In vitro and in silico methods for the biomechanical assessment of osseointegrated transfemoral prostheses: a systematic review
- Department of Industrial Engineering, School of Engineering and Architecture, Alma Mater Studiorum-University of Bologna, Bologna, Italy
The amputee population according to the World-Health-Organization is about 40 million. However, there is a high abandon rate of socket prostheses for the lower limb (25%–57%). The direct connection between the external prosthesis and the patient’s bone makes osseointegrated prostheses for transfemoral amputees advantageous (e.g., improvement of the motor control) compared to socket prostheses, which are currently the gold standard. However, similarly to other uncemented prostheses, the osseointegrated ones are at risk of aseptic loosening and adverse bone remodelling caused by stress-shielding. The preclinical assessment of these prostheses has already been evaluated using different methods which did not provide unanimous and comparable evidence. To compare data from different investigations, a clear and detailed overview of the methods used to assess the performance is necessary. In this review 17 studies investigating the primary stability, stress shielding and stress concentration of osseointegrated transfemoral prostheses are examined. Primary stability consists in the biomechanical stability upon implant insertion. Primary stability is assessed measuring extraction force (either with a pull-out or a push-out test) and micromotion at the interface between the implant and the host bone with LVDT (in vitro test) or numerical models. Stress-shielding causes adaptive changes in the bone density around metal implants, and thus in the bone strength and stiffness. Stress-shielding is assessed with strain gauges or numerical models measuring the load transfer and the strain distribution on the surface of the femur, and between the implant and the bone respectively. Stress concentration can lead to the formation of cracks inside the bone, resulting in fractures. The stress concentration is assessed measuring the load transfer and the strain energy density at the interface between the implant and the bone, using numerical models. As a result, a global view and consensus about the methods are missing from all these tests. Indeed, different setup and loading scenario were used in the in vitro test, while different model parameters (e.g., bone properties) were used in the numerical models. Once the preclinical assessment method is established, it would be important to define thresholds and acceptance criteria for each of the possible failure scenarios investigated.
1 Introduction
According to the World Health Organization, the global population of amputees is about 40 million (Marino et al., 2015; Windrich et al., 2016; Fanciullacci et al., 2021) reported that the lower limb amputation are about 36 million. In the European counties 15 to 30 every 100,000 subjects receive a lower limb amputation every year (Hughes et al., 2020). The leading causes of lower-limb amputation are wartime injury, traumatic events and atherosclerosis (Moxey et al., 2011; Newhall et al., 2016). Approximately 86% of patients with a transfemoral amputation are fitted with a socket prosthesis, which represents the current standard of care (Rommers et al., 1996). However, skin lesions occur in more than 50% of lower limb amputees, resulting in a negative impact on mobility, mechanical stability, and a high abandon rate of use of lower-limb prostheses (25%–57%) (Rommers et al., 1996; Hagberg and Brånemark, 2001; Paternò et al., 2018).
To overcome these problem, osseointegrated prostheses have been developed. In fact, osseointegrated prostheses provide a direct structural connection between the external prosthesis and the remaining living bone. Moreover, these prostheses show many advantages with respect to the socket prostheses, such as an improvement of the motor control, functional capacity and a better hip range of motion (ROM) (Thesleff et al., 2018). Osseointegrated transfemoral prostheses are mainly indicated for adults, generally under 65 years old, with a physically active lifestyle (typical of young patients). These subjects generally expect a significant improvement of the performance, for a long post-operative period. Hence the importance of improving the quality of life of these patients.
Currently commercially available osseointegrated transfemoral prostheses can be divided in two broad categories: the screw-fixation type and the press-fit type. Both types are designed to prevent infections and to achieve the mechanical stability of the implant. However, a correct osseointegration is a challenge, like in other osseointegrated prosthesis, and failure does occur due to septic or aseptic causes (Atallah et al., 2018). The main septic cause is the soft tissue infection at the stoma, and many of the associated consequences are reported in other reviews (Hebert, Rehani, and Stiegelmar 2017; Gerzina et al., 2020). In these reviews, the evolution, clinical outcomes, success rates and complications of different osseointegrated prostheses for amputees are reported, with a follow up greater than 12 months.
The main aseptic mechanical causes of failure are: post-operative periprosthetic fractures, loss of mechanical stability, and failure of the intramedullary stem or of the abutment. Post-operative periprosthetic fractures have an incidence between 3% and 10% (Brånemark et al., 2014; Juhnke et al., 2015; Al Muderis et al., 2016; Örgel et al., 2021). The loss of mechanical stability occur in between 3% and 29% (Roberts et al., 1991; Aschoff and Juhnke, 2016; Muderis et al., 2016; Kagan et al., 2017; Atallah et al., 2018; Hagberg et al., 2020). Other mechanical complications, such as mechanical failure of the intramedullary stem or of the abutment or of the dual cone, occur in between 5% and 30% of patients (Leijendekkers et al., 2017; Brånemark et al., 2019; Ranker et al., 2020; Reetz et al., 2020). Mechanical failures of the implantable components are more likely to occur in the long-term survival of the implant, especially among the more physically active patients (Hagberg et al., 2020; Hagberg et al., 2023).
The methods to investigate such failure scenarios are far from consolidated: quite different methods and different criteria and metrics are reported in the literature. For these reasons, results are difficult to compare or even conflicting. To enable comparing the results from the different investigations, a more systematic understanding of the test and simulation conditions would be important. This would help to improve the pre-clinical assessment of transfemoral osseointegrated prostheses.
Thus, the purpose of this systematic review is to summarize, evaluate and discuss the different methods used for the assessment of primary stability, stress shielding, and stress concentration of the transfemoral osseointegrated prostheses in order to find a systematic approach useful for all those who want to test these loading scenarios.
2 Methods
2.1 Search strategy
The research was performed using the databases: PubMed, ResearchGate, and Google Scholar, covering data before June 2022. PRISMA P guidelines (PRISMA-P, 2022) were followed to build this systematic review, focusing on the published studies of mechanical stability in osseointegrated prostheses for transfemoral amputations (Figure 1). The search strategy was as follow: (Osseointegrated prosthesis OR bone-anchored OR implant OR prosthesis) AND (lower limb OR transfemoral OR distal femur OR femur) AND (primary stability OR stress-shielding OR stress-concentration OR mechanical stability) AND (in vitro test OR mechanical test OR FE model OR numerical simulation). Results were limited to full-text articles in English. Articles were not restricted based on publication status or date.
2.2 Inclusion and exclusion criteria
To be included in the final review, the screened papers had to satisfy the following criteria:
• The first inclusion criterion was the presence of at least one of the search key words in the title and/or in the abstract. The second inclusion criterion was the use of in vitro mechanical test or FE models to evaluate the primary stability, the stress-shielding, or the stress concentration, and the failure mode of the osseointegrated transfemoral prostheses.
• The first exclusion criterion were studies about socket prosthesis, upper limb prosthesis and ankle-foot prosthesis. Second exclusion criterion were articles in which the analysis of the primary stability, the stress-shielding, or the stress concentration of the osseointegrated transfemoral prostheses was not reported. Moreover, clinical cohort study and case study were not reported in this review.
2.3 Screening methods/studies selection
The authors independently selected and reviewed the article title, key words and abstracts based on the inclusion and exclusion criteria. After the first screening (first exclusion criteria) the authors reviewed the full text articles. The articles that passed the first selection were then fully read. Any disagreements were solved between the reviewers and if something was unclear, the authors of the studies were contacted. The articles found in different databases were included only once. To further expand the research, citations of the papers and related articles were also tracked.
2.4 Logic flow of the systematic review
This systematic review aimed to summarize, evaluate, and discuss the different in vitro and in silico methods used for the biomechanical assessment of an osseointegrated transfemoral prosthesis (Figure 1). Thus, the next paragraphs are organized to present the methods related to the main three modes of biomechanical failure described in the Introduction: the lack of implant stability (Section 3.1), the strain/stress shielding (Section 3.2) and the strain/stress concentration (Section 3.3). Each of these topics will be analyzed and discussed in a similar by evaluating:
• The loading scenarios used to test each mode of failure, in terms of the loading conditions simulated either in the experimental test, or in the in silico model.
• Measured parameters and tools used either experimentally, or to implement the in silico models.
3 Results
A total of 630 articles were identified in the initial search process. Following the screening of the titles and abstract and after the exclusion of duplicates articles, 590 studies were excluded, and 40 studies remained for full-text evaluation (first exclusion criterion). After the full-text evaluation, 17 articles were included in this review (second exclusion criterion). The PRISMA flowchart for the study is reported in Figure 2. A recent review (Thesleff et al., 2018) covered specifically the constructive details of the existing osseointegrated prostheses. That review provided an overview of the devices currently available on the market in terms of different materials, coatings, modularity. Therefore, constructive details are not further discussed in the present review.
The papers included in this review use different terms to indicate the osseointegrated prosthesis. This review uses standardized nomenclature for comparison of data from different works. In particular:
• Osseointegrated limb prosthesis, percutaneous implant, intraosseous transcutaneous amputation prosthesis, bone anchored prostheses in this review, for consistency, will always be called “osseointegrated lower-limb prosthesis.”
• Osseointegrated prostheses for transfemoral amputees in this review will always be called “Transfemoral Osseointegrated Implant (TOI).”
3.1 Methods for the evaluation of the primary stability
Primary stability is defined as the biomechanical stability upon implant insertion, and it is crucial to achieve the long-term stability. Primary stability is mainly achieved when interface micromotion does not exceed the threshold for granting bone ingrowth and osseointegration. There is a general consensus about a range of micromotions that can be tolerated: between 20 and 150 μm (Pilliar, Lee, and Maniatopoulos, 1986; Kohli, Stoddart, and van Arkel, 2021). In fact, an excessive interface micromotion can interfere with the process of osseointegration, leading to formation of a fibrous tissue layer at the implant-bone interface. Thus, this negative self-sustained loop can lead to aseptic loosening (i.e., loss of primary stability of the implant within the bone, with no sign of sepsis) (Cameron, Pilliar, and Macnab, 1973; Pilliar, Lee, and Maniatopoulos, 1986; Fukuoka, Yoshida, and Yamano, 2000; Duyck et al., 2006; Winter, Klein, and Karl, 2012). Since micromotion can interfere with the process of osseointegration (Winter, Klein, and Karl, 2012), the mechanical test evaluating micromotion is crucial to assess the osseointegration process. Micromotion can be classified as permanent migrations and inducible micromotions:
• Permanent migrations are defined as the non-reversible implant motion that is accumulated cycle after cycle, that remains even after removing the load. Permanent migrations do not necessarily prevent implant stability, in case they settle over time. The main shortcoming of permanent migrations (if not accompanied by excessive inducible micromotions, see below) is that they may result in a final implant position different from the intended one.
• Inducible (or elastic) micromotions are defined as a temporary relative motion between the prosthesis and the host bone that is reversing after removing the load (Cristofolini et al., 2003). Excessive inducible micromotions can cause pain, mechanical instability and fibrous tissue formations.
Primary stability can be assessed with different methods, such as measuring the extraction force (pull-out or push-out tests) or the micromotions at the interface between the implant and the host bone. Since a standard procedure to test and assess the primary stability of osseointegrated prostheses does not exist, different methods were developed to measure the pull-out or push-out load and the micromotion.
Experimental in vitro pull-out and push-out tests were performed at the time of in vitro implantation (Welke et al., 2013; Jeyapalina et al., 2014; Barnes et al., 2019) and after 3-6-9-12 months ex vivo on sheep (Jeyapalina et al., 2014). Micromotions were investigated both in vitro at the time of implantation (Barnes et al., 2019), and through in silico simulated rehabilitation exercises (Prochor and Anna Mierzejewska, 2019).
3.1.1 Loading scenarios
The pull-out and push-out tests allow to assess how strong and well anchored the implant is under extremely simplified loading conditions. Pull-out tests were performed by applying a tensile force to the prostheses until the extraction of the prosthesis or the failure of the bone (A generic experimental setup is represented in Figure 3) (Welke et al., 2013; Jeyapalina et al., 2014). The proximal femur was embedded and clamped (ensuring axial alignment) (Welke et al., 2013; Jeyapalina et al., 2014). To grip the prosthesis a hole was drilled in the distal part of the prosthesis and a stainless-steel rod was placed through hole and connected to the actuator (Jeyapalina et al., 2014) or to a universal-joint (Welke et al., 2013). Also push-out tests can be used, in case of very short stems. To push-out the prosthesis a rod was attached to the actuator and pushed the implant out of the canal of a short segment resected from the femur (Barnes et al., 2019). Both in the pull-out and push-out tests, the specimens were loaded in displacement control with a constant rate of a range between 5 mm/min and 100 mm/min (Welke et al., 2013; Jeyapalina et al., 2014; Barnes et al., 2019) until ultimate failure (loads and methods are summarized in Table 1).
Measuring permanent migrations and inducible micromotions is useful to assess whether the prosthesis has mobilized during the movement and involves more complex experimental setups or numerical simulation. Prochor et al. simulated with a numerical model a static load bearing exercise (Prochor and Anna Mierzejewska, 2019). A static axial force of 1000 N was applied simulating a patient with a body weight (BW) of approximately 100 kg (Prochor and Anna Mierzejewska, 2019). A more complex experimental loading scenario has been designed by (Barnes et al., 2019) to measure inducible micromotions of the prostheses. Specimens were tilted and aligned following a modified version of the international society of Biomechanics (Wu et al., 2002). Thanks to that, the specimens were subjected to a combination of compression and bending moment, simulating physiological conditions (a generic experimental setup is represented in Figure 4). An increasing cyclic load until 1.4 of the body weight (BW) at 1 Hz was applied to measure the inducible micromotion of the prosthesis from the last 90 cycles (Barnes et al., 2019). Loads and methods are reported in Table 1.
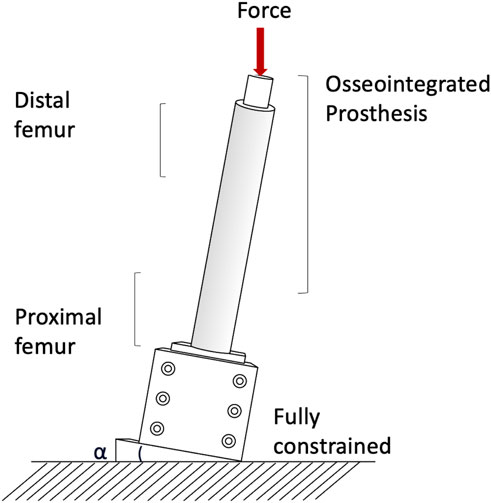
FIGURE 4. Generic setup of the experimental mechanical test used to assess the stress shielding and stress concentration.
3.1.2 Tools
Both the pull-out and push-out extraction force was measured with a uniaxial material testing system (Jeyapalina et al., 2014; Barnes et al., 2019). The applied force was measured directly by the load cell of the testing machine. In some case, the interface shear strength was computed by dividing the applied force by the interface surface area (Barnes et al., 2019).
The in silico model was based on a human femur CT scan. The friction coefficient at the interface between the simulated implant and the bone must reflect the immediate post-operative conditions. A coefficient of friction of 0.4 was considered by (Prochor and Anna Mierzejewska, 2019). Moreover, to simulate continuous bone remodeling around the implant, a suitable Fortran subroutine was used during the calculation process in which, for each calculation step, the density and Young’s modulus of bone tissues were updated in each finite element. The initial values of bone Young’s modulus of cortical bone and trabecular bone were respectively 20 GPa and 0.96 GPa. Poisson’s coefficient was assumed to be constant for both bone tissues throughout the simulation. The threshold values for bone density were 𝜌max = 2.000 g/cm3 and 𝜌min = 0.200 g/cm3 (Prochor and Anna Mierzejewska, 2019).
In the in vitro experimental tests, the inducible micromotion of the implant in the host bone was measured with linear variable differential transformers (LVDT) (Barnes et al., 2019). A combination of three LVDTs and a ring was used to measure micromotions in the axial direction and in bending.
3.2 Methods for the evaluation of the strain-stress shielding
Stress shielding is a phenomenon that causes adaptive changes in bone morphology, and in bone tissue strength and stiffness in response to the altered mechanical environment caused by the presence of metal implants. Adverse bone remodelling leads to a lack of bone stock in case of surgery revision, weakens the bone, and contributes to implant loosening (Kagan et al., 2017; L Cristofolini et al., 2009). To the authors’ knowledge, only few in vitro studies were performed to evaluate the load distribution and the subsequent strain shielding of an osseointegrated transfemoral prosthesis (Cristofolini et al., 1998; Tomaszewski et al., 2013; Ahmed et al., 2020). In fact, the stress and strain of the bone surrounding the implant are difficult to measure in vitro and nearly impossible to measure in vivo. Therefore, in silico finite element models can advantageously be used to generate these information (Xu et al., 2006; Tomaszewski et al., 2010; Tomaszewski et al., 2012a; Tomaszewski, et al., 2012b; Newcombe et al., 2013; Tomaszewski et al., 2013; Stenlund et al., 2017; Prochor and Sajewicz, 2018; Prochor and Anna Mierzejewska, 2019).
3.2.1 Loading scenarios
Both in vitro and in silico studies simulated specific loading cases from a normal walking cycle: at the heel strike (25%) (Cristofolini et al., 1998; Xu et al., 2006; Tomaszewski et al., 2013; 2010; Tomaszewski et al., 2012a; Tomaszewski et al., 2012b; Newcombe et al., 2013; Prochor and Sajewicz, 2018; Prochor and Sajewicz, 2019; Prochor and Anna Mierzejewska, 2019) and toe-off (55%) (Tomaszewski et al., 2013; 2010; Tomaszewski et al., 2012a; Tomaszewski et al., 2012b; Newcombe et al., 2013; Prochor and Sajewicz, 2018; Prochor and Sajewicz, 2019; Prochor and Anna Mierzejewska, 2019). Tomaszewski et al. also considered a one leg stance (Tomaszewski et al., 2013) and a forward fall loading condition (Tomaszewski et al., 2012a).
Both in the in vitro and in the in silico simulations, one end of the femur (usually the proximal one, away from the prosthetic tip to avoid artifacts in the peri-prosthetic region due to the boundary conditions) was fully constrained, and loads were applied to the other end (usually the distal one, through the implanted prosthesis) (A generic experimental setup is represented in Figure 4).
In the in vitro experiment, two configurations were tested: axial compression (Cristofolini et al., 1998; Tomaszewski et al., 2013), and a medio-lateral bending moment (in this case, the femur was tilted and aligned in order to apply a combination of compression and bending moment, simulating physiological conditions) (Cristofolini et al., 1998; Tomaszewski et al., 2013; Ahmed et al., 2020) (loads and methods are summarized in Table 2).
In all the in silico simulations different loads taken from the experimental measurements (Lee et al., 2008a; Stenlund et al., 2017) and from Orthoload were applied at the end of the femur (Xu et al., 2006; Tomaszewski et al., 2010; Tomaszewski et al. 2012a; Tomaszewski et al. 2012b; Newcombe et al., 2013; Stenlund et al., 2017; Prochor and Sajewicz, 2018; Prochor and Anna Mierzejewska, 2019; Ahmed et al., 2020) (loads and methods are summarized in Table 2).
3.2.2 Tools
In the in vitro experiments, both composite femur (Cristofolini et al., 1998) and human cadaveric ones (Tomaszewski et al., 2013; Ahmed et al., 2020) were used. Strain gauges were used to measure the strain magnitude at selected locations on the femur surface, before (Cristofolini et al., 1998) and after implantation (Cristofolini et al., 1998; Tomaszewski et al., 2013; Ahmed et al., 2020).
In the in silico simulations (Tomaszewski et al., 2010; Tomaszewski et al. 2012a; Tomaszewski et al. 2012b; Prochor and Anna Mierzejewska, 2019), derived the Young’s moduli of the bone elements from ash densities, while (Xu et al., 2006; Newcombe et al., 2013; Stenlund et al., 2017; Prochor and Sajewicz, 2018; Prochor and Anna Mierzejewska, 2019; Ahmed et al., 2020) considered the Young’s moduli of the bone elements homogeneous. In some models, to reflect the immediate post-operative condition, an interface contact with a coefficient of friction between 0.2 and 0.4 were used (Tomaszewski et al., 2010; Stenlund et al., 2017; Prochor and Sajewicz, 2019; Prochor and Anna Mierzejewska, 2019; Ahmed et al., 2020). In other cases, a fully bonded interface was simulated to represent ideal interface bonding (Xu et al., 2006; Tomaszewski et al., 2012a; Tomaszewski et al., 2012b; Newcombe et al., 2013; Newcombe et al., 2013; Stenlund et al., 2017; Prochor and Sajewicz, 2018) assessed the load distribution in a specific load condition. Instead, to assess the consequences of strain-stress shielding, the strain energy density threshold was used as the stimulus for adaptive bone remodeling (Xu et al., 2006; Tomaszewski et al., 2010; Tomaszewski et al. 2012a; Tomaszewski et al. 2012b; Prochor and Sajewicz, 2019; Ahmed et al., 2020).
3.3 Methods for the evaluation of the stress concentration and fracture
Post-operative periprosthetic fractures are most often caused by stress concentrations and occur when the patient abruptly changes the walking speed during gait (Juhnke et al., 2015; Al Muderis et al., 2016; Örgel et al., 2021; R; Brånemark et al., 2014). For this reason, the stress concentration tests focused on the ultimate load of the implanted femur (Welke et al., 2013) and on the stress concentration around the implant during daily activities (Ming, Xiang, and Yubo, 2005; Xu et al., 2006; Lee, et al., 2008b; Helgason et al., 2009; Tomaszewski et al., 2013; 2010; Tomaszewski et al., 2012b; Newcombe et al., 2013).
3.3.1 Loading scenarios
The risk of failure of the osseointegrated lower limb prostheses was evaluated when the prosthesis was subject to a pure bending moment, focusing on the most critical load under simplified loading scenario (Welke et al., 2013). The specimens were mounted in a custom four-point bending device on the material testing machine, applying a bending moment in anterior-posterior direction until failure (Welke et al., 2013). Tomaszewski et al. (2013) simulated specific loading cases from a normal walking cycle: at the heel strike (25%), at the toe-off (55%), and a one leg stance. One end of the specimens was fully constrained, and loads were applied to the other end. In order to simulate physiological conditions, the femur was tilted and aligned (Figure 4). Thanks to that, the specimen was subjected to a combination of compression and bending moment (Tomaszewski et al., 2013).
An in silico model was developed to simulate the maximum loads experienced during normal daily activities (Newcombe et al., 2013). The loading conditions (bending moment, axial force and axial torque) were applied individually as three different loading conditions, in order to investigate the effects of each component of load separately (Newcombe et al., 2013). A more complex loading scenarios have been designed, to simulate selected loading cases from a normal walking cycle at the heel strike (25%) (Xu et al., 2006; Helgason et al., 2009; Tomaszewski et al., 2010; Tomaszewski et al., 2012a) and the toe-off (55%) (Lee, et al., 2008b; Tomaszewski et al., 2010; Tomaszewski et al., 2012b), and forward fall loading (Tomaszewski et al., 2012a). The load values are summarized in Table 3.
3.3.2 Tools
In the in vitro experiments, both composite femur (Welke et al., 2013) and human cadaveric ones (Tomaszewski et al., 2013) were used. In the four-point-bending experiment, the force, the displacement, and the deflection in the mid-diaphysis were measured with a hydraulic material testing system and LVDT. This allowed estimating the stiffness, and the maximum moment at failure (Welke et al., 2013). Strain gauges were used to measure the strain magnitude at selected locations on the femur surface (Tomaszewski et al., 2013).
In the in silico simulations, Tomaszewski et al. derived the Young’s moduli of the bone elements from ash densities (Tomaszewski et al., 2010; Tomaszewski et al., 2012b), while (Ming, Xiang, and Yubo, 2005; Xu et al., 2006; Lee, et al., 2008b; Helgason et al., 2009; Newcombe et al., 2013) considered the Young’s moduli of the bone elements homogeneous (Ming, Xiang, and Yubo, 2005; Tomaszewski et al., 2010). used a coefficient of friction in a range between 0.2 and 2.0, which models the direct post-operative case, while in other cases, a fully bonded interface was simulated (Xu et al., 2006; Lee, et al., 2008b; Helgason et al., 2009; Tomaszewski et al., 2012a; Newcombe et al., 2013). Periprosthetic bone failure risk was evaluated based on the von Mises stress criterion in all the in silico simulations.
4 Discussion
Transfemoral osseointegrated prostheses might be an alternative to the socket prostheses, which represent the current standard-of-care. In fact, cost-benefit analyses reported that osseointegrated prostheses provide a better quality of life (in terms of physical functioning and bodily pain) at similar costs when compared to the socket prostheses (Haggstrom, Hansson, and Hagberg, 2013; Frossard et al., 2017; Black et al., 2022). However, like other uncemented prostheses, post-operative complications may occur due to lack of primary stability, stress-shielding and stress-concentration. The investigation of these failure scenarios is necessary for the preclinical assessment of transfemoral osseointegrated prostheses. However, the methods to investigate such failure scenarios, are far from consolidated: quite different methods and different criteria and metrics are reported in the literature. For these reasons, results are difficult to compare or even conflicting. Thus, the aim of this review was to summarize and compare the different methods used to assess the primary stability, stress shielding, and stress concentration of the osseointegrated lower limb prostheses.
4.1 Primary stability
The primary stability of the implant has been evaluated in vitro either measuring the extraction force, or the micromotions at the interface between the implant and the host bone. The extraction force was evaluated in the literature with two different methods: either with a pull-out or a push-out test. The pull-out test allows to evaluate the extraction force preserving the natural anatomy of the implanted femur (Welke et al., 2013; Jeyapalina et al., 2014). Conversely, the push-out test is usually performed on short segments resected from the femur, and is suitable if a short implant must be tested (Barnes et al., 2019). The push-out test allows to evaluate the extraction force of different stems on the same specimen in different osteotomy levels. However, the pull-out and push-out tests, which are used when a fast and reproducible test is needed, do not represent a common failure scenario for osseointegrated prostheses. In fact, the load is applied with a monotonic axial ramp until the exit of the prosthesis or the fracture of the specimen. However, the pull-out test is often used in other prosthetic devices as an FDA test (U.S. Department of Health and Human Services et al., 2003).
As aseptic loosening of osseointegrated prostheses is mainly driven by excessive micromotions under cyclic loading, a more representative pre-clinical assessment should quantify permanent migrations and/or inducible micromotions. The in vitro evaluation of the micromotion of the prosthesis was performed only by Barnes et al. using displacement transducers (LVDT) (Barnes et al., 2019). Nevertheless, a single LVDT allows to measure the displacement only in one direction. This limitation can be partially overcome, using multiple LVDTs to detect three-dimensional motions; however also in this case measurement errors may arise due to misalignment of the transducers. A further improvement is provided by DIC, which allows a full-field three-dimensional displacement analysis. In the literature it is possible to find similar studies with DIC on different devices (Morosato et al., 2020).
The micromotion can also be investigated with numerical models. In fact, once an in silico model has been developed and validated, it can be advantageously used to simulate and evaluate several complex loading scenarios and motor tasks, to compare different conditions such as different degrees of osseointegration, or modified bone quality (Martelli et al., 2011). In fact, numerical models give the opportunity to simulate experimental tests and repeat the same experiment a limitless number of times on the same models for an almost infinite variation of parameters (Romero et al., 2007; Martelli et al., 2011). Prochor et al. exploited the advantages of numerical models to explore the effect of different parameters such as implant length, implant diameter and interface bonding on the micromotions in a simplified load condition (Prochor and Anna Mierzejewska, 2019). A very important caveat is that numerical models should not be trusted if they are not thoroughly verified and validated, especially when making clinically relevant decisions (Cristofolini et al., 2010; ASME V&V 40, 2018; Oefner et al., 2021).
4.2 Strain-stress shielding
As bone remodelling is driven by frequently recurring cyclic loads, a commonly used method to evaluate the strain-stress shielding is simulating daily motor task. In vitro methodologies allow to apply controlled loading components. The strain on the surface of the specimens were evaluated using strain gauges, obtaining precise and accurate measurements on selected points (Cristofolini et al., 1998; Tomaszewski et al., 2013; Ahmed et al., 2020). DIC provides a full field strain analysis on the surface of the specimens and has already been used in literature (Palanca, Tozzi, and Cristofolini, 2016; Palanca et al., 2018).
However, in vitro mechanical tests allow to simulate only a limited set of loading conditions, while in silico methodologies allow to simulate different motor task and to investigate stress and strain at the interface between the prosthesis and the bone (Cristofolini et al., 2010). Moreover, the osseointegration between the prosthesis and the bone cannot be simulated in vitro, while with in silico models the different degree of osseointegration can be chosen, from unbonded with a given coefficient of friction (Tomaszewski et al., 2010; Prochor and Anna Mierzejewska, 2019; Ahmed et al., 2020) to fully bonded with a perfect osseointegration (Xu et al., 2006; Tomaszewski et al., 2012a; Tomaszewski et al., 2012b; Newcombe et al., 2013; Prochor and Sajewicz, 2018). All the numerical studies of this review are similar for what concerns the loading scenarios, while the quality of the bone tissue was represented with different degrees of detail. Indeed, some studies considered bone as isotropic (Tomaszewski et al., 2012a; Tomaszewski et al., 2012b; Prochor and Sajewicz, 2018; Prochor and Anna Mierzejewska, 2019), others anisotropic (Xu et al., 2006; Newcombe et al., 2013; Stenlund et al., 2017; Ahmed et al., 2020). In addition, some models considered the elastic modulus of the bone homogeneous (Xu et al., 2006; Newcombe et al., 2013; Stenlund et al., 2017; Prochor and Sajewicz, 2018; Prochor and Anna Mierzejewska, 2019; Ahmed et al., 2020), while others adapted the elastic modulus based on bone density (Tomaszewski et al., 2010; Tomaszewski et al. 2012a; Tomaszewski et al. 2012b). In a specific case, the bone was idealized with a cylindrical geometry (Stenlund et al., 2017) and with a generic geometry from standardized femur data (Prochor and Sajewicz, 2018), while in other cases the in silico model of the bone was based on computed tomography (CT) data of a femoral bone (Xu et al., 2006; Tomaszewski et al., 2010; Tomaszewski et al. 2012a; Tomaszewski et al. 2012b; Newcombe et al., 2013; Prochor and Anna Mierzejewska, 2019). However, bone density of some models was re-scaled to the typical young amputated patient since the CT images were of a cadaver old subject (Tomaszewski et al., 2010; Tomaszewski et al. 2012a; Tomaszewski et al. 2012b). Although different modelling strategies can be observed, comparable findings were obtained from different studies. This shows that the macro effects were captured by the models.
A limitation of these numerical studies is that in most cases the in silico models have not been experimentally validated. Indeed, Ahmed et al. and Tomaszewski et al. are among the few who used a validated model (Tomaszewski et al., 2013; Ahmed et al., 2020).
4.3 Strain-stress concentration
Evaluation of possible stress concentrations is crucial, as they can lead to bone fracture and subsequent implant failure. A commonly used method to evaluate the strain-stress concentration is simulating physiological loading, and the typical falling scenarios. Welke et al. (2013) aimed to investigate the ultimate load and the fracture modes with a simplified in vitro test. Typically, bending moment is the most critical loading scenario during normal daily activities, and a frequent cause for bone fractures. Thus, Welke et al. (2013) simulated the most critical condition by analyzing a highly repeatable loading scenario, i.e., the four-point-bending test. It must be noted that the four-point-bending test does not correspond to any realistic scenarios during the physiological conditions or falling. However, this testing condition may provide a first estimate of the load that can lead to a stress concentration and resulting in a bone fracture.
Subsequent studies evaluated the stress concentration around the implant using numerical simulations (Ming, Xiang, and Yubo, 2005; Xu et al., 2006; Lee, et al., 2008b; Helgason et al., 2009; Tomaszewski et al., 2010; Tomaszewski et al., 2012b; Newcombe et al., 2013). All the numerical models focusing on stress concentration are similar in loading strategies, while the quality of the bone tissue was represented with different degrees of detail. Indeed, some studies considered bone as isotropic (Helgason et al., 2009; Tomaszewski et al., 2010; Tomaszewski et al., 2012a), others anisotropic (Ming, Xiang, and Yubo, 2005; Xu et al., 2006; Lee, et al., 2008b; Newcombe et al., 2013). Some models applied homogeneous properties (Ming, Xiang, and Yubo, 2005; Xu et al., 2006; Lee, et al., 2008b; Helgason et al., 2009), while others modified the elastic modulus based on bone density (Tomaszewski et al., 2010; Tomaszewski et al., 2012b). In a specific case, the bone was idealized with a generic standardized geometry (Ming, Xiang, and Yubo, 2005), while in other cases the FE geometry of the bone was based on computed tomography (CT) scan of a femoral cadaveric bone (Xu et al., 2006; Helgason et al., 2009; Tomaszewski et al., 2010; Tomaszewski et al. 2012a; Tomaszewski et al. 2012b; Newcombe et al., 2013) or a femoral composite bone (Lee, et al., 2008b). However, as these models were based on a CT scan of an old subject, the bone density was re-scaled to the density that can be expected in amputees (typically young patients). Despite these different modelling strategies, comparable findings were obtained from the different studies showing that the macro effects were captured by the models.
In silico models estimating the risk of periprosthetic bone fractures mostly rely on the Von Mises stress criterion. The Von Mises criterion combines stress components and is applicable to ductile materials. Recent advances demonstrated that criteria based on the maximum principal strain are more accurate in predicting bone failure (Schileo et al., 2008; Miles et al., 2015).
Another limitation of many of these numerical studies is that in silico models have not been experimentally validated. To the authors’ knowledge, only Tomaszewski et al. reported a validated model (Tomaszewski et al., 2010; Tomaszewski et al., 2012a).
5 Conclusion
This systematic review highlighted and critically evaluated the main methods used to assess the primary stability, the stress-shielding and stress concentration of osseointegrated transfemoral prostheses, both through in vitro tests and in silico models. In some cases, results are difficult to compare as different methods were used to investigate the prostheses performance. It would be important to reach a consensus about the loading scenarios to be simulated, to enable comparison between studies. Furthermore, the use of a more versatile measurement systems, such as DIC, could be useful to develop a comprehensive method for in vitro testing the primary stability, the stress-shielding and stress concentration of an osseointegrated transfemoral prosthesis. The in silico models are generally more consistent in terms of loading scenarios, whereas different strategies are adopted to model the bone itself. More efforts should be dedicated to developing robustly validated in silico models. Moreover, in vitro and in silico methods can be synergistic, in order to provide more detailed and more reliable results than can be achieved with either approach singularly.
Author contributions
GG: conceptualization, methodology, investigation, resources, data curation, writing—original draft. LC: conceptualization, methodology, writing—review and editing, project administration, funding acquisition, supervision. All authors contributed to the article and approved the submitted version.
Funding
This study was partly funded by INAIL (PR19-CR-P5- OsteoCustom, E59C20000730005).
Acknowledgments
The Authors wish to thank MEng, Emanuele Gruppioni, PhD, Kavin Morellato, MEng, Valentina Betti, MEng, Stefano Zaffagnini, MD, and Domenico Alesi, MD, for the stimulating discussions on the topic. Giulia Cavazzoni, MEng, is gratefully acknowledged for her help to improve the manuscript. Marco Palanca, PhD, is gratefully acknowledged for his inputs in the organization of the review and for the valuable suggestions.
Conflict of interest
The authors declare that the research was conducted in the absence of any commercial or financial relationships that could be construed as a potential conflict of interest.
Publisher’s note
All claims expressed in this article are solely those of the authors and do not necessarily represent those of their affiliated organizations, or those of the publisher, the editors and the reviewers. Any product that may be evaluated in this article, or claim that may be made by its manufacturer, is not guaranteed or endorsed by the publisher.
References
Ahmed, K., Greene, R. J., Aston, W., Briggs, T., Pendegrass, C., Moazen, M., et al. (2020). Experimental validation of an ITAP numerical model and the effect of implant stem stiffness on bone strain energy. Ann. Biomed. Eng. 48 (4), 1382–1395. doi:10.1007/s10439-020-02456-6
Al Muderis, Munjed, Aditya, Khemka, Lord, Sarah J., de Meent, Henk Van, and Frölke, Jan Paul M. (2016). Safety of osseointegrated implants for transfemoral amputees: A two-center prospective cohort study. J. Bone Jt. Surg. 98 (11), 900–909. doi:10.2106/JBJS.15.00808
Aschoff, H.-H., and Juhnke, D.-L. (2016). Endo-exo prostheses: osseointegrated percutaneously channeled implants for rehabilitation after limb amputation. Der Unfallchirurg 119 (5), 421–427. doi:10.1007/s00113-016-0175-3
ASME V&V 40 (2018). Assessing credibility of computational modeling through verification and validation: Application to medical devices. V V 40- edition. New York, NY, USA: American Society of Mechanical Engineers ASME.
Atallah, Robin, Leijendekkers, Ruud A., Hoogeboom, Thomas J., and Paul Frölke, Jan (2018). Complications of bone-anchored prostheses for individuals with an extremity amputation: A systematic review. PLOS ONE 13 (8), e0201821. doi:10.1371/journal.pone.0201821
Barnes, Spencer C., Clasper, Jon C., Bull, Anthony M. J., and JonathanJeffers, R. T. (2019). Micromotion and push-out evaluation of an additive manufactured implant for above-the-knee amputees. J. Orthop. Res. 37 (10), 2104–2111. doi:10.1002/jor.24389
Black, Grant G., Jung, Wooram, Wu, Xian, Rozbruch, S. Robert, and Otterburn, David M. (2022). A cost-benefit analysis of osseointegrated prostheses for lower limb amputees in the US Health care system. Ann. Plastic Surg. 88 (3), S224–S228. doi:10.1097/SAP.0000000000003183
Brånemark, R., Berlin, Ö., Hagberg, K., Bergh, P., Gunterberg, B., and Rydevik, B. (2014). A novel osseointegrated percutaneous prosthetic system for the treatment of patients with transfemoral amputation: A prospective study of 51 patients. Bone & Jt. J. 96-B (1), 106–113. doi:10.1302/0301-620X.96B1.31905
Brånemark, Rickard P., Hagberg, Kerstin, Kulbacka-Ortiz, Katarzyna, Berlin, Örjan, and Rydevik, Björn (2019). Osseointegrated percutaneous prosthetic system for the treatment of patients with transfemoral amputation: A prospective five-year follow-up of patient-reported outcomes and complications. J. Am. Acad. Orthop. Surg. 27 (16), e743–e751. doi:10.5435/JAAOS-D-17-00621
Cameron, Hugh U., Pilliar, Robert M., and Macnab., Ian (1973). The effect of movement on the bonding of porous metal to bone. J. Biomed. Mater. Res. 7 (4), 301–311. doi:10.1002/jbm.820070404
Cristofolini, L., Juszczyk, M., Taddei, F., Field, R. E., Rushton, N., and Viceconti, M. (2009). Stress shielding and stress concentration of contemporary epiphyseal hip prostheses. Proc. Institution Mech. Eng. Part H J. Eng. Med. 223 (1), 27–44. doi:10.1243/09544119JEIM470
Cristofolini, Luca, Bini, Stefano, and Toni, Aldo (1998). In vitro testing of a novel limb salvage prosthesis for the distal femur. Clin. Biomech. 13 (8), 608–615. doi:10.1016/S0268-0033(98)00024-2
Cristofolini, Luca, Monti, Luisa, Angelo, Cappello, and Toni, AldoAmelia Saponara Teutonico (2003). Comparative in vitro study on the long term performance of cemented hip stems: validation of a protocol to discriminate between ‘good’ and ‘bad’ designs. J. Biomechanics 36 (11), 1603–1615. doi:10.1016/S0021-9290(03)00191-X
Cristofolini, Luca, Schileo, Enrico, Juszczyk, Mateusz, Taddei, Fulvia, Martelli, Saulo, and Viceconti, Marco (2010). Mechanical testing of bones: the positive synergy of finite–element models and in vitro experiments. Philosophical Trans. R. Soc. A Math. Phys. Eng. Sci. 368, 2725–2763. doi:10.1098/rsta.2010.0046
Duyck, J., Vandamme, K., Geris, L., Van Oosterwyck, H., De Cooman, M., Vandersloten, J., et al. (2006). The influence of micro-motion on the tissue differentiation around immediately loaded cylindrical turned titanium implants. Archives Oral Biol. 51 (1), 1–9. doi:10.1016/j.archoralbio.2005.04.003
Fanciullacci, Chiara, McKinney, Zach, Monaco, Vito, Milandri, Giovanni, Angelo, Davalli, Sacchetti, Rinaldo, et al. (2021). Survey of transfemoral amputee experience and priorities for the user-centered design of powered robotic transfemoral prostheses. J. NeuroEngineering Rehabilitation 18, 168. doi:10.1186/s12984-021-00944-x
Frossard, , Laurent, Alain, Merlo, Gregory, Burkett, Brendan, Quincey, Tanya, and Berg, Debra (2017). Cost-effectiveness of bone-anchored prostheses using osseointegrated fixation: myth or reality? Prosthetics Orthot. Int. 42, 318–327. doi:10.1177/0309364617740239
Fukuoka, S., Yoshida, K., and Yamano, Y. (2000). Estimation of the migration of tibial components in total knee arthroplasty. J. Bone Jt. Surg. Br. 82-B (2), 222–227. doi:10.1302/0301-620X.82B2.0820222
Gerzina, Christopher, Potter, Eric, Haleem, Amgad M., and Dabash, Sherif (2020). The future of the amputees with osseointegration: A systematic review of literature. J. Clin. Orthop. Trauma 11, S142–S148. doi:10.1016/j.jcot.2019.05.025
Hagberg, K., and Brånemark, R. (2001). Consequences of non-vascular trans-femoral amputation: a survey of quality of life, prosthetic use and problems. Prosthet. Orthot. Int. 25, 186–194. doi:10.1080/03093640108726601
Hagberg, Kerstin, Kulbacka-Ortiz, Katarzyna, Thomsen, Peter, Malchau, Henrik, and Reinholdt, CarinaShadi-Afarin Ghassemi Jahani (2020). A 15-year follow-up of transfemoral amputees with bone-anchored transcutaneous prostheses: mechanical complications and patient-reported outcomes. Bone & Jt. J. 102-B (1), 55–63. doi:10.1302/0301-620X.102B1.BJJ-2019-0611.R1
Hagberg, Kerstin, Omar, Omar, and Thomsen, PeterShadi Afarin Ghasemi Jahani (2023). Osseointegrated prostheses for the rehabilitation of patients with transfemoral amputations: A prospective ten-year cohort study of patient-reported outcomes and complications. J. Orthop. Transl. 38, 56–64. doi:10.1016/j.jot.2022.09.004
Haggstrom, Eva E., Hansson, Elisabeth, and Hagberg, Kerstin (2013). Comparison of prosthetic costs and service between osseointegrated and conventional suspended transfemoral prostheses. Prosthetics Orthot. Int. 37 (2), 152–160. doi:10.1177/0309364612454160
Hebert, Jacqueline S., Rehani, Mayank, and Robert, Stiegelmar (2017). Osseointegration for lower-limb amputation: A systematic review of clinical outcomes. JBJS Rev. 5 (10), e10. doi:10.2106/JBJS.RVW.17.00037
Helgason, Benedikt, Pálsson, Halldór, Philip Rúnarsson, Tómas, Frossard, Laurent, and Viceconti, Marco (2009). Risk of failure during gait for direct skeletal attachment of a femoral prosthesis: A finite element study. Med. Eng. Phys. 31 (5), 595–600. doi:10.1016/j.medengphy.2008.11.015
Hughes, Will, Goodall, Richard, Salciccioli, Justin D., Marshall, Dominic C., Davies, Alun H., and Joseph, Shalhoub (2020). Editor’s choice – trends in lower extremity amputation incidence in European union 15+ countries 1990–2017. Eur. J. Vasc. Endovascular Surg. 60 (4), 602–612. doi:10.1016/j.ejvs.2020.05.037
Jeyapalina, Sujee, Beck, Peter J., Bloebaum, Roy D., and Bachus, Kent N. (2014). Progression of bone ingrowth and attachment strength for stability of percutaneous osseointegrated prostheses. Clin. Orthop. Relat. Res. 472 (10), 2957–2965. doi:10.1007/s11999-013-3381-0
Juhnke, Dora-Lisa, Beck, James P., Jeyapalina, Sujee, and Aschoff, Horst H. (2015). Fifteen years of experience with integral-leg-prosthesis: cohort study of artificial limb attachment system. J. Rehabilitation Res. Dev. 52 (4), 407–420. doi:10.1682/JRRD.2014.11.0280
Kagan, Ryland, Adams, Jacob, Schulman, Caroline, Laursen, Rachel., Yee-Cheen, Doung, and Hayden, James (2017). What factors are associated with failure of compressive osseointegration fixation? Clin. Orthop. Relat. Res. 475 (3), 698–704. doi:10.1007/s11999-016-4764-9
Kohli, Nupur, Stoddart, Jennifer C., and van Arkel, Richard J. (2021). The limit of tolerable micromotion for implant osseointegration: A systematic review. Sci. Rep. 11 (1), 10797. doi:10.1038/s41598-021-90142-5
Lee, Winson C. C., Doocey, Jacinta M., Brånemark, Rickard, ClaytonAdam, J., Evans, John H., Pearcy, Mark J., et al. (2008a). FE stress analysis of the interface between the bone and an osseointegrated implant for amputees – implications to refine the rehabilitation program. Clin. Biomech. 23 (10), 1243–1250. doi:10.1016/j.clinbiomech.2008.06.012
Lee, Winson C. C., Frossard, Laurent A., Hagberg, Kerstin, Haggstrom, Eva, Gow, David Lee, Gray, Steven, et al. (2008b). Magnitude and variability of loading on the osseointegrated implant of transfemoral amputees during walking. Med. Eng. Phys. 30 (7), 825–833. doi:10.1016/j.medengphy.2007.09.003
Leijendekkers, Ruud A., Gerben van Hinte, , Jan Paul Frölke, , de Meent, Hendrik van, Nijhuis-van der Sanden, Maria W. G., and Bart Staal, J. (2017). Comparison of bone-anchored prostheses and socket prostheses for patients with a lower extremity amputation: a systematic review. Disabil. Rehabilitation 39 (11), 1045–1058. doi:10.1080/09638288.2016.1186752
Marino, Martin, Pattni, Shaan, Greenberg, Max, Miller, Alex, Hocker, Emma, Ritter, Sarah, et al. “Access to prosthetic devices in developing countries: pathways and challenges,” in Proceedings of the 2015 IEEE Global Humanitarian Technology Conference (GHTC), Seattle, WA, USA, October 2015 (IEEE). doi:10.1109/GHTC.2015.7343953
Martelli, S., Taddei, F., Cristofolini, L., Schileo, E., Rushton, N., and Viceconti, M. (2011). A new hip epiphyseal prosthesis: design revision driven by a validated numerical procedure. Med. Eng. Phys. 33 (10), 1203–1211. doi:10.1016/j.medengphy.2011.05.010
Miles, Brad, Kolos, Elizabeth, Walter, William L., Appleyard, Richard, Shi, Angela, Li, Qing, et al. (2015). Subject specific finite element modeling of periprosthetic femoral fracture using element deactivation to simulate bone failure. Med. Eng. Phys. 37 (6), 567–573. doi:10.1016/j.medengphy.2015.03.012
Ming, Zhang, Dong, Xiang, and Fan, Yubo “Stress analysis of osseointegrated transfemoral prosthesis: A finite element model,” in Proceedings of the 2005 IEEE Engineering in Medicine and Biology 27th Annual Conference, Shanghai, China, January 2005 (IEEE). doi:10.1109/IEMBS.2005.1615354
Morosato, Federico, Francesco Traina, , Schierjott, Ronja A., Hettich, Georg, Grupp, Thomas M., and Cristofolini, Luca (2020). Primary stability of revision acetabular reconstructions using an innovative bone graft substitute: A comparative biomechanical study on cadaveric pelvises. Materials 13 (19), 4312. doi:10.3390/ma13194312
Moxey, P. W., Gogalniceanu, P., Hinchliffe, R. J., Loftus, I. M., Jones, K. J., Thompson, M. M., et al. (2011). Lower extremity amputations--a review of global variability in incidence. Diabet. Med. A J. Br. Diabet. Assoc. 28 (10), 1144–1153. doi:10.1111/j.1464-5491.2011.03279.x
Muderis, M. Al, Tetsworth, K., Khemka, A., Wilmot, S., Bosley, B., Lord, S. J., et al. (2016). The osseointegration group of Australia accelerated protocol (OGAAP-1) for two-stage osseointegrated reconstruction of amputated limbs. Bone & Jt. J. 98-B (7), 952–960. doi:10.1302/0301-620X.98B7.37547
Newcombe, L., Dewar, M., Blunn, G. W., and Fromme, P. (2013). Effect of amputation level on the stress transferred to the femur by an artificial limb directly attached to the bone. Med. Eng. Phys. 35 (12), 1744–1753. doi:10.1016/j.medengphy.2013.07.007
Newhall, Karina, Spangler, Emily, Dzebisashvili, Nino, Goodman, David C., and Goodney, Philip (2016). Amputation rates for patients with diabetes and peripheral arterial disease: the effects of race and region. Ann. Vasc. Surg. 30, 292–298.e1. doi:10.1016/j.avsg.2015.07.040
Oefner, Christoph, Herrmann, Sven, Kebbach, Maeruan, Lange, Hans-E., Kluess, Daniel, and Woiczinski, Matthias (2021). Reporting checklist for verification and validation of finite element analysis in orthopedic and trauma Biomechanics. Med. Eng. Phys. 92, 25–32. doi:10.1016/j.medengphy.2021.03.011
Örgel, Marcus, Petri, Maximilian, Alexander, Ranker, Wirries, Nils, Graulich, Tilman, Krettek, Christian, et al. Horst-Heinrich Aschoff (2021). Management, outcome, and novel classification system of periprosthetic fractures in patients with transcutaneous osseointegrated prosthetic systems (TOPS)—A retrospective cohort analysis. Archives Orthop. Trauma Surg. 142, 1499–1509. doi:10.1007/s00402-021-03826-y
Palanca, Marco, Marco, Miguel, Ruspi, Maria Luisa, and Cristofolini, Luca (2018). Full-field strain distribution in multi-vertebra spine segments: an in vitro application of digital image correlation. Med. Eng. Phys. 52, 76–83. doi:10.1016/j.medengphy.2017.11.003
Palanca, Marco, Tozzi, Gianluca, and Cristofolini, Luca (2016). The use of digital image correlation in the biomechanical area: A review. Int. Biomech. 3 (1), 1–21. doi:10.1080/23335432.2015.1117395
Paternò, Linda, Ibrahimi, Michele, Gruppioni, Emanuele, Menciassi, Arianna, and Ricotti, Leonardo (2018). Sockets for limb prostheses: A review of existing technologies and open challenges. IEEE Trans. Biomed. Eng. 65 (9), 1996–2010. doi:10.1109/TBME.2017.2775100
Pilliar, R. M., Lee, J. M., and Maniatopoulos, C. (1986). Observations on the effect of movement on bone ingrowth into porous-surfaced implants. Clin. Orthop. Relat. Res. 208, 108–113. doi:10.1097/00003086-198607000-00023
Prisma, (2022). PRISMA-P. http://Www.Prisma-Statement.Org/.
Prochor, Piotr, and Sajewicz, Eugeniusz (2018). A comparative analysis of internal bone remodelling concepts in a novel implant for direct skeletal attachment of limb prosthesis evaluation: a finite element analysis. Proc. Institution Mech. Eng. Part H J. Eng. Med. 232 (3), 289–298. doi:10.1177/0954411917751003
Prochor, Piotr, and Anna Mierzejewska, Żaneta (2019). Influence of the surface roughness of PEEK GRF30 and Ti6Al4V SLM on the viability of primary human osteoblasts determined by the MTT test. Materials 12 (24), 4189. doi:10.3390/ma12244189
Prochor, P., and Sajewicz, E. (2019). The influence of geometry of implants for direct skeletal attachment of limb prosthesis on rehabilitation program and stress-shielding intensity. BioMed Res. Int. 2019, 1–17. doi:10.1155/2019/6067952
Ranker, Alexander, Örgel, Marcus, Beck, James Peter, Krettek, Christian, and Heinrich Aschoff, Horst (2020). Transkutane osseointegrierte Prothesensysteme (TOPS) zur Versorgung Oberschenkelamputierter: eine sechsjährige retrospektive analyse des aktuellen prothesendesigns in Deutschland. Die Rehabil. 59 (6), 357–365. doi:10.1055/a-1223-3205
Reetz, D., Atallah, R., Mohamed, J., van de Meent, H., Frölke, J. P. M., and Leijendekkers, R. (2020). Safety and performance of bone-anchored prostheses in persons with a transfemoral amputation: A 5-year follow-up study. J. Bone Jt. Surg. 102 (15), 1329–1335. doi:10.2106/JBJS.19.01169
Roberts, P., Chan, Daniel, Grimer, Robert, Sneath, R., and Scales, J. (1991). Prosthetic replacement of the distal femur for primary bone tumours. J. Bone Jt. Surg. Br. 73, 762–769. doi:10.1302/0301-620X.73B5.1894662
Romero, Francisco, Amirouche, Farid, Aram, Luke, and Gonzalez, Mark H. (2007). Experimental and analytical validation of a modular acetabular prosthesis in total hip arthroplasty. J. Orthop. Surg. Res. 2 (1), 7. doi:10.1186/1749-799X-2-7
Rommers, G. M., Vos, L. D., Groothoff, J. W., and Eisma, W. H. (1996). Clinical rehabilitation of the amputee: A retrospective study. Prosthetics Orthot. Int. 20 (2), 72–78. doi:10.3109/03093649609164422
Schileo, Enrico, Taddei, Fulvia, Cristofolini, Luca, and Viceconti, Marco (2008). Subject-specific finite element models implementing a maximum principal strain criterion are able to estimate failure risk and fracture location on human femurs tested in vitro. J. Biomechanics 41 (2), 356–367. doi:10.1016/j.jbiomech.2007.09.009
Stenlund, Patrik, Trobos, Margarita, Lausmaa, Jukka, Brånemark, Rickard, Thomsen, Peter, and Anders, Palmquist (2017). Effect of load on the bone around bone-anchored amputation prostheses: BONE-ANCHORED amputation prostheses. J. Orthop. Res. 35 (5), 1113–1122. doi:10.1002/jor.23352
Thesleff, Alexander, Brånemark, Rickard, Håkansson, Bo, and Ortiz-Catalan, Max (2018). Biomechanical characterisation of bone-anchored implant systems for amputation limb prostheses: A systematic review. Ann. Biomed. Eng. 46 (3), 377–391. doi:10.1007/s10439-017-1976-4
Tomaszewski, P. K., Lasnier, B., Hannink, G., Verkerke, G. J., and Verdonschot, N. (2013). Experimental assessment of a new direct fixation implant for artificial limbs. J. Mech. Behav. Biomed. Mater. 21, 77–85. doi:10.1016/j.jmbbm.2013.02.012
Tomaszewski, P. K., van Diest, M., Bulstra, S. K., Verdonschot, N., and Verkerke, G. J. (2012a). Numerical analysis of an osseointegrated prosthesis fixation with reduced bone failure risk and periprosthetic bone loss. J. Biomechanics 45 (11), 1875–1880. doi:10.1016/j.jbiomech.2012.05.032
Tomaszewski, P. K., Verdonschot, N., Bulstra, S. K., Rietman, J. S., and Verkerke, G. J. (2012b). Simulated bone remodeling around two types of osseointegrated implants for direct fixation of upper-leg prostheses. J. Mech. Behav. Biomed. Mater. 15, 167–175. doi:10.1016/j.jmbbm.2012.06.015
Tomaszewski, P. K., Verdonschot, N., Bulstra, S. K., and Verkerke, G. J. (2010). A comparative finite-element analysis of bone failure and load transfer of osseointegrated prostheses fixations. Ann. Biomed. Eng. 38 (7), 2418–2427. doi:10.1007/s10439-010-9966-9
U.S. Department of Health and Human ServicesFood and Drug Administration, Center for Devices and Radiological Health, Orthopedic Devices Branch, Division of General, Restorative, and Neurological Devices, and Office of Device Evaluation (2003). Knee joint patellofemorotibial and femorotibial metal/polymer porous-coated uncemented prostheses - class II special controls guidance document for industry and FDA. https://www.fda.gov/medical-devices/guidance-documents-medical-devices-and-radiation-emitting-products/knee-joint-patellofemorotibial-and-femorotibial-metalpolymer-porous-coated-uncemented-prostheses.
Welke, Bastian, Hurschler, Christof, Föller, Marie, Michael Schwarze, , and Calliess, Tilman (2013). Stiffness and ultimate load of osseointegrated prosthesis fixations in the upper and lower extremity. Biomed. Eng. OnLine 12 (1), 70. doi:10.1186/1475-925X-12-70
Windrich, Michael, Grimmer, Martin, Christ, Oliver, Rinderknecht, Stephan, and Beckerle, Philipp (2016). Active lower limb prosthetics: a systematic review of design issues and solutions. Biomed. Eng. OnLine 15 (3), 140. doi:10.1186/s12938-016-0284-9
Winter, Werner, Klein, Daniel, and Karl, Matthias (2012). Micromotion of dental implants: basic mechanical considerations. J. Med. Eng. 2013, 1–9. doi:10.1155/2013/265412
Wu, Ge, Sorin Siegler, , Allard, Paul, Kirtley, Chris, Alberto, Leardini, Rosenbaum, Dieter, et al. (2002). ISB recommendation on definitions of joint coordinate system of various joints for the reporting of human joint motion—Part I: ankle, hip, and spine. J. Biomechanics 35 (4), 543–548. doi:10.1016/S0021-9290(01)00222-6
Keywords: transfemoral osseointegrated prosthesis, load transfer, mechanical implant stability, stress shielding, stress concentration, in vitro mechanical test, in silico simulation
Citation: Galteri G and Cristofolini L (2023) In vitro and in silico methods for the biomechanical assessment of osseointegrated transfemoral prostheses: a systematic review. Front. Bioeng. Biotechnol. 11:1237919. doi: 10.3389/fbioe.2023.1237919
Received: 10 June 2023; Accepted: 08 August 2023;
Published: 17 August 2023.
Edited by:
Jose Manuel Garcia-Aznar, University of Zaragoza, SpainReviewed by:
Maria Angeles Perez Anson, University of Zaragoza, SpainPaola Ginestra, University of Brescia, Italy
Copyright © 2023 Galteri and Cristofolini. This is an open-access article distributed under the terms of the Creative Commons Attribution License (CC BY). The use, distribution or reproduction in other forums is permitted, provided the original author(s) and the copyright owner(s) are credited and that the original publication in this journal is cited, in accordance with accepted academic practice. No use, distribution or reproduction is permitted which does not comply with these terms.
*Correspondence: Luca Cristofolini, luca.cristofolini@unibo.it