- 1Chemical Engineering Department, University of Vigo, Ourense, Spain
- 2CINBIO, University of Vigo, Vigo, Spain
- 3Department of Statistics and Operations Research, University of Vigo, Ourense, Spain
- 4Faculty of Pharmacy and Food Science, Physiology Section, Department of Biochemistry and Physiology, University of Barcelona (UB), Barcelona, Spain
- 5Nutrition and Food Safety Research Institute (INSA-UB), Barcelona, Spain
Mixtures of xylooligosaccharides (XOS) were manufactured from Eucalyptus nitens samples by hydrothermal processing. In order to obtain a product suitable to be used as a prebiotic, the liquors obtained were subjected to a refining sequence consisting of a two-step membrane filtration followed by anion exchange and freeze-drying. The process proposed allowed to obtain a highly refined product mainly made up of a mixture of substituted XOS with a degree of polymerization, DP3–10, which was evaluated for its prebiotic potential by in vitro fermentation assays. Their effects on the microbiota composition and the metabolic activity were assessed along the fermentation time and compared to fructooligosaccharides (FOS, a gold standard prebiotic), using fecal inocula from donors belonging to two age-groups (young and elderly). Significant and similar increases were observed in most of the bacterial groups considered (including Bifidobacterium spp. or several butyrate-producers) in both XOS and FOS in vitro interventions, although XOS resulted in significantly higher increases in total bacteria and lower rises in Clostridium clusters I and II than FOS. Regarding the metabolic activity, higher amounts of total organic acid (TOA; 150 vs. 110 mM) and higher total short-chain fatty acid (SCFA)/TOA ratio (0.88 vs. 0.70 mol/mol) were achieved at 28 h using XOS as a carbon source in comparison with FOS. Moreover, both substrates resulted in different metabolite profiles. Higher percentages of acetate and propionate were achieved when XOS were used as substrates, whereas FOS resulted in slightly higher concentrations of butyrate. No differences were found between both age-groups. Taking together these results, it can be concluded that XOS produced from E. nitens by a biorefinery-based approach led to, at least, similar prebiotic activity as that observed with FOS.
Introduction
Even though the premise “we are what we eat” has been accepted around the world for more than 100 years, it only became an empirically demonstrated fact some decades ago because of the latest scientific advances in nutritional research. Diet has shown to play a crucial role in human gut microbiota development and establishment, and in its metabolic activity, having a direct impact on both human health and overall well-being (Albenberg and Wu, 2014; Voreades et al., 2014; Sonnenburg and Bäckhed, 2016). In view of these facts, several scientific efforts have been focused on studying diet as a potential tool for gut microbiota modulation aiming the host health improvement (Moles and Otaegui, 2020; Myhrstad et al., 2020).
In this context, elderly people (population which is continuously increasing) could be a suitable candidate for nutritional interventions, considering the relevant gut microbial shifts observed in this community and an increased vulnerability and frailty or the appearance of health alterations, such as inflammaging, immunosenescence, malnutrition, or metabolic syndrome, which strongly affect their life quality (Biagi et al., 2010; Merchant et al., 2016; Buford, 2017; O'Connor et al., 2017; Salazar et al., 2017, 2019).
Among dietary fiber compounds, oligosaccharides have been extensively explored as substrates to improve elderly health status throughout the gut microbial modulation (Salazar et al., 2014, 2017; O'Connor et al., 2017). Xylooligosaccharides (XOS) are prebiotic candidates made up of D-xylopyranosyl units (D-xylose) bounded through β(1→ 4)-xylosidic linkages that can include side chain substituents, such as arabinose, uronic acids, phenolic acids, and acetyl or methyl groups (Samanta et al., 2015). These oligomers can be manufactured from several biomass sources by using chemical and/or enzymatic technologies, followed by a sequence of fractionation and/or purification stages including membrane filtration, enzymatic tailoring, or ion exchange (Vázquez et al., 2000; Samanta et al., 2015; Poletto et al., 2020; Santibáñez et al., 2021). Both raw material and manufacture technology can affect the XOS composition and structure, therefore their effects on the gut microbiota and its metabolic activity (Samanta et al., 2015; Karlsson et al., 2018).
The use of lignocellulosic materials to produce XOS is of great interest, not only because it can reduce the final product costs but also due to its contribution toward a more sustainable economy by adding value to underutilized biomass (Amorim et al., 2019). Some XOS manufactured from agro-industrial by-products (such as corncob or sugarcane) are already available in the market and count with positive opinions from food safety authorities such as the Food and Drug Administration (FDA) or the European Food Safety Authority (EFSA) (Poletto et al., 2020).
Particularly, valorization of biomass from forestry industries, such as Eucalyptus wood from several species, has been gaining attention for XOS production (Vázquez et al., 2005; Penín et al., 2019b; Cebreiros et al., 2020; Neiva et al., 2020; Neto et al., 2020) due to certain advantages of these materials in comparison with agro-industrial by-products, such as a non-seasonal character, large availability, and their ability to grow in non-food cultivable lands (Penín et al., 2020). The particular interest of the species E. nitens as raw material for XOS production, in comparison with other species such as Eucalyptus globulus, resides in the following key aspects: (i) E. nitens is a fast growing species that, unlike E. globulus, is able to grow in frosting zones (Penín et al., 2019a); (ii) it is more resistant than E. globulus to certain plagues such as Gonipterus scutellatus (Pérez et al., 2006); and (iii) the physical, chemical, and structural properties of E. nitens limit its use in pulp and paper industries, so the development of valorization alternatives for the huge amounts of wood produced is of great interest.
This work aimed to contribute to the development of a new biorefinery process for this species, formerly proposed by Penín et al. (2019b), where E. nitens wood chips are first subjected to a hydrothermal treatment optimized for XOS solubilization in the liquid stream. The exhausted solid from the autohydrolysis treatment could be further subjected to organosolv delignification, obtaining as a result of a cellulose-enriched solid (suitable for the manufacture of biobased compounds) (Penín et al., 2019a), and the sulfur-free lignin has potential applications related to the production of materials and polymers (Penín et al., 2019a, 2020).
In summary, this work deals with: (i) the manufacture of XOS mixtures by hydrothermal treatment of E. nitens wood chips; (ii) the purification of the resulting liquors by a sequence of membrane filtration and ion exchange; (iii) the chemical and structural characterization of the refined product; and (iv) the evaluation of their prebiotic potential (including the effects on both microbiota composition and functionality) by in vitro fermentation assays using gut microbiota samples from elderly and young volunteers in comparison to the commercial fructooligosaccharides (FOS) as positive control.
To the best of our knowledge, this is the first time that XOS purified from E. nitens biomass are purified, fully characterized, and evaluated for their prebiotic potential in vitro.
Materials and Methods
Manufacture and Purification of XOS From Eucalyptus nitens
Eucalyptus nitens wood chips were obtained from a local pulp mill (Ence, Pontevedra, Spain), milled to a particle size lower than 1 mm, air-dried, homogenized in a single lot to avoid variations in composition and stored until use.
Hydrothermal Processing of Wood Chips
The manufacture scheme employed to obtain XOS from E. nitens is shown in Figure 1. Wood chips were treated with hot compressed water under non-isothermal conditions in a 3.75-L stirred stainless steel pressurized reactor (Parr Instrument Company, Moline, IL, USA). The operational conditions used were selected according to the data reported by Penín et al. (2019) for the highest xylan conversion into XOS using E. nitens as raw material. Briefly, wood samples and distilled water were introduced into the reactor at a liquid-to-solid ratio (LSR) of 8 kg/kg [oven-dry (o.d.) wood basis], heated up to a maximum temperature of 195°C (corresponding to a severity of 3.62), and immediately cooled. Then, the liquid phase (autohydrolysis liquors) was recovered by centrifugation and analyzed as described in section Analytical Methods.
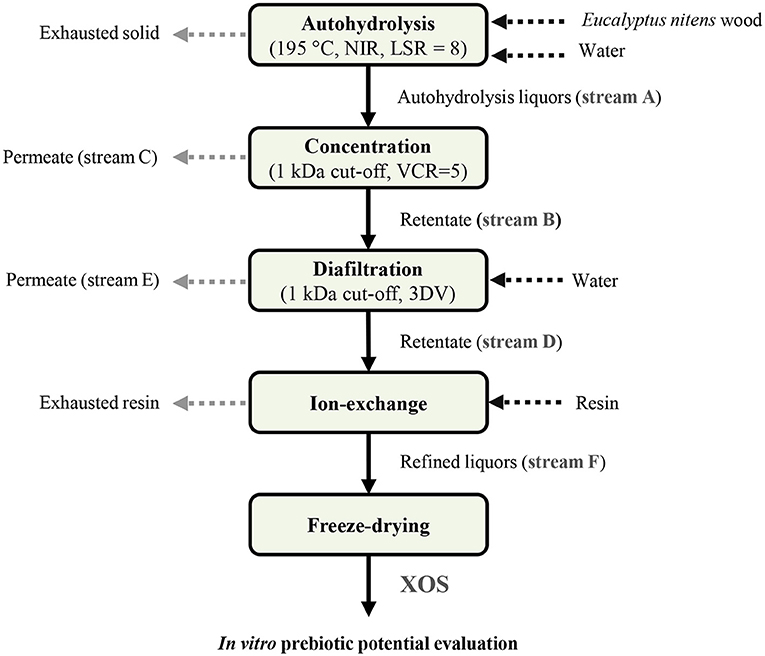
Figure 1. Process diagram employed for the manufacture of high-purity xylooligosaccharides (XOS) from E. nitens. NIR, non-isothermal regime; LSR, liquid-to-solid ratio [expressed as kg/kg (oven-dried wood basis)]; VCR, ratio between the volumes of feed and concentrate streams; DV, diafiltration volumes (1 DV = 1 ml of added water and permeated per ml of feed stream).
Membrane Processing: Nanofiltration
To reduce the concentration in monosaccharides and other undesired low molecular weight compounds, the autohydrolysis liquors containing the target XOS were refined employing a membrane filtration system at a mini-pilot scale. This system consisted of a 25-L feed tank accounting with a refrigeration device, a regenerated cellulose spiral-wound filter module of 1 kDa cut-off, and a filtration area of 5400 m2 (reference TFF6, Millipore, Burlington, MA, USA), a diaphragm pump, two pressure gauges, a needle valve, and a flowmeter (Gómez et al., 2014). The process was carried out at room temperature and at a transmembrane pressure of five bar to enhance the oligosaccharides retention as observed in the preliminary experiments. The autohydrolysis liquors (stream A in Figure 1) were first subjected to a concentration step at a volume–concentration ratio (VCR, defined as the ratio between the feed and the concentrate streams volume) of five. The resulting retentate (stream B) was then diafiltrated under discontinuous mode up to three diafiltration volumes (DV; 1 DV = 1 ml of added and permeated water/ml feed stream). The composition of the streams was determined as described below (see section Analytical Methods) and the final retentate (stream D) was subjected to an additional purification step using an ion exchange resin.
Ion Exchange
A weak anion exchange resin (Amberlite IRA-96 in OH− form, Sigma-Aldrich, Madrid, Spain) was then used at a liquid-to-resin mass ratio (LRR) of 30 g/g for increasing the purity of the stream D by removing, preferentially, undesired non-sugar compounds. The resins were mixed with stream D and left overnight at room temperature and under low-speed stirring. Refined liquors (stream F) were analyzed as described in section Analytical Methods, freeze-dried, and stored until use as substrates in the in vitro fermentation experiments.
In vitro Batch Experiments
Fecal Samples and Inocula Preparation
Three young (average age 40 years) and three elderly (average age 80 years) were recruited as donors of fecal samples for this work. All of them signed an informed consent to participate in this study and declared to not have consumed antibiotics, pre- or pro-biotics at least 3 months before fecal sample donation. These volunteers followed a normal omnivorous diet, were in a healthy status, and did not have any history of gastrointestinal or severe illnesses. Freshly voided feces were self-collected by the donors in sterile containers and then transferred to an anaerobic cabinet (filled with an anaerobic gas mixture consisting of 5% H2, 5% CO2, and 90% N2; Model Bactron II, Shel-Lab, Cornelius, OR, USA) and processed within 2 h after the collection. Fecal inocula were prepared following the protocol described by Gullón et al. (2011b). In short, fecal samples were diluted in a reduced physiological salt solution (0.5 g/L cysteine–HCl and 8.5 g/L NaCl) in a ratio of 10% w/v, and then the pH was adjusted to a value of 6.8. All experiments have been made in compliance with the relevant laws and institutional guidelines.
In vitro Fermentation Assays
The composition of the basal medium, which was prepared in NaOH 100 mM, is summarized in Supplementary Table 1. Solutions S1–S4 were prepared and stored at 4°C. The appropriate volumes of S1, S2, S3, and S4 (1, 1, 0.2, and 0.2% v/v, respectively), and amounts of tryptic soy broth and bactopeptone were added to the NaOH solution considering the desired final volume of fermentation media. This solution was deoxygenated overnight using a gas stream of N2, and then the cysteine–HCl was added to the solution after a pH adjustment to 6.8. The fermentation media was then transferred to airtight anaerobic serum bottles and sterilized in the autoclave.
Yeast nitrogen base (YNB; Becton Dickinson, Amersham, Buckinghamshire, UK), XOS, and commercial FOS from chicory (Sigma-Aldrich Co., St. Louis, MO, USA) stock solutions were prepared under anaerobic conditions, sterilized by filtration (Sartorius Minisart NML, Sartorius, Madrid, Spain) and transferred to sterile airtight serum bottles. Subsequently, YNB and either XOS or FOS were added to the anaerobic culture vessels to achieve a final concentration of 5 g/L of YNB and 8.0 g/L of either XOS or FOS. Finally, bottles were inoculated with the fecal slurry from the different donors (2% v/v) and incubated at 37°C for 28 h without stirring. At selected fermentation times (0, 8, and 28 h), each vessel content was gently homogenized, and then samples were withdrawn using sterile syringes for metabolite and microbiota analyses. Fermentation samples were centrifuged at 5,000 × g for 5 min, and the supernatants filtered through 0.22-μm sterile filters and analyzed by high-performance liquid chromatography (HPLC) as described in section Quantification of Bacterial Metabolites and Oligosaccharides in Fermentation Broths. All experiments were carried out in duplicate, and all additions and inoculations were performed in aseptic and anaerobic conditions.
Microbiota Analysis by Fluorescence In situ Hybridization–Flow Cytometry
Samples from fermentation vessels (375 μl) were taken at the corresponding fermentation times (0 and 28 h) and processed as per Gullón B. et al. (2011) for bacterial analyses. In short, cells were recovered by centrifugation (15,000 × g, 2 min), resuspended in 375 μl of phosphate-buffered saline (PBS, pH 7.2), and fixed overnight at 4°C after adding three volumes of ice-cold 4% (w/w) paraformaldehyde (PFA) solution. Afterward, cells were washed twice with PBS, resuspended in 1:1 (v/v) PBS: absolute ethanol (1:1 v/v), and stored at −20°C until further analysis.
The fluorescent in situ hybridization coupled with flow cytometry (FISH–FCM) analysis carried out to assess the differences among samples from batch cultures involved the use of fluorochrome-conjugated probes (Eurogentec S.A., Seraing, Belgium) targeting specific 16S rRNA sequences of either bacterial groups or specific bacterial genus/strains with gut physiological relevance (Supplementary Table 2). These probes were labeled at the 5′-end with either indodicarbocyanine (Cy5) or indocarbocyanine (Cy3) fluorochromes. Specific bacteria enumeration in fermentation samples was carried out by combining the positive events obtained using Cy5 or Cy3 probes, and total bacterial numbers were measured employing the nucleic-acid-binding dye SYBR green (Invitrogen, Carlsbad, CA, USA).
Samples from fermentation vessels containing fixed bacteria were hybridized as described by Grimaldi et al. (2017) with some modifications. In short, an initial volume of 65 or 40 μl of fixed cell samples was used for t = 0 h and t = 28 h, respectively. After centrifugation, samples were treated for cell permeabilization with 1 mg/ml lysozyme [≥40,000 U/mg protein; (Sigma-Aldrich, Madrid, Spain) diluted in a 1:1 solution of 1 M Tris–HCl, and 0.5 M ethylenediaminetetraacetic acid (EDTA, pH 8.0)] for 10 min at room temperature. The cells were then washed using PBS and resuspended in 1 ml of hybridization buffer [0.9 M NaCl, 20 mM Tris–HCl (pH 8), 30% formamide, distilled water, and 0.01% sodium dodecyl sulfate (SDS)]. Aliquots of 50 μl of each sample were mixed with 4 μl of each probe (Supplementary Table 2) and hybridized at 35°C overnight. Later, cells were centrifuged, and the cell pellets were washed using 200 μl of washing buffer [64 mM NaCl, 20 mM Tris–HCl (pH 8), 0.5 mM EDTA (pH 8), distilled water, and 0.01% SDS], and incubated for 20 min at 37°C. The cell pellets were then recovered by centrifugation, resuspended in either 100 or 200 μl (t = 0 h or t = 28 h, respectively) of PBS, and stored at 4°C until flow cytometry analysis. A Cytoflex S flow cytometer (Beckman Coulter, Indianapolis, IN, USA) was employed for bacterial identification and quantification by FISH–FCM. The SYBR green, Cy5, and Cy3 stainings were detected using the 488 nm, 638 nm, and 561 nm lasers; and 525/40 nm, 660/10 nm, and 610/20 nm filters, respectively. Up to 50,000 events per sample were recorded at a low flow rate (15 μl/min). The dilution factors employed along the process and the number of events/μl obtained in the corresponding sample control stained with the SYBR green were considered to calculate the number of a given specific bacteria or bacterial groups. All solutions used along the flow cytometry sample preparations were filtered through sterile 0.22-μm pore membranes.
Quantification of Bacterial Metabolites and Oligosaccharides in Fermentation Broths
To determine the fermentation metabolites produced by the intestinal bacteria during fermentation experiments, the supernatants recovered from fermentation vessels at t = 0 h, t = 8 h, and t = 28 h were filtered through 0.22-μm cellulose acetate filters and analyzed by HPLC following the method as described in section High-Performance Liquid Chromatography. Short-chain fatty acids (SCFA; acetate, propionate, and butyrate), intermediate products (lactate, succinate, formate, and valerate), and branched-chain fatty acids (isovalerate and isobutyrate) were identified and quantified based on commercial standards. In addition, to determine the consumption of oligosaccharides, an aliquot of each sample was subjected to an acid posthydrolysis and further analyzed by HPLC as described below (see section High-Performance Liquid Chromatography).
Analytical Methods
Non-volatile Compounds
Non-volatile compound (NVC) content in liquid streams was quantified as described by Gullón et al. (2011a) by oven-drying samples at 105°C until constant weight (usually, after 24 h). The difference between the NVC and total saccharides (calculated as the sum of monosaccharides, oligosaccharides, and acetyl and galacturonyl substituents) was defined as other non-saccharide, non-volatile compound (ONVC).
High-Performance Liquid Chromatography
Aliquots of liquid streams A, B, D, and F (see Figure 1) were filtered through 0.45-μm cellulose acetate filters and analyzed by HPLC (Agilent 1,200 series, Agilent, Waldbronn, Germany) to determine monosaccharides, galacturonyl substituents, and acetic acid and to evaluate the presence of sugar degradation products (particularly furfural and 5-hydroxymetyl furfural) using an Aminex HPX-87H column (from Bio-Rad, Hercules, CA, USA) working at 50°C, a H2SO4 3 mM solution as mobile phase (flow rate 0.6 ml/min) and a refractive index (RI) detector for the analyte identification. Another aliquot of each stream was subjected to a posthydrolysis with a 4% of H2SO4 at 121°C for 30 min, and the resulting hydrolysate was analyzed by HPLC as described above. The increase in monosaccharides, galacturonic acid, and acetic acid concentration after posthydrolysis allowed the calculation of the oligomer concentration in the samples and the degree of substitution by galacturonyl and acetyl groups. Oligosaccharide concentrations are expressed as monosaccharide equivalents, and the content of acetyl and uronyl substituents is expressed in terms of acetic and uronic acid equivalents, respectively (Gullón et al., 2011a). These analyses were performed by triplicate.
Ultraperformance Liquid Chromatography–Diode Array Detector–Electrospray Ionization Mass Spectrometry
Streams A and F were analyzed by ultraperformance liquid chromatography–diode array detector–electrospray ionization mass spectrometry (UPLC–DAD–ESI MS) as described by Dávila et al. (2019) with some modifications. Briefly, methanol was used to dissolve the sample up to ~0.5 g/L, and 5 μl of this solution was injected in a UPLC system (Proxeon EASY-nLC 1000 UHPLC, Thermo Fisher Scientific, Bremen, Germany) fitted with a diode array detector. The operational conditions were the following: temperature of 40°C, C18 analytical column (Zorbax Eclipse XDB-C18, 100 × 2.1 mm, 1.8 μm particle size from Agilent, Waldbronn, Germany), two mobile phases (A, 0.1% formic acid, v/v and B, methanol), and flow rate of 0.3 ml/min. The elution gradient was in the sequence: 0–2 min, 99% of A; 3–32 min, 99% of B; 32–40 min, 99% of B; 40–41 min, 99% of A; and 41–45, 99% of A. UV spectra were recorded from 200 to 500 nm, whereas chromatograms were registered at 254, 280, and 320 nm using an LTQ-Orbitrap ELITE (Thermo Fisher Scientific, Bremen, Germany) fitted with an electrospray ionization (ESI MS) interface for the MS analysis. Scans from m/z 250 to 2,000, capillary voltage of 2,000 V, and cone voltage of 100 V in positive ionization mode were used to perform these analyses.
Fourier Transform Infrared Spectroscopy
The Fourier transform infrared (FTIR) spectra of streams A and F were obtained employing a Thermo Nicolet 6,700 equipment (Thermo Fisher Scientific, Bremen, Germany) with an attenuated total reflection (ATR) diamond accessory. Spectra were recorded from 4,000 to 400 cm−1 (34 scans/sample, resolution of 4 cm−1).
Matrix-Assisted Laser Desorption Ionization/Time of Flight Mass Spectrometry
Streams A and F were further characterized by matrix-assisted laser desorption ionization/time of flight mass spectrometry (MALDI–TOF MS). These analyses were performed using an Autoflex III Smartbeam workstation (Bruker Daltonics, Bremen, Germany) equipped with a 337-nm nitrogen laser and were carried out in a linear positive ion mode as indicated by Gullón et al. (2010). In short, for sample analysis, a 1:1 solution consisting of a desalted sample solution (1 mg/ml) and a matrix solution [10 mg/ml 2,5-dihydroxybenzoic acid in 30% acetonitrile (v/v)] was applied on the MS target plate and air-dried. After a delayed extraction time of 150 ns, ions were accelerated with a 25 kV voltage, and data were collected from 100 laser shots at the lowest energy needed to obtain enough spectral intensity. The MS was calibrated with a mixture of XOS (degree of polymerization, DP 2–6) from Megazyme, Barcelona, Spain. Flex Control 3.0 and Flex Analysis 3.0 (Bruker Daltonik, Bremen, Germany) software applications were used for the sample spectra acquisition and treatment. All determinations were made, at least, by duplicate.
Statistical Analyses
In order to assess the significance of the variables: substrate, age-group, donor, and fermentation time, a statistical modeling based on the use of a linear mixed model was carried out (Zuur et al., 2009). The logarithmic transformation of data (or squared root when the existence of zero values was observed) was applied to the following dependent variables: oligosaccharide concentration in fermentation media, organic acids concentration, total bacterial numbers, and microbiota group concentration at t = 0 h (basal microbiota, BM) and t = 28 h composition. Within this approach, the donor factor was considered as a random component due to the lack of interest in the individual effect of a given donor and because the selection of donors was also a random process. Substrate (FOS/XOS), age-group (young/elderly), and their interactions were considered as fixed components. The variable “time” was included as a quadratic global term and with a random slope when more than two values were observed (substrate consumption or metabolite production). When just two values were available (microbiota composition), “time” was analyzed as a factor type variable. The estimation of the model was carried out in two stages: (i) the random effect was selected maximizing the restricted log-likelihood REML; and (ii) the fixed part was selected maximizing the log-likelihood. Finally, the model achieved was estimated using the REML. The statistical analyses were carried out using the free software R version 4.03 (R Core Team, 2020) and the nlme package (Pinheiro et al., 2020). Differences between models were considered significant when p < 0.05.
Results and Discussion
Raw Material Composition
Samples of E. nitens wood were characterized according to the standard protocols as those employed by Penín et al. (2019b). The E. nitens lot employed in this work contained (expressed as g/100 g dry wood): cellulose (42%), xylosyl units (14.7%), arabinosyl units (0.46%), acetyl groups (2.9%), uronyl groups (4.21%), Klason lignin (21.4%), extractives (4.68%), protein (0.52%), and ashes (0.25%). This composition is in close agreement with the results recently reported by Penín et al. (2019a,b).
Xylooligosaccharides Production and Characterization
Figure 1 summarizes the processing scheme employed in this work for the manufacture of XOS mixtures from E. nitens. This approach, where XOS-rich liquors can be obtained from the hemicelluloses fraction, is complementary with the process previously developed by Penín et al. (2019a,b), dealing with the integral fractionation and valorization of E. nitens wood following the biorefinery concept.
The composition of the liquid phase from the hydrothermal treatment (autohydrolysis liquors, stream A) is shown in Table 1. As can be seen, the substituted XOS fraction (calculated as the sum of XOS, acetyl groups, arabinose, and galacturonyl substituents) was the main component accounting for up to 57.6% of NVC (o.d. basis). Sugar degradation products were not detected under the autohydrolysis optimal conditions employed in this work.
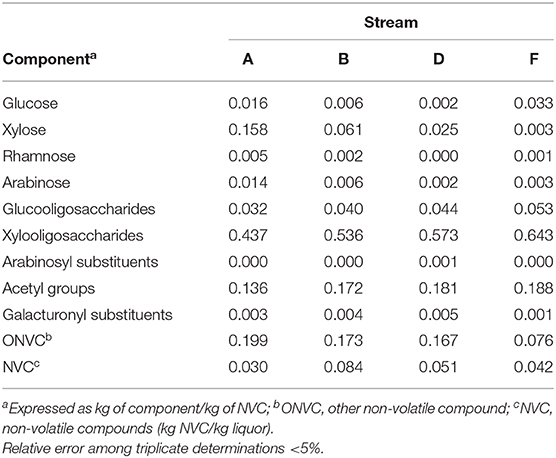
Table 1. Composition of the streams A, B, D, and F (see Figure 1).
In addition to the hemicelluloses breakdown, a variety of side undesirable processes take place during the autohydrolysis, and as a consequence, liquors containing complex mixtures of saccharides and non-saccharide components are obtained as a product (Vázquez et al., 2005). In this case, monosaccharides and ONVC represented up to 19.3 and 19.9%, respectively, of the dry content of liquors. This makes necessary the application of a sequence of purification steps to obtain a final product suitable for food and/or pharmaceutical uses (particularly as a prebiotic substrate). Based on previous experience in this field (Míguez et al., 2018), a sequence of three subsequent refining steps was applied to XOS-enriched liquors under fixed operational conditions.
Among the downstream processing strategies for the industrial production of highly purified oligosaccharides, membrane technology has been recently described as one of the most promising and sustainable approaches (Santibáñez et al., 2021). This technology includes certain advantages such as low energy requirements, easy modification of the critical operational variables, and relatively simple scale-up (Pinelo et al., 2009). In this work, two sequential membrane filtration stages (concentration followed by diafiltration) were employed for both removing monosaccharides and low molecular weight impurities from liquors (see Figure 1) and increasing the product concentration. This strategy significantly reduced the monosaccharides (from 0.193 to 0.029 g/g NVC) and ONVC content (from 0.199 to 0.167 g/g NVC), whereas increased the substituted-XOS percentage. Then, the resulting liquors (stream D, see Figure 1) were further subjected to an anion exchange process to reduce the presence of undesirable compounds, including low-molecular-weight phenolics, hemicellulose degradation products, charged organic compounds, or pigments (Santibáñez et al., 2021). The ion exchange stage led to a high ONVC removal, decreasing its value from 0.167 to 0.076 g/g, which represents a reduction of 98% of the impurities contained in stream A. Simultaneously, a significant raise of the substituted XOS content was achieved in stream F, reaching a value of 83.15% of the final refined product (o.d. basis). Overall, the manufacturing approach followed in this work allowed to recover 38% of the initial hemicelluloses content in E. nitens wood as XOS. Similar refining approaches were previously applied for the purification of XOS-enriched liquors obtained by the hydrothermal treatment of a variety of raw materials also showing a high refining efficiency (Gullón et al., 2011b; Míguez et al., 2018; Álvarez et al., 2020).
However, it can be deduced that the acetic acid/xylose molar ratio in the oligosaccharides fraction varied in the range 0.73–0.80 mol/mol, which results in an acetylation pattern of, approximately, four acetyl groups/five xylose units.
The molecular weight of the XOS [and also arabinoxylooligosaccharides (AXOS)], their DP, and substitution patterns have demonstrated to influence in their effect on the gut microbiota and also in the consumption rate and in the amount of organic acids produced (Singh et al., 2015; Ho et al., 2018; Míguez et al., 2018; Álvarez et al., 2020). To gain more insight into the structural features of the oligosaccharides present in crude liquors (stream A) and the refined product (stream F) and also to evaluate the effects of the manufacturing process, several analytical techniques (including FTIR, MALDI–TOF MS, and UPLC–DAD–ESI MS) were used in this work.
Fourier transform infrared has been widely employed for the identification of the functional groups present in the oligosaccharides molecular structure based on both the position and intensity of the bands in the FTIR spectrum. The spectra obtained from streams A and F (see Figure 2A) showed a typical pattern of hemicelluloses with signals consistent with those reported by Sun et al. (2004). For instance, a strong absorption band at 3,351 cm−1 (corresponding to hydroxyl groups), bands at 2,923 cm−1 (corresponding to stretch links CH as CH2 and CH3), a band at 1,734 cm−1 (which is related to ester groups such as acetyl and uronic groups), and strong bands between 1,300 and 1,000 cm−1 with a dominant peak at 1,033 cm−1 (related to C–O, C–C, C–OH, and C–O–C bonds), typical of XOS could be distinguished in the spectra. Moreover, the low intensity shoulders at 1,160 and 990 cm−1 revealed the presence of arabinosyl side chains (Buranov and Mazza, 2010).
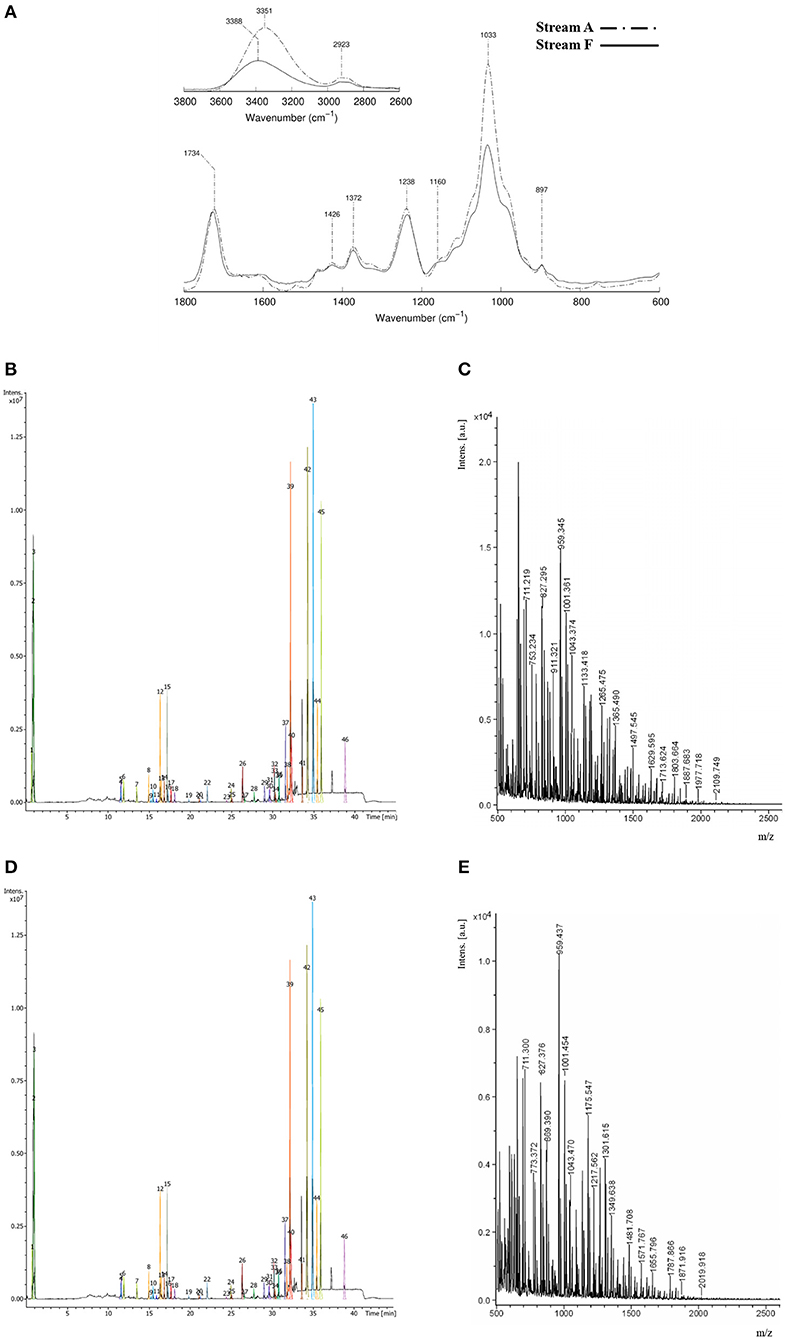
Figure 2. Structural characterization of autohydrolysis liquors and refined xylooligosaccharides (XOS)-enriched concentrate. (A) Fourier transform infrared (FTIR) spectra of stream A (autohydrolysis liquors) and stream F (refined XOS-enriched stream); (B,C) ultraperformance liquid chromatography–diode array detector–electrospray ionization mass spectrometry (UPLC–DAD–ESI MS) chromatogram and the matrix-assisted laser desorption ionization/time of flight mass spectrometry (MALDI–TOF MS) spectrum of stream A, respectively; and (D,E) UPLC–DAD–ESI MS chromatogram and MALDI–TOF MS spectrum of stream F, respectively.
The ESI MS and MALDI–TOF MS constitute powerful techniques for carbohydrate characterization, which allow the determination of the molecular mass of each component in a complex mixture.
The UPLC–DAD–ESI MS chromatograms from streams A and F (Figures 2B,D, respectively) show several peaks, which are mainly attributed to acetylated pentoses with DP3–6 (see Supplementary Table 3). Small loses of low DP oligosaccharides (DP2–3, partially acetylated) were observed from stream A to stream F (see peaks 7–32 in Figures 2B,D), and the peaks reaching the major area values were 1, 2, 3, 4, 54, 62, 63, and 65 in stream A (Figure 2B). A/The tentative peak identification in both streams based on the m/z values found in UPLC–DAD–ESI MS analyses is summarized in Supplementary Table 3. As it can be observed, the major area percentage of chromatograms corresponded to XOS with DP 5–6 with acidic substituents (mainly not only acetyl groups but also some uronic acids) and, in some cases, with one hexose on their structure.
Finally, DP of XOS in streams A and F was also analyzed by MALDI–TOF (see Figures 2C,E, respectively). Most of the oligomers found in the spectrum corresponded to adducts of sodium or potassium (see Supplementary Table 4). The main products obtained were oligomers made up of pentoses (mainly xylose), with a DP between 3 and 10. The analysis suggested that those oligomers were partially acetylated, and some of them had galacturonic substituents attached to the backbone. The most intense signals were assigned to a series of oligomers between 4 and 6 pentoses with a high degree of acetylation. This high degree of acetylation with some uronic substituent units is in accordance with the results obtained by UPLC–DAD–ESI MS and FTIR. Some differences have also been observed between the crude liquors (stream A) and the refined ones (stream F), both in the FTIR spectra (where a decrease in the peaks assigned to the hydroxyl groups was observed) and in UPLC–DAD–ESI MS, where some of the compounds present in the crude liquors were not found in the refined product (see Figure 2).
In summary, the manufacturing scheme used led to a refined product containing mainly substituted XOS with a DP in the range 3–10 as the main component (83.15%) mixed with glucooligosaccharides (GlcOS) as secondary constituents (5.3%); a composition in close agreement with some commercial XOS currently available in the market (Hong et al., 2019).
In vitro Fermentation Assays
The prebiotic potential of the refined XOS was assessed by in vitro fermentation assays using fecal inocula from volunteers belonging to two different age-groups: young (donors denoted as YA, YB, and YC) and elderly (donors ED, EE, and EF). Oligosaccharide consumption rate, metabolites production, and changes in microbiota bacterial populations were determined in this work, and the results are discussed along the following sections.
Xylooligosaccharides and GlcOS Consumption
Figure 3 shows the time course of GlcOS and XOS consumption by the gut microbiota from young and elderly donors measured in XOS-containing fermentation vessels. Particularly, substituted XOS data were here calculated as the sum of XOS and acetyl groups content as the last ones are, in fact, substituents in these xylose chains as it was confirmed by UPLC and MALDI–TOF MS analyses.
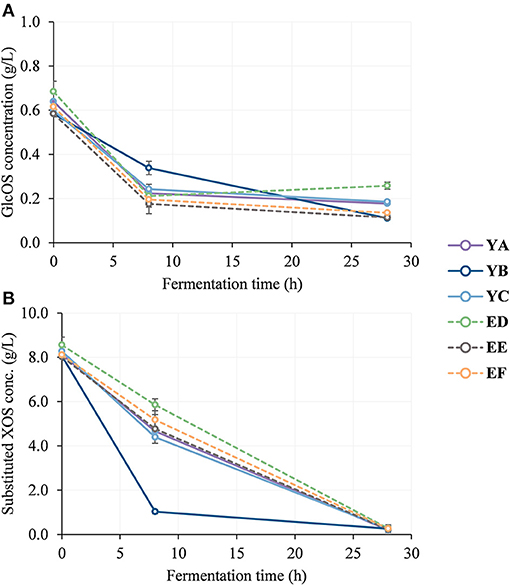
Figure 3. Time course of oligosaccharide consumption by gut microbiota from young (YA, YB, and YC) and elderly (ED, EE, and EF) donors up to t = 28 h of in vitro batch fermentation. (A) glucooligosaccharides (GlcOS) concentration (g/L) and (B) substituted xylooligosaccharides (XOS) concentration (g/L) measured in fermentation vessels at different fermentation times. Substituted xylooligosaccharides (XOS) concentration refers to the sum of XOS and acetyl groups concentrations measured in fermentation vessels. Error bars represent SDs between each donor fermentation experiments (n = 2).
As can be seen, GlcOS were mostly consumed during the period 0–8 h, whereas just 50% of substituted XOS were metabolized during this period, being the rest completely depleted after 28 h.
Even though no statistical differences were observed among age-groups with regard to oligosaccharide consumption rate, some variations among individual donors could be noticed (markedly donor YB).
Overall, the XOS consumption rates observed were slightly lower than those observed previously for XOS obtained from rice husks (Gullón et al., 2010) or AXOS from brewer's spent grain (BSG; Gómez et al., 2015), although in these works higher amounts of unconsumed XOS remained in the fermentation media. This is probably related to the differences in the chemical composition, the DP, and/or other structural features, which not only affect the gut microbial composition and metabolism but also to their utilization by the bacteria (Singh et al., 2015). Furthermore, the differences observed among donors at 8 h disappeared after 28 h of fermentation, as it was observed by Gullón et al. (2014) at 33 h using AXOS as substrate.
Effects on Gut Microbial Composition
The intestinal microbiota changes with age, and these modifications (mainly higher abundances in proteolytic and lower in saccharolytic bacteria) have been related to morbidity and mortality in the elderly population, being prebiotics a potential strategy for delaying such alterations (Bischoff, 2016).
Despite the several works highlighting the differences between young and elderly microbiota composition and function (Woodmansey et al., 2004; Odamaki et al., 2016), overall under the conditions tested in this work, no significant differences were found between young and elderly age-groups or among donors on the various microbial parameters evaluated. Therefore, for simplicity, this would not be repeated throughout the following subsections and, unless otherwise stated, no statistical differences between age-groups or donors have been found.
Effects on the Total Bacterial Number
Figure 4A shows the average of total bacteria numbers (expressed as log10) measured at t = 0 h (BM) and after substrate (XOS or FOS) fermentation (t = 28 h). The consumption of both substrates led to a significant raise in total bacteria in comparison with the initial values. XOS led to significantly greater increases (p < 0.05) with respect to BM of total bacterial numbers and also in comparison with FOS (7.5 × 108 vs. 6.4 × 108 cells/ml). This is in agreement with the results obtained by Míguez et al. (2018) who already reported a higher increase in total bacteria in comparison with FOS when employing XOS manufactured from E. globulus as substrate in in vitro experiments using elderly microbiota as fecal inocula.
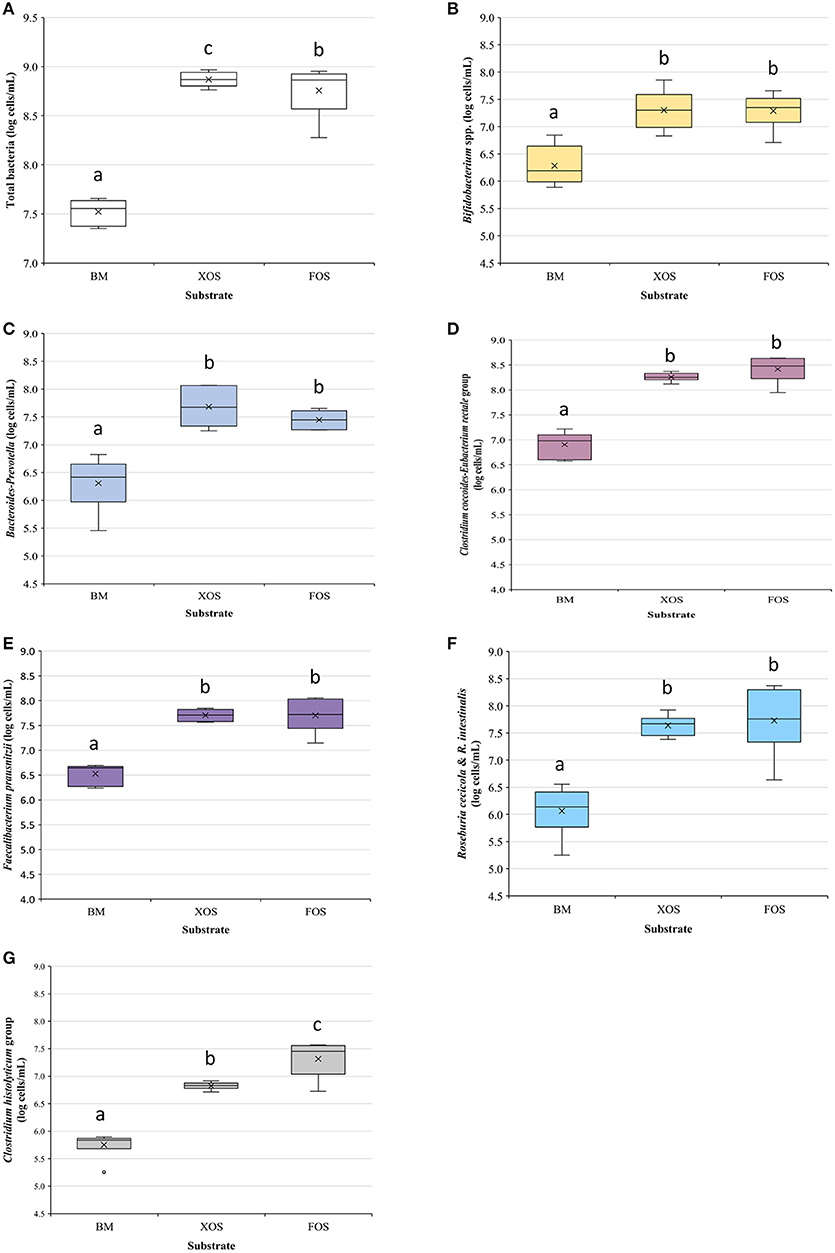
Figure 4. Average concentration (expressed as log cells/ml) in young and elderly age-groups of total bacteria and bacterial populations measured by fluorescent in situ hybridization coupled with flow cytometry (FISH–FCM) measured in fermentation vessels at t = 0 h (basal microbiota, BM) and t = 28 h to assess the effect of xylooligosaccharides (XOS) and fructooligosaccharides (FOS) in the gut microbiota. (A) Total bacteria (measured using SYBR green stain), (B) Bifidobacterium spp. (Bif164 probe), (C) Bacteroides–Prevotella group (Bac303 probe), (D) Clostridium coccoides–Eubacterium rectale group (Erec482 probe), (E) Faecalibacterium prausnitzii A2–165 and L2–6 (Fpra655 probe), (F) Roseburia cecicola and Roseburia intestinalis (Rint 623 probe), and (G) Clostridium histolyticum group (Clostridium clusters I and II) (Chis150 probe). Within the same box plot, average concentrations sharing the same letter (a, b or c) are not significantly different according to Tukey's post hoc test.
According to the statistical analysis, a significant effect of the donor was observed on the total bacterial number. In fact, donors A and D led to lower bacterial numbers when grown on FOS in comparison with the rest of the volunteers (data not shown). The FOS supplementation has previously shown to promote a larger variance in microbial responses in elderly volunteers between 65 and 80 years in comparison with other prebiotics, such as galactooligosaccharides (GOS) (Nyangale et al., 2014).
Effects on the Microbiota Composition
According to its scientific definition, a prebiotic is “a substrate that is selectively utilized by host microorganisms conferring a health benefit” (Gibson et al., 2017). With this regard, XOS have shown a greater selectivity than certain prebiotics, such as short-chain FOS (DP 2–8) or GOS, in experiments with pure cultures. The prebiotic beneficial effects might include changes in the microbiota composition and/or its metabolic activity (Gibson et al., 2004). Therefore, any preliminary assessment of the prebiotic potential of a given candidate should include the evaluation of not only microbial population shifts but also the effects on bacterial metabolic activity.
The fluorescent in situ hybridization coupled with flow cytometry has been demonstrated to be a reliable technique for the analysis of cell populations in fecal samples even though it does not allow the identification of unknown species (Fraher et al., 2012). This technique allows obtaining high-throughput multiparametric information of several bacterial groups/species of interest at the single cell level (Arrigucci et al., 2017; Ou et al., 2017). In addition, FISH–FCM permits the analysis of unlysed bacteria, which is not possible using, for example, quantitative PCR-based methods (Malinen et al., 2002) while yielding comparable abundance profiles in comparison with them (Vandeputte et al., 2017).
In this work, nine relevant microbiota bacterial groups were identified and quantified by FISH–FCM using 16S rRNA nucleotide probes (see Supplementary Table 2) to evaluate the changes promoted upon substrate consumption in gut microbiota populations. Figures 4B–G; Supplementary Figures S1A–C include the box and whisker plots showing the average bacterial concentrations expressed as log10[cells] measured in both age-groups using different fluorescently labeled probes.
Bifidobacterium Genus
Bifidobacterium spp. is one of the most important genus in the gut microbiota due to their capability for producing bacteriocins (Castillo Martinez et al., 2013), B vitamins, and for stimulating the immune system (Rivière et al., 2016), among other reasons. With respect to the elderly, this beneficial group was reported to be reduced with age, from 90% of the total microbiota in babies to 5% in the elderly (Rivière et al., 2016). In addition, a trend toward a lower presence of this bacterial population has been observed in Spanish elderly in comparison with middle-aged adults (Salazar et al., 2013). Moreover, recent clinical studies have also demonstrated, for instance, that bifidobacteria can regulate the gut microbiota improving the cognitive ability of patients with Alzheimer's disease (Lu and Wang, 2018).
Here, a tendency to lower bifidobacteria abundances with age was also found when comparing basal microbiotas from both age-groups (10.9 ± 6.0% vs. 3.5 ± 0.8% of the total bacteria in young and elderly, respectively).
In this context, the intake of adequate prebiotics could be a favorable strategy for increasing their abundance in the elderly. Figure 4B shows the initial bifidobacteria concentration and after XOS or FOS consumption (t = 28 h). As can be seen, XOS and FOS resulted in comparative Bifidobacterium spp. concentrations after 28 h of in vitro fermentation, showing similar bifidogenic effects. These results support the strong bifidogenic effect of XOS in comparison with FOS observed in vitro by other authors when used AXOS from BSG (Gómez et al., 2015), low-DP XOS from E. globulus (Míguez et al., 2018), or two different XOS fractions from barley straw (Álvarez et al., 2020).
Bacteroides–Prevotella Group
From a quantitative point of view, Bacteroides spp. and Prevotella spp. are two important genera in colonic microbiota, and two of the human enterotypes (enterotype 1 and 2) owe their names to these bacterial groups being both of them the most prevalent in the European population (Sebastián and Sánchez, 2018). The results obtained for the Bacteroidetes phylum in elderly people are variable. Some studies have reported lower levels in this population, whereas others have indicated increases of this group with age (Salazar et al., 2017). In this work, the elderly group showed a significantly higher average abundance of Bacteroides–Prevotella groups than young donors (11.3 ± 5.6% vs. 4.7 ± 3.0% of total bacteria, respectively), which is in agreement, for instance, with the increase with age reported for Bacteroides by Rondanelli et al. (2015).
No significant differences were found at t 28 h between the concentrations achieved in Bacteroides–Prevotella group using XOS or FOS as substrates, which demonstrates that both carbohydrates are suitable carbon sources for these genera. This is in agreement with other previous reported findings: Bacteroides spp. and Prevotella spp. have demonstrated capacity for degrading xylan and arabinoxylan and FOS (Basson et al., 2016). Significant increases in Bacteroides–Prevotella concentrations were reported by Gómez et al. (2015) when compared in vitro AXOS and FOS using fecal inocula from elderly donors or by La Rosa et al. (2019) using as substrates FOS, acetylated galactoglucomannan, and highly acetylated arabinoglucuronoxylan.
Butyrate-Producing Bacteria
Butyrate is an essential metabolite for human health as: (i) it is the preferred energy source for colon epithelial cells, (ii) it contributes to the maintenance of the gut barrier function (by the stimulation in the production of mucin, antimicrobial peptides, and tight-junction proteins); and (iii) it has immunomodulatory and anti-inflammatory properties (Rivière et al., 2016). This makes the butyrate-producing bacteria a group for which growth stimulation could have a great interest for elderly, especially taking into account that inflammaging pathology (a chronic, low-grade inflammation) is a common health issue in this population (Franceschi et al., 2018).
Butyrate-producing bacteria include Faecalibacterium prausnitzii (Clostridium cluster IV) and Eubacterium, Anaerostipes, and Roseburia species (Clostridium cluster XIVa), which can convert hexoses (such as glucose or fructose) and pentoses (such as arabinose and xylose) into butyrate, lactate, or formate being, therefore, essential targets for a prebiotic candidate. Moreover, other metabolites, such as acetate and lactate, generated by bifidobacteria or lactobacilli among others, can be also used as substrates for butyrate production by a cross-feeding interaction with this genus (Rivière et al., 2016). The species F. prausnitzii and Eubacterium rectale are abundant species in the human colon so that they are expected to have a significant contribution to butyrate production and preferentially colonize the mucus layer increasing the butyrate bioavailability for colon epithelial cells (Rivière et al., 2016).
The probe Erec482 allows quantifying the majority of bacteria belonging to the group Clostridium coccoides–Eubacterium rectale (Clostridium XIVa and XIVb clusters) (Franks et al., 1998). An average value of 19.4 ± 4.4% of the total bacteria was observed in the elderly before fermentation, whereas in younger donors, these groups reached higher values (31.1 ± 8.0%). Reduced levels of the Clostridium cluster XIV have been previously reported in the elderly (Rondanelli et al., 2015). Interestingly, the C. coccoides–E. rectale values found here in the elderly basal microbiota were more similar to that reported by Walker et al. (2014) for E. rectale in younger healthy adults living in France or in The Netherlands (12.9%) than to those found in the microbiota of Spanish young adults included in the present study. Significant increases in the number of these bacteria were observed after FOS or XOS fermentation, although no differences were found between both substrates (see Figure 4D), which is in agreement with the results reported by Gómez et al. (2016) who compared FOS and mixtures of sugar beet-derived oligomers rich in arabinooligosaccharides (AOS).
The probe Fpra655 was used for the determination of two specific F. prausnitzii strains (A2–165 and L2–6) (Hold et al., 2003). In addition to the general positive effects mentioned above for F. prausnitzii, the A2–165 strain has been shown to induce the production of interleukin IL-10 and to have anti-inflammatory effects toward colitis (Rossi et al., 2016). However, it has been reported that the abundance of F. prausnitzii decreases during adulthood and, especially, in the elderly (Miquel et al., 2014). Therefore, an increase in the presence of these bacteria within the intestinal environment is a desirable effect, mainly when the target population is of advanced age. Average values of 12.3 ± 2.4% and 8.6 ± 1.5% of total bacteria were observed in young and elderly BM, respectively, both being values in agreement with that reported (13.8%) by Walker et al. (2014) for adults of ages within the range of 29–54 years. In this work, both XOS and FOS consumption led to similar significant increases in the numbers of F. prausnitzii (Figure 4E). FOS have previously shown to support the growth of F. prausnitzii (Gómez et al., 2016) and, with regard to XOS, La Rosa et al. (2019) have suggested that certain F. prausnitzii strains present in the gut microbiota might be able to degrade XOS using different β-xylosidases/arabinosidases.
The Rint623 probe allows to identify species, such as Roseburia intestinalis and Roseburia cecicola (Hold et al., 2003). Figure 4F shows the average content of these bacteria in fermentation media at t = 0 h (BM) and after XOS or FOS consumption at 28 h. As for Erec482 and Fpra65 probes, significant increases of the bacterial concentration were observed after substrate fermentation with respect to the BM, leading to similar values as those reported by Gómez et al. (2016) when compared FOS and sugar beet-derived oligomers.
Clostridium Clusters I and II
The probe Chis150 was designed to identify bacteria belonging to Clostridium clusters I and II (Franks et al., 1998), which include some species that are mainly considered as harmful. Biagi et al. (2010) reported that even though Clostridium abundance is higher in centenarians, the increases in bacteria measured with this probe have often been considered a negative effect. After 28 h of XOS and FOS fermentation, significant increases were observed in Clostridium I and/or II with respect to BM (see Figure 4G). However, these increases were significantly lower when microbiota used XOS as a carbon source (p < 0.05). This effect is in agreement with the results reported by Gómez et al. (2015) when compared AXOS from BSG and FOS, although the differences found were not significant.
Other Bacterial Groups
Other bacterial groups (the ones quantified by using the probes Prop853, Enter1432, and Muc1437) showed initial average abundances in the range 0.4–2% of the total bacteria.
The Prop853 is the probe used for the determination of the number of bacteria belonging to the Clostridium cluster IX (Walker et al., 2005), which are mainly propionate producers (Basson et al., 2016). The organic acid propionate prevents the proliferation and induces apoptosis of colorectal cancer cells, interacts with the immune system, has anti-inflammatory effects, and also decreases the blood cholesterol levels, capacitating other positive effects (Rivière et al., 2016). Therefore, the increase in propionate producers should be considered a positive effect for a prebiotic candidate. In this work, significant increases in the final numbers of these bacterial groups were observed in the media after the XOS and FOS fermentation (see Supplementary Figure 1A).
Similar behavior was observed for Enterobacteriales (Enter1432 probe) and Akkermansia muciniphila (Muc1437 probe). Both XOS and FOS resulted in significant increases in these bacteria in comparison with BM (see Supplementary Figures 1B,C). An increase in Akkermansia numbers can be considered a beneficial outcome as different studies have observed higher abundance in this genus in the microbiota of long and super-long-lived elderly (Kong et al., 2016; Lu and Wang, 2018).
Taking these results into account, it can be confirmed that XOS led to, at least, similar outcomes in comparison with FOS. On the one hand, both led to similar changes in most of the bacterial groups considered, but XOS resulted in higher increases in total bacteria and lower increases in Clostridium clusters I and II (Chis150). On the other hand, no differences were found between young and elderly (<80 years old) donors in these assays. This could be related to the similarities of the initial microbiota of both age-groups, and this is in agreement with Biagi et al. (2010) who reported that there are no essential differences between young and elderly microbiota, and that the changes in their composition are more significant between elderly and centenarians, suggesting that the main shifts start at 75–80 years.
Effects on Metabolic Activity
The concentrations of the main SCFA and total SCFA measured in fermentation vessels at t = 8 h and t = 28 h are shown in Figure 5. For all these acids, no significant differences were found between the young and elderly groups. On the contrary, different acetic, propionic, butyric, and total SCFA concentrations were achieved, from a statistical point of view, when compared with the data obtained using different substrates (FOS vs. XOS) or fermentation times (8 and 28 h).
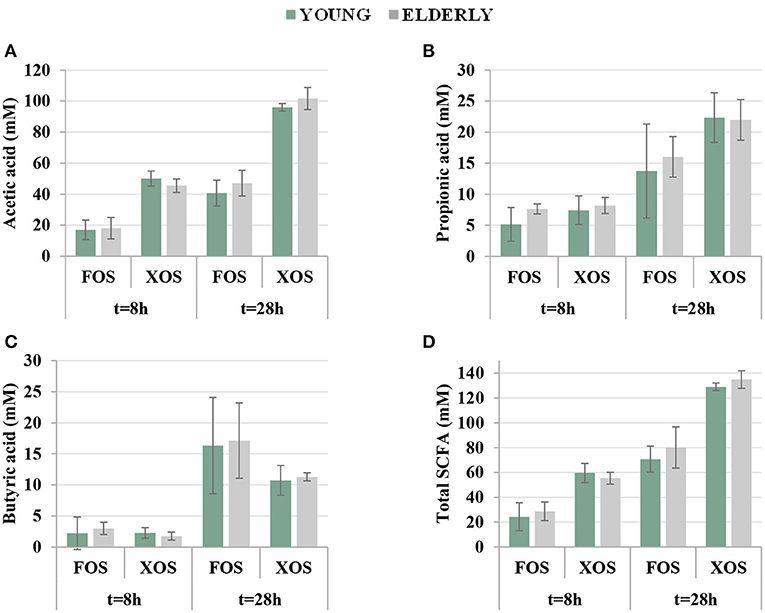
Figure 5. Concentrations (expressed as mM) of major organic acids: (A) acetic acid, (B) propionic acid, (C) butyric acid, and (D) total short-chain fatty acids (SCFA) measured in fermentation vessels at different fermentation times (t = 8 h and t = 28 h) using either fructooligosaccharides (FOS) or xylooligosaccharides (XOS) as major carbon source for intestinal bacteria. Within each group, SD among different donors (n = 3) is represented by error bars.
Acetic Acid
Acetate is the metabolite generated by several members of the gut microbiota, including bifidobacteria, Bacteroides, clostridia, or eubacteria, among others, and can exert a variety of physiological effects including the anti-inflammatory activity or the inhibition of pathogens throughout a pH decrease. In addition, it can serve as co-substrate for butyrate production (Rivière et al., 2016). In this work, acetic acid was the main organic acid generated by fermentation of both XOS and FOS (see Figure 5A). According to the statistical analysis, the acetic acid concentrations at 28 h were significantly higher when XOS were used as substrate in comparison with FOS (98.8 ± 5.9 mM vs. 43.9 ± 8.6 mM, respectively).
These results further support the findings of previous works dealing with XOS prebiotic potential, which reported a strong acetate production using XOS and also AXOS manufactured from diverse biomass sources as substrates in in vitro experiments using fecal inocula from both young (Gullón et al., 2010, 2014; Dávila et al., 2019; Álvarez et al., 2020) and elderly donors (Gómez et al., 2015; Míguez et al., 2018). Furthermore, the results observed here support the higher acetogenic effect of XOS previously observed (Gómez et al., 2015; Dávila et al., 2019; La Rosa et al., 2019; Álvarez et al., 2020), but it could be also related to the high acetylation degree of XOS employed in this work (see section Xylooligosaccharides Production and Characterization).
Propionic Acid
Figure 5B shows the time dependence of propionic acid production during XOS and FOS fermentation, respectively, for both age-groups. At t = 28 h, significantly higher concentrations were found in experiments with XOS in comparison with FOS (22.2 ± 3.5 mM vs. 14.9 ± 5.7 mM, respectively). As can be seen, propionic acid was the second main organic acid generated along with the fermentation of both substrates, but mainly when XOS were used as a carbohydrate source. This is in agreement with the results obtained in previous works using XOS (Ho et al., 2018; Dávila et al.,2019) and AXOS (Gómez et al., 2015). Conversely, other authors found lower propionate than butyrate concentrations when using the aforementioned oligosaccharides as substrates (Gullón et al., 2010, 2014; Míguez et al., 2018; Álvarez et al., 2020). These differences in the organic acid profiles (and in the effect on microbiota) could be related to the source and chemical structure of oligosaccharides and to the enormous complexity and inter-individual variability existent among the human gut microbiota, which might promote different fermentation profiles and which constitute, in fact, one of the major challenges for the establishment of microbial modulation strategies (Moles and Otaegui, 2020).
Butyric Acid
As it was mentioned above, butyrate is mainly produced by bacteria belonging to Clostridium clusters XIVa and IV. As can be seen in Figure 5C, butyrate was mainly produced during the period 8–28 h in which the concentration was increased almost six times. Statistical analyses revealed that the butyrate production was affected by the substrate, reaching significantly higher concentrations in FOS experiments, which is in agreement with the previous works using XOS manufactured from E. globulus and from oil palm empty fruit bunch fiber by the hydrothermal treatments (Ho et al., 2018; Míguez et al., 2018). In this case, an average concentration of 16.7 ± 6.6 and 11.0 ± 1.7 mM was achieved at t = 28 h with FOS and XOS, respectively.
Taking these results into account, it can be concluded that XOS and FOS lead to different total SCFA concentrations (calculated as the sum of acetate, propionate, and butyrate, see Figure 4D) and product profiles. Up to 132.0 ± 6.0 mM was obtained as average concentration by using XOS as carbon source, whereas 75.5 ± 14.1 mM was achieved with FOS. The SCFA concentrations obtained after 28 h of fermentation were very similar to those obtained by Dávila et al. (2019) (131.4 mM) after 24 h of XOS (obtained from vine shoots) in vitro fermentation and higher, although comparable, to those achieved by Álvarez et al. (2020) after 30 h using two different XOS fractions obtained from barley straw (92.5–114.4 mM). However, the product profiles defined here as acetate:propionate:butyrate molar ratios were 9:2:1 for XOS and 2.6:0.9:1 for FOS. Therefore, in comparison with FOS, XOS obtained from E. nitens resulted in higher amounts of total SCFA and mixtures enriched in acetate and propionate.
Other Organic Acids
Formic acid can be generated by bifidobacteria and butyrate-producing bacteria not only from pentoses or hexoses but also from lactate by butyrate-producers. A continuous production of formic acid was observed along the time for all donors, age-groups, and substrates. Significant differences were observed in the formic acid concentration between XOS and FOS. At 28 h, up to 25.9 ± 11.1 mM was achieved in media containing FOS, whereas just 15.2 ± 9.0 mM was produced by the XOS fermentation.
Other acids, such as lactic, succinic, isobutyric, valeric, and isovaleric acids, were simultaneously produced during the fermentation assays, and significant differences between substrates were found in the case of lactic acid where FOS led to a concentration of 6.8 ± 10.3 mM at 28 h, whereas a negligible production was observed with XOS (<0.15 mM). This is in agreement with previous studies where higher amounts of lactic acid were produced with FOS in comparison with AXOS (Gómez et al., 2015) or other substrates, such as mixtures of pectic oligosaccharides from sugar beet pulp (Gómez et al., 2016). Lower concentrations of succinic acid (range 0–9 mM) were also found for XOS and FOS at 28 h, and negligible amounts (<1 mM) of isobutyric, valeric, and isovaleric acids were achieved at 28 h in this work.
Taking together, these results highlight interesting differences between FOS and XOS at 28 h, not only in the total organic acid (TOA) concentration but also in the SCFA/TOA molar ratio. The FOS fermentation resulted in an average TOA concentration of 110.3 ± 24.4 mM, whereas XOS led to a value of 150.7 ± 10.8 mM being the SCFA/TOA molar ratio = 0.70 ± 0.11 for FOS and 0.88 ± 0.06 for XOS, indicating that XOS resulted in higher organic acid production with a profile more enriched in SCFA.
Conclusions
Mixtures of substituted XOS were obtained by hydrothermal processing of E. nitens wood and refined following a two-step sequence of membrane filtration and ion exchange. This purification strategy allowed to reduce the non-volatile impurities content from 16.70 to 7.56%, leading to a final product containing up to 84% of substituted XOS.
The in vitro fermentation assays (using inocula fecal samples from young and elderly donors) showed that refined XOS led to, at least, similar microbiota composition and functional profile in comparison with FOS.
On the one hand, both substrates resulted (in general) in similar effects in most of the bacterial groups considered, XOS led to higher increases in total bacteria and lower increases in Clostridium clusters I and II.
On the other hand, both substrates resulted in different metabolite productions and profiles. Higher amounts of TOA and total SCFA were achieved at 28 h, using XOS as carbon source in comparison with FOS. Moreover, SCFA mixtures enriched in acetate and propionate were achieved when XOS were used as substrate whereas FOS resulted in a slightly higher concentration of butyrate.
Therefore, refined XOS obtained from E. nitens wood showed, under the in vitro conditions tested in this study, the similar prebiotic potential to FOS, both in terms of microbiota composition and also in metabolite production. Even though these effects should be further tested on in vitro dynamic experiments or/and in vivo, the results obtained in this work support the prebiotic potential of these substituted XOS, which can be manufactured from woody biomass throughout a biorefinery scheme using green technologies.
Data Availability Statement
The raw data supporting the conclusions of this article will be made available by the authors, without undue reservation.
Author Contributions
JA: experimental design, coordination, and supervision of data analyses. Experimental work: (1) PG and CV: XOS manufacture and purification. (2) PG, CV, and BM: oligosaccharide chemical and structural characterization. (3) PG and BM: fecal samples collection and inocula preparation. (4) PG and BM: in vitro fermentation assays. (5) BM and CV: analyses of fermentation products. (6) BM, MM-C, and FP-C: microbial populations FISH–FCM analyses. (7) TC-Y: statistical analyses. All authors contributed to the literature review, data processing, results interpretation, figures/tables design and creation, contributed to the intellectual content, reviewed the manuscript, and approved the final version for publication.
Funding
This work was made possible by the financial support from the Spanish Ministry of Economy and Competitiveness and the FEDER program of the European Union (research project Modified aqueous media for wood biorefineries, reference CTQ2017-82962-R), the European Regional Development Fund (ERDF), and the Xunta de Galicia (Centro Singular de Investigación de Galicia, CINBIO, accreditation 2016–2019).
Conflict of Interest
The authors declare that the research was conducted in the absence of any commercial or financial relationships that could be construed as a potential conflict of interest.
Acknowledgments
The authors are very grateful to Dr. Alberto Álvarez Barrientos and Dra. Mercedes Peleteiro Olmedo for their support and assistance in the application of the FISH/FCM technique. The authors also express their gratitude to the volunteers who have participated in this study. Finally, BM would like to express her gratitude to the Xunta de Galicia for her predoctoral grant.
Supplementary Material
The Supplementary Material for this article can be found online at: https://www.frontiersin.org/articles/10.3389/fceng.2021.670440/full#supplementary-material
Abbreviations
AOS, arabinooligosaccharides; AXOS, arabinoxylooligosaccharides; BM, basal microbiota; BSG, brewer's spent grain; DP, degree of polymerization; DV, diafiltration volumes (1 DV = 1 ml of added water and permeated per mL of feed stream); EFSA, European Food Safety Authority; FDA, Food and Drug Administration; FISH–FCM, fluorescent in situ hybridization coupled with flow cytometry; FOS, fructooligosaccharides; GlcOS, glucooligosaccharides; GRAS, Generally Recognized as Safe; LRR, liquid-to-resin mass ratio; LSR, liquid-to-solid ratio; NVC, non-volatile compound; ONVC, other non-volatile compound; PBS, phosphate-buffered saline; REML, restricted maximum likelihood; SCFA, short-chain fatty acid; TOA, total organic acid; VCR, volume-to-concentrate ratio; XOS, xylooligosaccharides; YNB, yeast nitrogen base; FTIR, Fourier transform infrared; EDTA, ethylenediaminetetraacetic acid; HPLC, high-performance liquid chromatography; MALDI–TOF MS, matrix-assisted laser desorption ionization/time of flight mass spectrometry; SDS, sodium dodecyl sulfate; UPLC–DAD–ESI MS, ultraperformance liquid chromatography–diode array detector–electrospray ionization mass spectrometry.
References
Albenberg, L. G., and Wu, G. D. (2014). Diet and the intestinal microbiome: associations, functions, and implications for health and disease. Gastroenterology 146, 1564–1572. doi: 10.1053/j.gastro.2014.01.058
Álvarez, C., González, A., Alonso, J. L., Sáez, F., Negro, M. J., and Gullón, B. (2020). Xylooligosaccharides from steam-exploded barley straw: structural features and assessment of bifidogenic properties. Food Bioprod. Process. 124, 131–142. doi: 10.1016/j.fbp.2020.08.014
Amorim, C., Silvério, S. C., Prather, K. L. J., and Rodrigues, L. R. (2019). From lignocellulosic residues to market: production and commercial potential of xylooligosaccharides. Biotechnol. Adv. 37:107397. doi: 10.1016/j.biotechadv.2019.05.003
Arrigucci, R., Bushkin, Y., Radford, F., Lakehal, K., Vir, P., Pine, R., et al. (2017). FISH-Flow, a protocol for the concurrent detection of mRNA and protein in single cells using fluorescence in situ hybridization and flow cytometry. Nat. Protoc. 12, 1245–1260. doi: 10.1038/nprot.2017.039
Basson, A., Trotter, A., Rodriguez-Palacios, A., and Cominelli, F. (2016). Mucosal interactions between genetics, diet, and microbiome in inflammatory bowel disease. Front. Immunol. 7:290. doi: 10.3389/fimmu.2016.00290
Biagi, E., Nylund, L., Candela, M., Ostan, R., Bucci, L., Pini, E., et al. (2010). Through ageing, and beyond: gut microbiota and inflammatory status in seniors and centenarians. PLoS ONE 5:e10667. doi: 10.1371/annotation/df45912f-d15c-44ab-8312-e7ec0607604d
Bischoff, S. C. (2016). Microbiota and aging. Curr. Opin. Clin. Nutr. Metab. Care 19, 26–30. doi: 10.1097/MCO.0000000000000242
Buford, T. W. (2017). (Dis)Trust your gut: the gut microbiome in age-related inflammation, health, and disease. Microbiome 5:80. doi: 10.1186/s40168-017-0296-0
Buranov, A. U., and Mazza, G. (2010). Extraction and characterization of hemicelluloses from flax shives by different methods. Carbohydr. Polym. 79, 17–25. doi: 10.1016/j.carbpol.2009.06.014
Castillo Martinez, F. A., Balciunas, E. M., Converti, A., Cotter, P. D., and de Souza Oliveira, R. P. (2013). Bacteriocin production by Bifidobacterium spp. A review. Biotechnol. Adv. 31, 482–488. doi: 10.1016/j.biotechadv.2013.01.010
Cebreiros, F., Risso, F., Cagno, M., Cabrera, M. N., Rochón, E., Jauregui, G., et al. (2020). Enhanced production of butanol and xylosaccharides from Eucalyptus grandis wood using steam explosion in a semi-continuous pre-pilot reactor. Fuel 290:119818. doi: 10.1016/j.fuel.2020.119818
Dávila, I., Gullón, B., Alonso, J. L., Labidi, J., and Gullón, P. (2019). Vine shoots as new source for the manufacture of prebiotic oligosaccharides. Carbohydr. Polym. 207, 34–43. doi: 10.1016/j.carbpol.2018.11.065
Fraher, M. H., O'Toole, P. W., and Quigley, E. M. M. (2012). Techniques used to characterize the gut microbiota: a guide for the clinician. Nat. Rev. Gastroenterol. Hepatol. 9, 312–322. doi: 10.1038/nrgastro.2012.44
Franceschi, C., Garagnani, P., Parini, P., Giuliani, C., and Santoro, A. (2018). Inflammaging: a new immune–metabolic viewpoint for age-related diseases. Nat. Rev. Endocrinol. 14, 576–590. doi: 10.1038/s41574-018-0059-4
Franks, A. H., Harmsen, H. J. M., Gerwin, C. R., Jansen, G. J., Schut, F., and Gjalt, W. (1998). Variations of bacterial populations in human feces measured by fluorescent in situ hybridization with group-specific 16S rRNA-targeted oligonucleotide probes. Appl. Environ. Microbiol. 64, 3336–3345. doi: 10.1128/AEM.64.9.3336-3345.1998
Gibson, G. R., Hutkins, R., Sanders, M. E., Prescott, S. L., Reimer, R. A., Salminen, S. J., et al. (2017). Expert consensus document: the International Scientific Association for Probiotics and Prebiotics (ISAPP) consensus statement on the definition and scope of prebiotics. Nat. Rev. Gastroenterol. Hepatol. 14, 491–502. doi: 10.1038/nrgastro.2017.75
Gibson, G. R., Probert, H. M., Loo, J., Van Rastall, R. A., and Roberfroid, M. B. (2004). Dietary modulation of the human colonic microbiota: updating the concept of prebiotics. Nutr. Res. Rev. 17, 259–275. doi: 10.1079/NRR200479
Gómez, B., Gullón, B., Remoroza, C., Schols, H. A., Parajó, J. C., and Alonso, J. L. (2014). Purification, characterization, and prebiotic properties of pectic oligosaccharides from orange peel wastes. J. Agric. Food Chem. 62, 9769–9782. doi: 10.1021/jf503475b
Gómez, B., Gullón, B., Yáñez, R., Schols, H., and Alonso, J. L. (2016). Prebiotic potential of pectins and pectic oligosaccharides derived from lemon peel wastes and sugar beet pulp: a comparative evaluation. J. Funct. Foods 20, 108–121. doi: 10.1016/j.jff.2015.10.029
Gómez, B., Míguez, B., Veiga, A., Parajó, J. C., and Alonso, J. L. (2015). Production, purification, and in vitro evaluation of the prebiotic potential of arabinoxylooligosaccharides from brewer's spent grain. J. Agric. Food Chem. 63, 8429–8438. doi: 10.1021/acs.jafc.5b03132
Grimaldi, R., Cela, D., Swann, J. R., Vulevic, J., Gibson, G. R., Tzortzis, G., et al. (2017). In vitro fermentation of B-GOS: impact on faecal bacterial populations and metabolic activity in autistic and non-autistic children. FEMS Microbiol. Ecol. 93, 1–10. doi: 10.1093/femsec/fiw233
Gullón, B., Gullón, P., Sanz, Y., Alonso, J. L., and Parajó, J. C. (2011). Prebiotic potential of a refined product containing pectic oligosaccharides. LWT - Food Sci. Technol. 44, 1687–1696. doi: 10.1016/j.lwt.2011.03.006
Gullón, B., Gullón, P., Tavaria, F., Pintado, M., Gomes, A. M., Alonso, J. L., et al. (2014). Structural features and assessment of prebiotic activity of refined arabinoxylooligosaccharides from wheat bran. J. Funct. Foods 6, 438–449. doi: 10.1016/j.jff.2013.11.010
Gullón, P., González-Muñoz, M. J., and Parajó, J. C. (2011b). Manufacture and prebiotic potential of oligosaccharides derived from industrial solid wastes. Bioresour. Technol. 102, 6112–6119. doi: 10.1016/j.biortech.2011.02.059
Gullón, P., González-Muñoz, M. J., van Gool, M. P., Schols, H. A., Hirsch, J., Ebringerová, A., et al. (2010). Production, refining, structural characterization and fermentability of rice husk xylooligosaccharides. J. Agric. Food Chem. 58, 3632–3641. doi: 10.1021/jf904508g
Gullón, P., González-Muñoz, M. J., Van Gool, M. P., Schols, H. A., Hirsch, J., Ebringerová, A., et al. (2011a). Structural features and properties of soluble products derived from Eucalyptus globulus hemicelluloses. Food Chem. 127, 1798–1807. doi: 10.1016/j.foodchem.2011.02.066
Ho, A. L., Kosik, O., Lovegrove, A., Charalampopoulos, D., and Rastall, R. A. (2018). In vitro fermentability of xylo-oligosaccharide and xylo-polysaccharide fractions with different molecular weights by human faecal bacteria. Carbohydr. Polym. 179, 50–58. doi: 10.1016/j.carbpol.2017.08.077
Hold, G. L., Schwiertz, A., Aminov, R. I., Blaut, M., and Flint, H. J. (2003). Oligonucleotide probes that detect quantitatively significant groups of butyrate-producing bacteria in human feces. Appl. Environ. Microbiol. 69, 4320–4324. doi: 10.1128/AEM.69.7.4320-4324.2003
Hong, C., Corbett, D., Venditti, R., Jameel, H., and Park, S. (2019). Xylooligosaccharides as prebiotics from biomass autohydrolyzate. LWT - Food Sci. Technol. 111, 703–710. doi: 10.1016/j.lwt.2019.05.098
Karlsson, E. N., Schmitz, E., Linares-Pastén, J. A., and Adlercreutz, P. (2018). Endo-xylanases as tools for production of substituted xylooligosaccharides with prebiotic properties. Appl. Microbiol. Biotechnol. 102, 9081–9088. doi: 10.1007/s00253-018-9343-4
Kong, F., Hua, Y., Zeng, B., Ning, R., Li, Y., and Zhao, J. (2016). Gut microbiota signatures of longevity. Curr. Biol. 26, R832–R833. doi: 10.1016/j.cub.2016.08.015
La Rosa, S. L., Kachrimanidou, V., Buffetto, F., Pope, P. B., Pudlo, N. A., Martens, E. C., et al. (2019). Wood-derived dietary fibers promote beneficial human gut microbiota. mSphere 4, e00554–e00518. doi: 10.1128/mSphere.00554-18
Lu, M., and Wang, Z. (2018). Microbiota and aging. Adv. Exp. Med. Biol. 1086, 141–156. doi: 10.1007/978-981-13-1117-8_9
Malinen, E., Mättö, J., Salmitie, M., Alander, M., Saarela, M., and Palva, A. (2002). PCR-ELISA: II: Analysis of Bifidobacterium populations in human faecal samples from a consumption trial with Bifidobacterium lactis Bb-12 and a galacto-oligosaccharide preparation. Syst. Appl. Microbiol. 25, 249–258. doi: 10.1016/S0723-2020(04)70109-5
Merchant, H. A., Liu, F., Orlu Gul, M., and Basit, A. W. (2016). Age-mediated changes in the gastrointestinal tract. Int. J. Pharm. 512, 382–395. doi: 10.1016/j.ijpharm.2016.04.024
Míguez, B., Gómez, B., Parajó, J. C., and Alonso, J. L. (2018). Potential of fructooligosaccharides and xylooligosaccharides as substrates to counteract the undesirable effects of several antibiotics on elder fecal microbiota: a first in vitro approach. J. Agric. Food Chem. 66, 9426–9437. doi: 10.1021/acs.jafc.8b02940
Miquel, S., Martin, R., Bridonneau, C., Robert, V., Sokol, H., Bermúdez-Humarán, L. G., et al. (2014). Ecology and metabolism of the beneficial intestinal commensal bacterium Faecalibacterium prausnitzii. Gut Microbes 5, 146–151. doi: 10.4161/gmic.27651
Moles, L., and Otaegui, D. (2020). The impact of diet on microbiota evolution and human health. Is diet an adequate tool for microbiota modulation? Nutrients 12, 1–19. doi: 10.3390/nu12061654
Myhrstad, M. C. W., Tunsjø, H., Charnock, C., and Telle-Hansen, V. H. (2020). Dietary fiber, gut microbiota, and metabolic regulation—current status in human randomized trials. Nutrients 12, 1–18. doi: 10.3390/nu12030859
Neiva, D. M., Costa, R. A., Gominho, J., Ferreira-Dias, S., and Pereira, H. (2020). Fractionation and valorization of industrial bark residues by autohydrolysis and enzymatic saccharification. Bioresour. Technol. Reports 11:100441. doi: 10.1016/j.biteb.2020.100441
Neto, F. S. P. P., Roldán, I. U. M., Galán, J. P. M., Monti, R., de Oliveira, S. C., and Masarin, F. (2020). Model-based optimization of xylooligosaccharides production by hydrothermal pretreatment of Eucalyptus by-product. Ind. Crops Prod. 154:112707. doi: 10.1016/j.indcrop.2020.112707
Nyangale, E. P., Farmer, S., Keller, D., Chernoff, D., and Gibson, G. R. (2014). Effect of prebiotics on the fecal microbiota of elderly volunteers after dietary supplementation of Bacillus coagulans GBI-30, 6086. Anaerobe 30, 75–81. doi: 10.1016/j.anaerobe.2014.09.002
O'Connor, S., Chouinard-Castonguay, S., Gagnon, C., and Rudkowska, I. (2017). Prebiotics in the management of components of the metabolic syndrome. Maturitas 104, 11–18. doi: 10.1016/j.maturitas.2017.07.005
Odamaki, T., Kato, K., Sugahara, H., Hashikura, N., Takahashi, S., Xiao, J. Z., et al. (2016). Age-related changes in gut microbiota composition from newborn to centenarian: a cross-sectional study. BMC Microbiol. 16, 1–12. doi: 10.1186/s12866-016-0708-5
Ou, F., McGoverin, C., Swift, S., and Vanholsbeeck, F. (2017). Absolute bacterial cell enumeration using flow cytometry. J. Appl. Microbiol. 123, 464–477. doi: 10.1111/jam.13508
Penín, L., Lange, H., Santos, V., Crestini, C., and Parajó, J. C. (2020). Characterization of Eucalyptus nitens lignins obtained by biorefinery methods based on ionic liquids. Molecules 25:425. doi: 10.3390/molecules25020425
Penín, L., Peleteiro, S., Santos, V., Alonso, J. L., and Parajó, J. C. (2019a). Selective fractionation and enzymatic hydrolysis of Eucalyptus nitens wood. Cellulose 26, 1125–1139. doi: 10.1007/s10570-018-2109-4
Penín, L., Santos, V., del Río, J. C., and Parajó, J. C. (2019b). Assessment on the chemical fractionation of Eucalyptus nitens wood: characterization of the products derived from the structural components. Bioresour. Technol. 281, 269–276. doi: 10.1016/j.biortech.2019.02.098
Pérez, S., Renedo, C. J., Ortiz, A., Mañana, M., and Silió, D. (2006). Energy evaluation of the Eucalyptus globulus and the Eucalyptus nitens in the north of Spain (Cantabria). Thermochim. Acta 451, 57–64. doi: 10.1016/j.tca.2006.08.009
Pinelo, M., Jonsson, G., and Meyer, A. S. (2009). Membrane technology for purification of enzymatically produced oligosaccharides: molecular and operational features affecting performance. Sep. Purif. Technol. 70, 1–11. doi: 10.1016/j.seppur.2009.08.010
Pinheiro, J., Bates, D., DebRoy, S., Sarkar, D. R., and Core Team (2020). _nlme: Linear and Nonlinear Mixed Effects Models_. R; package version 3.1-149. Available online at: https://cran.r-project.org/package=nlme
Poletto, P., Pereira, G. N., Monteiro, C. R. M., Pereira, M. A. F., Bordignon, S. E., and de Oliveira, D. (2020). Xylooligosaccharides: transforming the lignocellulosic biomasses into valuable 5-carbon sugar prebiotics. Process Biochem. 91, 352–363. doi: 10.1016/j.procbio,.2020.01.005
R Core Team (2020). R: A Language and environment for statistical computing. Vienna: R Foundation for Statistical Computing. Available online at: https://www.r-project.org
Rivière, A., Selak, M., Lantin, D., Leroy, F., and De Vuyst, L. (2016). Bifidobacteria and butyrate-producing colon bacteria: importance and strategies for their stimulation in the human gut. Front. Microbiol. 7:979. doi: 10.3389/fmicb.2016.00979
Rondanelli, M., Giacosa, A., Faliva, M. A., Perna, S., Allieri, F., and Castellazzi, A. M. (2015). Review on microbiota and effectiveness of probiotics use in older. World J. Clin. Cases 3, 156–162. doi: 10.12998/wjcc.v3.i2.156
Rossi, O., Van Berkel, L. A., Chain, F., Tanweer Khan, M., Taverne, N., Sokol, H., et al. (2016). Faecalibacterium prausnitzii A2-165 has a high capacity to induce IL-10 in human and murine dendritic cells and modulates T cell responses. Sci. Rep. 6:18507. doi: 10.1038/srep18507
Salazar, N., Arboleya, S., Fernández-Navarro, T., de los Reyes-Gavilán, C. G., Gonzalez, S., and Gueimonde, M. (2019). Age-associated changes in gut microbiota and dietary components related with the immune system in adulthood and old age: a cross-sectional study. Nutrients 11, 1–11. doi: 10.3390/nu11081765
Salazar, N., Arboleya, S., Valdés, L., Stanton, C., Ross, P., Ruiz, L., et al. (2014). The human intestinal microbiome at extreme ages of life. Dietary intervention as a way to counteract alterations. Front. Genet. 5:406. doi: 10.3389/fgene.2014.00406
Salazar, N., López, P., Valdés, L., Margolles, A., Suárez, A., Patterson, Á. M., et al. (2013). Microbial targets for the development of functional foods accordingly with nutritional and immune parameters altered in the elderly. J. Am. Coll. Nutr. 32, 399–406. doi: 10.1080/07315724.2013.827047
Salazar, N., Valdés-Varela, L., González, S., Gueimonde, M., and de los Reyes-Gavilán, C. G. (2017). Nutrition and the gut microbiome in the elderly. Gut Microbes 8, 82–97. doi: 10.1080/19490976.2016.1256525
Samanta, A. K., Jayapal, N., Jayaram, C., Roy, S., Kolte, A. P., Senani, S., et al. (2015). Xylooligosaccharides as prebiotics from agricultural by-products: production and applications. Bioact. Carbohydrates Diet. Fibre 5, 62–71. doi: 10.1016/j.bcdf.2014.12.003
Santibáñez, L., Henríquez, C., Corro-Tejeda, R., Bernal, S., Armijo, B., and Salazar, O. (2021). Xylooligosaccharides from lignocellulosic biomass: a comprehensive review. Carbohydr. Polym. 251, 1–15. doi: 10.1016/j.carbpol.2020.117118
Sebastián, J. J., and Sánchez, C. (2018). De la flora intestinal al microbioma. Rev. Española Enfermedades Dig. 110, 51–56. doi: 10.17235/reed.2017.4947/2017
Singh, R. D., Banerjee, J., and Arora, A. (2015). Prebiotic potential of oligosaccharides: a focus on xylan derived oligosaccharides. Bioact. Carbohydrates Diet. Fibre 5, 19–30. doi: 10.1016/j.bcdf.2014.11.003
Sonnenburg, J. L., and Bäckhed, F. (2016). Diet-microbiota interactions as moderators of human metabolism. Nature 535, 56–64. doi: 10.1038/nature18846
Sun, J. X., Sun, X. F., Sun, R. C., and Su, Y. Q. (2004). Fractional extraction and structural characterization of sugarcane bagasse hemicelluloses. Carbohydr. Polym. 56, 195–204. doi: 10.1016/j.carbpol.2004.02.002
Vandeputte, D., Kathagen, G., D'Hoe, K., Vieira-Silva, S., Valles-Colomer, M., Sabino, J., et al. (2017). Quantitative microbiome profiling links gut community variation to microbial load. Nature 551, 507–511. doi: 10.1038/nature24460
Vázquez, M. J., Alonso, J. L., Domínguez, H., and Parajó, J. C. (2000). Xylooligo- saccharides : manufacture and applications. Trends Food Sci. Technol. 11, 387–393. doi: 10.1016/S0924-2244(01)00031-0
Vázquez, M. J., Garrote, G., Alonso, J. L., Domínguez, H., and Parajó, J. C. (2005). Refining of autohydrolysis liquors for manufacturing xylooligosaccharides: evaluation of operational strategies. Bioresour. Technol. 96, 889–896. doi: 10.1016/j.biortech.2004.08.013
Voreades, N., Kozil, A., and Weir, T. L. (2014). Diet and the development of the human intestinal microbiome. Front. Microbiol. 5:494. doi: 10.3389/fmicb.2014.00494
Walker, A. W., Duncan, S. H., Louis, P., and Flint, H. J. (2014). Phylogeny, culturing, and metagenomics of the human gut microbiota. Trends Microbiol. 22, 267–274. doi: 10.1016/j.tim.2014.03.001
Walker, A. W., Duncan, S. H., Mcwilliam leitch, E. C., Child, M. W., and Flint, H. J. (2005). ph and peptide supply can radically alter bacterial populations and short-chain fatty acid ratios within microbial communities from the human colon. Appl. Environ. Microbiol. 71, 3692–3700. doi: 10.1128/AEM.71.7.3692-3700.2005
Woodmansey, E. J., McMurdo, M. E. T., Macfarlane, G. T., and Macfarlane, S. (2004). Comparison of compositions and metabolic activities of fecal microbiotas in young adults and in antibiotic-treated and non-antibiotic-treated elderly subjects. Appl. Environ. Microbiol. 70, 6113–6122. doi: 10.1128/AEM.70.10.6113-6122.2004
Keywords: xylooligosaccharides, XOS, Eucalyptus nitens, prebiotic, in vitro, biomass, elderly, gut microbiota
Citation: Míguez B, Gullón P, Cotos-Yáñez T, Massot-Cladera M, Pérez-Cano FJ, Vila C and Alonso JL (2021) Manufacture and Prebiotic Potential of Xylooligosaccharides Derived From Eucalyptus nitens Wood. Front. Chem. Eng. 3:670440. doi: 10.3389/fceng.2021.670440
Received: 21 February 2021; Accepted: 30 March 2021;
Published: 05 May 2021.
Edited by:
Héctor A. Ruiz, Universidad Autónoma de Coahuila, MexicoReviewed by:
Javier Linares-Pastén, Lund University, SwedenParameth Thiennimitr, Chiang Mai University, Thailand
Rosana Goldbeck, State University of Campinas, Brazil
Copyright © 2021 Míguez, Gullón, Cotos-Yáñez, Massot-Cladera, Pérez-Cano, Vila and Alonso. This is an open-access article distributed under the terms of the Creative Commons Attribution License (CC BY). The use, distribution or reproduction in other forums is permitted, provided the original author(s) and the copyright owner(s) are credited and that the original publication in this journal is cited, in accordance with accepted academic practice. No use, distribution or reproduction is permitted which does not comply with these terms.
*Correspondence: José Luis Alonso, eGx1aXNAdXZpZ28uZXM=