- 1Oak Ridge National Laboratory, Nuclear Energy and Fuel Cycle Division, Oak Ridge, TN, United States
- 2Nonreactor Nuclear Facilities Division, Oak Ridge, TN, United States
- 3Radioisotope Science and Technology Division, Oak Ridge, TN, United States
- 4Materials Science and Technology Division, Oak Ridge, TN, United States
Molten chloride salts (including MgCl2, KCl, NaCl, and ZnCl2) are being considered for heat transfer media for renewable (solar) and nuclear power generators, as fuel carrier for nuclear reactors, and as thermal energy storage media. Impurities such as oxygen, hydroxides, moisture, and sulfur are known to negatively influence the corrosion of materials in contact with the salt (e.g., structural metals). Commercially available chloride salts come with a range of impurities. Before using the chloride salts at high temperature, it is desirable to remove the impurities to increase the performance of the salt and reduce corrosion. In this study, we tested the use of thionyl chloride vaporized into a stream of argon to react with oxygenated impurities in a mixture of MgCl2-KCl-NaCl, removing them as HCl and SO2. The reagent was bubbled through the salt when both above and below the melting point. The reaction was followed using thermocouple data from the salt and by Fourier transform infrared (FTIR) spectroscopy on the exhaust of the reactor. The reaction kinetics were followed by comparing the peaks from SO2 product to SOCl2 reagent in the FTIR spectra. The purity of the salt was assessed at the end of the purification process by x-ray diffraction and inductively coupled plasma analysis. Although the process was effective in removing the oxygen content of the mixture, ternary compounds were formed in the process, including KNiCl3 and KMgCl3. The nickel in KNiCl3 came from the reaction between the salt and the nickel vessel. Thus, these experiments suggest that improvements to the process must be made before using SOCl2 vapors for the purification of chloride salts.
Introduction
Concentrating solar power (CSP) for electricity generation has several advantages over distributed networks of photovoltaic panels (Lovegrove and Stein, 2021). CSP facilities have the economy of scale, and they can take advantage of the existing grid infrastructure and the current paradigm of centralized power generation. However, solar in all its forms relies on direct solar irradiance that fluctuates based on weather as well as the diurnal cycle. For concentrating solar to be considered as a feasible replacement for base-load fossil generation, energy storage must be part of the implementation, either using batteries or thermal energy storage. Molten salt mixtures have been developed for thermal energy storage because of their stability and high operating temperatures, which allow better thermodynamic efficiency in converting heat to electricity.
Sandia National Laboratories (SNL) has been leading research into the use of salts as thermal storage media and has published extensively on this topic (Armijo et al., 2020). The current state-of-the-art for thermal energy storage is a NaNO3-KNO3 eutectic that can operate up to 600°C. A range of novel thermal energy storage media have been proposed to improve economics through higher efficiency power conversion (Mehos et al., 2017). Chloride salts are a promising media which allows operation at higher temperatures, 750°C, and is stable up to 1400°C. A ternary chloride salt has been studied for this purpose, carnallite, is a mixture of NaCl-MgCl2-KCl with a melting point of 380–426°C. It has the additional advantages of being available at a low cost. However, chloride salts are highly corrosive, especially if there is any oxygen present in the mixture. They also have relatively low thermal conductivity—0.4 W/mK at 700°C, which is slightly less than nitrate salts at 500°C—and an order of magnitude lower than liquid sodium metal. In the SNL analysis of a 2 MWth facility, liquid sodium is passed through a tower receiver placed in the center of a field of solar reflectors. The sodium transfers heat to carnallite or the MgCl2-KCl-NaCl mixture. The heated carnallite is either directed towards a super-critical CO2 cycle for power generation or to a thermal energy storage reservoir. To support advancement of molten chloride salt technology, Oak Ridge National Laboratory (ORNL) is in the process of building a pumped-chloride test loop, a schematic of which is shown in Figure 1. The facility has been filled with MgCl2-KCl-NaCl with a nominal composition of (44–50; 48–33; and 2.5–10 weight % respectively), formed by a combination of two commercial salts [anhydrous Carnallite provided by Israel Chemicals Ltd. (ICL) and SPK Halite from Albemarle Inc.]. The nominal salt composition is given in Supplementary Table S1.
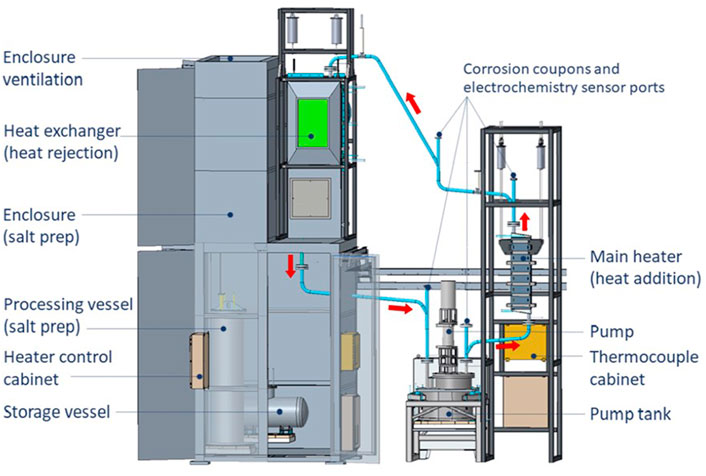
FIGURE 1. Layout of solar salt pumped loop, including a vessel for salt preprocessing, a heater, and a heat exchanger for heat rejection. Source: Robb et al., 2019.
To reduce corrosion of the metal alloys used to contain chloride salts to acceptable levels, impurities such as oxygen in the form of oxides, hydroxides, and sulfur must be removed to parts-per-million (ppm) concentrations (Kurley et al., 2019; Raiman et al., 2019; Pillai et al., 2021; Ezell et al., 2022). Carnallite cannot simply be dehydrated by heating it above 100°C because some of the water is chemically bound to the salt components. The options for salt purification were reviewed by Kurley and colleagues (Kurley et al., 2019): for instance, MgCl2 is stable as a hexahydrate, the last H2O of which is very difficult to remove by heating. Various means have been used to strip off the water molecules, including addition of a reducing agent such as magnesium metal or Mg-Cd alloy (Johnson et al., 1969; Zhao et al., 2019). Organic chlorinating agents can also be used, such as reaction with CCl4. The issue with this method is that the byproducts can be toxic organochlorine compounds and the process can become hazardous when operated on an industrial scale. This paper addresses the concern regarding the purity of the chloride salt mixture and investigates a novel way of reducing the oxygen activity in the salt, namely by reacting oxygen in the salt with a sparge of SOCl2 vapor in argon. The expected advantages to this method were that the reagent was in the liquid phase and could be handled more easily. Being a liquid allowed it to be introduced by sparging with an inert gas. The SOCl2 could be more easily handled in the off-gas of the reactor, with excess reagent being captured downstream of the reactor in a caustic scrubber. The possibility of a lower-temperature reaction, below the melting point of the salt, was also investigated.
Materials and Methods
To better quantify the initial conditions, rather than use the ICL salt, the carnallite to be purified was mixed from individual salts: NaCl (>99.5%Sigma-Aldrich lot #SLBV9985), KCl (>99.5%, Merck lot #K47045236551), and MgCl2 (>98%, Sigma #SLBX8218). The components were individually loaded into a 15-cm-diameter nickel alloy vessel (C-276) that could be purged with a flow of ultra-high purity (UHP) argon up to 500 cm3/min. The sealed vessel was placed into a tubular furnace located in a walk-in fume hood. The complete system is shown in Figure 2, and the inside of the hood is delineated as the dashed red line. The system included the heated vessel containing the salt and an unheated glass vessel containing liquid SOCl2 through which UHP argon carrier gas was bubbled at flow rates up to 400 cm3/min. The input gas handling system is shown on the right-hand-side of the figure. The off-gas monitoring and treatment is shown on the left-hand-side of the figure. Because production of acidic gases was anticipated, the argon flow was passed through a series of scrubbers, including a packed soda lime bed, a 2 M NaOH(aq) bubbler, and a 2 M Ca(OH)2 (aq) bubbler. A steel wool filter was placed in the flow exhausted into the hood to capture any Cl2 that formed. The hood exhaust also passed through a HEPA filter. A schematic of the overall process is shown in Supplementary Figure S1.
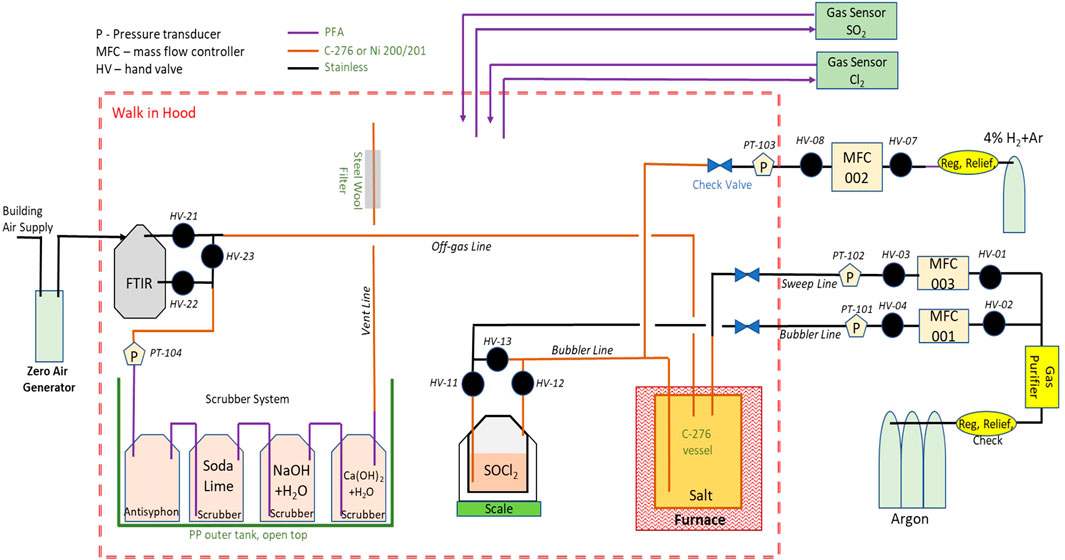
FIGURE 2. Schematic of the apparatus used for thionyl chloride purification of chloride salt. Shown in the figure are the vessels holding the salt and the thionyl chloride reagent at the bottom center, gas handling at the right on the outside of the walk-in hood (the dashed red line), post-reaction analysis of the off-gas by FTIR on the left hand side of the figure, and the four-part scrubber gas system (lower left).
The purification experiments took several days; the SOCl2 was first introduced into the salt when solid. The flow was continued as the salt was heated above its melting point. The various steps are listed in Table 1. Two batches of salt were purified: 2.159 kg (1,027 g MgCl2, 806 g KCl, and 316 g NaCl) and 6.096 kg (2,875 g MgCl2, 2,342 g KCl, and 880 g NaCl). These were chosen to represent the eutectic composition (Mohan et al., 2018). The apparatus was instrumented with three thermocouples measuring the temperature gradient through the furnace, gas flow monitors on the UHP argon, and off-gas monitored by passing it through a transmission gas cell probed by a Bomem FTIR spectrometer. Because the reactions were being monitored frequently, the purification processes were undertaken following several steps over 7 days as indicated in Table 1. More than half of that time the SOCl2 bubbler was by-passed and the reactor was purged with argon. The salt was kept at temperature throughout the run. The SOCl2 vessel was placed on a balance to measure the total amount used during the runs. Balance readings from the first run indicated that the amount of SOCl2 that passed through the salt was 134 g; however, that number has a high uncertainty, ± 20%, because the gas line connections may have affected the readings. Thus, the balance was not used in the second run. The amount of SOCl2 passing through the reactor unreacted was not assessed.
After the purification process, liquid samples were removed from the reactor at 600°C through a sampling tube. There are no standard characterization methods for salt samples, so traditional laboratory methods were used. The samples were sealed under argon and were sent for inductively coupled plasma mass spectrometry (ICP-MS) for determination of elemental composition. The samples were also examined by scanning electron microscopy (SEM) electron dispersive x-ray spectroscopy (EDS), as well as x-ray diffraction analysis (XRD). The SEM-EDS was used to detect oxygen as well as the other major salt components, and the XRD was used to assess binary or ternary phases. The oxygen content of the purified salt was determined by the standard method of titration with HCl (Kurley et al., 2019). The salts were also assessed after the purification process by thermogravimetric analysis (TGA) using a Setaram instrument to assess whether the water of hydration been removed by the purification process.
Results
The FTIR proved to be a valuable tool to monitor the reaction as it was progressing; the reagents and the products all had strong infrared signals that were well separated in the mid-IR. The infrared active frequencies used in this study are given in Table 2. The transmission of the CaCl2 window dropped quickly below 1,000 cm−1, but the FTIR still allowed us to distinguish the strong absorptions of SO2 and SOCl2.
Figure 3 shows overlayed FTIR spectra from 0 to 127 min after the SOCl2 sparge was started through the molten salt. These spectra were normalized to a reference spectrum taken at the start of the experiment before introduction of the SOCl2. The FTIR was purged with argon throughout the experiment, but the increase in CO2 over time shows a slow leak into the body of the instrument. The H2O peaks are likely derived from free water evolution from the salt, since they did not change much during the experiment. None of the peaks from the products of the purification (i.e., SO2 and HCl) decreased significantly, even after the reaction was terminated. Their signals slowly decreased when the gas cell had been purged with argon for 2 h. This suggested that these gases remained in the gas cell even though it was trace heated to 100°C, perhaps depositing on the CaF2 windows.
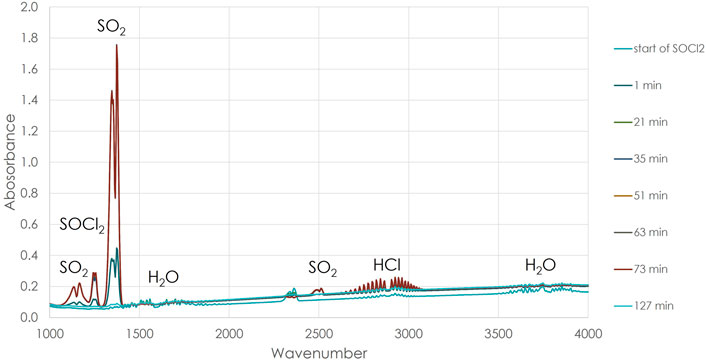
FIGURE 3. Overlayed FTIR spectra showing the increased production of SO2 over time—the units in the legend are minutes after the SOCl2 was valved into the system. Increases were also observed in the HCl peak but were smaller than those for SO2.
During the process, the SOCl2 signature at 1,250 cm−1 was quickly replaced with the SO2 peaks at 1,150 and 1,300 cm−1, showing that there was a rapid reaction of the salt with thionyl chloride. As the reaction progressed, the peaks associated with HCl (2,700–3,100 cm−1) also increased. Eventually, the SOCl2 was no longer being consumed, and its IR peak started to increase relative to those of the SO2. This indicated that the purification process was complete. When the salt was heated above 600°C, peaks corresponding to HCl and H2O increased significantly in the FTIR, and a very strong broad IR signal was observed that corresponded to the published spectrum of MgCl2 between 3,100 and 3,550 cm−1. Unfortunately, the deposition of MgCl2 on the gas cell windows meant that the IR spectrometer could no longer be used for the experiment. The increase in the signal of these species likely arises from revaporization of deposited material in the tubing coming from the reactor. At the highest reactor temperatures, 635 ± 15°C, the top of the crucible was measured as 507°C. This was high enough to cause secondary volatilization of the reaction products. So, although the FTIR was a useful tool to monitor the progress of the reaction, the deposition and revolatilization of reaction products through the tubing to the gas cell and the long residence time of the reaction products in the gas cell meant that the spectra could not be used for a chemical kinetic analysis.
The purified samples were analyzed by inductively-coupled plasma mass spectrometry (ICP-MS). Results are presented in Table 3 for the salts before and after the purification processes. Because the ICP-MS could not give oxygen analysis, oxygenated compounds were looked for via SEM and the salt was also analyzed by titration. The SEM shows the presence of oxygen, as shown in Figure 4. The analysis of the salt by titration was 14 μg/g in the second test. Note the XRD did not quantitatively capture the presence of sodium chloride because of its lower concentration than the other components.
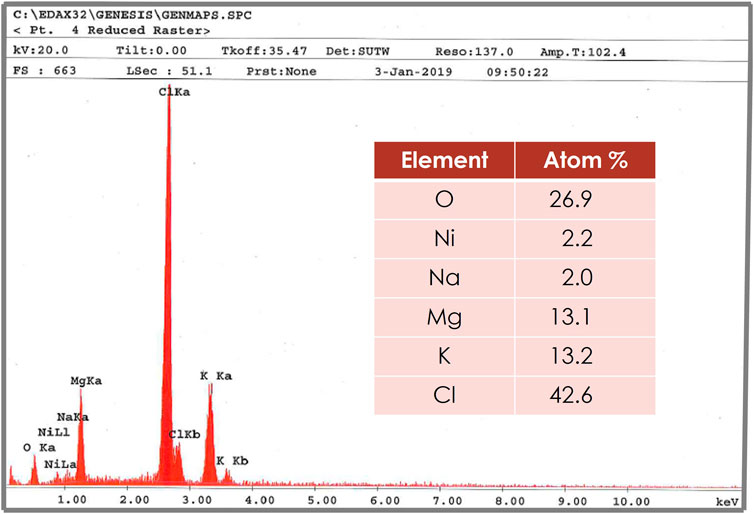
FIGURE 4. SEM analysis of purified salt showing the main elements including potassium, magnesium, and sodium chlorides. Some oxygen was observed, which was further quantified by titration.
The salt samples, which were white when introduced into the reaction vessel, became pink after the purification process. The source of the color change was thought to have arisen from an impurity that was absorbed into the melt. The SEM analysis indicated that the metal involved in the color change could be nickel that could have come from the C-276. The XRD analysis shown in Figure 5 corroborates this analysis, as a potassium-nickel ternary chloride was found in the analysis, or KNiCl3.
A post-purification TGA analysis assessed whether the water of hydration had been stripped from the salt. The results are shown in Figure 6. This chart shows that even when handled with care, the salt quickly absorbs water from the atmosphere, showing up as dehydration peaks between 100 and 200°C. The higher levels of hydration were not observed, which would have been observed above 300°C had they been present. The sharp peak at 400°C corresponds to the melting point of the KCl-MgCl2 mixture, the main components of the carnallite salt.
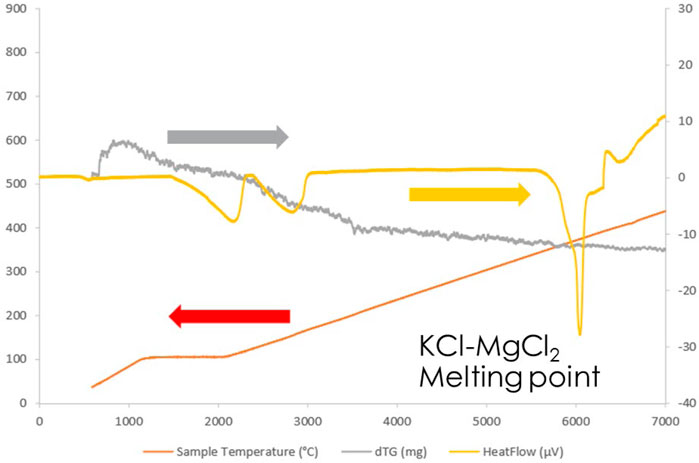
FIGURE 6. Thermogravimetric analysis of purified carnallite. The red line is the heating profile of the sample, with the temperatures shown in °C on the left axis. The grey line shows the mass loss in mg and the yellow line shows the heat flux in μV on the right axis.
Discussion
The purification of chloride molten salts needs to be operated under difficult chemical conditions. Kurley, et al. (2019) used thermodynamics to illustrate why HCl alone is not enough to remove MgO, the thermodynamically favored product when water is present with MgCl2. The current industrial scale purification of chloride salts is hazardous: using Cl2, HCl, and/or electrical currents to achieve REDOX control, but are themselves hazardous and create hazardous byproducts. The current proposed method in the CSP community using pyrophoric metallic Mg may appear more benign but can generate substantial amounts of HCl off-gas and explosive H2. REDOX control by addition of Mg powder may also result in any unreacted Mg causing abrasion corrosion during flow. Excess Mg may react with containment metals as well as the oxygen-bearing species. Hence, this study investigated the use of a sparged room-temperature liquid, SOCl2, to test a chlorination process to convert hydrates and hydroxides entrained in the salt into vaporized HCl and H2O.
Sparging the salt with room temperature SOCl2 successfully removed hydration H2O and O2 from the salt. The O2 content by titration was 14 ug/g after purification, but the salt is extremely hygroscopic and needs protection even during sampling. Most of the sulfur from the SOCl2 was transported to the scrubber as SO2. Little sulfur was retained in the salt. There appeared to be contamination of the purified salt with nickel coming from reaction of the nickel vessel or sparging tube to form KNiCl3. Because these purification tests were not carried out in different vessels, it is unclear if this reaction was promoted by the presence of SOCl2. Nickel has been reported elsewhere to form Ni(II) chlorides (Seifert, 1988), especially in the presence of acidic gases such as HCl and SOCl2 (e.g., Allamagny, 1960) and in mixtures of chloride salts (Lemaire et al., 1997). Removal of the nickel species can be done by filtration, after a secondary H2 reduction step if necessary to form an insoluble precipitate.
Results from this study were to have been applied to the purification of the batch of salt used to fill the loop being constructed at ORNL. Although the purification procedure was successful in removing oxygen, it was decided that an alternative measure would be used. The main reasons for this were the reaction with nickel, a key component of nickel alloy C-276 from which the loop is fabricated, and the difficulties working with SOCl2. Ambrosek (2011) investigated the use of several methods for chloride salt purification, including the addition of magnesium metal to the molten salt. Following this methodology, success has been achieved in the removal of oxides and hydroxides to the Facility to Alleviate Salt Technology Risks (FASTR) molten chloride salt loop at ORNL.
Conclusion
The current approach to removing oxygen-containing species from a mixture of MgCl2-KCl-NaCl for CSP applications is to add copious amounts of Mg, a process that is characterized by large releases of HCl. As the effectiveness of the process will be sensitive to the physical and chemical state of the Mg and the amount of Mg introduced versus the initial purity of the salt, there will be difficulties in scaling this process to an industrial size. Hence, an alternative purification process was investigated that suggested easier control, as the reagent was a sparged liquid, and better scalability. In this study, MgCl2-KCl-NaCl was purified by sparging the molten salt with SOCl2 vapor entrained in a stream of argon. The salt purification method was tested with the salt as a solid and as a liquid, about 150°C above its melting point. SOCl2 reacted with the main contribution to bound water, the oxides and hydroxides associated with MgCl2.
Although the oxygen content of the salt was reduced to the ppm level, nickel in the vessel assembly, tubing, and reactor, reacted with the SOCl2, forming a ternary nickel compound, KNiCl3. These effects could be mitigated by choosing different materials for the reactor, and removal of any unwanted precipitates by filtration. SOCl2 was easily added to the reaction vessel as a sparged reagent and unreacted vapor was neutralized in the off-gas scrubbing system. However, SOCl2 is highly reactive and needs to be stored with care. These effects need to be considered in any scaled-up operation using this purification method.
Data Availability Statement
The raw data supporting the conclusion of this article will be made available by the authors, without undue reservation.
Author Contributions
JM participated in the experiments, analyzed the data, and drafted the manuscript for publication. GC participated in the experiments. JRM participated in the experiments. RM contributed to the project planning and experimental design. KR participated in the experiments and was principal investigator for the project. DS analyzed the salt samples after purification. All authors reviewed the manuscript before submission.
Funding
Funding for this project was provided by the US Department of Energy EERE Solar Energy Technologies Office agreement 33875.
Conflict of Interest
The authors declare that the research was conducted in the absence of any commercial or financial relationships that could be construed as a potential conflict of interest.
Licenses and Permissions
This manuscript has been authored by UT-Battelle, LLC, under contract DE-AC05-00OR22725 with the US Department of Energy (DOE). The US government retains and the publisher, by accepting the article for publication, acknowledges that the US government retains a nonexclusive, paid-up, irrevocable, worldwide license to publish or reproduce the published form of this manuscript, or allow others to do so, for US government purposes. DOE will provide public access to these results of federally sponsored research in accordance with the DOE Public Access Plan (http://energy.gov/downloads/doe-public-access-plan).
Publisher’s Note
All claims expressed in this article are solely those of the authors and do not necessarily represent those of their affiliated organizations, or those of the publisher, the editors and the reviewers. Any product that may be evaluated in this article, or claim that may be made by its manufacturer, is not guaranteed or endorsed by the publisher.
Supplementary Material
The Supplementary Material for this article can be found online at: https://www.frontiersin.org/articles/10.3389/fceng.2022.811513/full#supplementary-material
References
Allamagny, P. (1960). Synthesis of Double Fluorides by Reaction of Gaseous HF with Crystalline Chlorides. Paris, France: Bulletin de la Societe Chimique de France, 1099–1106.
Ambrosek, J. W. (2011). Molten Chloride Salts for Heat Transfer in Nuclear Systems (Madison, WI: University of Wisconsin). PhD Dissertation.
Armijo, K. M., Carlson, M. D., Dorsey, D., Christian, J. M., and Turchis, C. (2020). Design of a 2.0 MWth Sodium/molten Salt Pilot System. Albuquerque, NM: Sandia National Laboratory. SAND2020-5549C.
Coblentz Society, Inc. (2022). Evaluated Infrared Reference Spectra. NIST Chemistry WebBook, NIST Standard Reference Database Number 69, Eds. P.J. Linstrom and W.G. Mallard, National Institute of Standards and Technology, Gaithersburg MD, 20899, (retrieved April 4, 2022). doi:10.18434/T4D303
Ezell, N. D. B., Raiman, S. S., Kurley, J. M., and McDuffee, J. (2022). Neutron Irradiation of alloy N and 316L Stainless Steel in Contact with a Molten Chloride Salt. 3(3), 920–926. doi:10.1016/j.net.2020.07.042x
Johnson, T. R., Teats, F. G., and Pierce, R. D. (1969). A Method for the Purification of Molten Chloride Salts. Argonne National Laboratory. Lemont, IL. ANL-7603.
Kurley, J. M., Halstenberg, P. W., McAlister, A., Raiman, S., Dai, S., and Mayes, R. T. (2019). Enabling Chloride Salts for thermal Energy Storage: Implications of Salt Purity. RSC Adv. 9, 25602–25608. doi:10.1039/c9ra03133b
Lemaire, G., Hébant, P., and Picard, G. S. (1997). DFT Analysis of Interfacial Processes Occurring in the First Steps of Electrodeposition of Nickel from Chloride Melt. J. Mol. Struct. THEOCHEM 419, 1–10. doi:10.1016/s0166-1280(97)00250-9
Lovegrove, K., and Stein, W. (2021). Concentrating Solar Power Technology, Principles, Developments, and Applications. 2nd Edition. Cambridge, MA: Woodhead Publishing Series in Energy, Elsevier.
Mehos, M., Turchi, C., Vidal, J., Wagner, M., Ma, Z., Ho, C., et al. (2017). Concentrating Solar Power Gen3 Demonstration Roadmap. Golden, CO (United States): National Renewable Energy Laboratory. NREL/TP-5500-67464.
Mohan, G., Venkataraman, M., Gomez-Vidal, J., and Coventry, J. (2018). Assessment of a Novel Ternary Eutectic Chloride Salt for Next Generation High-Temperature Sensible Heat Storage. Energ. Convers. Manage. 167, 156–164. doi:10.1016/j.enconman.2018.04.100
Pillai, R., Raiman, S. S., and Pint, B. A. (2021). First Steps toward Predicting Corrosion Behavior of Structural Materials in Molten Salts. J. Nucl. Mater. 546, 152755. doi:10.1016/j.jnucmat.2020.152755
Raiman, S. S., Mayes, R. T., Kurley, J. M., Parrish, R., and Vogli, E. (2019). Amorphous and Partially-Amorphous Metal Coatings for Corrosion Resistance in Molten Chloride Salt. Solar Energ. Mater. Solar Cell 201, 110028. doi:10.1016/j.solmat.2019.110028
Robb, K., Mulligan, P., Yoder, G., Smith, K., and Massengale, J. (2019). Facility to Alleviate Salt Technology Risks (FASTR): Preliminary Design Report with Failure Modes and Effects Analysis. ORNL/TM-2019/1370. doi:10.2172/1615802
Seifert, H. J. (1988). Why Do Ternary Compounds Exist? - Thermoanalytical Investigations on Binary Systems of Metal Chlorides. J. Therm. Anal. 33 (1), 147–155. doi:10.1007/bf01914594
Keywords: carnallite purification, dehydration with SOCl2, chloride salts for thermal energy storage, concentrating solar power, formation of KNiCl3
Citation: McFarlane J, Del Cul GD, Massengale JR, Mayes RT, Robb KR and Sulejmanovic D (2022) Chloride Salt Purification by Reaction With Thionyl Chloride Vapors to Remove Oxygen, Oxygenated Compounds, and Hydroxides. Front. Chem. Eng. 4:811513. doi: 10.3389/fceng.2022.811513
Received: 08 November 2021; Accepted: 29 March 2022;
Published: 25 April 2022.
Edited by:
Sotira Yiacoumi, Georgia Institute of Technology, United StatesReviewed by:
J. Paul Chen, National University of Singapore, SingaporeJun Gao, Shandong University of Science and Technology, China
Copyright © 2022 McFarlane, Del Cul, Massengale, Mayes, Robb and Sulejmanovic. This is an open-access article distributed under the terms of the Creative Commons Attribution License (CC BY). The use, distribution or reproduction in other forums is permitted, provided the original author(s) and the copyright owner(s) are credited and that the original publication in this journal is cited, in accordance with accepted academic practice. No use, distribution or reproduction is permitted which does not comply with these terms.
*Correspondence: Joanna McFarlane, bWNmYXJsYW5lakBvcm5sLmdvdg==