- 1Division of Materials Science, Department of Engineering Sciences and Mathematics, Luleå University of Technology, Luleå, Sweden
- 2Department of Molecular Sciences and Nanosystems, Ca’ Foscari University of Venice, Venezia, Italy
- 3CNR-IMM Bologna Section, Bologna, Italy
The design of oxygen evolution reaction (OER) electrocatalysts based on Earth-abundant materials holds great promise for realizing practically viable water-splitting systems. In this regard, two-dimensional (2D) nonlayered materials have received considerable attention in recent years owing to their intrinsic dangling bonds which give rise to the exposure of unsaturated active sites. In this work, we solved the synthesis challenge in the development of a 2D nonlayered Cr2S3 catalyst for OER application via introducing a controllable chemical vapor deposition scheme. The as-obtained catalyst exhibits a very good OER activity requiring overpotentials of only 230 mV and 300 mV to deliver current densities of 10 mA cm−2 and 30 mA cm−2, respectively, with robust stability. This study provides a general approach to optimize the controllable growth of 2D nonlayered material and opens up a fertile ground for studying the various strategies to enhance the water splitting reactions.
Introduction
Designing efficient water splitting catalysts is central to substantial progress for the sustainable development of modern society (Gray, 2009; Cook et al., 2010). In this respect, the production of hydrogen through the electrolysis of water at the cathode highly depends on efficient and stable oxygen evolution reaction (OER) at the anode. However, the OER process involves proton-coupled four-electron-transfer reactions exhibiting sluggish kinetics and higher energy demands compared to the reaction at the cathode counterpart (Gao et al., 2017; Tahir et al., 2017; Yao et al., 2018; Zhang et al., 2020; Arif et al., 2021). The past few decades have witnessed rapid advances in two-dimensional (2D) materials and other materials to improve their catalytic efficiency with manifold strategies (Geim and Grigorieva, 2013; Bhimanapati et al., 2015; Shifa and Vomiero, 2019; Arif et al., 2020; Gao and Yan, 2020; Wan et al., 2020; Mushtaq et al., 2021). For instance, electronic structure engineering via incorporating dopants (Min Tan and Pumera, 2019; Dou et al., 2020), vacancies (Yang et al., 2019), strains (Oakes et al., 2016), and heterostructures (Deng et al., 2016; Shifa et al., 2018) has been communicated as a promising strategy to modify the binding energies of reaction intermediates. Yet, the nonlayered class of 2D materials has been barely studied in the catalysis of OER. Unlike the layered 2D materials (the in-plane atoms are connected via strong chemical bonding, and the stacking layers are combined via weak van der Waals interaction), nonlayered 2D materials are connected via chemical bonding in the 3D network. This in turn endows the family unsaturated dangling bonds on the surface, thereby exhibiting a high-activity and high-energy surface (Wang et al., 2017). In addition to the dangling bonds, metal sulfides having higher conductivity as compared to oxides endow a conducive environment to accelerate the oxidation of the –OOH intermediate and enhance O2 gas generation (Peng et al., 2018; Joo et al., 2019). Moreover, chromium-based catalysts have recently won the attention of many research groups (Murray et al., 2010; Baek et al., 2012; Xu et al., 2018; Lin et al., 2019; Du et al., 2020; Park et al., 2020) due to the fact that Cr3 cations exhibit a special electronic configuration (t32ge0g), which facilitates electron transfer and electron capture. Despite this appealing feature, the realization of these materials is largely impeded by the fabrication challenges. Owing to the 3D strong bonding, it is difficult to controllably synthesize nonlayered 2D nanosheets through a mere exfoliation approach or conventional chemical vapor transport method. A promising approach has recently been reported on mica substrate using space confined CVD to synthesize Cr2S3 (Zhou et al., 2019; Cui et al., 2020; Xie et al., 2020). Yet, the OER performance of nonlayered metal sulfides remains far from satisfactory. In this work, we first synthesized Cr2O3 on a carbon fiber substrate (CF) and then controllably sulfurized it in a two-zone chemical vapor deposition furnace to get vertically oriented hexagonal Cr2S3 nanosheets. In the main CVD chamber, we employed two small tubes inserted into one another to saturate the vapors and direct their deposition at the desired target. The resulting sulfide could create a kinetically/energetically favorable environment for the adsorption/desorption of oxide moieties, thereby producing oxygen gas. Accordingly, in a typical three-electrode setup, it delivers current densities of 10 mA cm−2 and 30 mA cm−2 at overpotentials of 230 mV and 300 mV, respectively, with excellent stability for over 10 h of duration.
Material and Methods
Synthesis of Cr2O3Precursor: The Cr2O3 precursor was synthesized on the CF substrate via the CVD method. Typically, 0.5 g of Cr2O3 powder was kept in the center of the CVD chamber and the CF substrate was kept ∼7 cm away from the center. The chamber pressure was pumped down to about 0.1 Pa. Ar gas (50 standard cubic centimeters per minute (sccm)) was used to transport chromium oxide vapor species to the substrates. With this Ar-saturated environment, the temperature was raised to ∼700 °C with a heating rate of 30 °C min−1 which lasts for 40 min. Then, the system was allowed to cool naturally, and the product (i.e., Cr2O3/CF) was taken for the next step.
Sulfurization of Cr2O3/CF: Two-zone CVD setup was employed to undergo the sulfurization reaction. In addition to the main CVD tube, we used two small tubes whose ends coincide with the heating centers in each zone to concentrate the vapor. Then, 0.5 g of S powder was kept in the front zone, and the Cr2O3/CF precursor was kept in the back zone. Before the reaction starts, the CVD tube was deaired via repeated (at least thrice) pumping and purging with Ar gas. Subsequently, the front and back zones were simultaneously heated to ∼200°C and ∼600°C within 30 min under Ar gas (50 sccm), respectively, and the reaction was run for 60 min. Finally, the system was cooled down to room temperature and the sample was taken out to be used as an electrocatalyst without any further treatment.
Characterization
The SEM was collected via field emission scanning electron microscopy (FESEM), Magellan XHR 400 L, with 5 kV electron beam. The crystal structure was investigated through FEI Tecnai F20 high-resolution transmission electron microscope (HR-TEM), equipped with a Schottky emitter operating at 200 kV. Elemental analysis was performed by energy dispersive X-ray spectroscopy (EDS), equipped with scanning transmission electron microscopy (STEM-HAADF) to map elemental distribution. More crystallographic data were obtained from PanAlytical Empyrean XRD to get the X-ray diffractogram using Cu kα radiation.
Electrochemical Measurements
ModuLab XM ECS potentiostat (Solartron Instrument) was used to measure the electrochemical properties of the as-synthesized and characterized sample. The oxygen evolution reaction catalytic performance was studied in an alkaline medium (1 M KOH) using a three-electrode configuration with an H-type electrochemical cell. Saturated calomel electrode (SCE), Pt wire, and the synthesized samples were used as the reference, counter, and working electrodes, respectively. Linear sweep voltammetry was run with a sweep rate of 5 mV/s with continuous purging of Ar gas to remove dissolved oxygen that may limit the mass transfer. All the potentials are iR corrected and presented with respect to a reversible hydrogen electrode (RHE) which was obtained from the Nernst equation: ERHE = ESCE + 0.059 pH + EoSCE. Chronopotentiometry was run at a current density of 100 mAcm−2 to evaluate the stability test. Electrochemical impedance spectra (EIS) measurements were carried out from 0.1 to 100,000 Hz with an amplitude of 5 mV and overpotential of 300 mV.
Results and Discussions
The synthesis of Cr2S3 followed a two-step route. The Cr2O3 oxide precursor was first deposited on carbon fiber (CF) substrate via the chemical vapor deposition method using Cr2O3 powder as source. The produced material exhibits rough, platelet-like structures formed by sintered nanocrystals (Supplementary Figure S1A). The HRTEM micrographs and selected area electron diffraction (SAED) confirm the growth of hexagonal Cr2O3 on the CF substrate, as reported in Supplementary Figures S1B–D. The Cr2O3 obtained in the first step was used as a precursor for the second step which is a sulfurization process. Figure 1 shows the employed CVD setup meant for sulfurization, wherein S powder is kept in the front zone and the Cr2O3/CF was kept in the back zone. The growth of Cr2S3 is rather challenging as it requires a confined space to control the kinetics and/or the thermodynamics of the vapor transport and thereby the deposition process (Zhou et al., 2019). To this end, we employed two small tubes (socketed into one another as shown in Figure 1) to concentrate the sulfur vapor and direct to the back zone where Cr2S3 is formed. The temperatures in both zones are controllable and the optimized temperature profile is depicted in the left side (Figure 1). The morphologies of the as-obtained products were examined through field emission scanning electron microscope (FESEM) and transmission electron microscopy (TEM). As can be seen from Figure 2A, the marked region which is enlarged in Figure 2B, hexagonal-shaped nanosheets with thickness ∼52 nm are grown entirely on the surface of the CF substrate. The TEM image also supports this observation (Figure 2C). The elemental composition analysis, obtained from energy dispersive spectroscopy (EDS), illustrates the presence of Cr and S with uniform distribution throughout the entire sheet and with atomic ratio affirming the desired composition (2:3). The crystal structure was analyzed via X-ray diffraction and high-resolution TEM (HRTEM). The perspective and top views of structural modeling are illustrated in Figure 3A. Typically, Cr2S3 exhibits a periodic stacking of CrS6 wherein the Cr3+ bonded to six S2– atoms forming a mixture of edge sharing (with fully occupied CrS layer) and corner/face sharing (1/3 occupied Cr1/3S layer), thereby forming a stable structure (Maignan et al., 2012). A compelling difference between nonlayered 2D Cr2S3 and layered 2D materials (MoS2 and WS2) is that the adjacent [Cr1/3S] and [CrS] layers are connected via strong covalent bonds rather than the weak van der Waals forces (Chhowalla et al., 2013). The XRD pattern in Figure 3B shows that the peaks meant for the as-synthesized Cr2S3 matches the standard rhombohedral Cr2S3 pattern (PDF #10–0,340) with distinct peaks at 2θ values of 15.7o, 17.9o, 29.9o, 34.15o, 44.59o, 53.36o,58.7o, 64.9o, and 72.08o meant for (0,0,3), (1,0,1), (1,1,0), (1,1,3),(1,1,6),(3,0,0), (1,1,9), (2,2,3), and (2,2,6) crystal planes. The broad peak at around 2θ = 25° originated from the CF substrate, and there are no any other peaks associated with the oxide phase substantiating the fact that the synthesized product is indeed pure phase Cr2S3 (the XRD pattern of the Cr2O3/CF precursor is provided in Supplementary Figure S2). To further solidify this observation, the crystal phase was analyzed via HRTEM. Selected area electron diffraction (SAED) of a single platelet (Figure 3C) displays a sharp single crystal hexagonal pattern, indexed as rhombohedral Cr2S3 phase on [0,0,1] zone axis. The HRTEM displays the formation of a crystalline structure with a diffraction spot resulting from a typical pattern for Cr2S3 (Figure 3D). Moreover, there could also be some defects and/dislocations in the structure marked by the broken red lines (Supplementary Figure S3). It has been proved that defects favor electrocatalysis in water splitting reactions (Xie et al., 2013; Fan et al., 2018). They introduce unsaturated sites, thereby increasing the conductivity and catalytic activity.
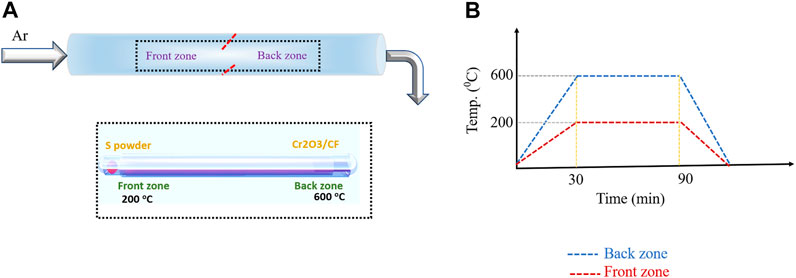
FIGURE 1. Controllable synthesis of Cr2S3. (A) Two-zone chemical vapor deposition setup with small tubes for concentrating the vapors. (B) Temperature profile for sulfurization reaction.
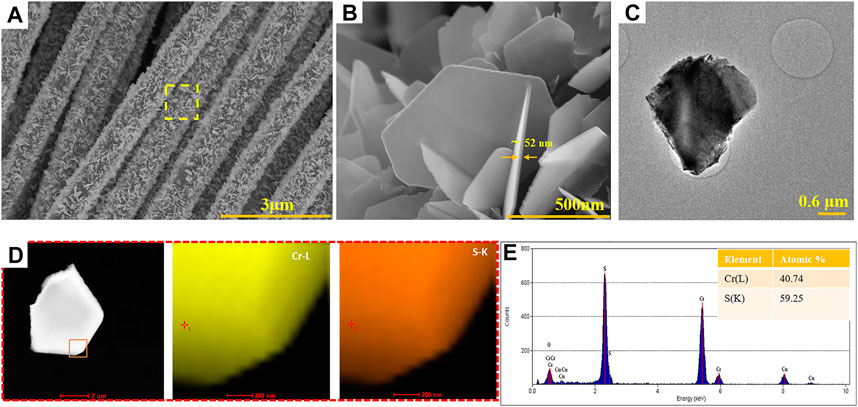
FIGURE 2. Morphology and elemental analysis: SEM (A,B) and TEM (C) images of Cr2S3. HAADF-STEM elemental mapping (D) and EDS elemental composition (E) of Cr2S3.
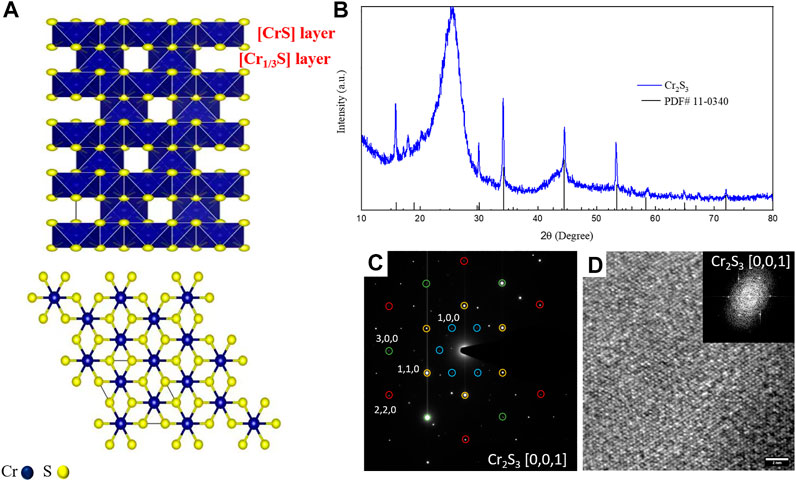
FIGURE 3. Crystal structure analysis. (A) Structural models of rhombohedral Cr2S3 showing top view and perspective view. XRD pattern (B), SAED (C) and HRTEM (D) image of Cr2S3.
Having proved the successful sulfurization, we tested the electrocatalytic performance toward oxygen evolution reaction (OER) via a three-electrode electrochemical cell consisting of saturated calomel electrode, Pt wire, and the synthesized sample (without any treatment) as reference, counter, and working electrodes, respectively. The unsulfurized (i.e., Cr2O3) and the bare CF samples were also tested for the sake of comparison. Figure 4A shows the anodic scan linear sweep voltammetry (LSV) polarization curves elucidating the electrocatalytic activity toward OER. It can be seen that the catalytic activity due to the bare substrate is negligible, substantiating the fact that the activities observed on other samples are solely from the grown materials. Accordingly, the sulfurized product exhibits a significantly improved activity toward OER as compared to the oxide counterparts. It requires overpotentials of only 230 mV and 300 mV to deliver current densities of 10 mA cm−2 and 30 mA cm−2, respectively. To get a clear picture of the intrinsic activity of the materials, we normalized the polarization curves with respect to electrochemically activated surface area (ECSA). Supplementary Figure S4 illustrates that there is still a significant enhancement in the catalytic activity for Cr2S3 as compared to its oxide counterpart. The OER performance reported in this study is compared fairly with most of the other recently reported materials (Supplementary Table S1). In fact, the OER process depends on the interaction strength of various intermediates with the surface of the catalyst (Equations 1–4). In the case of metal oxide, the increased 3d–2P repulsion between the metal d-band center and the coordinated oxygen P-band center results in the weakening of M-OH interaction. In the case of metal sulfides, with a more electronegative S, they accelerate the formation of ‒OOH intermediates from the adsorbed OH, thereby generating O2 gas (Joo et al., 2019). It is noteworthy that metal sulfides and phosphides turn into a more active surface during OER catalysis owing to the formation of the amorphous oxide phase contributing greatly to the enhancement of the catalytic reaction (Li et al., 2019; Shifa et al., 2021).
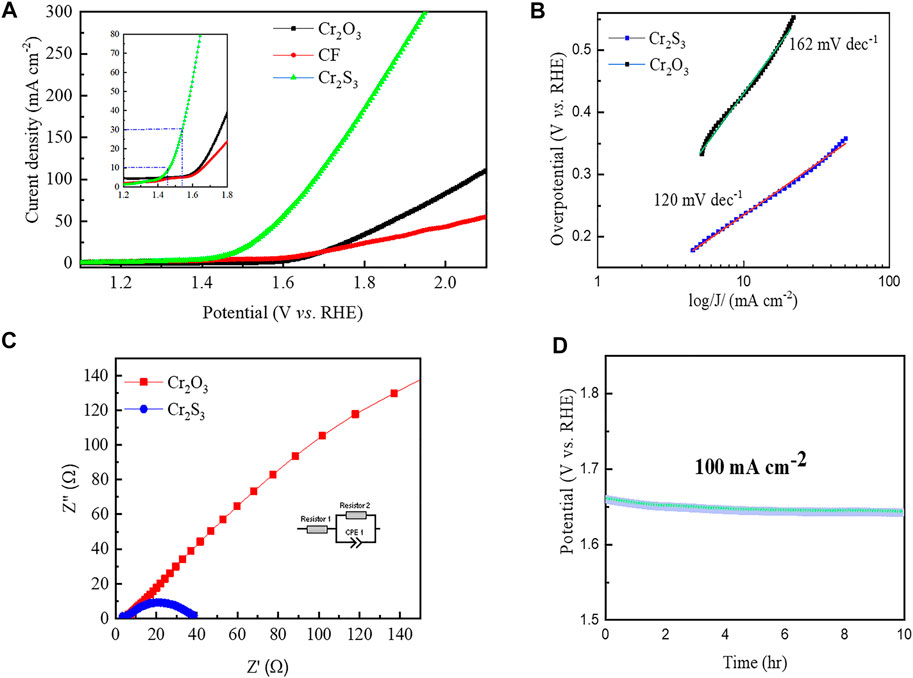
FIGURE 4. Catalytic performance for the oxygen evolution reaction. (A) Anodic scan of linear sweep voltammetry curves (inset shows the magnified plot for a maximum current density of 80 mAcm−2). (B) Tafel plot extracted from the linear sweep voltammetry curves. (C) Nyquist plots at an overpotential of 300 mV with an inset showing the equivalent circuit model. (D) Chronopotentiometry at 100 mAcm−2 showing the durability test.
The enhanced catalytic activity after sulfurization is even more evident from the Tafel plot analysis, derived from the polarization curve. It suggests the overpotential increment necessary to raise the current density by tenfold. Figure 4B shows the smaller Tafel slope for Cr2S3 (120 mV dec−1) as compared to Cr2O3 (162 mV dec−1). The smaller the Tafel slope, the better the kinetics of the electrochemical reaction in electrocatalysis, and hence, the OER rate will rapidly increase with the application of overpotential.
Another important figure of merits in OER catalysis is conductivity (or resistivity). Due to the low intrinsic electrical conductivity, metal oxides commonly have high charge transfer resistance, resulting in large overpotentials (Matsumoto and Sato, 1986; Liu et al., 2017). We employed electrochemical impedance spectroscopy (EIS) analysis to evaluate the charge transfer resistance (Rct). As expected, the Rct value (35.2Ω) of the sulfurized product is much lower than its oxide counterparts substantiated from the small semicircle in the Nyquist plot of Figure 4C (inset shows the equivalent circuit model, and the fitting detail is provided in the supporting information, Supplementary Figure S5).
Stability is a vital criterion to evaluate electrocatalysts for broader applications. The sulfurized product not only exhibits enhanced performance but also shows remarkable durability in OER catalysis. Figure 4D shows the chronopotentiometry result at 100 mA cm−2, showing the long-term stability of the catalysts without noticeable degradation.
To uncover the underlying mechanism of the enhanced OER activity, we estimated electrochemical active surface area (ECSA) via experimentally determining the electrochemical double-layer capacitance (Cdl). The ECSA of a material with similar composition is proportional to its Cdl value, and the Cdl value can be obtained from the slope of a linear curve constructed from half of the change in current density vs. scan rates. For this, cyclic voltammetries at various scan rates were run in the non-Faradaic region. The faster scan rates lead to a decrease in the size of the diffusion layer; as a consequence, higher currents are observed and thereby the current increases at faster voltage scan rates (Figures 5A,B). The higher value of Cdl for the sulfurized product in Figure 5C indicates that Cr2S3 exposes highest active sites that could be associated with the presence of unsaturated surfaces, dangling bonds, defects, and/or dislocation as has been observed in the HRTEM image (Figure 3C). This kind of active site exposure is supposed to be one of the possible reasons for enhanced OER performance.
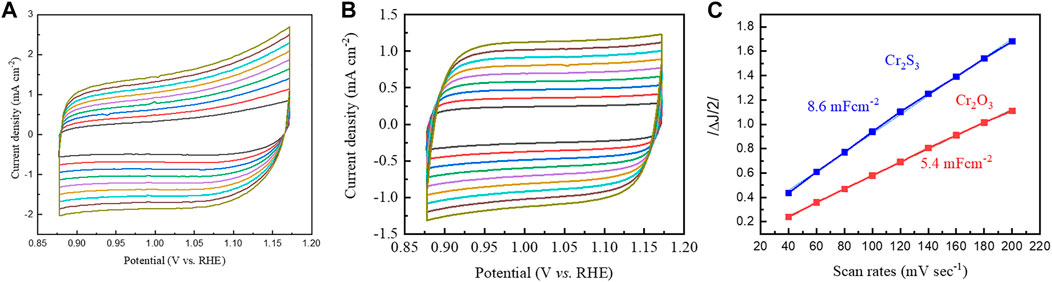
FIGURE 5. Cyclic voltammetries of Cr2S3(A) and Cr2O3(B) made at various scan rates in the non-Faradaic region. (C) The current density as a function of scan rate curves derived from (A) and (B).
Conclusion
In summary, we have controllably synthesized Cr2S3via sulfurization of Cr2O3 in a two-zone CVD method for electrocatalysis of OER. The two small tubes inside the main CVD chamber played a critical role to confine the system and hence saturate the sulfur vapor, thereby meeting the target at the deposition zone. Various characterization techniques reveal the formation of crystalline pure phase rhombohedral Cr2S3 without any impurities. Benefitting from the exposed active sites, Cr2S3 exhibits a remarkable electrocatalytic performance toward OER in an alkaline environment. It requires only 230 mV vs. RHE to deliver a current density of 10 mAcm−2 with robust stability for over 10 h of duration. Our synthesis design strategy is generally applicable, opening up a fresh insight into the advancement of nonlayered 2D materials. Future interest may lie in further optimization of the catalyst to be employed for overall water splitting in both acidic and alkaline media.
Data Availability Statement
The original contributions presented in the study are included in the article/Supplementary Material; further inquiries can be directed to the corresponding authors.
Author Contributions
TS initiated the idea, performed the synthesis, conducted characterizations, and wrote the manuscript. RM and VM conducted the TEM and interpreted the result. AV made overall supervision and led the project. All authors discussed, reviewed, and approved the manuscript.
Conflict of Interest
The authors declare that the research was conducted in the absence of any commercial or financial relationships that could be construed as a potential conflict of interest.
Acknowledgments
The authors acknowledge the Kempe Foundation (JCK1505, JCK1703, SMK 1839), the Knut och Alice Wallenberg Foundation (grant number KAW 2016.346), the ÅFORSK Foundation, and the Luleå University of Technology for financial support. The authors would also like to thank NSC, Tetralith, for allocation of high-performance computing time and resources (projects SNIC 2019/3-450 and SNIC 2019/3-684), through the Swedish National Infrastructure for Computing (SNIC). RM and VM acknowledge the EU community (Graphene Flagship Core 3, grant agreement ID 881603).
Supplementary Material
The Supplementary Material for this article can be found online at: https://www.frontiersin.org/articles/10.3389/fceng.2021.703812/full#supplementary-material
References
Arif, M., Yasin, G., Luo, L., Ye, W., Mushtaq, M. A., Fang, X., et al. (2020). Hierarchical Hollow Nanotubes of NiFeV-Layered Double hydroxides@CoVP Heterostructures towards Efficient, pH-Universal Electrocatalytical Nitrogen Reduction Reaction to Ammonia. Appl. Catal. B: Environ. 265, 118559. doi:10.1016/j.apcatb.2019.118559
Arif, M., Yasin, G., Shakeel, M., Mushtaq, M. A., Ye, W., Fang, X., et al. (2021). Highly Active Sites of NiVB Nanoparticles Dispersed onto Graphene Nanosheets towards Efficient and pH-Universal Overall Water Splitting. J. Energ. Chem. 58, 237–246. doi:10.1016/j.jechem.2020.10.014
Baek, J., Yun, H. J., Yun, D., Choi, Y., and Yi, J. (2012). Preparation of Highly Dispersed Chromium Oxide Catalysts Supported on Mesoporous Silica for the Oxidative Dehydrogenation of Propane Using CO2: Insight into the Nature of Catalytically Active Chromium Sites. ACS Catal. 2, 1893–1903. doi:10.1021/cs300198u
Bhimanapati, G. R., Lin, Z., Meunier, V., Jung, Y., Cha, J., Das, S., et al. (2015). Recent Advances in Two-Dimensional Materials beyond Graphene. ACS Nano 9, 11509–11539. doi:10.1021/acsnano.5b05556
Chhowalla, M., Shin, H. S., Eda, G., Li, L.-J., Loh, K. P., and Zhang, H. (2013). The Chemistry of Two-Dimensional Layered Transition Metal Dichalcogenide Nanosheets. Nat. Chem 5, 263–275. doi:10.1038/nchem.1589
Cook, T. R., Dogutan, D. K., Reece, S. Y., Surendranath, Y., Teets, T. S., and Nocera, D. G. (2010). Solar Energy Supply and Storage for the Legacy and Nonlegacy Worlds. Chem. Rev. 110, 6474–6502. doi:10.1021/cr100246c
Cui, F., Zhao, X., Xu, J., Tang, B., Shang, Q., Shi, J., et al. (2020). Controlled Growth and Thickness‐Dependent Conduction‐Type Transition of 2D Ferrimagnetic Cr 2 S 3 Semiconductors. Adv. Mater. 32, 1905896. doi:10.1002/adma.201905896
Deng, D., Novoselov, K., Fu, Q., Zheng, N., Tian, Z., and Bao, X. (2016). Undefined Catalysis with Two-Dimensional Materials and Their Heterostructures. nature.com. Available at: https://www.nature.com/nnano/journal/v11/n3/abs/nnano.2015.340.html (Accessed June 19, 2019).
Dou, Y., He, C.-T., Zhang, L., Yin, H., Al-Mamun, M., Ma, J., et al. (2020). Approaching the Activity Limit of CoSe2 for Oxygen Evolution via Fe Doping and Co Vacancy. Nat. Commun. 11, 1664. doi:10.1038/s41467-020-15498-0
Du, X., Su, H., and Zhang, X. (2020). Cr Doped-Co9S8 Nanoarrays as High-Efficiency Electrocatalysts for Water Splitting. J. Alloys Compd. 824, 153965. doi:10.1016/j.jallcom.2020.153965
Fan, X., Liu, Y., Chen, S., Shi, J., Wang, J., Fan, A., et al. (2018). Defect-enriched Iron Fluoride-Oxide Nanoporous Thin Films Bifunctional Catalyst for Water Splitting. Nat. Commun. 9, 1809. doi:10.1038/s41467-018-04248-y
Gao, R., and Yan, D. (2020). Recent Development of Ni/Fe‐Based Micro/Nanostructures toward Photo/Electrochemical Water Oxidation. Adv. Energ. Mater. 10, 1900954. doi:10.1002/aenm.201900954
Gao, R., Zhang, H., and Yan, D. (2017). Iron Diselenide Nanoplatelets: Stable and Efficient Water-Electrolysis Catalysts. Nano Energy 31, 90–95. doi:10.1016/j.nanoen.2016.11.021
Geim, A. K., and Grigorieva, I. V. (2013). Van der Waals heterostructures. Nature 499, 419–425. doi:10.1038/nature12385
Joo, J., Kim, T., Lee, J., Choi, S. I., and Lee, K. (2019). Morphology‐Controlled Metal Sulfides and Phosphides for Electrochemical Water Splitting. Adv. Mater. 31, 1806682. doi:10.1002/adma.201806682
Li, W., Xiong, D., Gao, X., and Liu, L. (2019). The Oxygen Evolution Reaction Enabled by Transition Metal Phosphide and Chalcogenide Pre-catalysts with Dynamic Changes. Chem. Commun. 55, 8744–8763. doi:10.1039/C9CC02845E
Lin, Y., Tian, Z., Zhang, L., Ma, J., Jiang, Z., Deibert, B. J., et al. (2019). Chromium-ruthenium Oxide Solid Solution Electrocatalyst for Highly Efficient Oxygen Evolution Reaction in Acidic media. Nat. Commun. 10, 162. doi:10.1038/s41467-018-08144-3
Liu, K., Zhang, C., Sun, Y., Zhang, G., Shen, X., Zou, F., et al. (2017). High-Performance Transition Metal Phosphide Alloy Catalyst for Oxygen Evolution Reaction. ACS Nano 12, 158–167. doi:10.1021/acsnano.7b04646
Maignan, A., Bréard, Y., Guilmeau, E., and Gascoin, F. (2012). Transport, Thermoelectric, and Magnetic Properties of a Dense Cr2S3 Ceramic. J. Appl. Phys. 112, 013716. doi:10.1063/1.4736417
Matsumoto, Y., and Sato, E. (1986). Electrocatalytic Properties of Transition Metal Oxides for Oxygen Evolution Reaction. Mater. Chem. Phys. 14, 397–426. doi:10.1016/0254-0584(86)90045-3
Murray, L. J., Yano, J., Chavan, S., Bordiga, S., Brown, C. M., Long, J. R., et al. (2010). Highly-Selective and Reversible O2 Binding in Cr3(1,3,5-Benzenetricarboxylate)2. J. Am. Chem. Soc. 132, 7856–7857. doi:10.1021/ja1027925
Mushtaq, M. A., Arif, M., Fang, X., Yasin, G., Ye, W., Basharat, M., et al. (2021). Photoelectrochemical Reduction of N2 to NH3 under Ambient Conditions through Hierarchical MoSe2@g-C3n4 Heterojunctions. J. Mater. Chem. A. 9, 2742–2753. doi:10.1039/D0TA10620H
Oakes, L., Carter, R., Hanken, T., Cohn, A. P., Share, K., Schmidt, B., et al. (2016). Interface Strain in Vertically Stacked Two-Dimensional Heterostructured Carbon-MoS2 Nanosheets Controls Electrochemical Reactivity. Nat. Commun. 7, 11796. doi:10.1038/ncomms11796
Park, H., Lee, E., Lei, M., Joo, H., Coh, S., and Fokwa, B. P. T. (2020). Canonic‐Like HER Activity of Cr1-xMoxB2 Solid Solution: Overpowering Pt/C at High Current Density. Adv. Mater. 32, 2000855. doi:10.1002/adma.202000855
Peng, L., Shah, S. S. A., and Wei, Z. (2018). Recent Developments in Metal Phosphide and Sulfide Electrocatalysts for Oxygen Evolution Reaction. Chin. J. Catal. 39, 1575–1593. doi:10.1016/S1872-2067(18)63130-4
Shifa, T. A., and Vomiero, A. (2019). Confined Catalysis: Progress and Prospects in Energy Conversion. Adv. Energ. Mater. 9, 1902307. doi:10.1002/aenm.201902307
Shifa, T. A., Wang, F., Liu, Y., and He, J. (2018). Heterostructures Based on 2D Materials: A Versatile Platform for Efficient Catalysis. Adv. Mater. 31, 1804828. doi:10.1002/adma.201804828
Shifa, T. A., Yusupov, K., Solomon, G., Gradone, A., Mazzaro, R., Cattaruzza, E., et al. (2021). In Situ-Generated Oxide in Sn-Doped Nickel Phosphide Enables Ultrafast Oxygen Evolution. ACS Catal. 11, 4520–4529. doi:10.1021/acscatal.1c00476
Tahir, M., Pan, L., Idrees, F., Zhang, X., Wang, L., Zou, J.-J., et al. (2017). Electrocatalytic Oxygen Evolution Reaction for Energy Conversion and Storage: A Comprehensive Review. Nano Energy 37, 136–157. doi:10.1016/j.nanoen.2017.05.022
Tan, S. M., and Pumera, M. (2019). Two-Dimensional Materials on the Rocks: Positive and Negative Role of Dopants and Impurities in Electrochemistry. ACS Nano 13, 2681–2728. doi:10.1021/acsnano.8b07795
Wan, W., Triana, C. A., Lan, J., Li, J., Allen, C. S., Zhao, Y., et al. (2020). Bifunctional Single Atom Electrocatalysts: Coordination-Performance Correlations and Reaction Pathways. ACS Nano 14, 13279–13293. doi:10.1021/acsnano.0c05088
Wang, F., Wang, Z., Shifa, T. A., Wen, Y., Wang, F., Zhan, X., et al. (2017). Two-Dimensional Non-Layered Materials: Synthesis, Properties and Applications. Adv. Funct. Mater. 27, 1603254. doi:10.1002/adfm.201603254
Xie, J., Zhang, H., Li, S., Wang, R., Sun, X., Zhou, M., et al. (2013). Defect-Rich MoS2Ultrathin Nanosheets with Additional Active Edge Sites for Enhanced Electrocatalytic Hydrogen Evolution. Adv. Mater. 25, 5807–5813. doi:10.1002/adma.201302685
Xie, L., Wang, J., Li, J., Li, C., Zhang, Y., Zhu, B., et al. (2020). An Atomically Thin Air‐Stable Narrow‐Gap Semiconductor Cr 2 S 3 for Broadband Photodetection with High Responsivity. Adv. Electron. Mater., 2000962. doi:10.1002/aelm.202000962
Xu, D., Stevens, M. B., Rui, Y., DeLuca, G., Boettcher, S. W., Reichmanis, E., et al. (2018). The Role of Cr Doping in Ni Fe Oxide/(oxy)hydroxide Electrocatalysts for Oxygen Evolution. Electrochimica Acta 265, 10–18. doi:10.1016/j.electacta.2018.01.143
Yang, J., Wang, Y., Lagos, M. J., Manichev, V., Fullon, R., Song, X., et al. (2019). Single Atomic Vacancy Catalysis. ACS Nano 13, 9958–9964. doi:10.1021/acsnano.9b05226
Yao, L., Zhang, N., Wang, Y., Ni, Y., Yan, D., and Hu, C. (2018). Facile Formation of 2D Co 2 P@Co 3 O 4 Microsheets through In-Situ Toptactic Conversion and Surface Corrosion: Bifunctional Electrocatalysts towards Overall Water Splitting. J. Power Sourc. 374, 142–148. doi:10.1016/j.jpowsour.2017.11.028
Zhang, B., Wang, L., Cao, Z., Kozlov, S. M., García de Arquer, F. P., Dinh, C. T., et al. (2020). High-valence Metals Improve Oxygen Evolution Reaction Performance by Modulating 3d Metal Oxidation Cycle Energetics. Nat. Catal. 3, 985–992. doi:10.1038/s41929-020-00525-6
Keywords: 2D materials, nonlayered materials, metal sulfides, chemical vapor deposition, oxygen evolution reaction
Citation: Shifa TA, Mazzaro R, Morandi V and Vomiero A (2021) Controllable Synthesis of 2D Nonlayered Cr2S3 Nanosheets and Their Electrocatalytic Activity Toward Oxygen Evolution Reaction. Front. Chem. Eng. 3:703812. doi: 10.3389/fceng.2021.703812
Received: 30 April 2021; Accepted: 07 June 2021;
Published: 25 June 2021.
Edited by:
Hassan M. A. Hassan, Suez University, EgyptReviewed by:
Dongpeng Yan, Beijing Normal University, ChinaGuangbo Chen, Technische Universität Dresden, Germany
Copyright © 2021 Shifa, Mazzaro, Morandi and Vomiero. This is an open-access article distributed under the terms of the Creative Commons Attribution License (CC BY). The use, distribution or reproduction in other forums is permitted, provided the original author(s) and the copyright owner(s) are credited and that the original publication in this journal is cited, in accordance with accepted academic practice. No use, distribution or reproduction is permitted which does not comply with these terms.
*Correspondence: Tofik Ahmed Shifa, dG9maWsuYWhtZWQuc2hpZmFAbHR1LnNl; Alberto Vomiero, YWxiZXJ0by52b21pZXJvQGx0dS5zZQ==