, C. A. (2014). Introduction of a synthetic CO2-fixing photorespiratory bypass into a cyanobacterium. J. Biol. Chem. 289, 9493–9500. doi:10.1074/jbc.C113.543132
South, P. F., Cavanagh, A. P., Liu, H. W., and Ort, D. R. (2019). Synthetic glycolate metabolism pathways stimulate crop growth and productivity in the field. Science 363, eaat9077. doi:10.1126/science.aat9077
South, P. F., Cavanagh, A. P., Lopez-Calcagno, P. E., Raines, C. A., and Ort, D. R. (2018). Optimizing photorespiration for improved crop productivity. J. Integr. Plant Biol. 60, 1217–1230. doi:10.1111/jipb.12709
Tiwari, P., Khare, T., Shriram, V., Bae, H., and Kumar, V. (2021). Plant synthetic biology for producing potent phyto-antimicrobials to combat antimicrobial resistance. Biotechnol. Adv. 48, 107729. doi:10.1016/j.biotechadv.2021.107729
Trudeau, D. L., Edlich-Muth, C., Zarzycki, J., Scheffen, M., Goldsmith, M., Khersonsky, O., et al. (2018). Design and in vitro realization of carbon-conserving photorespiration. Proc. Natl. Acad. Sci. U. S. A. 115, E11455-E11464. doi:10.1073/pnas.1812605115
Voon, C. P., and Lim, B. L. (2019). ATP translocation and chloroplast biology. Natl. Sci. Rev. 6, 1073–1076. doi:10.1093/nsr/nwz089
Walker, B. J., VanLoocke, A., Bernacchi, C. J., and Ort, D. R. (2016). The costs of photorespiration to food production now and in the future. Annu. Rev. Plant Biol. 67, 107–129. doi:10.1146/annurev-arplant-043015-111709
Wang, L. M., Shen, B. R., Li, B. D., Zhang, C. L., Lin, M., Tong, P. P., et al. (2019). A synthetic photorespiratory shortcut enhances photosynthesis to boost biomass and grain yield in rice. Mol. Plant 13, 1802–1815. doi:10.1016/j.molp.2020.10.007
Wimmer, D., Bohnhorst, P., Shekhar, V., Hwang, I., and Offermann, S. (2017). Transit peptide elements mediate selective protein targeting to two different types of chloroplasts in the single-cell C4 species Bienertia sinuspersici. Sci. Rep. 7, 41187. doi:10.1038/srep41187
Wingler, A., Lea, P. J., and Leegood, R. C. (1999). Photorespiratory metabolism of glyoxylate and formate in glycine-accumulating mutants of barley and Amaranthus edulis. Planta 207, 518–526. doi:10.1007/s004250050512
Yu, H., Li, X., Duchoud, F., Chuang, D. S., and Liao, J. C. (2018). Augmenting the calvin-benson-bassham cycle by a synthetic malyl-CoA-glycerate carbon fixation pathway. Nat. Commun. 9. doi:10.1038/s41467-018-04417-z
Yu, H., and Liao, J. C. (2018). A modified serine cycle in Escherichia coli coverts methanol and CO2 to two-carbon compounds. Nat. Commun. 9, 3992. doi:10.1038/s41467-018-06496-4
Keywords: carbon fixation, plant chassis, metabolic pathway design, photorespiration, bioproduction
Citation: Wang Q, Yang H, Cao P, Chen F and Zhao L (2022) Biosynthetic approaches to efficient assimilation of CO2 via photorespiration modification in plant chassis. Front. Bioeng. Biotechnol. 10:979627. doi: 10.3389/fbioe.2022.979627
Received: 27 June 2022; Accepted: 13 July 2022;
Published: 08 August 2022.
Edited by:
Tao Chen, Tianjin University, ChinaReviewed by:
Chikahiro Miyake, Kobe University, JapanCopyright © 2022 Wang, Yang, Cao, Chen and Zhao. This is an open-access article distributed under the terms of the Creative Commons Attribution License (CC BY). The use, distribution or reproduction in other forums is permitted, provided the original author(s) and the copyright owner(s) are credited and that the original publication in this journal is cited, in accordance with accepted academic practice. No use, distribution or reproduction is permitted which does not comply with these terms.
*Correspondence: Lei Zhao, zhaol@tib.cas.cn