- 1Centre for Environmental Policy, Imperial College London, London, United Kingdom
- 2Centre for Process Systems Engineering, Imperial College London, London, United Kingdom
- 3Ecosystems Services and Management Program, International Institute for Applied Systems Analysis, Laxenburg, Austria
- 4Grantham Institute – Climate Change and the Environment, Imperial College London, London, United Kingdom
The large-scale removal of carbon dioxide from the atmosphere is likely to be important in maintaining temperature rise “well below” 2°C, and vital in achieving the most stringent 1.5°C target. Whilst various literature efforts have estimated the global potential of carbon dioxide removal (CDR) for a range of technologies with different degrees of certainty, regional bottlenecks for their deployment remain largely overlooked. Quantifying these barriers, through national and local case studies, rather than with aggregated approaches, would guide policy and research, as well as investments, toward regions that are likely to play a prominent role in CDR deployment. Five CDR technologies—including afforestation/reforestation, bioenergy with carbon capture and storage, biochar, direct air capture and enhanced weathering—are compared in this work. We discuss main technical, socio-economic and regulatory bottlenecks that have been scarcely investigated at regional level, and provide directions for further research. We identify the availability of accessible land, water, low carbon energy and CO2 storage as key regional drivers and bottlenecks to most CDR technologies. We discuss the caveats in CO2 accounting in assessing the performance of each technology, and the need for an international regulatory framework which captures these differences. Finally, we highlight the social, economic and political drivers which are central in unlocking the large scale deployment of CDR technologies, in a cost attractive, socially acceptable and politically achievable way.
Introduction
Insufficient climate change mitigation action has led to the need for large-scale carbon dioxide removal (CDR) from the atmosphere to meet the Paris Agreement target (IPCC, 2018). Several technologies have been identified as capable of delivering CDR at scale: afforestation/reforestation (AR/RE), bioenergy with carbon capture and storage (BECCS), biochar, direct air carbon capture and storage (DACCS), and enhanced weathering of minerals (EW) (Minx et al., 2018). Other technologies, such as ocean fertilization exist, but were excluded from the study because of their limited potential, particularly when compared to alternative portfolio options (Fuss et al., 2018). The global and regional deployment potentials of these technologies—often in isolation—have been quantified, with different degrees of certainty. A recent review quantifies annual technical and sustainable CDR potentials in 2050 at 0.5–7 GtCO2 for AR/RE, 0.5–5 GtCO2 for BECCS, 0.3–2 GtCO2 for biochar, 0.5–5 GtCO2 for DACCS, and 2–4 GtCO2 for EW (Fuss et al., 2018). These estimates are conservative and account for both physical limits—land, water, CO2 storage, suitable minerals and energy availabilities—and broader environmental risks—biodiversity loss and albedo effects. Integrated Assessment Models (IAMs) represent the world in aggregated regions, consequently they are incapable of identifying the extent to which these factors represent regional bottlenecks for CDR deployment. Instead, CDR potentials are quantified in a rather coarse manner, by focusing on standalone CDR options (Azar et al., 2010; Klein et al., 2014; Popp et al., 2014), or by investigating two options (generally afforestation and BECCS) at the same time (Humpenöder et al., 2014; Harper et al., 2018). Case studies accounting for a compressive portfolio of CDR technologies and adopting a regional or national approach are currently scarce. The presence of such literature gaps has a 2-fold implication: (1) limited guidance toward implementation of CDRs at national or regional scale (2) difficult identification of key regions for CDR deployment based on site-specific factors.
In the following sections, we highlight regional enabling factors of CDR deployment that have received minimal investigation in the literature, and need further research.
From Global to Regional Bottlenecks of CDR Deployment
Land and Water
The effectiveness of land-based mitigation technologies—AR/RE, BECCS, Biochar and EW—are inherently dependent on land availability, and the quality and accessibility (here considered as proximity to infrastructure) of that land will impact the regional implementation of the CDR options differently. While largely unaffected by land accessibility issues, land availability and quality are critical to AR/RE potential. A key regional limitation for afforestation is also the potential reduction of the earth albedo effect in boreal/northern hemisphere areas (Smith et al., 2016a). Tropical regions are therefore the most attractive places for forest growth practices, because the combined effect of carbon sequestration and albedo change are assumed to lead to a net cooling (Kreidenweis et al., 2016). Whilst some authors have warned against potential side effects, such as food price increase and land use change (Calvin et al., 2014; Kreidenweis et al., 2016) of intense afforestation in such regions, case studies detailing such socio-economic impacts at the local level, are still scarce in the literature (Stoy et al., 2018).
In many regional approaches, the role of water and land for BECCS deployment is relatively nuanced, because of the 2-fold flexibility of biomass feedstock supply. Indeed, BECCS plants can rely on imported biomass feedstock (Fajardy et al., 2018), and opt for biomass feedstock, such as wastes (Pour et al., 2018), residues from forestry and agriculture (Creutzig et al., 2015), pulp and paper industry biomass (Onarheim et al., 2017), and algae biomass (Beal et al., 2018), which do not require dedicated plant growth. Similarly, biochar can be derived form a wide range of feedstock, with properties and utility differing with feedstock type and production condition, including pyrolysis temperature (Kwak et al., 2019). However, large scale deployment of BECCS and biochar will likely require dedicated bioenergy crops, hence the importance of land productivity in regions likely to contribute to bioenergy production. In addition to biomass production, BECCS also requires water at the CO2 capture level, which is mainly a function of the cooling technology. However, BECCS total water requirement is largely driver by the biomass water footprint (Fajardy and Mac Dowell, 2017). Water requirements for DACCS and EW are typically lower than that of BECCS or biochar by an order of magnitude (Smith et al., 2016a).
Assumptions underlying IAMs typically feature a land productivity increase of a 1 to 3% over time (Fisher et al., 2012; Winchester and Reilly, 2015). Since these assumptions are based on historical trends of conventional (e.g., wheat/corn) crops, the extent of which these increases will be applicable to second generation bioenergy crops, is uncertain. Regional quantifications of actual and potential yields for bioenergy crops is therefore crucial, especially considering that some high yielding and resilient types (e.g., perennial grasses) could remediate set-aside/low productivity land, thus reducing the overall land requirement for BECCS (Smith et al., 2013; Liu et al., 2014). Quantifying the regional availability of such land parcels, and determining to which extent they will be accessible and used by energy producers will enable further evaluations on sustainable bioenergy potential.
Other key drivers for BECCS deployment include land accessibility, for biomass transport and CO2 infrastructure roll-out, and water requirement at the plant gate (Smith et al., 2016a; Fajardy and Mac Dowell, 2017), which needs to be factored to a smaller extent when deciding on the location of a BECCS plant.
Biochar and minerals for EW can be applied on agricultural lands, in conjunction with conventional farming, which means than there is no direct competition with other uses of the land. Furthermore, potential yield increase in the land from biochar and minerals application could be an added benefit of CDR deployment (Lenton, 2010; Jeffery et al., 2011).
Low Carbon Energy and Energy Systems Integration
A key enabling factor for CDR technologies deployment is the possibility to access low carbon energy sources: whilst BECCS produces energy, its life cycle energy requirement can be high and regions with low carbon fuels and electricity will be favored to produce sustainable biomass (Fajardy et al., 2018). EW, biochar and afforestation also require some form of potentially energy-intensive steps—the total energy requirement typically decreasing in this order—such as material grinding and transport for EW (Renforth, 2012), biochar production via pyrolisis, transport and application for biochar (Smith, 2016), and forest management (e.g., fertilizer application) for afforestation. The availability of low carbon energy becomes even more important when considering DACCS, which process requires ~9–10 GJ of thermal energy per ton of CO2. Such energy requirement has three main implications: (1) high running costs of DACCS facilities compared to other CDRs options, (2) carbon efficiency requirement (de Jonge et al., 2019), and (3) implications on the energy system configuration (Daggash et al., 2019).
CDRs options implying energy production (BECCS) or consumption (DACCS), face the challenge of being efficiently integrated into the energy systems, especially considering their anticipated role in climate stabilization scenarios. A recent study calculated that, if emission reductions in line with the 2°C target are to be met by 2100, high rates of end-use energy produced by BECCS and consumed by DACCS (8 and 20%, respectively) would be needed (Creutzig et al., 2019). Given such a prominent role in both energy generation and load, the deployment of these options needs to account for future energy systems configurations, especially considering that the rate of energy systems decarbonization has important consequences for the scale of CDR adoption.
However, studies attempting to understand how CDR technologies will integrate into future energy systems are currently scarce in the literature. Especially crucial are energy systems modeling efforts with high spatiotemporal and technological resolution, to understand: (1) the role of CDR deployment in regional energy demand (DACCS) and energy supply (BECCS) curves, and (2) potential feedback loops between energy market prices and cost/carbon efficiencies of CDRs options.
CO2 Storage
Accessible CO2 storage capacity is ultimately the main regional driver of BECCS and DACCS deployment. Few regions (e.g., the USA, UK and Norway) have performed a thorough geological survey to assess its CO2 storage capacity and quality. When surveys have been carried out, the data is not always publicly available and/or exploitable (e.g., China and Europe) (Dahowski et al., 2009; Geological Survey of Denmark Greenland, 2009; Li et al., 2009). Other regional studies have been performed, but they often consist in qualitative assessments at the country level (e.g., India, Brazil) (Holloway et al., 2008; Ketzer et al., 2014) or quantitative assessment at the basin level (e.g., China). Furthermore, differences in assumptions from one survey to another render them difficult to compare. An open-access and consistent database gathering regional geological storage quality and capacity is mandatory to further explore which role will a region play—if any—in deploying BECCS and DACCS. Table 1 summarizes the relative importance of regional bottlenecks for each CDR method.
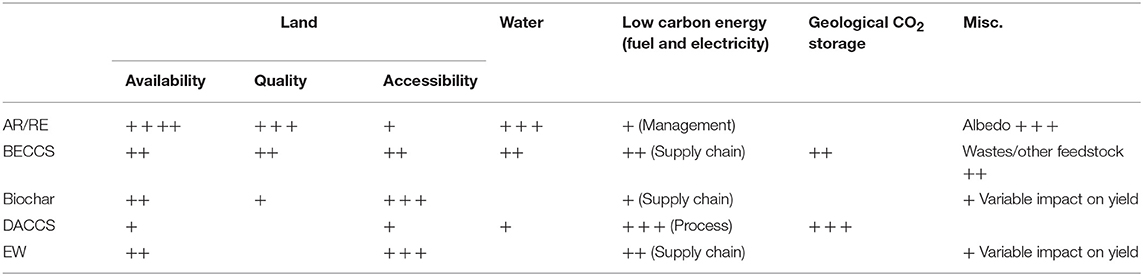
Table 1. The comparative importance of regional bottlenecks to the deployment of different CDR technologies.
Tracking CO2 Removal in Space and Time
Common to all CDR technologies is the requirement to track the CO2 along the value chain in both space and time. The CO2 negativity—how much CO2 is actually removed when accounting for life cycle emissions, or the CO2 efficiency—ratio of CO2 removed to CO2 absorbed by the biomass (Renforth, 2012; Fajardy and Mac Dowell, 2017; de Jonge et al., 2019; Tanzer and Ramirez, 2019) including land use change emissions in particular (Searchinger et al., 2008; Harper et al., 2018)—are key metrics that need to be carefully assessed. This is particularly important when comparing different CDR technologies, as illustrated in a recent work comparing BECCS and AR at the global level (Harper et al., 2018), highlighting the relative success of BECCS when accounting for changes on the land total carbon budget. Implementing sustainability criteria and supply chain certification frameworks are central to this endeavor.
Forest certification, a practice that recognizes responsible management via verified compliance with underlying criteria and indicators (CIFOR, 2013), represent a useful tool in biological carbon offsets projects. However, the lack of policy related to certification guidelines and the absence of a universal accreditation framework has resulted in large discrepancies between the claims of different projects (CIFOR, 2013). This, in turn, leads to uncertainty, which might discredit AR as a valid CDR practice and contributes to the growing concern about carbon neutrality of forestry-based biomass sources used in BECCS projects (Searchinger, 2012).
Tracking CO2 emissions becomes particularly challenging when CDR value chains are potentially international and multipolar, which is specific to BECCS (Fajardy et al., 2018).
Time matters differently for each CDR technology, when it comes to tracking CO2 removal, hence the need for different metrics to measure their performances. The rate of CO2 removal represents a crucial factor for biological or mineral sinks, i.e., in BECCS, AR, EW, and biochar, and remain a point of uncertainty for EW in particular (Renforth, 2012).
Specific to land-based CDR potentially involving land use change, i.e., BECCS in this case, high initial land use change emissions—also referred to as the carbon debt (Fargione et al., 2008)—as compared to the technology CO2 removal rate, can lead to delayed carbon removal: a CDR system only becomes net negative after several years of operation, if at all (Fajardy and Mac Dowell, 2017).
Natural sinks reach their maximum capacity within decades, as compared to centuries for geological underground storage. Therefore, when CO2 is stored in biological sinks (trees, land, oceans), the issue of sink saturation, i.e., the fact that CO2 removal is limited in time, is a major limitation. In case of AR, this relates to the fact that trees reach maturity, and therefore the net removal of greenhouse gases from the atmosphere decline to zero (Smith et al., 2016b; Alcalde et al., 2018). Its direct implication is that forest sinks need to be managed and monitored to maintain the optimal CO2 uptake over time. Finally, monitoring CO2 sequestered is also key to ensure permanence CO2 removal. Whilst long term geological storage raises questions of liability and insurance (Bui et al., 2018), monitoring CO2 storage sites represent more of a legal and financial challenge than a technical one. In the case of afforestation however, natural (for e.g., decay) and accidental (for e.g., forest fires, land use change) degradation of the carbon stock, both above ground and below ground, put the permanence of CO2 removal at risk.
The relative difficulty for each CDR option of tracking CO2 removal in space and time, could ultimately impact: 1) the complexity of the regulation/certification frameworks required to ensure the performance and sustainability of each option and 2) the economic potential of the technology—by deterring investors with high upfront financial risks and high regulation, and therefore need to be included in comparative assessments. These observations are summarized in Table 2.
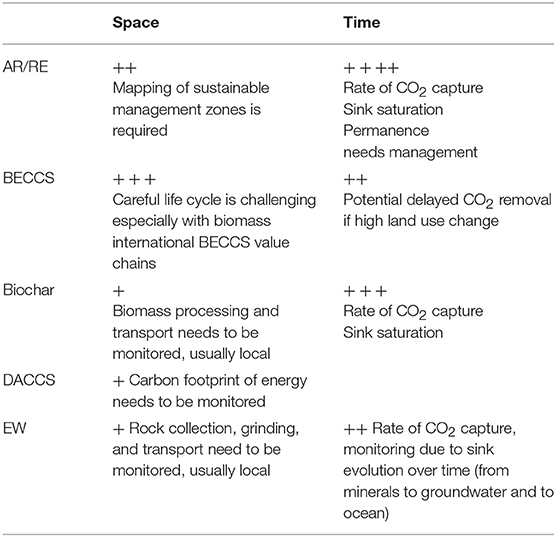
Table 2. The comparative importance of life cycle analysis to the performance of different CDR technologies in space and time.
Socio-economic Issues and the Need for a Landscape Approach
Much of the controversy about CDR methods to date is directed at BECCS, emerging as the key technology for cost-effectively limiting global warming to the level set by the Paris Agreement. Studies applying global IAMs indicates a global potential deployment of biomass for BECCS in the range of 1.3–1.6 GtCO2/yr by 2050 (Huppmann et al., 2018; Rogelj et al., 2018). It is well-recognized that such a high deployment of biomass would require a massive transformation of agricultural systems worldwide, with implications for both environmental and social change. Outcomes from IAM studies, have extensively investigated the widespread ecological impacts, such as biodiversity loss, food security along whit access to energy and water (Müller et al., 2008; Popp et al., 2011, 2014) that would occur if BECCS is extrapolated to the required scale. However, case study evidence detailing the societal impacts of bioenergy and BECCS deployment is currently missing in climate actions debates. When the socio-economic dimension is accounted for, this is generally done by considering economic growth or food prices. Other dimensions of human well-beings, such as employment opportunities or change in socio-economic conditions of local communities, remain largely overlooked.
In the attempt to narrow this gap, Creutzig et al. (2013) have analyzed the factors shaping the interaction between bioenergy and livelihood, highlighting how global competition for land use emerging from IAMs scenarios, would directly affect 1.5 billion smallholders. The authors concluded that, because calculation of bioenergy potentials is often presented at aggregated level, impacts of bioenergy schemes on local communities remain systematically underexplored.
The argument of global scale as an inadequate dimension to see potential social impacts at work, has been recently brought up by other authors (Buck, 2018; Lenzi, 2018). While conducting structured interviews and site visits to examine the challenges of the deployment of CDR technologies (Buck, 2018) have identified, in the landscape level, larger than a farm but smaller than a region, the crucial dimension in designing the governance of CDR technologies. A landscape approach, the author argues, would promote a paradigm shift in the way CDR is perceived, as a valuable practice that creates jobs, rather than artifacts being deployed. Hence, adopting a landscape/local prospective, would not only address distributional issues associated with the production model underlying CDR schemes, but also help to resolve some narrative issues around CDR technologies.
The value of the societal dimension is also crucial to quantify potential local co-benefits, such as employment opportunities (Patrizio et al., 2018) and income growth. This is particularly relevant for BECCS and afforestation projects in developing countries, where large areas of land are still unregistered, and surrounding communities must obtain benefits from forest and be actively involved in their management (Greve et al., 2013). Accounting for such elements may influence geographic priorities and serve as a useful initial decision-making tool to maximize the overall benefits of CDR projects.
Financing CDR
The literature on CDR has focused on techno-economic assessments of CDR methods at scale (Fuss et al., 2018). The economic costs of deploying these technologies have been evaluated, albeit with significant uncertainty owing to the lack of demonstration and commercial-scale projects. It has been repeatedly highlighted that regional carbon prices are insufficient to incentivize CDR deployment. More relevant to note is that, should carbon prices be sufficient, mainstream carbon pricing schemes often only penalize CO2 emissions and do not remunerate removal. CDR is a public good as it furthers climate change mitigation, so its value needs to be recognized and appropriately remunerated. Failing regulatory/policy changes to carbon pricing mechanisms to incentivize CDR and an increase in carbon prices, a negative emissions credit—a payment for net CO2 removal from the atmosphere—is necessary. Financing mechanisms that can deliver these incentives in a socially-acceptable manner (i.e., without disproportionately affecting some segments of society e.g., fuel tax hikes) have not received enough attention. It has been shown that CDR deployment can allow for the continued utilization of existing CO2-emitting assets, thereby avoiding stranded assets (Daggash et al., 2019). The revenue generated by some of these assets which would have been otherwise constrained from productive utilization should accrue to the providers of CDR. The market and governance mechanisms that allow for the verification and trading of such negative emissions credits (as discussed earlier), however, are currently non-existent and need to be designed.
Enhanced oil recovery and merchant CO2 markets have been proposed as routes to CDR commercialization. However, CO2 utilization processes generally do not contribute to climate change mitigation as they only delay emissions1. Commercial-scale CDR which also contributes to mitigation is only possible if a stable revenue stream is available for the service of permanently sequestering atmospheric carbon. Additionally, the volumes of these CO2 markets are small relative to the scale of CDR needed to meet the Paris Agreement.
Majority of the CDR options interact, to different extents, with the energy system. Since the 1990s, energy markets have been increasingly liberalized, such that investment in energy technologies is largely private sector-driven. Private investment seeks the greatest economic return, therefore incentives (both policy and financial) offered to CDR need to make the technologies not just economically-viable but the most attractive destination for investment.
International Diplomacy
The ability to deliver CDR at scale is contingent on the availability of several bio-geophysical resources—land, CO2 storage capacity, rocks, ocean, etc.—, all of which are scarce and unevenly distributed geographically. In addition to quantity, the nature and quality of each resource will determine the carbon removal potential of the CDR technique pursued. Economic factors, such as labor costs and taxation rates will influence local CDR costs. Owing to geographic and economic factors therefore, the physical and economic efficiency of CDR technologies for climate change mitigation will be location-dependent. The Paris Agreement—and climate policy, in general—has sought to distribute the burden of climate change mitigation based on “the principle of equity and common but differentiated responsibilities and respective capabilities, in the light of different national circumstances.” To achieve both a cost- and politically-optimal distribution of CDR burden, therefore, will require mechanisms that allow for cross-border trade/exchange of resources (e.g., of sustainable biomass, or energy) and emissions (should it prove cheaper for a country to pay for CDR to be done elsewhere). This, in addition to the dominant market-based approach to development of energy infrastructure, means multi-region, multi-stakeholder supply chains and market/governance systems will manifest.
Whilst these socioeconomic and political challenges surrounding CDR have been acknowledged (Honegger and Reiner, 2018; Nemet et al., 2018), there has not been an attempt to quantify what, firstly, a cost optimal distribution of CDR burden globally is. If that involves cross-border biomass trade (in the case of BECCS deployment) as has been suggested (Fajardy et al., 2018; Daggash et al., 2019), then the development of international biomass sustainability certification standards is critical. The development of such frameworks, particularly in countries without pre-existing expertise of sustainability certification, will require cooperation between expected trading partners so as to align verification and monitoring methods. Although several regional emissions trading schemes (ETS) and carbon offsetting mechanisms exist globally, they have failed to incentivize real and verifiable storage of CO2–and consequently, CDR—by encouraging short-term CO2 utilization instead (Haszeldine et al., 2018). Adapting carbon offsetting mechanisms to incentivize CDR and include negative emissions in trading permits, and further expansion of emissions trading zones will facilitate the deployment of CDR at scale. Through the nationally-determined contributions (NDCs), commitments to the Paris Agreement are assessed at country-level. If cross-border financing and bio-geophysical resources are used to deliver CDR, a framework needs to be developed to determine to whom the emissions reduction is ascribed. The aforementioned present significant regulatory and political barriers, both locally and internationally, to efficient CDR deployment. However, they have received insufficient investigation, indicated by their absence in the literature. Failure to fully understand these challenges within different socioeconomic and geopolitical contexts, and design appropriate policy solutions to address them will stifle necessary climate change mitigation action.
Knowledge Transfer
IAMs estimate that the largest cumulative contributions of CDR are made by six regions: China, the USA, India, the EU-28, Brazil and Russia (Peters and Geden, 2017). Discussions surrounding CDR, and more broadly, CCS, deployment have centered on these regions, principally the USA and Northwestern Europe. Accordingly, there has been an abundance of research quantifying the bio-geophysical limitations to CDR deployment, often by publicly-funded bodies, such as the countries' geological surveys or energy/environment ministries. High-quality data enables accurate quantification of CDR potential within these regions—crucial in understanding how the rest of the energy system and society is required to transition to meet the Paris Agreement.
With the exception of India, the centers of population and economic growth in the coming decades are expected outside the aforementioned regions—mostly in Sub-Saharan Africa and South East Asia. Increasing population leads to increased energy demand, and economic development (hence more affluent lifestyles) will lead to higher per capita demand. The rate and scale of energy supply expansion needed to accommodate these demographic explosions means majority of developing countries continue to pursue largely fossil fuel-powered development. Prolonged use of fossil fuels may lead to an increasing reliance on CDR (and CCS) to meet emissions reductions commitments. However, typically, the expertise needed to gather knowledge on CDR/CCS potential is lacking and budgetary constraints limit resource availability to undertake RD&D within those environments. Climate equity—as interpreted in the Paris Agreement—warrants that developed countries provide finance, technology transfer and capacity-building to “assist developing country Parties with respect to both mitigation and adaptation in continuation of their existing obligations under the Convention.” This is ongoing via special funds under the Global Environment Facility but financing has thus far eluded CDR/CCS projects and focused on alternative mitigation/adaptation solutions. Focus and financing must be re-channeled if the necessary expertise is to be developed, so that it is available when CDR/CCS deployment become necessary. How much and to whom this financing should be given—that is the countries for which CCS/CDR is likely to be a necessity—need to be identified through further research.
Author Contributions
MF, PP, and HD wrote the first draft of the manuscript. All authors contributed to the conception and design of the study. All authors contributed to manuscript revision, read, and approved the submitted version.
Funding
The authors thank the Science and Solutions for a Changing Planet Doctoral Training Programme (SSCP DTP) sponsored by the Natural Environment Research Council (NERC), the Comparative assessment and region-specific optimization of GGR project under grant NE/P019900/1 from the NERC for the funding of a Ph.D. scholarship, and Imperial College for the funding of a President's Ph.D. scholarship in support of this project.
Conflict of Interest Statement
The authors declare that the research was conducted in the absence of any commercial or financial relationships that could be construed as a potential conflict of interest.
Footnotes
1. ^Conversion of CO2 into building materials (e.g., concrete) is one of the few CCUS processes that result in the permanence of stored CO2.
References
Alcalde, J., Smith, P., Stuart Haszeldine, R., and Bond, C. E. (2018). The potential for implementation of negative emission technologies in Scotland. Int. J. Greenhouse Gas Control 76, 85–91. doi: 10.1016/j.ijggc.2018.06.021
Azar, C., Lindgren, K., Obersteiner, M., Riahi, K., van Vuuren, D. P., den Elzen, K. M. G., et al. (2010). The feasibility of low CO2 concentration targets and the role of bio-energy with carbon capture and storage (BECCS). Clim. Change 100, 195–202. doi: 10.1007/s10584-010-9832-7
Beal, C. M., Archibald, I., Huntley, M. E., Greene, C. H., and Johnson, Z. I. (2018). Integrating algae with bioenergy carbon capture and storage (ABECCS) increases sustainability. Earths Future 6, 524–542. doi: 10.1002/2017EF000704
Buck, H. J. (2018). The politics of negative emissions technologies and decarbonization in rural communities. Glob. Sustain. 1:e2. doi: 10.1017/sus.2018.2
Bui, M., Adjiman, C. S., Bardow, A., Anthony, E. J., Boston, A., Brown, S., et al. (2018). Carbon capture and storage (CCS): the way forward. Energy Environ. Sci. 11, 1062–1176. doi: 10.1039/C7EE02342A
Calvin, K., Wise, M., Kyle, P., Patel, P., Clarke, L., and Edmonds, J. (2014). Trade-offs of different land and bioenergy policies on the path to achieving climate targets. Clim. Change 123, 691–704. doi: 10.1007/s10584-013-0897-y
CIFOR (2013). Evaluasi Dampak Sertifikasi Forest Stewardship Council (FSC) Terhadap Pengelolaan Hutan Alam Di Daerah Tropis. Int. For. Rev. 19. doi: 10.17528/cifor/004347
Creutzig, F., Breyer, C., Hilaire, J., Minx, J., Peters, G. P., and Socolow, R. (2019). The mutual dependence of negative emission technologies and energy systems. Energy Environ. Sci. 12, 1805–1817. doi: 10.1039/C8EE03682A
Creutzig, F., Corbera, E., Bolwig, S., and Hunsberger, C. (2013). Integrating place-specific livelihood and equity outcomes into global assessments of bioenergy deployment. Environ. Res. Lett. 8:035047. doi: 10.1088/1748-9326/8/3/035047
Creutzig, F., Ravindranath, N. H., Berndes, G., Bolwig, S., Bright, R., Cherubini, F., et al. (2015). Bioenergy and climate change mitigation: an assessment. GCB Bioenergy 7, 916–944. doi: 10.1111/gcbb.12205
Daggash, H. A., Heuberger, C. F., and Mac Dowell, N. (2019). The role and value of negative emissions technologies in decarbonising the UK energy system. Int. J. Greenhouse Gas Control 81, 181–198. doi: 10.1016/j.ijggc.2018.12.019
Dahowski, R. T., Li, X., Davidson, C. L., Wei, N., and Dooley, J. J. (2009). Regional Opportunities for Carbon Dioxide Capture and Storage in China. Available online at: https://energyenvironment.pnnl.gov/pdf/roccs_china_pnnl_19091.pdf
de Jonge, M. M. J., Daemen, J., Loriaux, J. M., Steinmann, Z. J. N, Huijbregts, M. A., et al. (2019). Life cycle carbon efficiency of direct air capture systems with strong hydroxide sorbents. Int. J. Greenhouse Gas Control 80, 25–31. doi: 10.1016/j.ijggc.2018.11.011
Department for Business Energy Industrial Strategy (2018). Contracts for Difference Scheme for Renewable Electricity Generation. London. Available online at: https://assets.publishing.service.gov.uk/government/uploads/system/uploads/attachment_data/file/714067/contracts-for-difference-renewable-energy-consultation-response-part-a.pdf
DRAX GROUP plc (2015). Delivering Europe's Largest Decarbonisation Project, On Time and On Budget, Whilst Producing 8% of the UK's Electricity. Available online at: https://www.drax.com/wp-content/uploads/2016/09/2015-Half-Year-Results-for-the-six-months-ended-30-June-20151.pdf
Fajardy, M., Chiquier, S., and Mac Dowell, N. (2018). Investigating the BECCS Resource Nexus: delivering sustainable negative emissions. Energy Environ. Sci. 11, 3408–3430. doi: 10.1039/C8EE01676C
Fajardy, M., and Mac Dowell, N. (2017). Can BECCS deliver sustainable and resource efficient negative emissions?” Energy Environ Sci. 10, 1389–1426. doi: 10.1039/C7EE00465F
Fargione, J., Hill, J., Tilman, D., Polasky, S., and Hawthorne, P. (2008). Land clearing and the biofuel carbon debt. Science 319, 1235–1237. doi: 10.1126/science.1152747
Fisher, E. M., Dupont, C., Darvell, L. I., Commandré, J. M., Saddawi, A., Jones, J. M., et al. (2012). Combustion and gasification characteristics of chars from raw and torrefied biomass. Bioresour. Technol. 119, 157–165. doi: 10.1016/j.biortech.2012.05.109
Fuss, S., Lamb, W. F., Callaghan, M. W., Hilaire, J., Creutzig, F., Amann, T., et al. (2018). Negative emissions—part 2: costs, potentials and side effects. Environ. Res. Lett. 13:063002. doi: 10.1088/1748-9326/aabf9f
Geological Survey of Denmark Greenland (2009). Assessing European Capacity for Geological Storage of Carbon Dioxide–WP2 Report Storage Capacity. EU GeoCapacity project. Available online at: http://www.geology.cz/geocapacity/publications/D16%20WP2%20Report%20storage%20capacity-red.pdf
Greve, M., Reyers, B., Lykke, A. M., and Svenning, J. C. (2013). Spatial optimization of carbon-stocking projects across Africa integrating stocking potential with co-benefits and feasibility. Nat. Commun. 4:2975. doi: 10.1038/ncomms3975
Harper, A. B., Powell, T., Cox, P. M., House, J., Huntingford, C., Lenton, T. M., et al. (2018). Land-use emissions play a critical role in land-based mitigation for paris climate targets. Nat. Commun. 9:2938. doi: 10.1038/s41467-018-05340-z
Haszeldine, R. S., Flude, S., Johnson, G., and Scott, V. (2018). Negative emissions technologies and carbon capture and storage to achieve the Paris Agreement Commitments. Philos. Trans. R. Soc. A Math. Phys. Eng. Sci. 376:20160447. doi: 10.1098/rsta.2016.0447
Holloway, S., Garg, A., Kapshe, M., Deshpande, A., Pracha, A. S., and Khan, S. R. (2008). A Regional Assessment of the Potential for CO2 Storage in the Indian Subcontinent. The British Geological Survey. Available online at: https://ukccsrc.ac.uk/system/files/publications/ccs-reports/DECC_CCS_47.pdf
Honegger, M., and Reiner, D. (2018). The political economy of negative emissions technologies: consequences for international policy design. Clim. Policy 18, 306–321. doi: 10.1080/14693062.2017.1413322
Humpenöder, F., Popp, A., Philip Dietrich, J., Klein, D., Lotze-Campen, H., Bonsch, M., et al. (2014). Investigating afforestation and bioenergy CCS as climate change mitigation strategies. Environ. Res. Lett. 9:064029. doi: 10.1088/1748-9326/9/6/064029
Huppmann, D., Rogelj, J., Krey, V., Kriegler, E., and Riahi, K. (2018). A new scenario resource for integrated 1.5°C research. Nat. Clim. Change 8, 1027–1030. doi: 10.1038/s41558-018-0317-4
IPCC (2018). “Summary for policymakers,” in Global Warming of 1.5°C. An IPCC Special Report on the Impacts of Global Warming of 1.5°C above Pre-Industrial Levels and Related Global Greenhouse Gas Emission Pathways, in the Context of Strengthening the Global Response to the Threat of Climate Change, eds V. Masson-Delmotte, P. Zhai, H.-O. Pörtner, D. Roberts, J. Skea, P. R. Shukla, A. Pirani, et al. (Geneva: World Meteorological Organization), 32 pp.
Jeffery, S., Verheijen, F. G., van der Velde, A M, and Bastos, A. C. (2011). A quantitative review of the effects of biochar application to soils on crop productivity using meta-analysis. Agric. Ecosyst. Environ. 144, 175–187. doi: 10.1016/j.agee.2011.08.015
Ketzer, J. M. M., Machado, C. X., Rockett, G. C., and Iglesias, R. S. (2014). Brazilian Atlas of CO2 Capture and Geological Storage. Global CCS Institute. Available online at: https://www.globalccsinstitute.com/archive/hub/publications/202033/atlas-brasileiro.pdf
Klein, D., Luderer, G., Kriegler, E., Strefler, J., Bauer, N., Leimbach, M., et al. (2014). The value of bioenergy in low stabilization scenarios: an assessment using REMIND-MAgPIE. Clim. Change 123, 705–718. doi: 10.1007/s10584-013-0940-z
Kreidenweis, U., Humpenöder, F., Stevanović, M., Bodirsky, B. L., Kriegler, E., Lotze-Campen, H., et al. (2016). Afforestation to mitigate climate change: impacts on food prices under consideration of Albedo effects. Environ. Res. Lett. 11:085001. doi: 10.1088/1748-9326/11/8/085001
Kwak, J., Islam, M. S., Wang, S., Messele, S. A., Naeth, M. A., El-Din, M. G., et al. (2019). Biochar properties and lead(II) adsorption capacity depend on feedstock type, pyrolysis temperature, and steam activation. Chemosphere 231, 393–404. doi: 10.1016/j.chemosphere.2019.05.128
Lenton, T. M. (2010). The potential for land-based biological CO2 removal to lower future atmospheric CO2 concentration. Carbon Manage. 1, 145–160. doi: 10.4155/cmt.10.12
Li, X., Wei, N., Liu, Y., Fang, Z., Dahowski, R. T., and Davidson, C. L. (2009). CO2 point emission and geological storage capacity in China. Energy Proc. 1, 2793–2800. doi: 10.1016/j.egypro.2009.02.051
Liu, W., Mi, J., Song, Z., Yan, J., Li, J., and Sang, T. (2014). Long-term water balance and sustainable production of miscanthus energy crops in the Loess Plateau of China. Biomass Bioenergy 62, 47–57. doi: 10.1016/j.biombioe.2014.01.018
Minx, J. C., Lamb, W. F., Callaghan, M. W., Fuss, S., Hilaire, J., Creutzig, F., et al. (2018). Negative emissions—part 1: research landscape and synthesis. Environ. Res. Lett. 13:063001. doi: 10.1088/1748-9326/aabf9b
Müller, A., Schmidhuber, J., Hoogeveen, J., and Steduto, P. (2008). Some insights in the effect of growing bio-energy demand on global food security and natural resources. Water Policy 10, 83–94. doi: 10.2166/wp.2008.053
Nemet, G. F., Callaghan, M. W., Creutzig, F., Fuss, S., Hartmann, J., Hilaire, J., et al. (2018). Negative emissions—part 3: innovation and upscaling. Environ. Res. Lett. 13:063003. doi: 10.1088/1748-9326/aabff4
Onarheim, K., Santos, S., Kangas, P., and Hankalin, V. (2017). Performance and costs of CCS in the pulp and paper industry part 1: performance of amine-based post-combustion CO2 capture. Int. J. Greenhouse Gas Control 59, 58–73. doi: 10.1016/j.ijggc.2017.02.008
Patrizio, P., Leduc, S., Kraxner, F., Fuss, S., Kindermann, G., Mesfun, S., et al. (2018). Reducing US coal emissions can boost employment. Joule 2, 2633–2648. doi: 10.1016/j.joule.2018.10.004
Peters, G. P., and Geden, O. (2017). Catalysing a political shift from low to negative carbon. Nat. Clim. Change 7, 619–621. doi: 10.1038/nclimate3369
Popp, A., Dietrich, J. P., Lotze-Campen, H., Klein, D., Bauer, N., Krause, M., et al. (2011). The economic potential of bioenergy for climate change mitigation with special attention given to implications for the land system. Environ. Res. Lett. 6. doi: 10.1088/1748-9326/6/3/034017
Popp, J., Lakner, Z., Harangi-Rákos, M., and Fári, M. (2014). The effect of bioenergy expansion: food, energy, and environment. Renew. Sustain. Energy Rev. 32, 559–578. doi: 10.1016/j.rser.2014.01.056
Pour, N., Webley, P. A., and Cook, P. J. (2018). Potential for using municipal solid waste as a resource for bioenergy with carbon capture and storage (BECCS). Int. J. Greenhouse Gas Control 68, 1–15. doi: 10.1016/j.ijggc.2017.11.007
Renforth, P. (2012). The potential of enhanced weathering in the UK. Int. J. Greenhouse Gas Control 10, 229–243. doi: 10.1016/j.ijggc.2012.06.011
Rogelj, J., Popp, A., Calvin, K. V., Luderer, G., Emmerling, J., Gernaat, D., et al. (2018). Scenarios towards limiting global mean temperature increase below 1.5°C. Nat. Clim. Change 8, 325–332. doi: 10.1038/s41558-018-0091-3
Searchinger, T. (2012). Sound Principles and an Important Inconsistency in the 2012 UK Bioenergy Strategy. Available online at: http://ww2.rspb.org.uk/Images/Searchinger_comments_on_bioenergy_strategy_SEPT_2012_tcm9-329780.pdf
Searchinger, T., Heimlich, R., Houghton, R. A., Dong, F., Elobeid, A., Fabiosa, J., et al. (2008). Use of U.S. croplands for biofuels increases greenhouse gases through emissions from land-use change. Science 319, 1238–1240. doi: 10.1126/science.1151861
Smith, P. (2016). Soil carbon sequestration and biochar as negative emission technologies. Glob. Change Biol. 22, 1315–1324. doi: 10.1111/gcb.13178
Smith, P., Davis, S. J., Creutzig, F., Fuss, S., Minx, J., Gabrielle, B., et al. (2016a). Biophysical and economic limits to negative CO2 emissions. Nat. Clim. Change 6, 42–50. doi: 10.1038/nclimate2870
Smith, P., Haszeldine, R. S., and Smith, S. M. (2016b). Preliminary assessment of the potential for, and limitations to, terrestrial negative emission technologies in the UK. Environ. Sci. Process. Impacts 18, 1400–1405. doi: 10.1039/C6EM00386A
Smith, S. L., Thelen, K. D., and MacDonald, S. J. (2013). Yield and quality analyses of bioenergy crops grown on a regulatory brownfield. Biomass Bioenergy 49, 123–130. doi: 10.1016/j.biombioe.2012.12.017
Stoy, P. C., Ahmed, S., Jarchow, M., Rashford, B., Swanson, D., Albeke, S., et al. (2018). Opportunities and trade-offs among BECCS and the food, water, energy, biodiversity, and social systems nexus at regional scales. Bioscience 68, 100–111. doi: 10.1093/biosci/bix145
Tanzer, S. E., and Ramirez, A. (2019). When are negative emissions negative emissions? Energy Environ. Sci. 12, 1210–1218. doi: 10.1039/C8EE03338B
Keywords: negative emissions, carbon dioxide removal, climate change mitigation, BECCS, DACCS
Citation: Fajardy M, Patrizio P, Daggash HA and Mac Dowell N (2019) Negative Emissions: Priorities for Research and Policy Design. Front. Clim. 1:6. doi: 10.3389/fclim.2019.00006
Received: 28 April 2019; Accepted: 04 September 2019;
Published: 01 October 2019.
Edited by:
Jennifer Wilcox, Worcester Polytechnic Institute, United StatesReviewed by:
Rafael Mattos Dos Santos, University of Guelph, CanadaStefano Stendardo, Italian National Agency for New Technologies, Energy and Sustainable Economic Development (ENEA), Italy
Copyright © 2019 Fajardy, Patrizio, Daggash and Mac Dowell. This is an open-access article distributed under the terms of the Creative Commons Attribution License (CC BY). The use, distribution or reproduction in other forums is permitted, provided the original author(s) and the copyright owner(s) are credited and that the original publication in this journal is cited, in accordance with accepted academic practice. No use, distribution or reproduction is permitted which does not comply with these terms.
*Correspondence: Niall Mac Dowell, bmlhbGxAaW1wZXJpYWwuYWMudWs=