Lachnospiraceae are emerging industrial biocatalysts and biotherapeutics
- Génomique Métabolique, Genoscope, Institut François Jacob, CEA, CNRS, University of Evry, Université Paris-Saclay, Evry, France
The Lachnospiraceae is a family of anaerobic bacteria in the class Clostridia with potential to advance the bio-economy and intestinal therapeutics. Some species of Lachnospiraceae metabolize abundant, low-cost feedstocks such as lignocellulose and carbon dioxide into value-added chemicals. Others are among the dominant species of the human colon and animal rumen, where they ferment dietary fiber to promote healthy gut and immune function. Here, we summarize recent studies of the physiology, cultivation, and genetics of Lachnospiraceae, highlighting their wide substrate utilization and metabolic products with industrial applications. We examine studies of these bacteria as Live Biotherapeutic Products (LBPs), focusing on in vivo disease models and clinical studies using them to treat infection, inflammation, metabolic syndrome, and cancer. We discuss key research areas including elucidation of intra-specific diversity and genetic modification of candidate strains that will facilitate the exploitation of Lachnospiraceae in industry and medicine.
Introduction
The Lachnospiraceae, from the ancient Greek “lachnos” (wooly hair) and “spira” (coil, twist), is a family of anaerobic, mesophilic bacteria with Gram-positive ultrastructure. Lachnospiraceae, which generally correspond to Clostridia cluster XIVa (Whitman, 2009), inhabit diverse ecosystems. Host-associated species are found in the gastrointestinal tracts of humans (Gosalbes et al., 2011), mice (Meehan and Beiko, 2014), insects (Vera-Ponce de Leon et al., 2022), and ruminants (Seshadri et al., 2018) as well as the human gingival crevice (Antezack et al., 2021). Metagenomic studies have shown Lachnospiraceae account for 10%–45% of the total bacteria in feces of healthy adults (Liu C. et al., 2021) and have a life-long association with humans; they colonize the guts of infants and are enriched in the fecal microbiomes of long-living (>90 years old) individuals (Kong et al., 2016). Other Lachnospiraceae live in anaerobic soil (Hengstmann et al., 1999) where they recycle plant matter and mediate biological soil disinfestation, a pesticide-free method to control soil-borne pathogens (Huang et al., 2019). Lachnospiraceae also inhabit aquatic sediments (Lomans et al., 2001; Dai et al., 2016), Antarctic green snow (Smirnova et al., 2021), wastewater (McLellan et al., 2013), and deep sea hydrothermal vents (Schouw et al., 2016).
Recent interest in the important roles of Lachnospiraceae in gut and environmental ecosystems has led to advances in the genomics, cultivation, and genetic manipulation of these bacteria. Here, we examine these advances and explore how they have set the stage for applying Lachnospiraceae in industrial and medical biotechnology. Many metagenomic studies comparing the fecal communities of healthy and diseased subjects have drawn associations between Lachnospiraceae and human health. However, associations from fecal metagenomics are sometimes difficult to interpret or conflicting, likely due to differences in study design and strain-specific differences, and have been reviewed previously (Vacca et al., 2020; Liu X. et al., 2021). Thus, we focus on insights gained from preclinical, rodent models and human clinical studies involving administration of live Lachnospiraceae. We discuss opportunities and current needs to develop Lachnospiraceae to produce value-added biochemicals from low-cost feedstocks and as live biotherapeutic products (LBPs).
Phylogeny and genomes
Our knowledge of Lachnospiraceae diversity and genomics has greatly expanded over the past decade. In 2014, the NCBI taxonomy of Lachnospiraceae included 24 genera (Meehan and Beiko, 2014), which by 2023 had increased to 118 genera with 1,941 species (NCBI Genome Datasets, 2023). The number of Lachnospiraceae genomes has similarly increased. In 2014, NCBI included 30 Lachnospiraceae genomes (Meehan and Beiko, 2014), which reached 201 genomes in 2023 (NCBI Genome Datasets, 2023). The Integrated Microbial Genomes & Microbiomes (IMG) portal (Chen I.-M. A. et al., 2023) includes 1,292 Lachnospiraceae genomic sequences, of which 57 are finished genomes. Most of the finished Lachnospiraceae genomes are of strains isolated from human feces or oral cavity, with strains from the cow rumen, animal feces, and environmental isolates forming clusters in the Lachnospiraceae phylogeny (Figure 1).
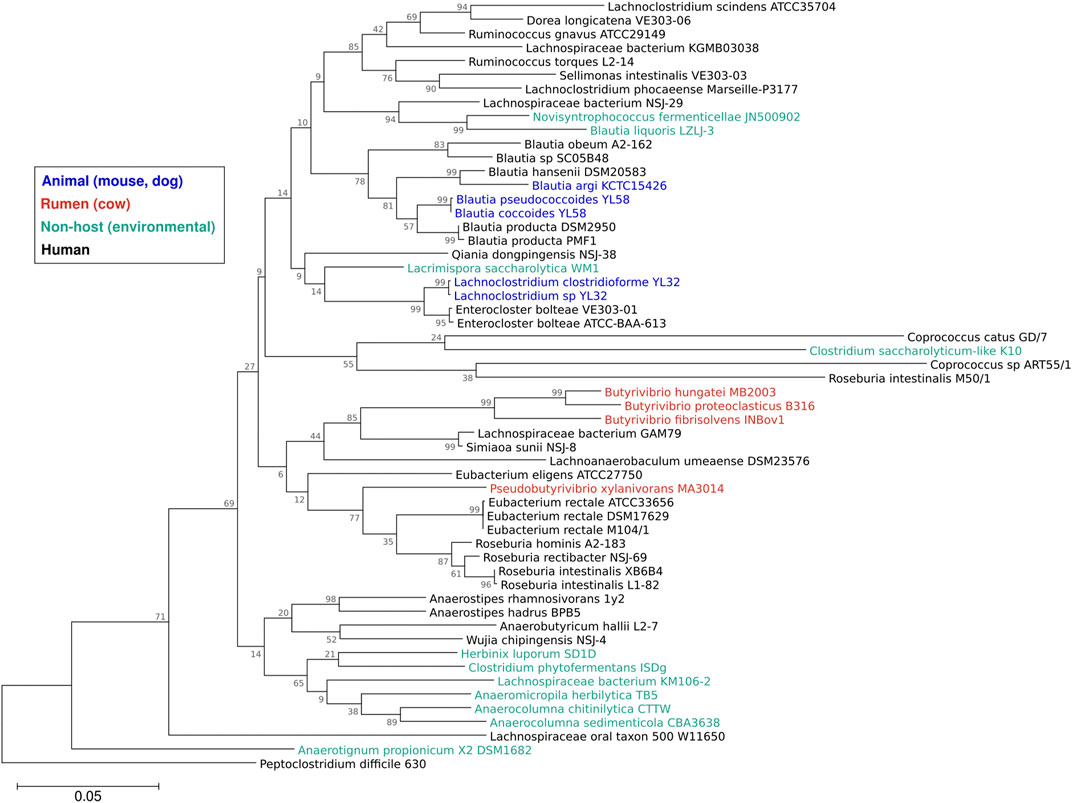
FIGURE 1. Phylogeny of the family Lachnospiraceae. Sequences of the 16S rRNA gene from the 57 Lachnospiraceae finished genomes in the IMG database were aligned using Muscle (Edgar, 2004) and a maximum likelihood tree (1,000 bootstraps) was constructed using Mega11 software (Tamura et al., 2021) with Clostridioides (Peptoclostridium) difficile 630 as the outgroup. Colors show strain isolation sites: animal (dog, mouse) feces (blue), cow rumen (red), human feces or oral cavity (black), or non-host environmental (aqua). Branch numbers are bootstrap percentages, branch lengths are substitution per site.
The GC content of Lachnospiraceae genomes varies between 35%–50%, with members of the same genus typically sharing similar GC levels (Sorbara et al., 2020). The distribution of Lachnospiraceae genome sizes (mean 4.06 Mb, median 3.59 Mb) is similar across habitats (Figure 2A). Herbinix luporum SD1D, a cellulose-degrading strain isolated from a biogas reactor, has the smallest genome of 2.6 Mb encoding 2,632 genes (Koeck et al., 2016). Lachnoclostridium clostridioforme YL32, from the mouse gut, has the largest genome of 7.2 Mb with 7,735 genes (Garzetti et al., 2017). Among these finished genomes, non-host species have a higher number of 16S genes than species isolated from humans (Figure 2B). Higher ribosomal gene copy number is linked to increased maximum growth rate and ability to respond to resource changes (Stevenson and Schmidt, 2004), suggesting these traits are under greater selection in non-host species.
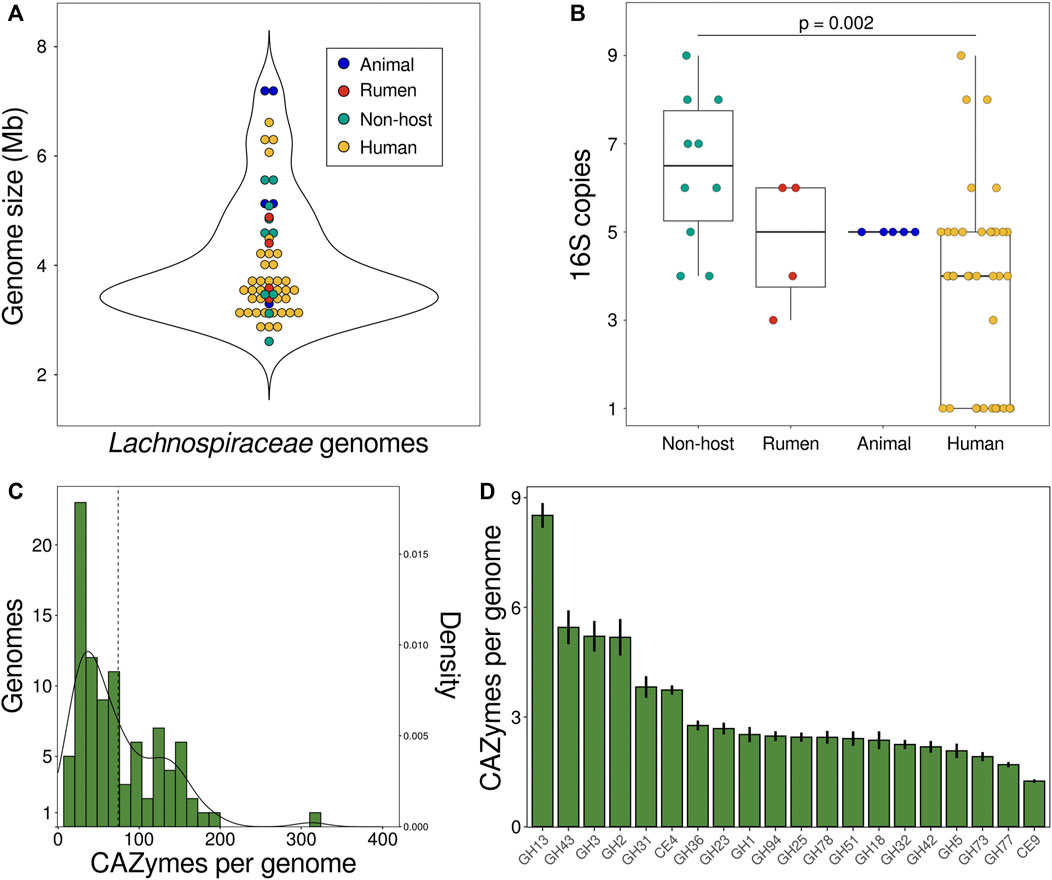
FIGURE 2. Characteristics of Lachnospiraceae genomes. (A) Genomes sizes and (B) number of copies of the 16S gene in 57 finished Lachnospiraceae genomes in the IMG database. Each data point is a genome and colors show strain isolation sites: animal (dog, mouse) feces (blue), cow rumen (red), human feces or oral cavity (yellow), or non-host environmental (aqua). (C) Number of CAZyme genes encoded by Lachnospiraceae genomes. The dashed line is the mean number of CAZymes per genome and the curve shows the kernel density estimation. (D) Number of CAZyme genes per genome for the 20 most abundant CAZyme families summed across Lachnospiraceae genomes. Bars show the mean ± SEM. Data in (C,D) is for degradative enzymes (glycoside hydrolases, carbohydrate esterases, pectin lyases) for 98 species in the CAZy database defined as Lachnospiraceae based on NCBI taxonomy. Mb DNA megabases. SEM standard error of the mean.
Substrate utilization
Species of Lachnospiraceae collectively metabolize plant biomass through the assimilation of polysaccharides, peptides, and aromatics as well as subsequent transformation of the fermentation products by acetogens and cross-feeding species (Figure 3). As primary degraders of plant biomass, many Lachnospiraceae ferment a variety of complex polysaccharides including glucans, mannans, xylans, galactans, pectins, and arabinans (Boutard et al., 2014). Biomass-fermenting species sometimes grow faster on polysaccharides than on the constituent monosaccharides (Boutard et al., 2016). While typically able to ferment multiple polysaccharides, species of Lachnospiraceae are ecologically differentiated by specializing on certain substrates. As such, addition of different glycans results in compound-specific changes to the relative abundances of Lachnospiraceae in mixed communities (Tolonen et al., 2022). For example, Roseburia intestinalis efficiently metabolizes β-mannans and xylan (Leth et al., 2018; La Rosa et al., 2019), while Roseburia faecis ferments arabinogalactan (Sheridan et al., 2016). Lachnospira include pectin specialists, called pectinophiles (Cornick et al., 1994), that are stimulated by pectin addition (Bang et al., 2018). Lachnospiraceae specialized to metabolize cellulose are found in soil (Warnick et al., 2002; Wolin et al., 2003; Dai et al., 2016) and the rumen (Cai and Dong, 2010).
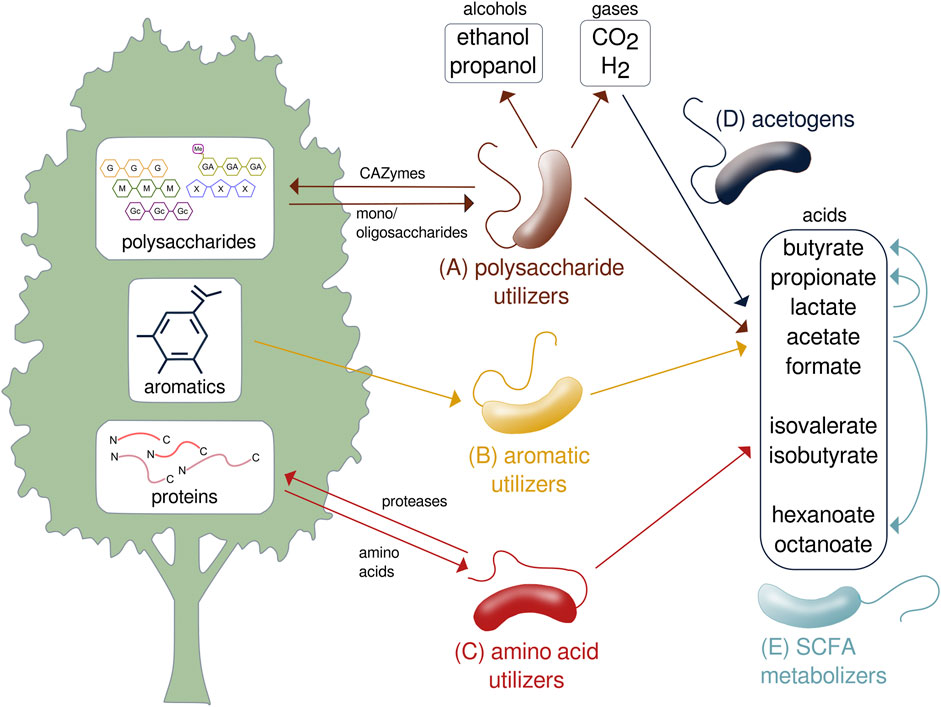
FIGURE 3. Metabolic transformation of lignocellulose performed by species of Lachnospiraceae. Specialized species metabolize the (A) polysaccharide, (B) aromatic, and (C) protein components of lignocellulose to produce gases, acids, and alcohols. (D) Acetogens transform carbon dioxide and hydrogen to SCFAs. (E) SCFAs are further transformed and assimilated by other species. SCFA, short-chain fatty acid; CO2, carbon dioxide; H2, hydrogen; CAZyme, carbohydrate-active enzyme.
Genomes of plant-fermenting Lachnospiraceae encode numerous carbohydrate-active enzymes (CAZymes), each of which cleave a specific glycosidic linkage to depolymerize complex glycans. Lachnospiraceae genomes encode an average of 73 degradative CAZymes (glycoside hydrolases, pectin lyases, carbohydrate esterases) with less than 10% of genomes encoding more than 150 degradative CAZymes (Figure 2C). Lachnospiraceae bacterium CE91-St56, closely related to Eisenbergiella massiliensis, encodes over 100 more CAZymes than any other strain with 313 CAZymes including 294 glycoside hydrolases (Figure 2C). CE91-St56 was isolated from a supercentenarian (>110 years old) (Sato et al., 2021), highlighting the presence of Lachnospiraceae with extensive polysaccharide utilization capabilities in long-lived individuals. Lachnospiraceae genomes encode many families of CAZymes, the most abundant of which is GH13 to depolymerize α-glucans including starch and pullulan (Figure 2D). Other abundant CAZyme families enable degradation of substrates such as β-glucans (GH3,5,51,94), β-galactans (GH42), xylan (GH43), pectin (GH78), and chitin (GH18) (Figure 2D).
Some Lachnospiraceae assimilate C1 and C2 carbon sources that are produced during the initial stages of plant biomass fermentation. Blautia hydrogenotrophica is a human gut acetogen that grows autotrophically using hydrogen to fix carbon dioxide into acetyl-CoA by the Wood–Ljungdahl pathway (Bernalier et al., 1996) and other Lachnospiraceae acetogens consume H2 and CO2 in the cow rumen (Greening and Leedle, 1989). Species that assimilate other C1 and C2 molecules include Sporobacterium, isolated from an olive mill, that grows on methanol (Mechichi et al., 1999). Various Lachnospiraceae such as Anaerobutyricum soehngenii (previously Eubacterium hallii) metabolize acetate to butyrate (Udayappan et al., 2016; Zhang et al., 2019), which represents an important cross-feeding interaction in the gut (Flint et al., 2012).
Other Lachnospiraceae are specialized to metabolize peptides, alkanes, and aromatics. Falcatimonas natans, isolated from a methanogenic reactor, ferments peptides but not carbohydrates (Watanabe et al., 2016). Abyssivirga alkaniphila, a hydrothermal vent species, can grow on straight and branched alkanes (C5 to C25) using thiosulfate as an external electron acceptor (Schouw et al., 2016). Syntrophococcus sucromutans can oxidize sugars to acetate using formate or methoxymonobenzenoids as electron acceptors (Krumholz and Bryant, 1986). Parasporobacterium and Sporobacterium are soil bacteria that grow on methoxylated aromatic compounds as sole carbon and energy sources by catabolizing them to short-chain fatty acids (SCFAs) (Lomans et al., 2001). These aromatics are degraded by transforming the side chains and cleaving the aromatic ring using the phloroglucinol pathway (Whitman, 2009), as has recently been shown for Clostridium scatologenes (Zhou et al., 2023).
Metabolic products
Lachnospiraceae metabolism yields alcohols, gases, and acids with importance in industry and human health (Table 1). Species producing industrially-relevant alcohols include Lachnoclostridium phytofermentans, which ferments cellulose to ethanol at 68% of the maximum theoretical yield (Tolonen et al., 2011) and, similar to Roseburia inulinivorans, metabolizes fucose and rhamnose to 1-propanol through a 1,2-propanediol intermediate in polyhedral microcompartments (Scott et al., 2006; Petit et al., 2013). Lachnospiraceae produce hydrogen by co-expressing monomeric and multimeric, bifurcating [FeFe] hydrogenases along with energy conserving [NiFe] hydrogenases (Calusinska et al., 2010) to enable yields reaching 2–3 moles of hydrogen per mole glucose (Harvey et al., 2008).
Fermentation of dietary fiber by gut Lachnospiraceae yields three main SCFA (acetate, propionate, and butyrate) (Table 1), all of which have been shown to benefit health. Acetate, which is synthesized in two steps from acetyl-CoA, reduces adipose accumulation and improves glucose tolerance (Yamashita et al., 2007). Lachnospiraceae use the acrylate, propanediol, and myo-inositol pathways to synthesize propionate (Reichardt et al., 2014; Bui et al., 2021), which is absorbed from the gut into the bloodstream to regulate cholesterol (Berggren et al., 1996) and reduce visceral and liver fat accumulation (Chambers et al., 2015). Propionate also enhances satiety (Arora et al., 2011), making it a potential way to reduce obesity. Along with Ruminococcaceae, Lachnospiraceae are the dominant butyrate producers in the human gut (Vital et al., 2014). While butyrate is most often synthesized from acetyl-CoA using butyryl-CoA transferase or butyrate kinase, some Lachnospiraceae can produce butyrate from lysine, glutarate, and 4-aminobutyrate (Vital et al., 2014), highlighting their abilities produce butyrate from different nutritional sources. Butyrate is the preferred energy source of colonocytes (Litvak et al., 2018), suppresses pathogens (Walker et al., 2021), and stimulates differentiation of anti-inflammatory T-regulatory cells (Furusawa et al., 2013).
Other organic acids produced by Lachnospiraceae include the branched-chain fatty acids isobutyrate and isovalerate that are synthesized from valine and leucine, respectively (Watanabe et al., 2016). Candidatus Weimeria bifida metabolizes pentoses to medium-chain fatty acids (hexanoate and octanoate) using the reverse β-oxidation cycle for SCFA chain elongation (Scarborough et al., 2020). Species of Blautia metabolize pyruvate to succinate (Hosomi et al., 2022) and, depending on the species, Lachnospiraceae can either produce L-lactate (Tolonen et al., 2011) or metabolize DL-lactate to acetate or butyrate using stereospecific lactate dehydrogenases (Sheridan et al., 2022). Gut Lachnospiraceae ferment proline to 5-aminovalerate in competition with Clostridioides difficile, representing a potential means to prevent colonization by this pathogen (Lopez et al., 2020). Lachnospiraceae ferment aromatic amino acids (tryptophan, tyrosine, phenylalanine) to phenolic and indolic acids (Russell et al., 2013), including compounds such as indoleacetic acid that promote intestinal homeostasis by signaling through the aryl hydrocarbon receptor (Roager and Licht, 2018).
Lachnospiraceae also produce other metabolites with health and industrial applications. Lachnospiraceae can modify and cleave the heterocyclic C-ring of flavonoids (Braune and Blaut, 2016) yielding molecules such as equol, which is linked to prevention of colorectal cancer (Sugiyama et al., 2013) and aging-related disorders (Mayo et al., 2019). Gut Lachnospiraceae generate reactive sulfur species that protect the host from oxidative stress-induced liver injury (Uchiyama et al., 2022). Lachnospiraceae produce farnesol (Abdugheni et al., 2022), an isoprene-derived molecule with anti-inflammatory and neuroprotective activities (Sell et al., 2022). Farnesol also has industrial applications as a fragrance ingredient (Lapczynski et al., 2008) and diesel fuel precursor (Rude and Schirmer, 2009). Butyrivibrio fibrisolvens synthesizes an exopolysaccharide with potential industrial applications that is rheologically similar to xanthan gum, but is composed of rare sugars including L-altrose and L-iduronic acid (Wachenheim and Patterson, 1992; Ferreira et al., 1997).
Lachnospiraceae are a potential source of other antimicrobial and immunomodulatory compounds. Blautia obeum synthesizes a lantibiotic, nisin O, that inhibits pathogens, including C. difficile and Clostridium perfringens (Hatziioanou et al., 2017). Lachnospiraceae produce pyrazines, which are being developed as antimicrobial and anti-fungal drugs (Hassan et al., 2020). Gut Lachnospiraceae convert primary bile acids by 7α-dehydroxylation to secondary bile acids that inhibit enteric pathogens and regulate mucosal immunity (Jin et al., 2022). Nonribosomal peptide synthetases (NRPS) of human gut Lachnospiraceae produce immunomodulatory secondary metabolites such as di-peptide aldehydes that act as cell-permeable cathepsin inhibitors, which could act as immunosuppressors by blocking antigen processing (Guo et al., 2017).
Cultivation and engineering
Lachnospiraceae are mesophiles that grow at 30°C–45°C and can be cultivated under standard anaerobic conditions using jars with anaerobic sachets or a glove box. A human gut Lachnospiraceae biobank (hLchsp) was established as part of the China General Microorganism Culture Collection by isolating strains from healthy adult fecal samples, yielding a collection of 77 species across 33 genera with Lachnospira, Blautia, and Roseburia being the most abundant genera (Abdugheni et al., 2022). Among the seven growth media used for isolations, Yeast Casitone Fatty Acid (YCFA) medium supported the greatest number of Lachnospiraceae species, which comprised 19.6% of all bacterial isolates from the fecal samples. Another project to cultivate human gut Lachnospiraceae using rich, non-selective media yielded 273 isolates, including all the Lachnospiraceae genera that were detected by metagenomic sequences of the donor feces (Sorbara et al., 2020). In contrast to gut species, more oligotrophic, GS2 medium is used to cultivate environmental heterotrophic Lachnospiraceae such as Herbinix hemicellulosilytica and L. phytofermentans (Warnick et al., 2002; Koeck et al., 2015). The acetogen B. hydrogenotrophica can be grown on DMSZ 114 or general-acetogen (GA) medium, either as an autotroph using H2:CO2 or as a heterotroph by adding a carbon source (Groher and Weuster-Botz, 2016).
Methods for the genetic modification of Lachnospiraceae are being developed to study their molecular biology and to engineer optimized strains. Early studies found that conjugative transposons bearing antibiotic resistance genes transfer DNA between Lachnospiraceae (Barbosa et al., 1999). Experimental methods have been developed to transfer plasmid DNA into species of Lachnoclostridium, Roseburia, Eubacterium, Enterocloster, Lacrimispora, and Blautia by conjugation with Escherichia coli (Tolonen et al., 2009; Cuív et al., 2015; Sheridan et al., 2019; Jin et al., 2022) and by electroporation into species of Lachnoclostridium and Butyrivibrio (Beard et al., 1995; Rostain et al., 2022) (Figure 4A).
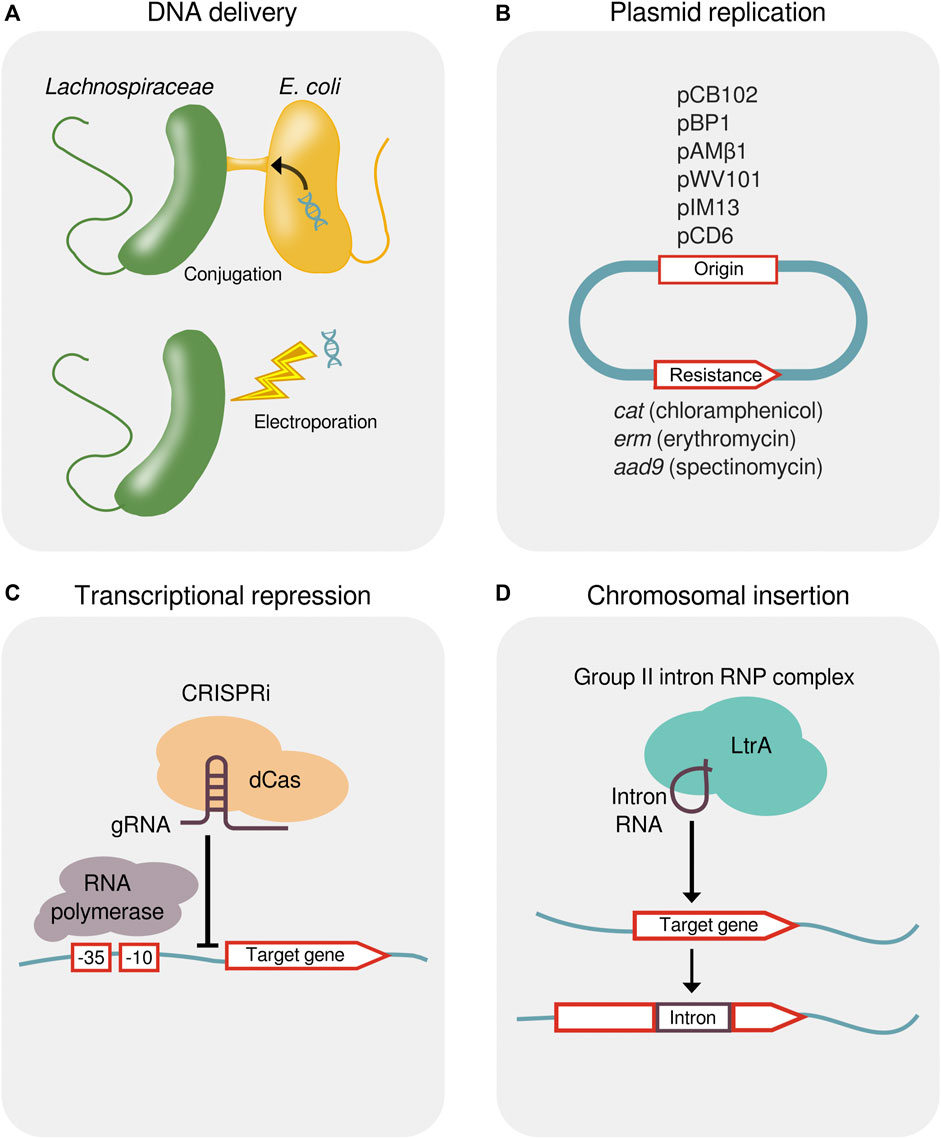
FIGURE 4. Methods for the genetic manipulation of Lachnospiraceae. (A) Delivery of foreign DNA into Lachnospiraceae by either conjugal transfer from E. coli or electroporation. (B) Plasmid origins that replicate in Lachnospiraceae and antibiotic resistance genes for plasmid selection. (C) Transcriptional repression by CRISPRi showing a dCas protein targeted by a gRNA to bind upstream of a gene, thereby blocking progression of RNA polymerase. (D) Targeted chromosomal insertion using a designed group II intron (targetron). Genomic insertion of the group II intron RNA containing a 13–16 bp target recognition sequence is facilitated by the endonuclease and reverse transcriptase activity of the LtrA protein. CRISPRi, CRISPR interference; dCas, dead CRISPR-associated protein; gRNA, guide RNA; RNP, ribonucleoprotein.
Native plasmids from Lachnospiraceae have been used as expression vectors (Hefford et al., 1997) and the pMTL plasmid system (Heap et al., 2009) can be applied to identify plasmid origins and resistance markers that function in strains of interest (Jin et al., 2022; Rostain et al., 2022). Plasmid origins that have been shown to replicate in Lachnospiraceae include pCB102 from Clostridium butyricum, pBP1 from Clostridium botulinum, pAMβ1 from Enterococcus faecalis, pWV101 from Lactococcus lactis, pIM13 from Bacillus subtilis, and pCD6 from C. difficile, and these plasmids can be maintained using antibiotic resistance genes catP (chloramphenicol), ermB (erythromycin), or aad9 (spectinomycin) (Sheridan et al., 2019; Jin et al., 2022; Rostain et al., 2022) (Figure 4B).
Shuttle vectors containing Gram-negative and Gram-positive origins of replication have permitted the heterologous expression of a β-(1,3–1,4)-glucanase in Eubacterium rectale and R. inulinivorans (Sheridan et al., 2019) and of a synthetic ethanol formation pathway in L. phytofermentans (Tolonen et al., 2015b). Plasmid-based expression of a NanoLuc reporter has identified a library of promoters of varying strength, and reporter gene expression can be regulated by anhydrotetracycline using a promoter flanked tet repressor sites (Rostain et al., 2022). Clustered Regularly Interspaced Short Palindromic Repeats interference (CRISPRi) using dCas12a has been applied to repress the transcription of chromosomal genes for fermentation, thereby reducing production of butyrate in Blautia luti and Enterocloster boltae (Jin et al., 2022) and acetate in L. phytofermentans (Rostain et al., 2022) (Figure 4C).
Similar to other Clostridia, low rates of DNA transfer and homologous recombination in Lachnospiraceae have led to the use of other recombination systems to make targeted chromosomal changes. Designed group II intron called targetrons enabled gene inactivation by targeted chromosome insertion in various Lachnospiraceae with efficiencies ranging from 12.5%–100% (Tolonen et al., 2009; Tolonen et al., 2015a; Cerisy et al., 2019a; Jin et al., 2022) (Figure 4D). Multi-gene fragments can be excised and inserted by modifying targetrons to deliver lox sites into the genome that act as anchor points for Cre-mediated recombination, which has been applied to delete a 39 kb prophage in L. phytofermentans (Cerisy et al., 2019b).
Lachnospiraceae proteins that have been applied as molecular tools in other organisms are strong candidates to advance genome engineering in Lachnospiraceae. For example, LbCas12a, which was isolated from Lachnospiraceae bacterium ND 2006 (Tak et al., 2017), has been applied for genome editing in eukaryotes including fungi (Chen T. et al., 2023), plants (Kim et al., 2021), flies (Port et al., 2020), and human cells (Zhang et al., 2023). Another Cas12a variant, Lb2Cas12a, derived from Lachnospiraceae bacterium MA 2020, has been developed to have enhanced editing activity and broadened protospacer adjacent motif (PAM) recognition in human cells (Tran et al., 2021). A protein with anti-CRISPR function has been identified from Lachnospiraceae phage (Forsberg et al., 2019) and a cytosine deaminase from R. intestinalis has been fused to CRISPR and Transcription Activator-Like Effector (TALE) proteins to make targeted C-to-T transitions in the genomes of cultured cells and mouse embryos (Guo et al., 2023).
Bioeconomy applications
The capabilities of Lachnospiraceae to metabolize the polysaccharides, aromatics, and proteins that compose lignocellulose make them candidates to transform low-cost, sustainable, lignocellulosic feedstocks (i.e., forestry, agricultural, and municipal wastes) into value-added biochemicals (Table 1). For example, L. phytofermentans, a species with wide polysaccharide utilization capabilities, ferments corn stover to ethanol with efficiencies similar to commercial enzymes and xylose-fermenting yeast (Jin et al., 2011). Synthesis of longer chain acids from lignocellulose residues by Ca. Weimeria bifida (Scarborough et al., 2020) holds potential to develop ‘drop in’ fuels that are compatible with the current petroleum infrastructure. F. natans metabolizes the protein fraction of organic matter to produce isobutyrate and n-butyrate, which are used for artificial fibers, plastics and herbicides as well as isovalerate used for flavoring and perfumes (Agnihotri et al., 2022).
Lachnospiraceae have been identified as biocatalysts for conversion of biomass to hydrogen (Bu et al., 2021), and strains have been isolated that produce 2–3 moles hydrogen per mole of glucose equivalent (Harvey et al., 2008). In addition to its use as a fuel, hydrogen produced from lignocellulosic fermentation can be used as a reductant to fix CO and CO2 by acetogens through gas fermentation (Figure 3), a process that has potential to convert industrial carbon emissions to useful biochemicals (Liew et al., 2016). Lachnospiraceae acetogens compete with methanogens in the cow rumen, representing a means to reduce bovine methane production (Yang et al., 2015).
Alternatively, hydrogen from lignocellulosic fermentation can be used to fix carbon dioxide by methanogens, and Lachnospiraceae have been shown to actuate the transformation of cellulose to methane by methanogenic consortia (Dai et al., 2016). Combination of Lachnospiraceae with other microorganisms to form synthetic consortia has been generally used to enhanced product formation rates (Zuroff et al., 2013; El Hage et al., 2019; Park et al., 2020), and engineered consortia including biomass-fermenting Lachnospiraceae and methanogens or acetogens have potential for the direct conversion of lignocellulose to methane and other value-added biochemicals.
Therapeutic applications: preclinical models
Preclinical in vivo models are providing evidence that supplementation with live Lachnospiraceae improves gut health and prevents pathogen colonization. For example, in a rat model of irritable bowel syndrome, addition of Roseburia hominis increased cecal butyrate content, reduced visceral hypersensitivity, and prevented the decreased expression of occludin (Zhang et al., 2019). Administration of Blautia producta directly inhibited growth of vancomycin-resistant Enterococcus (Caballero et al., 2017) and a murine Lachnospiraceae inhibits C. difficile colonization in mice (Reeves et al., 2012).
Lachnospiraceae can alleviate inflammatory and allergic diseases by modulating the immune system through production of antigens presented by innate immune cells and immunomodulatory metabolites. R. intestinalis promoted differentiation of regulatory T cells, activation of type 3 innate lymphoid cells, and suppression of inflammation through TLR5 (Shen et al., 2022). E. rectale supplementation regulated dendritic cell activation by reducing the frequency of CD83+ cells and improved symptoms in a mouse model of Behçet’s disease, a systemic inflammatory condition (Islam et al., 2021). Inoculation of germ-free mice with a 17 strain consortium including 8 Lachnospiraceae increased anti-inflammatory, regulatory T-cells (CD4+, FoxP3+) in the colonic lamina propria of multiple mouse lines and alleviated colitis in experimental models (Atarashi et al., 2013). In addition, administration of this 17 strain consortium to mice in an ovalbumin (OVA)-induced allergic diarrhea mouse model reduced diarrhea and OVA-specific serum IgE levels (Atarashi et al., 2013). Additional evidence that Lachnospiraceae can mitigate food allergies was demonstrated by colonization of germ-free mice with Anaerostipes caccae, which protected against an anaphylactic response upon challenge with β-Lactoglobulin (BLG), a cow’s milk allergen, and reduced BLG-specific IgE levels (Feehley et al., 2019).
Lachnospiraceae have provided benefits in preclinical models of metabolic syndrome and diabetes. Administration of Blautia wexlerae to male C57BL/6 mice on a high fat diet reduced body weight and multiple diabetes indicators, which was linked to B. wexlerae metabolites such as S-adenosylmethionine, acetylcholine, and L-ornithine conferring anti-adipogenesis and anti-inflammatory properties to adipocytes (Hosomi et al., 2022). B. producta suppressed lipid accumulation in HepG2 cells and gavage of B. producta alleviated hyperlipidemia in mice through production of 12-methylmyristic acid (Wu et al., 2021). Treatment of obese, diabetic db/db mice with A. soehngenii reduced plasma glucose, epididymal fat, and liver triglycerides and improved peripheral insulin sensitivity (Udayappan et al., 2016).
Mouse studies have also shown beneficial roles of Lachnospiraceae in cancer treatment. C57BL/6 mice bearing B16-F10 melanoma or CT-26 colorectal tumors showed reduced tumor growth when administered Blautia massiliensis (Goodman et al., 2019). Anti-PD1 mediated tumor control and survivorship of C57BL/6 mice bearing B16-F10 melanoma tumors was improved by administration of E. rectale, which was proposed to result from it consuming L-serine, leading to NK cell activation and tumor infiltration (Liu et al., 2023). E. rectale treatment also reduced the incidence of lymphoma and reduced TNF levels in sensitized Eμ-Myc mice (Lu et al., 2022). Survivorship and clinical scores of C57BL/6 mice following full body irradiation were increased by prior inoculation with a mix of 23 Lachnospiraceae, which resulted from increased hematopoiesis and reduced intestinal epithelial injury (Guo et al., 2020).
Therapeutic applications: clinical trials
Lachnospiraceae are being tested as LBPs in clinical studies for a number of diseases (Table 2), the most advanced of which is the treatment of recurrent C. difficile infections (rCDI). In an exploratory study of two rCDI patients at Queen’s University, treatment with a synthetic community of 33 strains, including 10 Lachnospiraceae, resulted in full remission in both patients (Petrof et al., 2013). Subsequently, microbial consortia containing Lachnospiraceae have been developed to combat rCDI. SER-109, consisting of spores purified from fecal samples, is composed of 77 genera of which 36% are Lachnospiraceae (Feuerstadt et al., 2022). In a phase 3 study, SER-109 reduced rCDI at week 8 (Feuerstadt et al., 2022). In 2023, the US Food and Drug Administration approved SER-109 under the commercial name VowstTM to treat recurrent C. difficile infection (Commission of the US FDA, 2023). VE303, a consortium of 8 strains including 5 Lachnospiraceae, engrafted into the microbiomes of healthy volunteers to boost production of SCFA and secondary bile acids without any serious adverse events (Dsouza et al., 2022). Phase 2 results in patients supported that rCDI at 8 weeks was reduced by VE303 (Louie et al., 2023). VE303 is being tested in a phase 3 study to treat rCDI starting in 2023, and as an experimental treatment for hepatic encephalopathy (Vedanta Biosciences Inc, 2023).
LBPs that include Lachnospiraceae are also being developed for metabolic syndrome and inflammatory bowel disease (Table 2). For example, A. soehngenii L2-7 is being tested as a probiotic to improve insulin sensitivity in metabolic syndrome patients. A phase 1/2 study of 24 metabolic syndrome patients correlated A. soehngenii engraftment with improved peripheral insulin sensitivity (Gilijamse et al., 2020). A phase 2 study of 12 metabolic syndrome patients showed duodenal infusion of A. soehngenii reduced glucose variability and elevated GLP-1, secondary bile acids in plasma, duodenal REGB1 expression, and fecal SCFAs (Koopen et al., 2022). As B. hydrogenotrophica consumes intestinal gas (H2, CO2), it can treat irritable bowel syndrome (IBS) by reducing intestinal bloating. A phase 2 study of MRx1234 (Blautix), consisting of lyophilized B. hydrogenotrophica, improved bowel habits in IBS patients (Quigley et al., 2023).
Looking forward: challenges and opportunities
Lachnospiraceae have numerous potential applications due to their native fermentation of low-cost substrates and importance for intestinal health, but key challenges remain to harness them as industrial biocatalysts and LBPs. In particular, work is needed to isolate and characterize additional Lachnospiraceae strains, uncover the genetic basis of their physiological traits, and, ultimately, apply these learning to engineer optimized strains. Recently established Lachnospiraceae collections demonstrated methods with which many species can be cultivated (Seshadri et al., 2018; Sorbara et al., 2020; Abdugheni et al., 2022). Additional cultivation efforts could build Lachnospiraceae collections from environments such as soil and human patient populations. Further, Lachnospiraceae culture collections revealed that isolates from the same species can differ in traits such as substrate utilization and production of antimicrobials (Sorbara et al., 2020). Intraspecific comparative genomics of isolates sharing similar genomes, but with specific physiological differences, is an opportunity to define genotype-phenotype relationships in Lachnospiraceae.
Development of microorganisms for biotechnology often requires rewiring native metabolism to improve product yields, highlighting the importance of future research on genetic manipulation of Lachnospiraceae. As described above, genetic tools have been ported to Lachnospiraceae from other well-studied mesophiles including B. subtilis and L. lactis. Species of Lachnospiraceae have been established as genetically tractable hosts with methods for genetic transformation, plasmid replication and selection, transcriptional repression, and chromosomal insertions (Figure 4); a recent study demonstrates that these methods can be generally applied to many species (Jin et al., 2022). Existing molecular tools derived from Lachnospiraceae are promising candidates to advance genome engineering in these bacteria, including Cas proteins with broadened PAM recognition (Tran et al., 2021), anti-CRISPR proteins (Forsberg et al., 2019), and cytosine deaminases (Guo et al., 2023). Moreover, adaptive laboratory evolution can be used to generate Lachnospiraceae strains with complex, multigenic traits such as inhibitor tolerance that are intractable by rational genome engineering (Cerisy et al., 2017).
Engineering of Lachnospiraceae for industrial production of biochemicals from low-cost feedstocks will need to focus on substrate assimilation and product formation. The rate of lignocellulose solubilization remains a primary obstacle to its utilization as a feedstock (Preethi et al., 2021), which could be addressed by engineering strains with modified expression of CAZymes and associated ABC transporters to accelerate solubilization and uptake of target lignocellulosic substrates. As Lachnospiraceae typically produce a mixture of fermentation products, redistribution of metabolic flux by repression or inactivation of genes for undesired products is a valuable approach to streamline and increase fermentation yields.
Genetic manipulation of Lachnospiraceae will also be important to define the mechanisms by which they promote intestinal health. Significant advances have been made to elucidate innate and adaptive immune responses modulated by Lachnospiraceae (Islam et al., 2021, 1) (Atarashi et al., 2013; Feehley et al., 2019; Liu et al., 2023). It is generally believed that Lachnospiraceae modulate host immunity producing SCFAs. Recently, it was shown that differential recognition of Lachnospiraceae flagellins by TLR5 contributes to immune tolerance (Clasen et al., 2023). Genetic studies with Lachnospiraceae mutants will be useful to further define the mechanisms that underlie host interactions and build strains with customized immunomodulatory properties.
Industrial-scale cultivation of Lachnospiraceae for bioproduction and LBPs will necessitate a greater understanding of the genetics and ecology of the phage that infect them. Phage infection is recognized as a persistent threat in industrial microbiology where scale-up of bacterial populations in large bioreactors favors phage outbreaks. For example, phage infection is the main cause of fermentation failures in the dairy industry, leading to intense study of the phage of lactic acid bacteria (Fernández et al., 2017). Prophages are common in the genomes of gut Lachnospiraceae (Dikareva et al., 2023). Although hypervirulent phage can drive temporal variation of R. intestinalis abundances in the intestine, no phage infecting Lachnospiraceae have been deposited in public databases (Cornuault et al., 2020). Thus, isolation and characterization of Lachnospiraceae phage are important subjects for future research.
Over the past decade, our understanding of Lachnospiraceae molecular biology and physiology has greatly increased. In 2023, VowstTM became the first FDA-approved oral medication containing live Lachnospiraceae (Commission of the US FDA, 2023) and clinical studies are evaluating additional therapeutic benefits of Lachnospiraceae (Table 2). Development of optimized strains by genome engineering will enable us to realize the potential of Lachnospiraceae in biotechnology. In the next few years, we expect to see further development of Lachnospiraceae to produce useful chemicals and to manage disease through targeted changes to the composition and metabolites produced by the gut microbiome.
Author contributions
TZ: Writing–original draft, Writing–review and editing. SM: Writing–original draft, Writing–review and editing. AT: Conceptualization, Writing–original draft, Writing–review and editing.
Funding
The authors declare financial support was received for the research, authorship, and/or publication of this article. This work was supported by Genoscope-CEA and the Évry Genopole under the “Action Thématique Incitative Genopole” Grant (funding ATIGE 2021 No. 2698).
Conflict of interest
The authors declare that the research was conducted in the absence of any commercial or financial relationships that could be construed as a potential conflict of interest.
Publisher’s note
All claims expressed in this article are solely those of the authors and do not necessarily represent those of their affiliated organizations, or those of the publisher, the editors and the reviewers. Any product that may be evaluated in this article, or claim that may be made by its manufacturer, is not guaranteed or endorsed by the publisher.
References
Abdugheni, R., Wang, W.-Z., Wang, Y.-J., Du, M.-X., Liu, F.-L., Zhou, N., et al. (2022). Metabolite profiling of human-originated Lachnospiraceae at the strain level. iMeta 1, e58. doi:10.1002/imt2.58
Agnihotri, S., Yin, D.-M., Mahboubi, A., Sapmaz, T., Varjani, S., Qiao, W., et al. (2022). A glimpse of the world of volatile fatty acids production and application: a review. Bioengineered 13, 1249–1275. doi:10.1080/21655979.2021.1996044
Antezack, A., Boxberger, M., La Scola, B., and Monnet-Corti, V. (2021). Isolation and description of catonella massiliensis sp. nov., a novel catonella species, isolated from a stable periodontitis subject. Pathogens 10, 367. doi:10.3390/pathogens10030367
Arora, T., Sharma, R., and Frost, G. (2011). Propionate. Anti-obesity and satiety enhancing factor? Appetite 56, 511–515. doi:10.1016/j.appet.2011.01.016
Atarashi, K., Tanoue, T., Oshima, K., Suda, W., Nagano, Y., Nishikawa, H., et al. (2013). Treg induction by a rationally selected mixture of Clostridia strains from the human microbiota. Nature 500, 232–236. doi:10.1038/nature12331
Bang, S.-J., Kim, G., Lim, M. Y., Song, E.-J., Jung, D.-H., Kum, J.-S., et al. (2018). The influence of in vitro pectin fermentation on the human fecal microbiome. Amb. Express 8, 98. doi:10.1186/s13568-018-0629-9
Barbosa, T. M., Scott, K. P., and Flint, H. J. (1999). Evidence for recent intergeneric transfer of a new tetracycline resistance gene, tet(W), isolated from Butyrivibrio fibrisolvens, and the occurrence of tet(O) in ruminal bacteria. Environ. Microbiol. 1, 53–64. doi:10.1046/j.1462-2920.1999.00004.x
Beard, C. E., Hefford, M. A., Forster, R. J., Sontakke, S., Teather, R. M., and Gregg, K. (1995). A stable and efficient transformation system for Butyrivibrio fibrisolvens OB156. Curr. Microbiol. 30, 105–109. doi:10.1007/BF00294191
Berggren, A. M., Nyman, E. M., Lundquist, I., and Björck, I. M. (1996). Influence of orally and rectally administered propionate on cholesterol and glucose metabolism in obese rats. Br. J. Nutr. 76, 287–294. doi:10.1079/bjn19960032
Bernalier, A., Willems, A., Leclerc, M., Rochet, V., and Collins, M. D. (1996). Ruminococcus hydrogenotrophicus sp. nov., a new H2/CO2-utilizing acetogenic bacterium isolated from human feces. Arch. Microbiol. 166, 176–183. doi:10.1007/s002030050373
Boutard, M., Cerisy, T., Nogue, P.-Y., Alberti, A., Weissenbach, J., Salanoubat, M., et al. (2014). Functional diversity of carbohydrate-active enzymes enabling a bacterium to ferment plant biomass. PLoS Genet. 10, e1004773. doi:10.1371/journal.pgen.1004773
Boutard, M., Ettwiller, L., Cerisy, T., Alberti, A., Labadie, K., Salanoubat, M., et al. (2016). Global repositioning of transcription start sites in a plant-fermenting bacterium. Nat. Commun. 7, 13783. doi:10.1038/ncomms13783
Braune, A., and Blaut, M. (2016). Bacterial species involved in the conversion of dietary flavonoids in the human gut. Gut Microbes 7, 216–234. doi:10.1080/19490976.2016.1158395
Bu, J., Wei, H.-L., Wang, Y.-T., Cheng, J.-R., and Zhu, M.-J. (2021). Biochar boosts dark fermentative H2 production from sugarcane bagasse by selective enrichment/colonization of functional bacteria and enhancing extracellular electron transfer. Water Res. 202, 117440. doi:10.1016/j.watres.2021.117440
Bui, T. P. N., Mannerås-Holm, L., Puschmann, R., Wu, H., Troise, A. D., Nijsse, B., et al. (2021). Conversion of dietary inositol into propionate and acetate by commensal Anaerostipes associates with host health. Nat. Commun. 12, 4798. doi:10.1038/s41467-021-25081-w
Caballero, S., Kim, S., Carter, R. A., Leiner, I. M., Sušac, B., Miller, L., et al. (2017). Cooperating commensals restore colonization resistance to vancomycin-resistant Enterococcus faecium. Cell Host Microbe 21, 592–602.e4. doi:10.1016/j.chom.2017.04.002
Cai, S., and Dong, X. (2010). Cellulosilyticum ruminicola gen. nov., sp. nov., isolated from the rumen of yak, and reclassification of Clostridium lentocellum as Cellulosilyticum lentocellum comb. nov. Int. J. Syst. Evol. Microbiol. 60, 845–849. doi:10.1099/ijs.0.014712-0
Calusinska, M., Happe, T., Joris, B., and Wilmotte, A. (2010). The surprising diversity of clostridial hydrogenases: a comparative genomic perspective. Microbiol. Read. 156, 1575–1588. doi:10.1099/mic.0.032771-0
Cerisy, T., Iglesias, A., Rostain, W., Boutard, M., Pelle, C., Perret, A., et al. (2019a). ABC transporters required for hexose uptake by Clostridium phytofermentans. J. Bacteriol. 201, e00241-19. doi:10.1128/JB.00241-19
Cerisy, T., Rostain, W., Chhun, A., Boutard, M., Salanoubat, M., and Tolonen, A. C. (2019b). A targetron-recombinase system for large-scale genome engineering of clostridia. mSphere 4, e00710-19. doi:10.1128/mSphere.00710-19
Cerisy, T., Souterre, T., Torres-Romero, I., Boutard, M., Dubois, I., Patrouix, J., et al. (2017). Evolution of a biomass-fermenting bacterium to resist lignin phenolics. Appl. Environ. Microbiol. 83, e00289-17. doi:10.1128/AEM.00289-17
Chambers, E. S., Viardot, A., Psichas, A., Morrison, D. J., Murphy, K. G., Zac-Varghese, S. E. K., et al. (2015). Effects of targeted delivery of propionate to the human colon on appetite regulation, body weight maintenance and adiposity in overweight adults. Gut 64, 1744–1754. doi:10.1136/gutjnl-2014-307913
Chen, I.-M. A., Chu, K., Palaniappan, K., Ratner, A., Huang, J., Huntemann, M., et al. (2023a). The IMG/M data management and analysis system v.7: content updates and new features. Nucleic Acids Res. 51, D723–D732. doi:10.1093/nar/gkac976
Chen, T., Chen, Z., Zhang, H., Li, Y., Yao, L., Zeng, B., et al. (2023b). Development of a CRISPR/Cpf1 system for multiplex gene editing in Aspergillus oryzae. Folia Microbiol. (Praha). doi:10.1007/s12223-023-01081-9
Clasen, S. J., Bell, M. E. W., Borbón, A., Lee, D.-H., Henseler, Z. M., de la Cuesta-Zuluaga, J., et al. (2023). Silent recognition of flagellins from human gut commensal bacteria by Toll-like receptor 5. Sci. Immunol. 8, eabq7001. doi:10.1126/sciimmunol.abq7001
Commission of the US Food and Drug Administration (2023). FDA approves first orally administered fecal microbiota product for the prevention of recurrence of Clostridioides difficile infection. https://www.fda.gov/news-events/press-announcements/fda-approves-first-orally-administered-fecal-microbiota-product-prevention-recurrence-clostridioides.
Cornick, N. A., Jensen, N. S., Stahl, D. A., Hartman, P. A., and Allison, M. J. (1994). Lachnospira pectinoschiza sp. nov., an anaerobic pectinophile from the pig intestine. Int. J. Syst. Bacteriol. 44, 87–93. doi:10.1099/00207713-44-1-87
Cornuault, J. K., Moncaut, E., Loux, V., Mathieu, A., Sokol, H., Petit, M.-A., et al. (2020). The enemy from within: a prophage of Roseburia intestinalis systematically turns lytic in the mouse gut, driving bacterial adaptation by CRISPR spacer acquisition. ISME J. 14, 771–787. doi:10.1038/s41396-019-0566-x
Cuív, P. Ó., Smith, W. J., Pottenger, S., Burman, S., Shanahan, E. R., and Morrison, M. (2015). Isolation of genetically tractable most-wanted bacteria by metaparental mating. Sci. Rep. 5, 13282. doi:10.1038/srep13282
Dai, Y., Yan, Z., Jia, L., Zhang, S., Gao, L., Wei, X., et al. (2016). The composition, localization and function of low-temperature-adapted microbial communities involved in methanogenic degradations of cellulose and chitin from Qinghai-Tibetan Plateau wetland soils. J. Appl. Microbiol. 121, 163–176. doi:10.1111/jam.13164
Dikareva, E., Matharu, D., Lahtinen, E., Kolho, K.-L., De Vos, W. M., Salonen, A., et al. (2023). An extended catalog of integrated prophages in the infant and adult fecal microbiome shows high prevalence of lysogeny. Front. Microbiol. 14, 1254535. doi:10.3389/fmicb.2023.1254535
Dostal, A., Lacroix, C., Bircher, L., Pham, V. T., Follador, R., Zimmermann, M. B., et al. (2015). Iron modulates butyrate production by a child gut microbiota in vitro. mBio 6, e01453–e01415. doi:10.1128/mBio.01453-15
Dsouza, M., Menon, R., Crossette, E., Bhattarai, S. K., Schneider, J., Kim, Y.-G., et al. (2022). Colonization of the live biotherapeutic product VE303 and modulation of the microbiota and metabolites in healthy volunteers. Cell Host Microbe 30, 583–598.e8. doi:10.1016/j.chom.2022.03.016
Edgar, R. C. (2004). MUSCLE: a multiple sequence alignment method with reduced time and space complexity. BMC Bioinforma. 5, 113. doi:10.1186/1471-2105-5-113
El Hage, R., Hernandez-Sanabria, E., Calatayud Arroyo, M., Props, R., and Van de Wiele, T. (2019). Propionate-producing consortium restores antibiotic-induced dysbiosis in a dynamic in vitro model of the human intestinal microbial ecosystem. Front. Microbiol. 10, 1206. doi:10.3389/fmicb.2019.01206
Feehley, T., Plunkett, C. H., Bao, R., Choi Hong, S. M., Culleen, E., Belda-Ferre, P., et al. (2019). Healthy infants harbor intestinal bacteria that protect against food allergy. Nat. Med. 25, 448–453. doi:10.1038/s41591-018-0324-z
Fernández, L., Escobedo, S., Gutiérrez, D., Portilla, S., Martínez, B., García, P., et al. (2017). Bacteriophages in the dairy environment: from enemies to allies. Antibiotics 6, 27. doi:10.3390/antibiotics6040027
Ferreira, F., Kenne, L., Cotta, M. A., and Stack, R. J. (1997). Structural studies of the extracellular polysaccharide from Butyrivibrio fibrisolvens strain CF3. Carbohydr. Res. 301, 193–203. doi:10.1016/s0008-6215(97)00097-9
Feuerstadt, P., Louie, T. J., Lashner, B., Wang, E. E. L., Diao, L., Bryant, J. A., et al. (2022). SER-109, an oral microbiome therapy for recurrent Clostridioides difficile infection. N. Engl. J. Med. 386, 220–229. doi:10.1056/NEJMoa2106516
Flint, H. J., Scott, K. P., Duncan, S. H., Louis, P., and Forano, E. (2012). Microbial degradation of complex carbohydrates in the gut. Gut Microbes 3, 289–306. doi:10.4161/gmic.19897
Forsberg, K. J., Bhatt, I. V., Schmidtke, D. T., Javanmardi, K., Dillard, K. E., Stoddard, B. L., et al. (2019). Functional metagenomics-guided discovery of potent Cas9 inhibitors in the human microbiome. eLife 8, e46540. doi:10.7554/eLife.46540
Furusawa, Y., Obata, Y., Fukuda, S., Endo, T. A., Nakato, G., Takahashi, D., et al. (2013). Commensal microbe-derived butyrate induces the differentiation of colonic regulatory T cells. Nature 504, 446–450. doi:10.1038/nature12721
Garzetti, D., Brugiroux, S., Bunk, B., Pukall, R., McCoy, K. D., Macpherson, A. J., et al. (2017). High-quality whole-genome sequences of the oligo-mouse-microbiota bacterial community. Genome Announc. 5, 007588-17. doi:10.1128/genomeA.00758-17
Gilijamse, P. W., Hartstra, A. V., Levin, E., Wortelboer, K., Serlie, M. J., Ackermans, M. T., et al. (2020). Treatment with Anaerobutyricum soehngenii: a pilot study of safety and dose-response effects on glucose metabolism in human subjects with metabolic syndrome. NPJ Biofilms Microbiomes 6, 16. doi:10.1038/s41522-020-0127-0
Goodman, B., Sandy, P., Papkoff, J., Gardner, H., and Ponichtera, H. (2019). Treating cancer using a blautia strain. US Patent application 2019/0240267 A1.
Gosalbes, M. J., Durbán, A., Pignatelli, M., Abellan, J. J., Jiménez-Hernández, N., Pérez-Cobas, A. E., et al. (2011). Metatranscriptomic approach to analyze the functional human gut microbiota. PLOS ONE 6, e17447. doi:10.1371/journal.pone.0017447
Greening, R. C., and Leedle, J. A. Z. (1989). Enrichment and isolation of Acetitomaculum ruminis, gen. nov., sp. nov.: acetogenic bacteria from the bovine rumen. Arch. Microbiol. 151, 399–406. doi:10.1007/BF00416597
Groher, A., and Weuster-Botz, D. (2016). General medium for the autotrophic cultivation of acetogens. Bioprocess Biosyst. Eng. 39, 1645–1650. doi:10.1007/s00449-016-1634-5
Guo, C.-J., Chang, F.-Y., Wyche, T. P., Backus, K. M., Acker, T. M., Funabashi, M., et al. (2017). Discovery of reactive microbiota-derived metabolites that inhibit host proteases. Cell 168, 517–526.e18. doi:10.1016/j.cell.2016.12.021
Guo, H., Chou, W.-C., Lai, Y., Liang, K., Tam, J. W., Brickey, W. J., et al. (2020). Multi-omics analyses of radiation survivors identify radioprotective microbes and metabolites. Science 370, eaay9097. doi:10.1126/science.aay9097
Guo, J., Yu, W., Li, M., Chen, H., Liu, J., Xue, X., et al. (2023). A DddA ortholog-based and transactivator-assisted nuclear and mitochondrial cytosine base editors with expanded target compatibility. Mol. Cell 83, 1710–1724.e7. doi:10.1016/j.molcel.2023.04.012
Harvey, S., Chambers, A., and Zhang, P. (2008). Overproduction of hydrogen from an anaerobic bacterium. https://apps.dtic.mil/sti/citations/ADA505872.
Hassan, N. W., Saudi, M. N., Abdel-Ghany, Y. S., Ismail, A., Elzahhar, P. A., Sriram, D., et al. (2020). Novel pyrazine based anti-tubercular agents: design, synthesis, biological evaluation and in silico studies. Bioorg Chem. 96, 103610. doi:10.1016/j.bioorg.2020.103610
Hatziioanou, D., Gherghisan-Filip, C., Saalbach, G., Horn, N., Wegmann, U., Duncan, S. H., et al. (2017). Discovery of a novel lantibiotic nisin O from Blautia obeum A2-162, isolated from the human gastrointestinal tract. Microbiol. Read. 163, 1292–1305. doi:10.1099/mic.0.000515
Heap, J. T., Pennington, O. J., Cartman, S. T., and Minton, N. P. (2009). A modular system for Clostridium shuttle plasmids. J. Microbiol. Methods 78, 79–85. doi:10.1016/j.mimet.2009.05.004
Hefford, M. A., Kobayashi, Y., Allard, S. E., Forster, R. J., and Teather, R. M. (1997). Sequence analysis and characterization of pOM1, a small cryptic plasmid from Butyrivibrio fibrisolvens, and its use in construction of a new family of cloning vectors for Butyrivibrios. Appl. Environ. Microbiol. 63, 1701–1711. doi:10.1128/aem.63.5.1701-1711.1997
Hengstmann, U., Chin, K. J., Janssen, P. H., and Liesack, W. (1999). Comparative phylogenetic assignment of environmental sequences of genes encoding 16S rRNA and numerically abundant culturable bacteria from an anoxic rice paddy soil. Appl. Environ. Microbiol. 65, 5050–5058. doi:10.1128/AEM.65.11.5050-5058.1999
Henn, M. R., O’Brien, E. J., Diao, L., Feagan, B. G., Sandborn, W. J., Huttenhower, C., et al. (2021). A phase 1b safety study of SER-287, a spore-based microbiome therapeutic, for active mild to moderate ulcerative colitis. Gastroenterology 160, 115–127.e30. doi:10.1053/j.gastro.2020.07.048
Hillman, E. T., Kozik, A. J., Hooker, C. A., Burnett, J. L., Heo, Y., Kiesel, V. A., et al. (2020). Comparative genomics of the genus Roseburia reveals divergent biosynthetic pathways that may influence colonic competition among species. Microb. Genom 6, mgen000399. doi:10.1099/mgen.0.000399
Hosomi, K., Saito, M., Park, J., Murakami, H., Shibata, N., Ando, M., et al. (2022). Oral administration of Blautia wexlerae ameliorates obesity and type 2 diabetes via metabolic remodeling of the gut microbiota. Nat. Commun. 13, 4477. doi:10.1038/s41467-022-32015-7
Huang, X., Liu, L., Zhao, J., Zhang, J., and Cai, Z. (2019). The families Ruminococcaceae, Lachnospiraceae, and Clostridiaceae are the dominant bacterial groups during reductive soil disinfestation with incorporated plant residues. Appl. Soil Ecol. 135, 65–72. doi:10.1016/j.apsoil.2018.11.011
Islam, S. M. S., Ryu, H.-M., Sayeed, H. M., Byun, H.-O., Jung, J.-Y., Kim, H.-A., et al. (2021). Eubacterium rectale attenuates HSV-1 induced systemic inflammation in mice by inhibiting CD83. Front. Immunol. 12, 712312. doi:10.3389/fimmu.2021.712312
Jin, M., Balan, V., Gunawan, C., and Dale, B. E. (2011). Consolidated bioprocessing (CBP) performance of Clostridium phytofermentans on AFEX-treated corn stover for ethanol production. Biotechnol. Bioeng. 108, 1290–1297. doi:10.1002/bit.23059
Jin, W.-B., Li, T.-T., Huo, D., Qu, S., Li, X. V., Arifuzzaman, M., et al. (2022). Genetic manipulation of gut microbes enables single-gene interrogation in a complex microbiome. Cell 185, 547–562.e22. doi:10.1016/j.cell.2021.12.035
Kim, D., Hager, M., Brant, E., and Budak, H. (2021). Efficient genome editing in wheat using Cas9 and Cpf1 (AsCpf1 and LbCpf1) nucleases. Funct. Integr. Genomics 21, 355–366. doi:10.1007/s10142-021-00782-z
Koeck, D. E., Ludwig, W., Wanner, G., Zverlov, V. V., Liebl, W., and Schwarz, W. H. (2015). Herbinix hemicellulosilytica gen. nov., sp. nov., a thermophilic cellulose-degrading bacterium isolated from a thermophilic biogas reactor. Int. J. Syst. Evol. Microbiol. 65, 2365–2371. doi:10.1099/ijs.0.000264
Koeck, D. E., Maus, I., Wibberg, D., Winkler, A., Zverlov, V. V., Liebl, W., et al. (2016). Complete genome sequence of Herbinix luporum SD1D, a new cellulose-degrading bacterium isolated from a thermophilic biogas reactor. Genome Announc. 4, 006877-16. doi:10.1128/genomeA.00687-16
Kong, F., Hua, Y., Zeng, B., Ning, R., Li, Y., and Zhao, J. (2016). Gut microbiota signatures of longevity. Curr. Biol. 26, R832–R833. doi:10.1016/j.cub.2016.08.015
Koopen, A., Witjes, J., Wortelboer, K., Majait, S., Prodan, A., Levin, E., et al. (2022). Duodenal Anaerobutyricum soehngenii infusion stimulates GLP-1 production, ameliorates glycaemic control and beneficially shapes the duodenal transcriptome in metabolic syndrome subjects: a randomised double-blind placebo-controlled cross-over study. Gut 71, 1577–1587. doi:10.1136/gutjnl-2020-323297
Krumholz, L. R., and Bryant, M. P. (1986). Syntrophococcus sucromutans sp. nov. gen. nov. uses carbohydrates as electron donors and formate, methoxymonobenzenoids or Methanobrevibacter as electron acceptor systems. Arch. Microbiol. 143, 313–318. doi:10.1007/BF00412795
Lapczynski, A., Bhatia, S. P., Letizia, C. S., and Api, A. M. (2008). Fragrance material review on farnesol. Food Chem. Toxicol. 46 (Suppl. 11), S149–S156. doi:10.1016/j.fct.2008.06.046
La Rosa, S. L., Leth, M. L., Michalak, L., Hansen, M. E., Pudlo, N. A., Glowacki, R., et al. (2019). The human gut Firmicute Roseburia intestinalis is a primary degrader of dietary β-mannans. Nat. Commun. 10, 905. doi:10.1038/s41467-019-08812-y
Leth, M. L., Ejby, M., Workman, C., Ewald, D. A., Pedersen, S. S., Sternberg, C., et al. (2018). Differential bacterial capture and transport preferences facilitate co-growth on dietary xylan in the human gut. Nat. Microbiol. 3, 570–580. doi:10.1038/s41564-018-0132-8
Liew, F., Martin, M. E., Tappel, R. C., Heijstra, B. D., Mihalcea, C., and Köpke, M. (2016). Gas fermentation—a flexible platform for commercial scale production of low-carbon-fuels and chemicals from waste and renewable feedstocks. Front. Microbiol. 7, 694. doi:10.3389/fmicb.2016.00694
Litvak, Y., Byndloss, M. X., and Bäumler, A. J. (2018). Colonocyte metabolism shapes the gut microbiota. Science 362, eaat9076. doi:10.1126/science.aat9076
Liu, C., Du, M.-X., Abuduaini, R., Yu, H.-Y., Li, D.-H., Wang, Y.-J., et al. (2021a). Enlightening the taxonomy darkness of human gut microbiomes with a cultured biobank. Microbiome 9, 119. doi:10.1186/s40168-021-01064-3
Liu, N., Chen, L., Yan, M., Tao, Q., Wu, J., Chen, J., et al. (2023). Eubacterium rectale improves the efficacy of anti-PD1 immunotherapy in melanoma via l-serine-mediated NK cell activation. Research 6, 0127. doi:10.34133/research.0127
Liu, X., Mao, B., Gu, J., Wu, J., Cui, S., Wang, G., et al. (2021b). Blautia-a new functional genus with potential probiotic properties? Gut Microbes 13, 1–21. doi:10.1080/19490976.2021.1875796
Lomans, B. P., Leijdekkers, P., Wesselink, J. J., Bakkes, P., Pol, A., van der Drift, C., et al. (2001). Obligate sulfide-dependent degradation of methoxylated aromatic compounds and formation of methanethiol and dimethyl sulfide by a freshwater sediment isolate, Parasporobacterium paucivorans gen. nov., sp. nov. Appl. Environ. Microbiol. 67, 4017–4023. doi:10.1128/AEM.67.9.4017-4023.2001
Lopez, C. A., McNeely, T. P., Nurmakova, K., Beavers, W. N., and Skaar, E. P. (2020). Clostridioides difficile proline fermentation in response to commensal clostridia. Anaerobe 63, 102210. doi:10.1016/j.anaerobe.2020.102210
Louie, T., Golan, Y., Khanna, S., Bobilev, D., Erpelding, N., Fratazzi, C., et al. (2023). VE303, a defined bacterial consortium, for prevention of recurrent Clostridioides difficile infection: a randomized clinical trial. Jama 329, 1356–1366. doi:10.1001/jama.2023.4314
Lu, H., Xu, X., Fu, D., Gu, Y., Fan, R., Yi, H., et al. (2022). Butyrate-producing Eubacterium rectale suppresses lymphomagenesis by alleviating the TNF-induced TLR4/MyD88/NF-κB axis. Cell Host Microbe 30, 1139–1150.e7. doi:10.1016/j.chom.2022.07.003
Mayo, B., Vázquez, L., and Flórez, A. B. (2019). Equol: a bacterial metabolite from the daidzein isoflavone and its presumed beneficial health effects. Nutrients 11, 2231. doi:10.3390/nu11092231
McGovern, B. H., Ford, C. B., Henn, M. R., Pardi, D. S., Khanna, S., Hohmann, E. L., et al. (2021). SER-109, an investigational microbiome drug to reduce recurrence after Clostridioides difficile infection: lessons learned from a phase 2 trial. Clin. Infect. Dis. 72, 2132–2140. doi:10.1093/cid/ciaa387
McLellan, S. L., Newton, R. J., Vandewalle, J. L., Shanks, O. C., Huse, S. M., Eren, A. M., et al. (2013). Sewage reflects the distribution of human faecal Lachnospiraceae. Environ. Microbiol. 15, 2213–2227. doi:10.1111/1462-2920.12092
Mechichi, T., Labat, M., Garcia, J. L., Thomas, P., and Patel, B. K. (1999). Sporobacterium olearium gen. nov., sp. nov., a new methanethiol-producing bacterium that degrades aromatic compounds, isolated from an olive mill wastewater treatment digester. Int. J. Syst. Bacteriol. 49 (Pt 4), 1741–1748. doi:10.1099/00207713-49-4-1741
Meehan, C. J., and Beiko, R. G. (2014). A phylogenomic view of ecological specialization in the Lachnospiraceae, a family of digestive tract-associated bacteria. Genome Biol. Evol. 6, 703–713. doi:10.1093/gbe/evu050
NCBI Genome Datasets (Lachnospiraceae) (2023). Genome, https://www.ncbi.nlm.nih.gov/datasets/genome/?taxon=186803&reference_only=true.
O Sheridan, P., Martin, J. C., Lawley, T. D., Browne, H. P., Harris, H. M. B., Bernalier-Donadille, A., et al. (2016). Polysaccharide utilization loci and nutritional specialization in a dominant group of butyrate-producing human colonic Firmicutes. Microb. Genom 2, e000043. doi:10.1099/mgen.0.000043
Park, H., Patel, A., Hunt, K. A., Henson, M. A., and Carlson, R. P. (2020). Artificial consortium demonstrates emergent properties of enhanced cellulosic-sugar degradation and biofuel synthesis. NPJ Biofilms Microbiomes 6, 59. doi:10.1038/s41522-020-00170-8
Petit, E., LaTouf, W. G., Coppi, M. V., Warnick, T. A., Currie, D., Romashko, I., et al. (2013). Involvement of a bacterial microcompartment in the metabolism of fucose and rhamnose by Clostridium phytofermentans. PLoS ONE 8, e54337. doi:10.1371/journal.pone.0054337
Petrof, E. O., Gloor, G. B., Vanner, S. J., Weese, S. J., Carter, D., Daigneault, M. C., et al. (2013). Stool substitute transplant therapy for the eradication of Clostridium difficile infection: ‘RePOOPulating’ the gut. Microbiome 1, 3. doi:10.1186/2049-2618-1-3
Port, F., Starostecka, M., and Boutros, M. (2020). Multiplexed conditional genome editing with Cas12a in Drosophila. Proc. Natl. Acad. Sci. U. S. A. 117, 22890–22899. doi:10.1073/pnas.2004655117
Preethi, M., Kumar, G., Karthikeyan, O. P., and Varjani, S. (2021). Lignocellulosic biomass as an optimistic feedstock for the production of biofuels as valuable energy source: techno-economic analysis, Environmental Impact Analysis, Breakthrough and Perspectives. Environ. Technol. Innovation 24, 102080. doi:10.1016/j.eti.2021.102080
Quigley, E. M. M., Markinson, L., Stevenson, A., Treasure, F. P., and Lacy, B. E. (2023). Randomised clinical trial: efficacy and safety of the live biotherapeutic product MRx1234 in patients with irritable bowel syndrome. Aliment. Pharmacol. Ther. 57, 81–93. doi:10.1111/apt.17310
Reeves, A. E., Koenigsknecht, M. J., Bergin, I. L., and Young, V. B. (2012). Suppression of Clostridium difficile in the gastrointestinal tracts of germfree mice inoculated with a murine isolate from the family Lachnospiraceae. Infect. Immun. 80, 3786–3794. doi:10.1128/IAI.00647-12
Reichardt, N., Duncan, S. H., Young, P., Belenguer, A., McWilliam Leitch, C., Scott, K. P., et al. (2014). Phylogenetic distribution of three pathways for propionate production within the human gut microbiota. ISME J. 8, 1323–1335. doi:10.1038/ismej.2014.14
Roager, H. M., and Licht, T. R. (2018). Microbial tryptophan catabolites in health and disease. Nat. Commun. 9, 3294. doi:10.1038/s41467-018-05470-4
Rostain, W., Zaplana, T., Boutard, M., Baum, C., Tabuteau, S., Sanitha, M., et al. (2022). Tuning of gene expression in Clostridium phytofermentans using synthetic promoters and CRISPRi. ACS Synth. Biol. 11, 4077–4088. doi:10.1021/acssynbio.2c00385
Rude, M. A., and Schirmer, A. (2009). New microbial fuels: a biotech perspective. Curr. Opin. Microbiol. 12, 274–281. doi:10.1016/j.mib.2009.04.004
Russell, W. R., Duncan, S. H., Scobbie, L., Duncan, G., Cantlay, L., Calder, A. G., et al. (2013). Major phenylpropanoid-derived metabolites in the human gut can arise from microbial fermentation of protein. Mol. Nutr. Food Res. 57, 523–535. doi:10.1002/mnfr.201200594
Sato, Y., Atarashi, K., Plichta, D. R., Arai, Y., Sasajima, S., Kearney, S. M., et al. (2021). Novel bile acid biosynthetic pathways are enriched in the microbiome of centenarians. Nature 599, 458–464. doi:10.1038/s41586-021-03832-5
Scarborough, M. J., Myers, K. S., Donohue, T. J., and Noguera, D. R. (2020). Medium-chain fatty acid synthesis by “Candidatus Weimeria bifida” gen. Nov., sp. nov., and “Candidatus pseudoramibacter fermentans” sp. nov. Appl. Environ. Microbiol. 86, 022422-19. doi:10.1128/AEM.02242-19
Schouw, A., Leiknes Eide, T., Stokke, R., Pedersen, R. B., Steen, I. H., and Bødtker, G. (2016). Abyssivirga alkaniphila gen. nov., sp. nov., an alkane-degrading, anaerobic bacterium from a deep-sea hydrothermal vent system, and emended descriptions of Natranaerovirga pectinivora and Natranaerovirga hydrolytica. Int. J. Syst. Evol. Microbiol. 66, 1724–1734. doi:10.1099/ijsem.0.000934
Scott, K. P., Martin, J. C., Campbell, G., Mayer, C.-D., and Flint, H. J. (2006). Whole-genome transcription profiling reveals genes up-regulated by growth on fucose in the human gut bacterium “Roseburia inulinivorans.”. J. Bacteriol. 188, 4340–4349. doi:10.1128/JB.00137-06
Sell, L. B., Ramelow, C. C., Kohl, H. M., Hoffman, K., Bains, J. K., Doyle, W. J., et al. (2022). Farnesol induces protection against murine CNS inflammatory demyelination and modifies gut microbiome. Clin. Immunol. 235, 108766. doi:10.1016/j.clim.2021.108766
Seshadri, R., Leahy, S. C., Attwood, G. T., Teh, K. H., Lambie, S. C., Cookson, A. L., et al. (2018). Cultivation and sequencing of rumen microbiome members from the Hungate1000 Collection. Nat. Biotechnol. 36, 359–367. doi:10.1038/nbt.4110
Shen, Z., Luo, W., Tan, B., Nie, K., Deng, M., Wu, S., et al. (2022). Roseburia intestinalis stimulates TLR5-dependent intestinal immunity against Crohn’s disease. eBioMedicine 85, 104285. doi:10.1016/j.ebiom.2022.104285
Sheridan, P. O., Louis, P., Tsompanidou, E., Shaw, S., Harmsen, H. J., Duncan, S. H., et al. (2022). Distribution, organization and expression of genes concerned with anaerobic lactate utilization in human intestinal bacteria. Microb. Genom 8, 000739. doi:10.1099/mgen.0.000739
Sheridan, P. O., Martin, J. C., Minton, N. P., Flint, H. J., O’Toole, P. W., and Scott, K. P. (2019). Heterologous gene expression in the human gut bacteria Eubacterium rectale and Roseburia inulinivorans by means of conjugative plasmids. Anaerobe 59, 131–140. doi:10.1016/j.anaerobe.2019.06.008
Silber, J., Norman, J., Kanno, T., Crossette, E., Szabady, R., Menon, R., et al. (2022). Randomized, double-blind, placebo (pbo)-controlled, single- and multiple-dose phase 1 study of ve202, a defined bacterial consortium for treatment of ibd: safety and colonization dynamics of a novel live biotherapeutic product (lbp) in healthy adults. Inflamm. Bowel Dis. 28, S65–S66. doi:10.1093/ibd/izac015.106
Smirnova, M., Miamin, U., Kohler, A., Valentovich, L., Akhremchuk, A., Sidarenka, A., et al. (2021). Isolation and characterization of fast-growing green snow bacteria from coastal East Antarctica. MicrobiologyOpen 10, e1152. doi:10.1002/mbo3.1152
Sorbara, M. T., Littmann, E. R., Fontana, E., Moody, T. U., Kohout, C. E., Gjonbalaj, M., et al. (2020). Functional and genomic variation between human-derived isolates of Lachnospiraceae reveals inter- and intra-species diversity. Cell Host Microbe 28, 134–146.e4. doi:10.1016/j.chom.2020.05.005
Stevenson, B. S., and Schmidt, T. M. (2004). Life history implications of rRNA gene copy number in Escherichia coli. Appl. Environ. Microbiol. 70, 6670–6677. doi:10.1128/AEM.70.11.6670-6677.2004
Sugiyama, Y., Masumori, N., Fukuta, F., Yoneta, A., Hida, T., Yamashita, T., et al. (2013). Influence of isoflavone intake and equol-producing intestinal flora on prostate cancer risk. Asian Pac J. Cancer Prev. 14, 1–4. doi:10.7314/apjcp.2013.14.1.1
Tak, Y. E., Kleinstiver, B. P., Nuñez, J. K., Hsu, J. Y., Horng, J. E., Gong, J., et al. (2017). Inducible and multiplex gene regulation using CRISPR-Cpf1-based transcription factors. Nat. Methods 14, 1163–1166. doi:10.1038/nmeth.4483
Takada, T., Kurakawa, T., Tsuji, H., and Nomoto, K. (2013). Fusicatenibacter saccharivorans gen. nov., sp. nov., isolated from human faeces. Int. J. Syst. Evol. Microbiol. 63, 3691–3696. doi:10.1099/ijs.0.045823-0
Tamura, K., Stecher, G., and Kumar, S. (2021). MEGA11: molecular evolutionary genetics analysis version 11. Mol. Biol. Evol. 38, 3022–3027. doi:10.1093/molbev/msab120
Tolonen, A. C., Beauchemin, N., Bayne, C., Li, L., Tan, J., Lee, J., et al. (2022). Synthetic glycans control gut microbiome structure and mitigate colitis in mice. Nat. Commun. 13, 1244. doi:10.1038/s41467-022-28856-x
Tolonen, A. C., Cerisy, T., El-Sayyed, H., Boutard, M., Salanoubat, M., and Church, G. M. (2015a). Fungal lysis by a soil bacterium fermenting cellulose. Environ. Microbiol. 17, 2618–2627. doi:10.1111/1462-2920.12495
Tolonen, A. C., Chilaka, A. C., and Church, G. M. (2009). Targeted gene inactivation in Clostridium phytofermentans shows that cellulose degradation requires the family 9 hydrolase Cphy3367. Mol. Microbiol. 74, 1300–1313. doi:10.1111/j.1365-2958.2009.06890.x
Tolonen, A. C., Haas, W., Chilaka, A. C., Aach, J., Gygi, S. P., and Church, G. M. (2011). Proteome-wide systems analysis of a cellulosic biofuel-producing microbe. Mol. Syst. Biol. 7, 461. doi:10.1038/msb.2010.116
Tolonen, A. C., Zuroff, T. R., Ramya, M., Boutard, M., Cerisy, T., and Curtis, W. R. (2015b). Physiology, genomics, and pathway engineering of an ethanol-tolerant strain of Clostridium phytofermentans. Appl. Environ. Microbiol. 81, 5440–5448. doi:10.1128/AEM.00619-15
Tran, M. H., Park, H., Nobles, C. L., Karunadharma, P., Pan, L., Zhong, G., et al. (2021). A more efficient CRISPR-Cas12a variant derived from Lachnospiraceae bacterium MA2020. Mol. Ther. Nucleic Acids 24, 40–53. doi:10.1016/j.omtn.2021.02.012
Uchiyama, J., Akiyama, M., Hase, K., Kumagai, Y., and Kim, Y.-G. (2022). Gut microbiota reinforce host antioxidant capacity via the generation of reactive sulfur species. Cell Rep. 38, 110479. doi:10.1016/j.celrep.2022.110479
Udayappan, S., Manneras-Holm, L., Chaplin-Scott, A., Belzer, C., Herrema, H., Dallinga-Thie, G. M., et al. (2016). Oral treatment with Eubacterium hallii improves insulin sensitivity in db/db mice. NPJ Biofilms Microbiomes 2, 16009. doi:10.1038/npjbiofilms.2016.9
Vacca, M., Celano, G., Calabrese, F. M., Portincasa, P., Gobbetti, M., and De Angelis, M. (2020). The controversial role of human gut Lachnospiraceae. Microorganisms 8, E573. doi:10.3390/microorganisms8040573
Vedanta Biosciences, Inc (2023). Vedanta Biosciences VE303 program. https://www.vedantabio.com/pipeline/ve303.
Vera-Ponce de Leon, A., Schneider, M. G., Jahnes, B. C., Sadowski, V., Camuy-Vélez, L. A., Duan, J., et al. (2022). Genetic drift and host-adaptive features likely underlie cladogenesis of insect-associated Lachnospiraceae. Genome Biol. Evol. 14, evac086. doi:10.1093/gbe/evac086
Vital, M., Howe, A. C., and Tiedje, J. M. (2014). Revealing the bacterial butyrate synthesis pathways by analyzing (meta)genomic data. MBio 5, e00889. doi:10.1128/mBio.00889-14
Wachenheim, D. E., and Patterson, J. A. (1992). Anaerobic production of extracellular polysaccharide by Butyrivibrio fibrisolvens nyx. Appl. Environ. Microbiol. 58, 385–391. doi:10.1128/aem.58.1.385-391.1992
Walker, A. C., Bhargava, R., Vaziriyan-Sani, A. S., Pourciau, C., Donahue, E. T., Dove, A. S., et al. (2021). Colonization of the Caenorhabditis elegans gut with human enteric bacterial pathogens leads to proteostasis disruption that is rescued by butyrate. PLoS Pathog. 17, e1009510. doi:10.1371/journal.ppat.1009510
Warnick, T. A., Methé, B. A., and Leschine, S. B. (2002). Clostridium phytofermentans sp. nov., a cellulolytic mesophile from forest soil. Int. J. Syst. Evol. Microbiol. 52, 1155–1160. doi:10.1099/ijs.0.02125-0
Watanabe, M., Kaku, N., Ueki, K., and Ueki, A. (2016). Falcatimonas natans gen. nov., sp. nov., a strictly anaerobic, amino-acid-decomposing bacterium isolated from a methanogenic reactor of cattle waste. Int. J. Syst. Evol. Microbiol. 66, 4639–4644. doi:10.1099/ijsem.0.001403
Whitman, W. B. (2009). Systematic bacteriology. New York, NY, USA: Springer. doi:10.1007/978-0-387-68489-5
Wolin, M. J., Miller, T. L., Collins, M. D., and Lawson, P. A. (2003). Formate-dependent growth and homoacetogenic fermentation by a bacterium from human feces: description of Bryantella formatexigens gen. nov., sp. nov. Appl. Environ. Microbiol. 69, 6321–6326. doi:10.1128/AEM.69.10.6321-6326.2003
Wu, C., Xu, W., Yu, W., Li, Z., Zhang, Y., and Zhang, F. (2021). Strain-level screening of human gut microbes identifies Blautia producta as a novel anti-hyperlipidemic probiotic via the production of 12-methylmyristic acid. https://www.researchsquare.com/article/rs-989302/v1.
Yamashita, H., Fujisawa, K., Ito, E., Idei, S., Kawaguchi, N., Kimoto, M., et al. (2007). Improvement of obesity and glucose tolerance by acetate in Type 2 diabetic Otsuka Long-Evans Tokushima Fatty (OLETF) rats. Biosci. Biotechnol. Biochem. 71, 1236–1243. doi:10.1271/bbb.60668
Yang, C., Guan, L., Liu, J., and Wang, J. (2015). Rumen fermentation and acetogen population changes in response to an exogenous acetogen TWA4 strain and Saccharomyces cerevisiae fermentation product. J. Zhejiang Univ. Sci. B 16, 709–719. doi:10.1631/jzus.B1500013
Zhang, J., Song, L., Wang, Y., Liu, C., Zhang, L., Zhu, S., et al. (2019). Beneficial effect of butyrate-producing Lachnospiraceae on stress-induced visceral hypersensitivity in rats. J. Gastroenterol. Hepatol. 34, 1368–1376. doi:10.1111/jgh.14536
Zhang, L., Li, G., Zhang, Y., Cheng, Y., Roberts, N., Glenn, S. E., et al. (2023). Boosting genome editing efficiency in human cells and plants with novel LbCas12a variants. Genome Biol. 24, 102. doi:10.1186/s13059-023-02929-6
Zhou, Y., Wei, Y., Jiang, L., Jiao, X., and Zhang, Y. (2023). Anaerobic phloroglucinol degradation by Clostridium scatologenes. mBio 14, e0109923–23. doi:10.1128/mbio.01099-23
Keywords: Lachnospiraceae, microbial biotechnology, microbiome, biocatalyst, live biotherapeutics, Clostridia
Citation: Zaplana T, Miele S and Tolonen AC (2024) Lachnospiraceae are emerging industrial biocatalysts and biotherapeutics. Front. Bioeng. Biotechnol. 11:1324396. doi: 10.3389/fbioe.2023.1324396
Received: 19 October 2023; Accepted: 05 December 2023;
Published: 04 January 2024.
Edited by:
Donatella Cimini, University of Campania Luigi Vanvitelli, ItalyReviewed by:
Zhengming Zhu, Nanjing Tech University, ChinaZhenshang Xu, Qilu University of Technology, China
Copyright © 2024 Zaplana, Miele and Tolonen. This is an open-access article distributed under the terms of the Creative Commons Attribution License (CC BY). The use, distribution or reproduction in other forums is permitted, provided the original author(s) and the copyright owner(s) are credited and that the original publication in this journal is cited, in accordance with accepted academic practice. No use, distribution or reproduction is permitted which does not comply with these terms.
*Correspondence: Andrew C. Tolonen, atolonen@genoscope.cns.fr