- Institute for Molecular Microbiology and Biotechnology, University of Münster, Münster, Germany
Exopolysaccharides formation against harmful biotic and abiotic environmental influences is common among bacteria. By using renewable resources as a substrate, exopolysaccharides represent a sustainable alternative to fossil-based polymers as rheological modifiers in food, cosmetics, and pharmaceutical applications. The family of Acetobacteraceae, traditionally associated with fermented food products, has demonstrated their ability to produce a wide range of structural and functional different polymers with interesting physicochemical properties. Several strains are well known for their production of homopolysaccharides of high industrial importance, such as levan and bacterial cellulose. Moreover, some Acetobacteraceae are able to form acetan-like heteropolysaccharides with a high structural resemblance to xanthan. This mini review summarizes the current knowledge and recent trends in both homo- and heteropolysaccharide production by Acetobacteraceae.
1 Introduction
The biosynthesis of carbohydrate polymers is a common characteristic of both prokaryotic and eukaryotic organisms. Extracellularly secreted glycosides are classified as exopolysaccharides (EPS). Major functions include the protection against environmental influences such as desiccation, osmotic stress, phagocytosis, or antibiotics. Furthermore, intercellular interactions like cell recognition and surface adhesion are also promoted (Suresh Kumar et al., 2007; Corbett et al., 2010; Moradali and Rehm, 2020). EPS are known for their high diversity in terms of physicochemical and rheological properties (Hundschell and Wagemans, 2019).
EPS are either classified as homopolysaccharides or heteropolysaccharides based on their general chemical complexity. Although homopolysaccharides consist per definition of only one kind of monomer, the linkage pattern usually varies a lot resulting in branched (e.g. glycogen) and unbranched (e.g. cellulose) polymer structures. Heteropolysaccharides, on the other hand tend to have highly complex structures as they are composed of at least two different sugar moieties. Additionally, polymers can be further decorated with organic and inorganic moieties such as acetyl, pyruvyl, glyceryl, succinyl, and sulphate constituents (Sutherland, 1990; Freitas et al., 2011; Nwodo et al., 2012). Along with the conformation of glycosidic linkages, a vast amount of potential structures emerge, giving rise to a wide range of physicochemical properties (Freitas et al., 2011).
Those variable material properties in combination with a high natural water-binding capacity are one of the main reasons for the broad commercial potential of EPS, representing an alternative to replace petrochemical polymers in current applications (Kamaruddin et al., 2021). However, only a rather limited number of microbial EPS can be regarded as industrially established, e.g. hyaluronan, xanthan, pullulan, dextran and gellan gum (Sutherland, 1998; Heinze et al., 2006; Osmałek et al., 2014; Singhsa et al., 2018; Schilling et al., 2020; Meliawati et al., 2022). Low titers and yields, as well as expensive downstream processing, result in high production costs and consequently impede the industrial establishment of new strains and polymers. Thus, EPS are up to now mainly used in high-value niche products in cosmetics, food, and pharmacy (More et al., 2021). This applies in particular to the Gram-negative Acetobacteraceae which are mainly known for the production of fermented food products like vinegar, kefir, or acetic acid production but have also demonstrated their ability to produce structurally different EPS with interesting physicochemical properties (La China et al., 2018).
This mini review aims to summarize the knowledge of homopolysaccharides and heteropolysaccharides production in Acetobacteraceae with regard to the current state of strain development, bioprocess optimization, and knowledge of rheological properties to evaluate the status quo and provide a further outlook on this particular group of promising biopolymers. Since the phylogenetic classification of Acetobacteraceae is not finalized and currently consists of 47 genera in April 2022, the classification of the original publication is used in this article (Parr et al., 2014).
2 Homopolysaccharide production in Acetobacteraceae
2.1 Levan
Acetobacteraceae are known for their production of high-value homopolysaccharides such as levan. Levan synthesis is widespread within the family of Acetobacteraceae and was reported for numerous organisms including the genera Neoasaia, Kozakia, and Gluconobacter (Kornmann et al., 2003; Hermann et al., 2015; Hövels et al., 2020; Anguluri et al., 2022). Its formation is catalyzed by an extracellular enzyme named levansucrase (LS, EC 2.4.1.10). By cleaving sucrose, LSs are capable of polymerizing the emerging D-fructose monomers to β-(2,6) linked polyfructans (Öner et al., 2016; Xu et al., 2019). Meanwhile, D-glucose as a sacrificial substrate is metabolized and used for bacterial growth resulting in a theoretical maximum levan yield of 0.5 gLev/gSuc. Based on the evaluation of phylogenetic clades in Acetobacteraceae, two types of LS with different ecological relationship could be distinguished, differing in yield and molecular weight (Jakob et al., 2019). Levan is currently highly requested as a stabilizer, emulsifier, and flavor enhancing agent in food applications (Öner et al., 2016).
Up to now, investigations on levan production by Acetobacteraceae focused mainly on the characterization of wild-type strains which might be explained by the large number of levan-producing strains in this particular family as well as high titers already obtained under non-optimized cultivation conditions (Table 1). Comparatively low titers of 6.3 g L-1 and 7.3 g L-1 were reported for cultivations in shake flask experiments for Gluconobacter cerinus DSM 9533 and Neoasaia chiangmaiensis NBRC 101099, respectively. Slightly higher titers of 7.8 g L-1 were obtained under identical conditions for Kozakia baliensis DSM 14400 (Jakob et al., 2012). However, for all strains carbon yields remained at a low level of approximately 0.1 gLev/gSuc. Anguluri et al. (2022) reported a final titer of 35.0 g L-1 for the same Neoasaia chiangmaiensis strain after increasing the final sucrose concentration up to 250 g L-1. Despite increased product titers, in both studies carbon yields of only 0.10 and 0.14 gLev/gSuc were achieved, respectively. For Gluconacetobacter diazotrophicus PA1 5 a decent titer of 24.8 g L-1 was obtained showing similar carbon yields (0.16 gLev/gSuc). Significantly higher yields of 0.33 and 0.38 gLev/gSuc were observed for the species Acetobacter xylinum NCIM 2526 and Gluconobacter frateurii TMW 2.767, respectively (Jakob et al., 2012; Semjonovs et al., 2016). Recently, Tanticharoenia sakaeratensis TBRC 22 was identified as a promising alternative production strain with a final levan titer of 24.7 g L-1 using 200 g L-1 sucrose as the initial substrate concentration (Aramsangtienchai et al., 2020). By plasmid-based overexpression of the native LS gene sacB in Gluconobacter japonicus LMG 2417, LS activity could be successfully increased 2.5-fold compared to the wild-type strain, resulting in higher space-time yields and titers (Hövels et al., 2020). In general, in-depth investigations on bioprocess optimization approaches for levan production in Acetobacteraceae seem to be rare and mainly limited to the identification of the best media compositions so far as extensively reviewed by Öner et al. (2016).
Levan formation is controlled by LS as the only enzyme in the biosynthesis process (Schmid, 2018). Depending on the available fructosyl acceptor molecule, the enzyme catalyzes hydrolysis, transfructosylation (in the presence of small oligosaccharides) and polymerization (in the presence of a increasing fructan chain) (Li et al., 2015). In consequence, defined process conditions are essential to push the reaction equilibrium towards levan formation while avoiding product degradation. Several studies in Gram-positive and Gram-negative bacteria demonstrated the importance of the right temperature settings during cultivation and the influence of metal ions, which need to be carefully determined for each LS respectively (Park et al., 2003; Rairakhwada et al., 2010; Tian et al., 2011; Belghith et al., 2012). Moreover, the optimal length of the fermentation process has to be carefully evaluated since the equilibrium naturally tends towards hydrolysis with the depletion of sucrose as the substrate during the cultivation (Chambert et al., 1974; Hernandez et al., 1995). In a recent study of Anguluri et al. (2022), the authors could show that a longer process time of 96 h resulted in a significant product decrease for Kozakia baliensis DSM 14400 in comparison to 48 h of cultivation. In contrast, 96 h of fermentation increased yields for Neoasaia chiangmaiensis NBRC 101099 by 32 %, thus underlining the need for further strain-specific bioprocess optimization approaches.
2.2 Bacterial cellulose
In addition, Acetobacteraceae are associated with the biosynthesis of β-(1,4) linked polyglucans which are referred to as bacterial cellulose (BC). Due to the absence of hemicellulose and lignin as present in its eukaryotic plant counterpart, BC is known to be of extremely high purity. Moreover, due to the lack of required energy-intensive downstream processing which is essential for plant-derived cellulose, BC typically demonstrates a low amount of inorganic impurities (Klemm et al., 2011). In applications, BC is valued for its high crystallinity and superior mechanical strength (Nakayama et al., 2004; Castro et al., 2011; Ul-Islam et al., 2012). All of these properties are highly desired in current product development and makes BC an excellent biocompatible material for pharmaceutical products. High potential is reported for wound dressing materials, drug delivery systems and packing materials (Czaja et al., 2006; Abeer et al., 2014; El-Gendi et al., 2023). In order to address this trend, current research focus on in situ (optimization during fermentation) and ex situ (optimization of existing microfibers) BC properties modifications (Stumpf et al., 2018; Cazón and Vázquez, 2021). Addition of 30% (v/v) aloe vera gel for instant resulted in significantly increased mechanical strength and water absorption capacity (Saibuatong and Phisalaphong, 2010).
Traditionally, BC is generated in the air-liquid interface in static fermentation processes. By accumulation on the surface, a gelatinous layer around bacterial cells is formed (Cannon and Anderson, 1991; Jonas and Farah, 1998). In consequence, maximum yields positively correlate to the surface area (Masaoka et al., 1993). However, this leads to several practical problems during production, e.g. insufficient oxygen supply and long lasting fermentations, or a barely separable mixture of biomass and polymer (Shoda and Sugano, 2005; Hsieh et al., 2016). Especially when it comes to industrial scale-up, these issues limit the economic feasibility. Production in large scale are therefore conducted in modified horizontal lift, gas lift, rotary discs and membrane bioreactors (Shi et al., 2014). Titers of 6.2 g L-1 BC were achieved by using a rotary biofilm conductor with eight discs (Kim et al., 2007). However, it has to be mentioned that the optimal static process conditions are often not met completely in those set-ups.
In order to reduce manufacturing costs, optimization approaches focus nowadays more on the establishment of low-cost media and the investigation of alternative raw materials in order to replace glucose, fructose or glycerol as established substrates (Jalili Tabaii and Emtiazi, 2015; Mohammadkazemi et al., 2015; Molina-Ramírez et al., 2017; Revin et al., 2018; Saleh et al., 2021; El-Gendi et al., 2022). Tyagi and Suresh achieved remarkable titers of 12.6 g L-1 for BC with Gluconaceteobacter intermedius SNT1 on sugarcane molasses (Tyagi and Suresh, 2016). Numerous further publications indicate the high potential of this approach, including the redirection of waste streams and by-products of chemical processes (Hong et al., 2012; Chen et al., 2013; Vazquez et al., 2013; Barshan et al., 2019). In addition, BC can also be produced in submerge cultivation systems through agitated or aerated bioreactors with respectable titers between 15 and 20 g L-1 BC (Kouda et al., 1998). However, the occurrence of unintended cellulose-deficient mutants and therefore a decline in product titers have been reported in several studies (Vandamme et al., 1998; Jung et al., 2005; Matsutani et al., 2015). Moreover, higher oxygen supply during cultivation was demonstrated to alter BC morphology towards granule and pellet formation, thus affecting material properties (Singhsa et al., 2018). Recent trends also focus on the impact of additives and co-cultivations in order to optimize both BC titers and rheological properties. Positive effects were demonstrated for pullulan, whose supplementation resulted in improved mechanical polymer properties and 4.4-fold increased BC yield (Hu et al., 2022).
Contrarily to the previously discussed levan-type polyfructans, BC biosynthesis and polymerization is more complex as it is organized in a cellulase synthase operon consisting of at least four different genes (Römling and Galperin, 2015). Several studies aimed to increase and optimize BC production on a molecular level. In order to enable metabolization of sucrose as a cheaper carbon source, a recombinant sucrose synthase was successfully expressed in Acetobacter xylinum BRP 2001. By this, final titers on glucose as carbon source could be doubled to 8 g L-1 (Nakai et al., 1999). Furthermore, 28-fold increased BC formation was demonstrated for Acetobacter xylinus ITZ3 after the successful genomic integration of the β-galactosidase lacZ, thus adding lactose to the group of potential substrates (Battad-Bernardo et al., 2004). Heterologous expression studies might present one way to overcome the prominent issue of long lasting cultivation by Komagataeibacter spp. Imai et al. (2014) demonstrated BC production via the much faster growing Escherichia coli by heterologous expression of the cellulase synthase complex subunits cesAB as well as the cyclic-di-GMP diguanylate cylase dgc of Gluconacetobacter xylinus (Imai et al., 2014). Recently, for the first time, a CRISPR-Cas tool was successfully applied in Komagataeibacter spp. The study of Huang et al. (2020), used a CRISPRi-based approach to downregulate galU, which controls the metabolic flux between the BC synthesis and the pentose phosphate pathway. By minimizing the expression level of galU, BC of higher crystallinity was obtained, although enhanced material porosity as an severe adverse effect was documented as well (Huang et al., 2020).
3 Heteropolysaccharide production in Acetobacteraceae
The formation of heteropolysaccharides within the family of Acetobacteraceae has been investigated in several publications (Brandt et al., 2016; Škraban et al., 2018; Rath et al., 2022). Interestingly, many if not all of the yet elucidated heteropolysaccharides in this family are structural related to acetan, whose production was first described in Acetobacter xylinum (Figure 1). Acetan consists of a molar subunit ratio of 4 : 1: 1 : 1 (glucose, mannose, glucuronic acid, rhamnose). In addition to the cellulose-like backbone with a trisaccharide branching sidechain at every other glucose monomer, the first two monomers of the side chain, identified as mannose and glucuronic acid, are identical in sequence and linkage pattern to the core structure of xanthan gum (Jansson et al., 1975; Couso et al., 1987). However, the further side chain composition and acetyl- and pyruvation pattern differs, giving rise to variety of structures and different rheological properties (Tayama et al., 1986; Brandt et al., 2018; Rath et al., 2022). This resemblance is also displayed by a high degree of homology between the heteropolysaccharides encoding genomic regions in Acetobacteraceae and the xanthan biosynthesis cluster of Xanthomonas campestris (Becker et al., 1998). Genetic alignments demonstrated a strong homology for aceA of Acetobacter xylinum and gumD from Xanthomonas campestris, both of these so-called priming glycosyltransferases in heteropolysaccharides synthesis initiating the assembly of the repeating unit at an undecaprenyl-pyrophosphate lipid anchor (Griffin et al., 1994; Schmid and Sieber, 2015). Moreover, a more recent study of Brandt et al. (2016) compared and confirmed homologies in the underlying heteropolysaccharides biosynthesis clusters of Kozakia baliensis DSM 14400 and NBRC 16680, Gluconacetobacter diazotrophicus PA1 5, Komagataeibacter xylinus E25 and Xanthomonas campestris ATCC 33913. Although all of the examined clusters showed high structural similarities, variations in numbers and size of the predicted genes and clusters were revealed, explaining the strain-dependent differences in the resulting polymer structures.
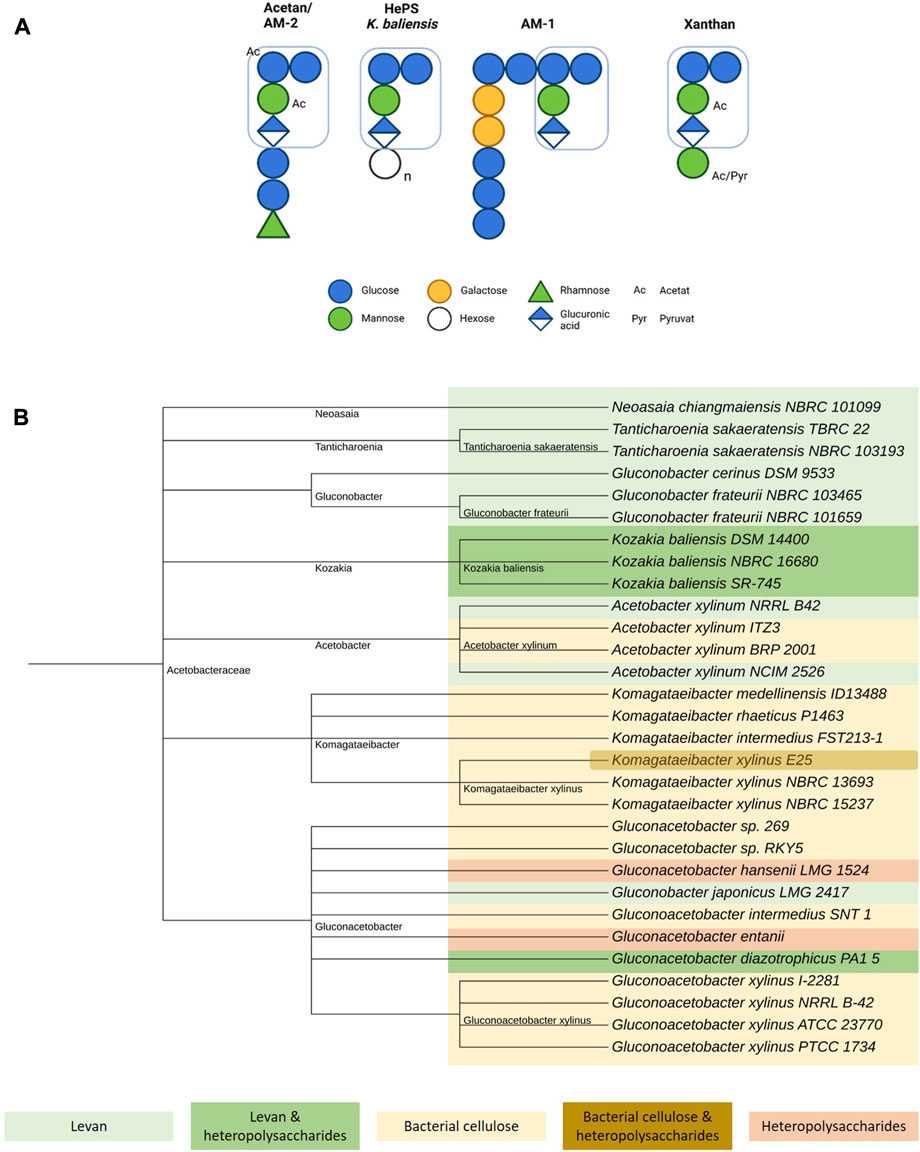
FIGURE 1. Overview of acetan-like heteropolysaccharide production in Acetobacteraceae. (A) Schematic comparison of selected acetan-like heteropolysaccharides produced by Acetobacteraceae including acetan, a yet unnamed heteropolysaccharide by Kozakia baliensis, AM-1, and xanthan (Jansson et al., 1975; Tayama et al., 1985, 1986; Edwards et al., 1999; Brandt et al., 2018). The xanthan-like core structure is marked for all polymers. Figure created with BioRender.com. (B) Taxonomy tree of EPS-producing Acetobacteraceae. Levan producing strains are marked in light green, levan and acetan-like heteropolysaccharides producing strains in dark green, bacterial cellulose producing strains in yellow, bacterial cellulose and acetan-like heteropolysaccharide producing strains in brown and only acetan-like heteropolysaccharide producing strains in light red. Figure created with iTOL (Letunic and Bork, 2021).
Xanthan gum is highly requested in industrial applications as a viscosifier due to its pseudoplastic behavior, high salt tolerance and thermostability amongst others properties (Chaturvedi et al., 2021). Similar beneficial rheological characteristics have also been described for the structure-related heteropolysaccharides of Acetobacteraceae, although studies in this field are rather limited. Already in 1989, the first rheological characterization of acetan was performed (Morris et al., 1989). Moreover, rheological behavior investigations of heteropolysaccharides produced by Kozakia baliensis confirmed pseudoplastic behavior and high viscosity (Brandt et al., 2018). Although the first results appear to be promising, further in-depth rheological studies are absolutely required in consideration of the rather insufficient data situation.
With regard to strain cultivation, respectable titers for heteropolysaccharides production in Acetobacteraceae wild-type strains have been reported. A titer of 5.4 g L-1 acetan was obtained under controlled cultivation for Gluconoacetobacter entanii (Velasco-Bedrán and López-Isunza, 2007). Significantly higher titers of 11.3 g L-1 gluconacetan were achieved for Gluconoacetobacter xylinus I-2281, likewise under controlled fermentation conditions in bioreactors and using fructose as the main carbon source (Kornmann et al., 2003). In a recent study based on an systematic optimization by use of experimental design, the putative gluconacetan titer for Gluconoacetobacter sp. could be even increased to 25.4 g L-1 although the parallel formation of second ribose-containing heteropolysaccharides could not be completely precluded (Rath et al., 2022). By using glycerol as the carbon source, the authors aimed to minimize the formation and accumulation of undesirable oxidized compounds such as gluconates, which affect the pH of the fermentation broth and contaminate the final polymer. The oxidation of sugar and alcohols within the respiratory chain mechanism in the outer membrane is a characteristic feature of Acetobacteraceae (Adachi and Yakushi, 2016). As the formation of numerous (by-) products is a main issue for Acetobacteraceae, the right choice of carbon source and cultivation conditions are critical for EPS production and should be investigated further. Moreover, cultivation of Gluconacetobacter hansenii LMG 1524 in a media consisting of glycerol as the main carbon source and ammonium sulphate as the corresponding nitrogen source resulted in a maximum titer of 1.22 g L-1, in comparison to other examined carbon and nitrogen sources variations (Valepyn et al., 2012). This once more underlines the importance of strain-dependent bioprocess optimization as the authors were also able to demonstrate that lower temperatures at 25°C and a slightly decreased pH value of 5.0 favored EPS over cell biomass production. Cultivation in the presence of two initial carbon sources (glucose and fructose) and 200 mg L-1 of magnesium resulted in a titer of 3.9 g L-1 for Kozakia baliensis NBRC 16680 in shake flasks (Brandt et al., 2018). Additional magnesium has previously been shown to positively affect heteropolysaccharides production in Pseudomonadaceae (Vargas-García et al., 2001). However, in the previously mentioned study of Brandt, significantly increased EPS production in Kozakia baliensis due to the presence of magnesium could not be confirmed.
4 Conclusion and further perspectives
The increasing demand for healthier and more sustainable products as driven by the customers, offers a unique chance to increase the replacement of petrol-based compounds and chemicals in a broad range of applications. Hugh potential can be assumed for EPS which possess the required material properties for usage in food, cosmetic and pharmaceutical applications. This applies especially to EPS produced by Acetobacteraceae, whose homopolysaccharides levan and BC have shown promising material properties. Due to their structural resemblance to xanthan, acetan-like heteropolysaccharides are also highly interesting.
However, for industrial scale-up processes and in order to enhance economic feasible production, future research must address the need for higher titers and carbon yields as well as utilization of second-generation feed stocks to produce both homopolysaccharides and heteropolysaccharides. In addition, investigation and improvement of rheological polymer properties via genetic engineering or fine-tuned formulations are also highly desired to promote future application development for acetan-like polymers.
Author contributions
JW: Literature research, conceptualization, visualization, writing–original draft, writing–review and editing; JS: Conceptualization, writing–review and editing, funding acquisition.
Funding
JW would like to thank the State of North Rhine-Westphalia’s Ministry of Economic Affairs, Innovation, Digitalization, and Energy (Germany) as well as the Exzellenz Start-up Center. NRW program at the REACH - EUREGIO Start-Up Center (Grant No. 03ESCNW09) for their kind support of her work.
Acknowledgments
The authors would like to thank Christoph Schilling for the fruitful discussions.
Conflict of interest
The authors declare that the research was conducted in the absence of any commercial or financial relationships that could be construed as a potential conflict of interest.
Publisher’s note
All claims expressed in this article are solely those of the authors and do not necessarily represent those of their affiliated organizations, or those of the publisher, the editors and the reviewers. Any product that may be evaluated in this article, or claim that may be made by its manufacturer, is not guaranteed or endorsed by the publisher.
References
Abeer, M. M., Mohd Amin, M. C. I., and Martin, C. (2014). A review of bacterial cellulose-based drug delivery systems: Their biochemistry, current approaches and future prospects. J. Pharm. Pharmacol. 66, 1047–1061. doi:10.1111/jphp.12234
Adachi, O., and Yakushi, T. (2016). “Membrane-bound dehydrogenases of acetic acid bacteria,” in Acetic acid bacteria. Editors K. Matsushita, H. Toyama, N. Tonouchi, and A. Okamoto-Kainuma (Tokyo: Springer Japan), 273–297. doi:10.1007/978-4-431-55933-7_13
Anguluri, K., La China, S., Brugnoli, M., De Vero, L., Pulvirenti, A., Cassanelli, S., et al. (2022). Candidate acetic acid bacteria strains for levan production. Polymers 14, 2000. doi:10.3390/polym14102000
Aramsangtienchai, P., Kongmon, T., Pechroj, S., and Srisook, K. (2020). Enhanced production and immunomodulatory activity of levan from the acetic acid bacterium, Tanticharoenia sakaeratensis. Int. J. Biol. Macromol. 163, 574–581. doi:10.1016/j.ijbiomac.2020.07.001
Barshan, S., Rezazadeh-Bari, M., Almasi, H., and Amiri, S. (2019). Optimization and characterization of bacterial cellulose produced by Komagatacibacter xylinus PTCC 1734 using vinasse as a cheap cultivation medium. Int. J. Biol. Macromol. 136, 1188–1195. doi:10.1016/j.ijbiomac.2019.06.192
Battad-Bernardo, E., McCrindle, S. L., Couperwhite, I., and Neilan, B. A. (2004). Insertion of an E. coli lacZ gene in Acetobacter xylinus for the production of cellulose in whey. FEMS Microbiol. Lett. 231, 253–260. doi:10.1016/S0378-1097(04)00007-2
Becker, A., Katzen, F., Pühler, A., and Ielpi, L. (1998). Xanthan gum biosynthesis and application: A biochemical/genetic perspective. Appl. Microbiol. Biotechnol. 50, 145–152. doi:10.1007/s002530051269
Belghith, K. S., Dahech, I., Belghith, H., and Mejdoub, H. (2012). Microbial production of levansucrase for synthesis of fructooligosaccharides and levan. Int. J. Biol. Macromol. 50, 451–458. doi:10.1016/j.ijbiomac.2011.12.033
Brandt, J. U., Jakob, F., Behr, J., Geissler, A. J., and Vogel, R. F. (2016). Dissection of exopolysaccharide biosynthesis in Kozakia baliensis. Microb. Cell Fact. 15, 170. doi:10.1186/s12934-016-0572-x
Brandt, J. U., Jakob, F., Wefers, D., Bunzel, M., and Vogel, R. F. (2018). Characterization of an acetan-like heteropolysaccharide produced by Kozakia baliensis NBRC 16680. Int. J. Biol. Macromol. 106, 248–257. doi:10.1016/j.ijbiomac.2017.08.022
Cannon, R. E., and Anderson, S. M. (1991). Biogenesis of bacterial cellulose. Crit. Rev. Microbiol. 17, 435–447. doi:10.3109/10408419109115207
Castro, C., Zuluaga, R., Putaux, J.-L., Caro, G., Mondragon, I., and Gañán, P. (2011). Structural characterization of bacterial cellulose produced by Gluconacetobacter swingsii sp. from Colombian agroindustrial wastes. Carbohydr. Polym. 84, 96–102. doi:10.1016/j.carbpol.2010.10.072
Cazón, P., and Vázquez, M. (2021). Improving bacterial cellulose films by ex-situ and in-situ modifications: A review. Food Hydrocoll. 113, 106514. doi:10.1016/j.foodhyd.2020.106514
Chambert, R., Treboul, G., and Dedonder, R. (1974). Kinetic studies of levansucrase of Bacillus subtilis. Eur. J. Biochem. 41, 285–300. doi:10.1111/j.1432-1033.1974.tb03269.x
Chaturvedi, S., Kulshrestha, S., Bhardwaj, K., and Jangir, R. (2021). “A review on properties and applications of xanthan gum,”. Microbial polymers. Editors A. Vaishnav, and D. K. Choudhary (Singapore: Springer Singapore), 87–107. doi:10.1007/978-981-16-0045-6_4
Chen, L., Hong, F., Yang, X., and Han, S. (2013). Biotransformation of wheat straw to bacterial cellulose and its mechanism. Bioresour. Technol. 135, 464–468. doi:10.1016/j.biortech.2012.10.029
Corbett, D., Hudson, T., and Roberts, I. S. (2010). “Bacterial polysaccharide capsules,” in Prokaryotic cell wall compounds. Editors H. König, H. Claus, and A. Varma (Berlin, Heidelberg: Springer Berlin Heidelberg), 111–132. doi:10.1007/978-3-642-05062-6_3
Couso, R. O., Ielpi, L., and Dankert, M. A. (1987). A xanthan-gum-like polysaccharide from Acetobacter xylinum. Microbiology 133, 2123–2135. doi:10.1099/00221287-133-8-2123
Czaja, W., Krystynowicz, A., Bielecki, S., and Brownjr, R. (2006). Microbial cellulose—The natural power to heal wounds. Biomaterials 27, 145–151. doi:10.1016/j.biomaterials.2005.07.035
Edwards, K. J., Jay, A. J., Colquhoun, I. J., Morris, V. J., Gasson, M. J., and Griffin, A. M. (1999). Generation of a novel polysaccharide by inactivation of the aceP gene from the acetan biosynthetic pathway in Acetobacter xylinum. Microbiology 145, 1499–1506. doi:10.1099/13500872-145-6-1499
El-Gendi, H., Salama, A., El-Fakharany, E. M., and Saleh, A. K. (2023). Optimization of bacterial cellulose production from prickly pear peels and its ex situ impregnation with fruit byproducts for antimicrobial and strawberry packaging applications. Carbohydr. Polym. 302, 120383. doi:10.1016/j.carbpol.2022.120383
El-Gendi, H., Taha, T. H., Ray, J. B., and Saleh, A. K. (2022). Recent advances in bacterial cellulose: A low-cost effective production media, optimization strategies and applications. Cellulose 29, 7495–7533. doi:10.1007/s10570-022-04697-1
Freitas, F., Alves, V. D., and Reis, M. A. M. (2011). Advances in bacterial exopolysaccharides: From production to biotechnological applications. Trends Biotechnol. 29, 388–398. doi:10.1016/j.tibtech.2011.03.008
Griffin, A. M., Morris, V. J., and Gasson, M. J. (1994). Genetic analysis of the acetan biosynthetic pathway in Acetobacter xylinum. Int. J. Biol. Macromol. 16, 287–289. doi:10.1016/0141-8130(94)90057-4
Heinze, T., Liebert, T., Heublein, B., and Hornig, S. (2006). “Functional polymers based on dextran,” in Polysaccharides II advances in polymer science. Editor D. Klemm (Germany: Springer Berlin Heidelberg), 199–291. doi:10.1007/12_100
Hermann, M., Petermeier, H., and Vogel, R. F. (2015). Development of novel sourdoughs with in situ formed exopolysaccharides from acetic acid bacteria. Eur. Food Res. Technol. 241, 185–197. doi:10.1007/s00217-015-2444-8
Hernandez, L., Arrieta, J., Menendez, C., Vazquez, R., Coego, A., Suarez, V., et al. (1995). Isolation and enzymic properties of levansucrase secreted by Acetobacter diazotrophicus SRT4, a bacterium associated with sugar cane. Biochem. J. 309, 113–118. doi:10.1042/bj3090113
Hong, F., Guo, X., Zhang, S., Han, S., Yang, G., and Jönsson, L. J. (2012). Bacterial cellulose production from cotton-based waste textiles: Enzymatic saccharification enhanced by ionic liquid pretreatment. Bioresour. Technol. 104, 503–508. doi:10.1016/j.biortech.2011.11.028
Hövels, M., Kosciow, K., Kniewel, J., Jakob, F., and Deppenmeier, U. (2020). High yield production of levan-type fructans by Gluconobacter japonicus LMG 1417. Int. J. Biol. Macromol. 164, 295–303. doi:10.1016/j.ijbiomac.2020.07.105
Hsieh, J.-T., Wang, M.-J., Lai, J.-T., and Liu, H.-S. (2016). A novel static cultivation of bacterial cellulose production by intermittent feeding strategy. J. Taiwan Inst. Chem. Eng. 63, 46–51. doi:10.1016/j.jtice.2016.03.020
Hu, H., Catchmark, J. M., and Demirci, A. (2022). Effects of pullulan additive and co-culture of Aureobasidium pullulans on bacterial cellulose produced by. Komagataeibacter Hansen. Bioprocess Biosyst. Eng. 45, 573–587. doi:10.1007/s00449-021-02680-x
Huang, L., Liu, Q., Sun, X., Li, X., Liu, M., Jia, S., et al. (2020). Tailoring bacterial cellulose structure through CRISPR interference-mediated downregulation of galU in Komagataeibacter xylinus CGMCC 2955. Biotechnol. Bioeng. 117, 2165–2176. doi:10.1002/bit.27351
Hundschell, C. S., and Wagemans, A. M. (2019). Rheology of common uncharged exopolysaccharides for food applications. Curr. Opin. Food Sci. 27, 1–7. doi:10.1016/j.cofs.2019.02.011
Imai, T., Sun, S., Horikawa, Y., Wada, M., and Sugiyama, J. (2014). Functional reconstitution of cellulose synthase in Escherichia coli. Biomacromolecules 15, 4206–4213. doi:10.1021/bm501217g
Jakob, F., Quintero, Y., Musacchio, A., Estrada-de los Santos, P., Hernández, L., and Vogel, R. F. (2019). Acetic acid bacteria encode two levansucrase types of different ecological relationship. Environ. Microbiol. 21, 4151–4165. doi:10.1111/1462-2920.14768
Jakob, F., Steger, S., and Vogel, R. F. (2012). Influence of novel fructans produced by selected acetic acid bacteria on the volume and texture of wheat breads. Eur. Food Res. Technol. 234, 493–499. doi:10.1007/s00217-011-1658-7
Jalili Tabaii, M., and Emtiazi, G. (2015). Comparison of bacterial cellulose production among different strains and fermented media. Appl. Food Biotechnol. 3. doi:10.22037/afb.v3i1.10582
Jansson, P., Kenne, L., and Lindberg, B. (1975). Structure of the extracellular polysaccharide from Xanthomonas campestris. Carbohydr. Res. 45, 275–282. doi:10.1016/S0008-6215(00)85885-1
Jonas, R., and Farah, L. F. (1998). Production and application of microbial cellulose. Polym. Degrad. Stab. 59, 101–106. doi:10.1016/S0141-3910(97)00197-3
Jung, J. Y., Park, J. K., and Chang, H. N. (2005). Bacterial cellulose production by Gluconacetobacter hansenii in an agitated culture without living non-cellulose producing cells. Enzyme Microb. Technol. 37, 347–354. doi:10.1016/j.enzmictec.2005.02.019
Kamaruddin, I., Dirpan, A., and Bastian, F. (2021). The novel trend of bacterial cellulose as biodegradable and oxygen scavenging films for food packaging application: An integrative review. IOP Conf. Ser. Earth Environ. Sci. 807, 022066. doi:10.1088/1755-1315/807/2/022066
Kim, Y.-J., Kim, J.-N., Wee, Y.-J., Park, D.-H., and Ryu, H.-W. (2007). Bacterial cellulose production by Gluconacetobacter sp. PKY5 in a rotary biofilm contactor. Appl. Biochem. Biotechnol. 137–140, 529–537. doi:10.1007/s12010-007-9077-8
Klemm, D., Kramer, F., Moritz, S., Lindström, T., Ankerfors, M., Gray, D., et al. (2011). Nanocelluloses: A new family of nature-based materials. Angew. Chem. Int. Ed. 50, 5438–5466. doi:10.1002/anie.201001273
Kornmann, H., Duboc, P., Marison, I., and von Stockar, U. (2003). Influence of nutritional factors on the nature, yield, and composition of exopolysaccharides produced by Gluconacetobacter xylinus I-2281. Appl. Environ. Microbiol. 69, 6091–6098. doi:10.1128/AEM.69.10.6091-6098.2003
Kouda, T., Naritomi, T., Yano, H., and Yoshinaga, F. (1998). Inhibitory effect of carbon dioxide on bacterial cellulose production by Acetobacter in agitated culture. J. Ferment. Bioeng. 85, 318–321. doi:10.1016/S0922-338X(97)85682-6
La China, S., Zanichelli, G., De Vero, L., and Gullo, M. (2018). Oxidative fermentations and exopolysaccharides production by acetic acid bacteria: A mini review. Biotechnol. Lett. 40, 1289–1302. doi:10.1007/s10529-018-2591-7
Letunic, I., and Bork, P. (2021). Interactive tree of life (iTOL) v5: An online tool for phylogenetic tree display and annotation. Nucleic Acids Res. 49, W293–W296. doi:10.1093/nar/gkab301
Li, W., Yu, S., Zhang, T., Jiang, B., and Mu, W. (2015). Recent novel applications of levansucrases. Appl. Microbiol. Biotechnol. 99, 6959–6969. doi:10.1007/s00253-015-6797-5
Masaoka, S., Ohe, T., and Sakota, N. (1993). Production of cellulose from glucose by Acetobacter xylinum. J. Ferment. Bioeng. 75, 18–22. doi:10.1016/0922-338X(93)90171-4
Matsutani, M., Ito, K., Azuma, Y., Ogino, H., Shirai, M., Yakushi, T., et al. (2015). Adaptive mutation related to cellulose producibility in Komagataeibacter medellinensis (Gluconacetobacter xylinus) NBRC 3288. Appl. Microbiol. Biotechnol. 99, 7229–7240. doi:10.1007/s00253-015-6598-x
Meliawati, M., Gansbiller, M., and Schmid, J. (2022). “Hyaluronic acid (hyaluronan),” in Microbial Production of high-value products microbiology monographs. Editors B. H. A. Rehm, and D. Wibowo (Cham: Springer International Publishing), 159–184. doi:10.1007/978-3-031-06600-9_7
Mohammadkazemi, F., Azin, M., and Ashori, A. (2015). Production of bacterial cellulose using different carbon sources and culture media. Carbohydr. Polym. 117, 518–523. doi:10.1016/j.carbpol.2014.10.008
Molina-Ramírez, C., Castro, M., Osorio, M., Torres-Taborda, M., Gómez, B., Zuluaga, R., et al. (2017). Effect of different carbon sources on bacterial nanocellulose production and structure using the low pH resistant strain komagataeibacter medellinensis. Materials 10, 639. doi:10.3390/ma10060639
Molinari, M. L., and Boiardi, J. L. (2013). Levans production by Gluconacetobacter diazotrophicus. Electron. J. Biotechnol. 16. doi:10.2225/vol16-issue3-fulltext-9
Moradali, M. F., and Rehm, B. H. A. (2020). Bacterial biopolymers: From pathogenesis to advanced materials. Nat. Rev. Microbiol. 18, 195–210. doi:10.1038/s41579-019-0313-3
More, V. S., Ebinesar, A., Prakruthi, A., Praveen, P., Fasim, A., Rao, A., et al. (2021). “Isolation and purification of microbial exopolysaccharides and their industrial application,” in Microbial polymers. Editors A. Vaishnav, and D. K. Choudhary (Singapore: Springer Singapore), 69–86. doi:10.1007/978-981-16-0045-6_3
Morris, V. J., Brownsey, G. J., Cairns, P., Chilvers, G. R., and Miles, M. J. (1989). Molecular origins of acetan solution properties. Int. J. Biol. Macromol. 11, 326–328. doi:10.1016/0141-8130(89)90002-0
Nakai, T., Tonouchi, N., Konishi, T., Kojima, Y., Tsuchida, T., Yoshinaga, F., et al. (1999). Enhancement of cellulose production by expression of sucrose synthase in Acetobacter xylinum. Proc. Natl. Acad. Sci. U.S.A. 96, 14–18. doi:10.1073/pnas.96.1.14
Nakayama, A., Kakugo, A., Gong, J. P., Osada, Y., Takai, M., Erata, T., et al. (2004). High mechanical strength double-network hydrogel with bacterial cellulose. Adv. Funct. Mat. 14, 1124–1128. doi:10.1002/adfm.200305197
Nwodo, U., Green, E., and Okoh, A. (2012). Bacterial exopolysaccharides: Functionality and prospects. IJMS 13, 14002–14015. doi:10.3390/ijms131114002
Öner, E. T., Hernández, L., and Combie, J. (2016). Review of Levan polysaccharide: From a century of past experiences to future prospects. Biotechnol. Adv. 34, 827–844. doi:10.1016/j.biotechadv.2016.05.002
Osmałek, T., Froelich, A., and Tasarek, S. (2014). Application of gellan gum in pharmacy and medicine. Int. J. Pharm. 466, 328–340. doi:10.1016/j.ijpharm.2014.03.038
Park, H.-E., Park, N. H., Kim, M.-J., Lee, T. H., Lee, H. G., Yang, J.-Y., et al. (2003). Enzymatic synthesis of fructosyl oligosaccharides by levansucrase from Microbacterium laevaniformans ATCC 15953. Enzyme Microb. Technol. 32, 820–827. doi:10.1016/S0141-0229(03)00062-0
Parr, C. S., Wilson, N., Leary, P., Schulz, K., Lans, K., Walley, L., et al. (2014). The encyclopedia of life v2: Providing global access to knowledge about life on earth. BDJ 2, e1079. doi:10.3897/BDJ.2.e1079
Rairakhwada, D., Seo, J.-W., Seo, M., Kwon, O., Rhee, S.-K., and Kim, C. H. (2010). Gene cloning, characterization, and heterologous expression of levansucrase from Bacillus amyloliquefaciens. J. Ind. Microbiol. Biotechnol. 37, 195–204. doi:10.1007/s10295-009-0664-2
Rath, T., Rühmann, B., Schmid, J., and Sieber, V. (2022). Systematic optimization of exopolysaccharide production by Gluconacetobacter sp. and use of (crude) glycerol as carbon source. Carbohydr. Polym. 276, 118769. doi:10.1016/j.carbpol.2021.118769
Revin, V., Liyaskina, E., Nazarkina, M., Bogatyreva, A., and Shchankin, M. (2018). Cost-effective production of bacterial cellulose using acidic food industry by-products. Braz. J. Microbiol. 49, 151–159. doi:10.1016/j.bjm.2017.12.012
Römling, U., and Galperin, M. Y. (2015). Bacterial cellulose biosynthesis: Diversity of operons, subunits, products, and functions. Trends Microbiol. 23, 545–557. doi:10.1016/j.tim.2015.05.005
Saibuatong, O., and Phisalaphong, M. (2010). Novo aloe vera–bacterial cellulose composite film from biosynthesis. Carbohydr. Polym. 79, 455–460. doi:10.1016/j.carbpol.2009.08.039
Saleh, A. K., El-Gendi, H., Ray, J. B., and Taha, T. H. (2021). A low-cost effective media from starch kitchen waste for bacterial cellulose production and its application as simultaneous absorbance for methylene blue dye removal. Biomass Conv. bioref. doi:10.1007/s13399-021-01973-1
Schilling, C., Badri, A., Sieber, V., Koffas, M., and Schmid, J. (2020). Metabolic engineering for production of functional polysaccharides. Curr. Opin. Biotechnol. 66, 44–51. doi:10.1016/j.copbio.2020.06.010
Schmid, J. (2018). Recent insights in microbial exopolysaccharide biosynthesis and engineering strategies. Curr. Opin. Biotechnol. 53, 130–136. doi:10.1016/j.copbio.2018.01.005
Schmid, J., and Sieber, V. (2015). Enzymatic transformations involved in the biosynthesis of microbial exo-polysaccharides based on the assembly of repeat units. ChemBioChem 16, 1141–1147. doi:10.1002/cbic.201500035
Semjonovs, P., Shakirova, L., Treimane, R., Shvirksts, K., Auzina, L., Cleenwerck, I., et al. (2016). Production of extracellular fructans by Gluconobacter nephelii P1464. Lett. Appl. Microbiol. 62, 145–152. doi:10.1111/lam.12521
Shi, Z., Zhang, Y., Phillips, G. O., and Yang, G. (2014). Utilization of bacterial cellulose in food. Food Hydrocoll. 35, 539–545. doi:10.1016/j.foodhyd.2013.07.012
Shoda, M., and Sugano, Y. (2005). Recent advances in bacterial cellulose production. Biotechnol. Bioprocess Eng. 10, 1–8. doi:10.1007/BF02931175
Singhsa, P., Narain, R., and Manuspiya, H. (2018). Physical structure variations of bacterial cellulose produced by different Komagataeibacter xylinus strains and carbon sources in static and agitated conditions. Cellulose 25, 1571–1581. doi:10.1007/s10570-018-1699-1
Škraban, J., Cleenwerck, I., Vandamme, P., Fanedl, L., and Trček, J. (2018). Genome sequences and description of novel exopolysaccharides producing species Komagataeibacter pomaceti sp. nov. and reclassification of Komagataeibacter kombuchae (Dutta and Gachhui 2007). Syst. Appl. Microbiol. 41, 581–592. doi:10.1016/j.syapm.2018.08.006
Srikanth, R., Siddartha, G., Sundhar Reddy, C. H. S. S., Harish, B. S., Janaki Ramaiah, M., and Uppuluri, K. B. (2015). Antioxidant and anti-inflammatory levan produced from Acetobacter xylinum NCIM2526 and its statistical optimization. Carbohydr. Polym. 123, 8–16. doi:10.1016/j.carbpol.2014.12.079
Stephan, M. P., Oliveira, M., Teixeira, K. R. S., Martinez-Drets, G., and Döbereiner, J. (1991). Physiology and dinitrogen fixation of Acetobacter diazotrophicus. FEMS Microbiol. Lett. 77, 67–72. doi:10.1111/j.1574-6968.1991.tb04323.x
Stumpf, T. R., Yang, X., Zhang, J., and Cao, X. (2018). In situ and ex situ modifications of bacterial cellulose for applications in tissue engineering. Mater. Sci. Eng. C 82, 372–383. doi:10.1016/j.msec.2016.11.121
Suresh Kumar, A., Mody, K., and Jha, B. (2007). Bacterial exopolysaccharides – A perception. J. Basic Microbiol. 47, 103–117. doi:10.1002/jobm.200610203
Sutherland, I. W. (1990). Biotechnology of microbial exopolysaccharides. 1st ed. Cambridge: Cambridge University Press. doi:10.1017/CBO9780511525384
Sutherland, I. W. (1998). Novel and established applications of microbial polysaccharides. Trends Biotechnol. 16, 41–46. doi:10.1016/S0167-7799(97)01139-6
Tayama, K., Minakami, H., Entani, E., Fujiyama, S., and Masai, H. (1985). Structure of an acidic polysaccharide from acetobacter sp. NBI 1022. Agric. Biol. Chem. 49, 959–966. doi:10.1080/00021369.1985.10866836
Tayama, K., Minakami, H., Fujiyama, S., Masai, H., and Misaki, A. (1986). Structure of an acidic polysaccharide elaborated by acetobacter sp. NBI 1005. Agric. Biol. Chem. 50, 1271–1278. doi:10.1080/00021369.1986.10867547
Tian, F., Inthanavong, L., and Karboune, S. (2011). Purification and characterization of levansucrases from Bacillus amyloliquefaciens in intra- and extracellular forms useful for the synthesis of levan and fructooligosaccharides. Biosci. Biotechnol. Biochem. 75, 1929–1938. doi:10.1271/bbb.110315
Tyagi, N., and Suresh, S. (2016). Production of cellulose from sugarcane molasses using Gluconacetobacter intermedius SNT-1: Optimization & characterization. J. Clean. Prod. 112, 71–80. doi:10.1016/j.jclepro.2015.07.054
Ul-Islam, M., Khan, T., and Park, J. K. (2012). Water holding and release properties of bacterial cellulose obtained by in situ and ex situ modification. Carbohydr. Polym. 88, 596–603. doi:10.1016/j.carbpol.2012.01.006
Valepyn, E., Berezina, N., and Paquot, M. (2012). Optimization of production and preliminary characterization of new exopolysaccharides from Gluconacetobacter hansenii LMG1524. AiM 02, 488–496. doi:10.4236/aim.2012.24062
Vandamme, E. J., De Baets, S., Vanbaelen, A., Joris, K., and De Wulf, P. (1998). Improved production of bacterial cellulose and its application potential. Polym. Degrad. Stab. 59, 93–99. doi:10.1016/S0141-3910(97)00185-7
Vargas-García, M. C., López, M. J., Elorrieta, M. A., Suárez, F., and Moreno, J. (2001). Influence of nutritional and environmental factors on polysaccharide production by Azotobacter vinelandii cultured on 4-hydroxybenzoic acid. J. Ind. Microbiol. Biotech. 27, 5–10. doi:10.1038/sj.jim.7000152
Vazquez, A., Foresti, M. L., Cerrutti, P., and Galvagno, M. (2013). Bacterial cellulose from simple and low cost production media by Gluconacetobacter xylinus. J. Polym. Environ. 21, 545–554. doi:10.1007/s10924-012-0541-3
Velasco-Bedrán, H., and López-Isunza, F. (2007). The unified metabolism of Gluconacetobacter entanii in continuous and batch processes. Process Biochem. 42, 1180–1190. doi:10.1016/j.procbio.2007.05.017
Keywords: bacterial exopolysaccharides, Acetobacteraceae, acetan-like biopolymers, bacterial cellulose, levan, xanthan-like biopolymers
Citation: Wünsche J and Schmid J (2023) Acetobacteraceae as exopolysaccharide producers: Current state of knowledge and further perspectives. Front. Bioeng. Biotechnol. 11:1166618. doi: 10.3389/fbioe.2023.1166618
Received: 15 February 2023; Accepted: 15 March 2023;
Published: 30 March 2023.
Edited by:
Sang Yup Lee, Korea Advanced Institute of Science and Technology (KAIST), Republic of KoreaReviewed by:
Ahmed Saleh, National Research Centre, EgyptMarzena Jędrzejczak-Krzepkowska, Lodz University of Technology, Poland
Copyright © 2023 Wünsche and Schmid. This is an open-access article distributed under the terms of the Creative Commons Attribution License (CC BY). The use, distribution or reproduction in other forums is permitted, provided the original author(s) and the copyright owner(s) are credited and that the original publication in this journal is cited, in accordance with accepted academic practice. No use, distribution or reproduction is permitted which does not comply with these terms.
*Correspondence: Jochen Schmid, am9jaGVuLnNjaG1pZEB1bmktbXVlbnN0ZXIuZGU=
†ORCID ID: Jochen Schmid, orcid.org/0000-0003-2557-5532