- 1Climeworks AG, Zurich, Switzerland
- 2Risk Dialogue Foundation, St. Gallen, Switzerland
In recent years Direct Air Capture (DAC) has established itself as a promising approach to atmospheric Carbon Dioxide Removal (CDR) also referred to as Negative Emissions. However, due to the amounts likely needed to be removed CDR technologies like DAC will only become climate relevant if they rapidly reach gigaton scale, around the middle of this century. Here we give a brief insight into DAC and in particular, the modular low temperature DAC technology developed by Climeworks of Switzerland. We discuss potential co benefits, in particular in relation to the Sustainable Development Goals (SDGs) of the United Nations and conclude by suggesting some policy approaches on how a climate relevant scale could be achieved in time.
Introduction
In the 2015 landmark Paris Agreement, the international community committed to limit global warming by the year 2100 to “well below 2°C” and “to pursue efforts to keep warming below 1.5°C” compared to pre-industrial levels (United Nations, 2015).
To achieve that, first of all a worldwide rapid decline toward zero emissions is urgently needed. However, currently annual greenhouse emissions continue to rise (Quere et al., 2018), rendering these goals increasingly hard to achieve in time and near impossible with emissions reductions alone (Strefler et al., 2018). Therefore, atmospheric Carbon Dioxide Removal (CDR) or negative emissions technologies have increasingly been included in the vast majority of mitigation pathways and are now considered necessary to keep below 2°C and even more so for 1.5°C.
More concretely, all IPCC emissions pathways that are compatible with 1.5°C without overshoot1 and 87% of pathways compatible with 2°C, rely on the assumption of large-scale atmospheric CDR, reaching zero emissions at some point in the 21st century followed by a period of net-negative emissions—meaning that CDR rates exceed residual emissions (IPCC, 2014, 2018; European Academies Science Advisory Council, 2018). In most scenarios the rate of CDR reaches several billions of tons (1Gt = 1 billion tons) per year over several decades as shown in Figure 1. Such large scale remains indicative, as global mitigation efforts and their success varies depending on shared socio-economic pathways, e.g., outlined by Rogelj et al. (2018) and technological feasibility of country-level net zero emission targets, e.g., outlined by Kaya et al. (2019). In all cases, CDR within a gigaton order of magnitude remain relevant and show a substantial impact on achieving climate targets, e.g., Realmonte et al. (2019). It is however most important to highlight that CDR is complementing rather than replacing mitigation.
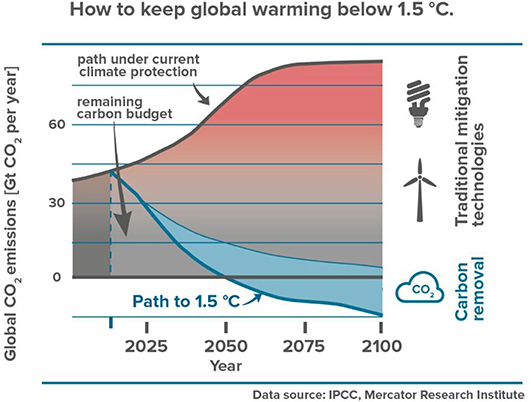
Figure 1. Schematic illustration of NET deployment timing and scale2.
Prominent approaches to CDR discussed in the scientific literature include afforestation and change of land use, enhanced weathering, bioenergy and carbon capture and storage or BECCS, as well as direct air capture and storage of CO2 or DACS (Smith et al., 2015).
DAC refers to a range of technological solutions that are able to extract CO2 from ambient air at any location on the planet This is possible because in ambient air, CO2 is nearly evenly distributed around the globe at average concentrations of, at present 405.5 parts per million and rising3 (World Meteorological Organization, 2019).
Currently there are a handful of companies active in the DAC field, all currently designing and utilizing different DAC technologies, to focus on different markets.
In Canada, Carbon Engineering (CE) uses liquid alkali metal oxide sorbents regenerated by heat at around 800°C. CE currently uses natural gas to power its machines, co-capturing CO2 from the flue gas stream of the burnt natural gas in addition to atmospheric capture (Keith et al., 2018).
A further key DAC player is Global Thermostat (GT) in the US. The GT process uses a solid amine-based sorbent material, regenerated at around 80–100°C (Ping et al., 2018).
Switzerland-based Climeworks AG uses a DAC design based on an adsorption/desorption process on alkaline-functionalized adsorbents, which is described in more detail in the following. CO2 adsorption is performed at ambient conditions and CO2 desorption is performed through a temperature-vacuum-swing (TVS) process (Wurzbacher et al., 2016). During this process, the pressure in the system is reduced and the temperature is increased to 80 to 120°C, thereby releasing the CO2. After a cooling phase, the whole process begins anew, as illustrated in Figure 2 below. The process results in the production of gaseous CO2 at 1 bar with a purity level of >99.8%. Depending on the ambient conditions, in particular relative humidity, the current Climeworks process can also extract H2O from the air as a byproduct4.
Climeworks adopted a modular design to reduce manufacturing and operating costs, support scalability and diversity in deployment, ease of transportability and enable automated mass manufacturing. CO2 adsorption and desorption is performed within the same devices, referred to as “CO2 Collectors” or “Collectors.” Collectors are engineered to fit efficiently into a steel frame, with 6 Collectors fitting into a standard 40-foot shipping container. The present nominal annual CO2 Collector capacity is 50 tons of CO2. This will increase as the technology is optimized. The modular Collectors are designed to operate together as a unit and can be scaled with the addition of new modules to expand capacity. They have been designed for commercial mass production that uses conventional metal fabrication technology.
Another important characteristic of the Climeworks DAC process is that a large share (around 80%) of the energy demand can be met by low-temperature heat in the range of 80–120°C. Heat at these temperatures is available from a variety of sources including industrial low-grade waste heat (Ammar et al., 2012). Long-term energy requirement projections based on current technology assumptions for the DAC process are expected at around 2,000 kWh per ton of CO2 (400 kWh electrical and 1,600 kWh thermal). Climeworks plants can and will be solely powered by renewable energy sources and/or waste heat. The plants do not require a nearby fossil power plant in order to work. Costs per ton of CO2 permanently removed from the atmosphere and safely stored via mineralization (CDR via DACS) are calculated to come down to around $100 per ton of CO25 within a decade, based on the detailed development roadmap for cost reduction and the learnings derived from the total of 14 DAC plants built and set into operation by the company across Europe so far.
In 2009 Climeworks was founded and first working laboratory prototypes were developed. In 2011, Climeworks built its first demonstration prototype. In 2015, a Climeworks system was commissioned for the world's first synthesis of renewable methane from atmospheric CO2.
In 2017, Climeworks commissioned the world's first commercial DAC plant in Hinwil, Switzerland (Figure 3). The plant nominally captures 900 tons of CO2 per year from the air and delivers the CO2 to a nearby greenhouse.
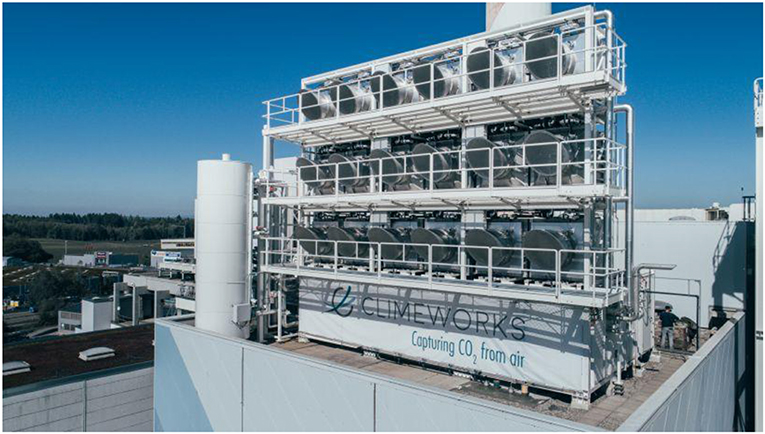
Figure 3. The world's first commercial plant for direct air capture of CO2 located in Hinwil, Switzerland.
2017 marked another milestone: together with their partners and project leader Reykjavik Energy, Climeworks built the world's first negative emissions plant in Icleand using DACS technology, known as the CarbFix2 project. The first CarbFix project was initiated by Reykjavik Energy. The aim of the project is to develop safe, simple and economical methods and technology for permanent CO2 mineral storage in basalts. The first pilot injections took place at Hellisheidi in South West Iceland in 2012; 175 tons of pure CO2 were dissolved and injected at about 500 meters depth and about 35°C (Matter et al., 2016). Following the success of the pilot injections, the project was scaled up to industrial-scale in June 2014 as a part of the CarbFix-SulFix project, with injection of 65% CO2–35% H2S gas mixture at about 800 m depth and about 230°C. The injection is now an integral part of the operations at the Hellisheidi power plant (Sigfusson et al., 2018).
The CarbFix2 project builds on the success of the CarbFix project (as illustrated in Figure 4 below), with the goal of moving the demonstrated CarbFix technology from the demonstration phase, to a general and economically viable complete CDR process chain that is replicable in other parts of the world. To this end, CarbFix2 extended the original CarbFix approach from the demonstration stage in one location to implementation under more diverse conditions. A main goal of the CarbFix2 project is to combine the storage approach with DAC technology, as developed by Climeworks, and thus create an integrated CDR solution with potential for negative emissions as well as global multiplication (Gutknecht et al., 2018). An integrated and safe solution toward permanent CDR has been created through the combination of the CarbFix and Climeworks technologies (Friedl, 2017). To date, the project has run for almost 2 years and demonstrates that negative emissions via DAC with rapid mineralization is possible.
In December 2018, Climeworks announced its partnership with Coca-Cola HBC Switzerland and that Climeworks will be supplying atmospheric CO2 to Coca-Cola HBC Switzerland's mineral water brand Valser from April 2019 on6. For 2020, Climeworks plans to build its so far largest DAC plant, scaling its DACS operations in Iceland by offering to remove emissions from the atmosphere to individuals7. Overall, the 14 DAC plants built by Climeworks so far will be complemented during 2020 with three more plants currently in planning or production. The company employs the currently largest team of DAC experts in the world at 70 full time equivalent positions8.
When facing the need for negative emissions at gigaton scale, considerations have to be given to potentially limiting factors such as land use and resource constraints. Different CDR approaches have different requirements in terms of surface area and type of land they need to operate. Whilst afforestation and BECCS need large quantities arable land to grow trees and biomass that binds carbon, and will thus likely put pressure on ecosystems and food production systems if implemented at gigaton scale (Smith et al., 2015; IPCC, 2018), DACS can operate anywhere where renewable energy (such as PV, wind, or geothermal) can be found or installed. Further advantages of DAC(S) are that it does not require arable land, and has a much smaller physical footprint than bio-based approaches. According to Climeworks' estimations, around 2,000 km2 of non-arable land would be needed to remove 1 gigaton of CO2 net from the atmosphere, including the required renewable energy production. This calculation is based on the assumption that solar photovoltaic (PV) is the sole energy source, where heat is produced using heat pumps with a coefficient of performance (COP) of 3.5 and energy consumption is based on the long-term requirements as shown above. For the PV system, the following assumptions are taken: the PV ground cover ratio is 100% (to be adapted individually to local sites), the PV module efficiency is 25% and the annual solar radiation of suitable solar PV sites is assumed at 1.9 TWh per km2, a value that can be achieved in parts of the US for example9. The footprint of the actual DAC plants would cover just 62 km2. It is important to point out that depending on the location these footprints may vary and their calculation should receive further analysis. However, even if for example doubled in size, the DAC footprint would still be several orders of magnitude smaller than that of other CDR solutions.
CO2 from the atmosphere can also be used for the production of a range of synthetic materials and fuels by using renewable energy (Bhanage and Arai, 2014). This has the advantage that “hard-to-electrify” sectors such as aviation, or long-distance heavy transportation, can be indirectly electrified via synthetic hydrocarbon fuels such as synthetic methane or Fischer-Tropsch fuels (Olah et al., 2009; Verdegaal et al., 2015), as well as a range of other hydrocarbon based products (e.g., polymers) can be made that previously relied on fossil resources. Together with its partners, Climeworks has demonstrated the viability of combining these technologies—usually referred to as Power-to-X (or PtX)—in Troia, Italy10. Here, CO2 from the air is provided for conversion into renewable methane as part of the Horizon 2020-funded STORE&GO research project. The primary objective of the STORE&GO project is to demonstrate the viability of large-volume energy storage through Power-to-Gas technology in real-life applications.
Besides energy storage, another advantage of air-captured CO2 as a feedstock for synthetic fuels is that DAC allows for location-independent sourcing of CO2. This means transport costs as well as emissions from transport are greatly reduced for any application. Being location-independent in particular allows for synthetic fuel production to be performed at locations with most favorable renewable energy prices. A cost advantage of just 1ct/KWh could offset or even overcompensate any potential extra costs of CO2 derived from DAC compared to, for example, CO2 that is captured from industrial point source emissions. Also, if atmospheric CO2 is used, the fuels can be near-CO2 neutral as the CO2 that is emitted to the atmosphere when the fuels are burnt have been previously captured from that atmosphere (as illustrated in Figure 5 above). In this way, DAC technology can contribute to the development of a circular economy independent from fossil hydrocarbons and to conventional mitigation as well. There are further co-benefits of DAC, especially in relation to the UN sustainable development goals. There are positive effects on SDGs 13, 9, 6 as the byproduct H2O could be used as fresh water in general and, after corresponding purification, as drinking water in particular. A DAC expansion would bring new green jobs and thereby contribute to SDG 8, whilst it can also drive the scale-up of renewable energy, thereby driving SDG 7. DAC as a feedstock for PtX has positive effects on SDG 12 and 11 by allowing cities to produce their own fuels and materials, including synthetic building materials made from atmospheric CO2. Lastly because synthetic fuels release far less air pollutants when burned (Olah et al., 2009) DAC could also have a positive effect on health and well-being (SDG 3).
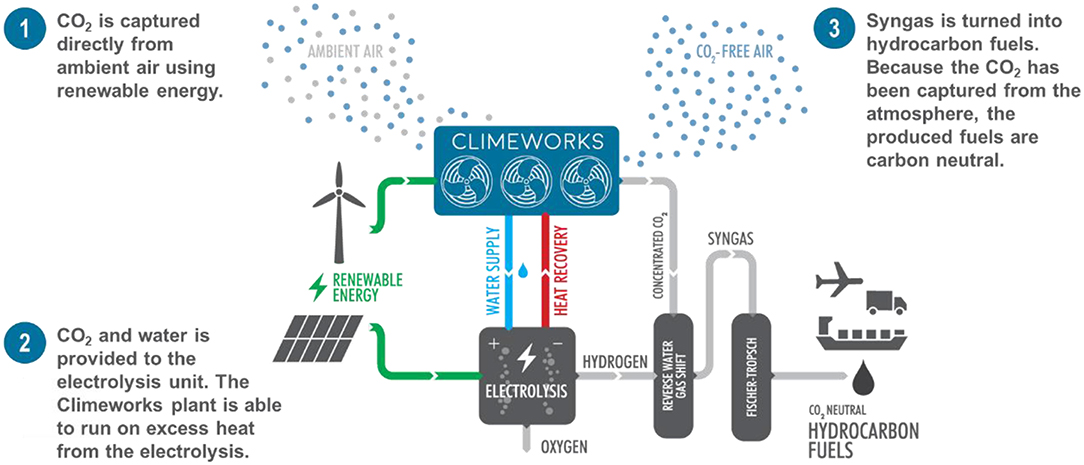
Figure 5. Schematic illustration of the process chain for the production of renewable hydrocarbon fuels (in this case Fischer-Tropsch fuels), including DAC.
Besides their removal and mitigation potential and contribution to SDGs, DAC and other NETs might offer more intangible but likewise valuable benefits—namely their contributions to the outlook on, and narrative around, the current climate crisis.
Over the last four decades, it has become apparent how difficult it is to agree on, and implement, appropriate policies that result in the mitigation pathways so urgently needed. In part, because in the absence of technologies that could replace fossil resources, such policies would have to be based on abstinence thereby resulting in having to relinquish a large proportion of lifestyles that especially those in industrialized countries have become accustomed to. Being able to decarbonize, for example, air travel with DAC and synthetic fuels at least offers the possibility of continuing to fly in a carbon neutral way.
Also notable is the current narrative around the reduction of carbon emissions to zero, in which lies implicitly the assumption that any human activity is a burden on the planet, as it is bound to have some emissions. In this context NETs open up the possibility of a much more positive self-image around a balance between residual (unavoidable) carbon emissions and natural carbon sinks or CDR, that humankind can actively manage and be the responsible stewards of.
Under current economic conditions that largely have not priced in the externalities of greenhouse gas emissions, CO2 for niche markets, fuels and materials is a way for DAC companies to operate already today.
Current markets will likely allow DAC and NETs companies to grow to considerable size. However, these markets will almost certainly not be large enough to become “climate relevant,” i.e., to produce negative emissions at the scales that are forecast/needed in time. To reach these scales, these externalities need to be priced in or regulated, which in turn means designing appropriate policies to ensure the scale-up needed.
Various climate scenarios predict negative emissions at gigaton scale by mid-century. What does this mean for CDR scale-up pathways? An example: to reach a mean pathway of around 6 gigatons of CDR by 2050 as calculated in a recent comprehensive review of the relevant literature (Nemet et al., 2018), from 2019 onwards, CDR would require an annual growth rate of over 55%. Delaying scale-up to 2025 would already require a sustained growth of 80% per year, whilst scale-up starting in 2030 when most CDR policies are currently recommended to set in, would require roughly a yearly doubling of CDR capacity. Scales like these are hard to achieve and from a risk perspective, it would therefore be vital to start scaling earlier.
To achieve such a feat, the dialogue between science, NET providers and policy makers urgently needs strengthening. Effective policy measures need to be developed and adopted, both for R&D on CDR and for its scale-up. How should these look like?
From the perspective of a NET/DAC company, first the technologies need to be made eligible in existing mitigation policies wherever possible. However, new policies also need to be designed so current NETs can make use of them.
A good example is the recently passed 45Q tax credit in the US, where DAC is eligible for CDR11. However, the policy still has a number of limitations. Firstly, the minimum installed capacity for eligibility is 100,000 tons of CDR per year. Building plants of this size would very likely exceed current market capitalization of all leading DAC companies and put the ones that decide to try at considerable risk of failure. In addition, the revenue stream the 45Q would create (at up to USD 50 per ton of CO2), would not be sufficient to cover the costs of atmospheric CDR with current technologies (Keith et al., 2018)12.
In general, any policy that puts a price on carbon usually plans to start low and raise the price subsequently in order to not disrupt current economic processes too much. However, technologies follow the opposite pathway. These are expensive to start with and have low capacities. Importantly, though, costs will fall rapidly as soon as the new technologies are being implemented. Future CDR policies thus need to accommodate this fact by allowing for lower removal thresholds at higher prices. As the installed capacity increases, the prices may fall corresponding to the (assumed rapid) technological development. In other words, the overall CDR volume that requires policies with higher prices, is relatively small and the absolute amount of money, “the world needs to spend on high-price CDR” is negligible compared to the required gigaton-scale carbon removal.
Also, polices like the recently passed European Renewable Energy Directive 2 (RED2) allow for atmospheric CO2 as a feedstock for synthetic fuels (renewable fuels of non-biological origin) as described above. However, the regulation also allows for CO2 captured from flue gas from (fossil) point sources. CO2 from flue gas can be typically sourced at much lower cost, as the CO2 concentration in the gas stream is higher. In the marketplace, such regulations therefore place an incentive on CCS CO2 rather than CO2 captured from air, again not triggering much needed CDR scale-up effects.
Lastly, unless the CO2 in the flue gas stream comes from biomass, CO2 from fossil point sources does not have the same CO2 reduction effect, as fossil CO2 in essence is reused once more in the synthetic fuel, before it is released back into the atmosphere. It is therefore important that this is reflected in appropriate life cycle analysis (LCA) methodologies (e.g., cradle to grave instead of cradle to gate) and regulations (von der Assen et al., 2013; Cuéllar-Franca and Azapagic, 2015; von der Assen, 2015).
Conclusion
DAC is a promising set of technologies that can deliver negative emissions and contribute to mitigation at scale. There are different technological approaches to DAC resulting in different resource requirements. It is important that these differences are taken into account, especially for large-scale predictions of NETs potentials. In general, DAC's main benefit is that the technology is space efficient and can be built on non-arable land, therefore not adding further pressure on either ecosystems or food systems. The technology can be used as a sustainable CO2 feedstock for PtX technologies (synthetic fuels and materials). Such applications can help the scaling-up of DAC in the absence of a functioning price mechanism or policy for CDR. Negative emissions technologies like DAC can have a positive Impact on UN sustainable development goals and can create a positive impetus for narratives around climate change. DAC's biggest challenge is achieving climate-relevant scale, which—as any other NET—it cannot do without a sufficiently high price on carbon or other relevant policy measures. Because of the scales likely needed and the time it takes to develop these, climate policy urgently needs to develop and implement suitable mechanisms to trigger sufficient mitigation and scaling of NETs alike. As any other NET, DAC is not a substitute for mitigation but rather needed in addition to ambitious mitigation efforts. Like any NET, DAC is also not a silver bullet though likely to be a main contributor to a future NETs portfolio.
Author Contributions
This paper was written by CB with inputs from JW and LC. Any opinions and own calculations presented in this article state the views of the main author only and are not necessarily shared by his affiliations.
Conflict of Interest
All authors are employed by Climeworks AG.
Acknowledgments
The authors would like to thank Christoph Gebald and Louis Uzor for their contributions to this paper. Further, the authors thank the editors of this journal for the invitation to contribute to this issue.
Footnotes
1. ^Notably, “overshoot” is actually just a different formulation for building a carbon removal debt.
2. ^Graph by Climeworks, based on a Graph by Mercator Research Institute on Gloabl Commons and Climate Change (2019) and Data from IPCC (2018). 2.6 RCP. Blue shading represents the amount of negative emissions likely needed in this scenario.
3. ^There is regional and seasonal variation, of several ppm, which affects DAC only marginally. The variability in near surface CO2 concentrations is visualized on the cover of the Royal Society report on Greenhouse Gas Removal: https://royalsociety.org/-/media/policy/projects/greenhouse-gas-removal/royal-society-greenhouse-gas-removal-report-2018.pdf
4. ^With current technology in moderate climate conditions roughly at an average ratio of 1 ton of H2O per ton of CO2.
5. ^For its cost calculations, Climeworks currently assumes 12 years plant life time and 20 years in the long term. Lifetimes in industries of comparable equipment are typically at the order of 30 years.
6. ^https://ch.coca-colahellenic.com/en/news-and-media/news/valser-to-get-its-fizz-from-the-air-with-climeworks/
8. ^As of August 2019.
9. ^https://solargis.com/maps-and-gis-data/download/world
11. ^https://www.betterenergy.org/blog/primer-section-45q-tax-credit-for-carbon-capture-projects/
12. ^As well as Climeworks's own calculations.
References
Ammar, Y., Joyce, S., Norman, R., Wang, Y., and Roskilly, A. (2012). Low grade thermal energy sources and uses from the process industry in the UK. Appl. Energy 89, 3–20. doi: 10.1016/j.apenergy.2011.06.003
Bhanage, B., and Arai, M. (2014). Transformation and Utilization of Carbon Dioxide. Oxford: Springer.
Cuéllar-Franca, R., and Azapagic, A. (2015). Carbon capture, storage and utilisation technologies: a critical analysis and comparison of their life cycle environmental impacts. J. CO2 Util. 9, 82–102. doi: 10.1016/j.jcou.2014.12.001
European Academies Science Advisory Council (2018). Negative Emission Technologies: What Role in Meeting Paris Agreement Targets? EASAC Policy Report 35. Available online at: https://easac.eu/fileadmin/PDF_s/reports_statements/Negative_Carbon/EASAC_Report_on_Negative_Emission_Technologies.pdf
Friedl, M. (2017). Pilot- und Demonstrationsanlage Power-to-Methane HSR. Schlussberich. Available online at: https://www.iet.hsr.ch/fileadmin/user_upload/iet.hsr.ch/Power-to-Gas/Berichte_Illustrationen/HSR_Schlussbericht_PiDo_V3.pdf
Gutknecht, V., Snaebjörnsdottir, S., Sigfusson, B., Aradottir, E., and Charles, L. (2018). Creating a carbon dioxide removal solution by combining rapid mineralization of CO2 with direct air capture. Energy Procedia 146, 129–134. doi: 10.1016/j.egypro.2018.07.017
IPCC (2014). Climate Change 2014: Synthesis Report. Contribution of Working Groups I, II and III to the Fifth Assessment Report of the Intergovernmental Panel on Climate Change. Geneva: IPCC. Available online at: https://www.ipcc.ch/report/ar5/syr/
IPCC (2018). Global Warming of 1.5°C. Available online at: https://www.ipcc.ch/sr15/
Kaya, Y., Yamaguchi, M., and Geden, O. (2019). Towards net zero CO2 emissions without relying on massive carbon dioxide removal. Sustain. Sci. 14, 1739–1743. doi: 10.1007/s11625-019-00680-1
Keith, D., Holmes, G., St. Angelo, D., and Kenton, H. (2018). A process for capturing CO2 from the atmosphere. Joule 2, 1573–1594. doi: 10.1016/j.joule.2018.05.006
Matter, J., Stute, M., Snaebjörnsdottir, S., Oelkers, E., Gislason, S., Aradottir, E., et al. (2016). Rapid carbon mineralization for permanent disposal of anthropogenic carbon dioxide emissions. Science 352, 1312–1314. doi: 10.1126/science.aad8132
Mercator Research Institute on Gloabl Commons Climate Change (2019). Betting on Negative Emissions. Available online at: https://www.mccberlin.net/en/research/negativeemissions.html (accessed July 30, 2019).
Nemet, G., Callaghan, M., Fuss, S., Creutzig, F., Hartmann, J., Hilaire, J., et al. (2018). Negative emissions—Part 3: innovation and upsacaling. Environ. Res. Lett. 13:6. doi: 10.1088/1748-9326/aabff4
Olah, G., Goeppert, A., and Surya Prakash, G. (2009). Chemical recycling of carbon dioxide to methanol and dimethyl ether: from greenhouse gas to renewable, environmentally carbon neutral fuels and synthetic hydrocarbons. J. Organ. Chem. 74, 487–498. doi: 10.1021/jo801260f
Ping, E., Sakw-Novak, M., and Eisenberger, P. (2018). “Global thermostat low cost direct air capture technology,” in International Conference on Negative CO2 Emissions (Göteborg).
Quere, C. L., Andrew, R. M., Friedlingstein, P., Sitch, S., Hauck, J., and Pongratz, J. (2018). Global carbon budget 2018. Earth Syst. Sci. Data 10, 2141–2194. doi: 10.5194/essd-10-2141-2018
Realmonte, G., Drouet, L., Gambhir, A., Glynn, J., Hawkes, A., Köberle, A., et al. (2019). An inter-model assessment of the role of direct air capture in deep mitigation. Nat. Commun. 10:3277. doi: 10.1038/s41467-019-10842-5
Rogelj, J., Popp, A., Calvin, K. V., Luderer, G., Emmerling, J., Gernaat, D., et al. (2018). Scenarios towards limiting global mean temperature increase below 1.5°C. Nat. Clim. Change 8, 325–332. doi: 10.1038/s41558-018-0091-3
Sigfusson, B., Arnarson, M., Snaebjörnsdottir, S., Karlsdottir, M., Aradottir, E., and Gunnarsson, I. (2018). Reducing emissions of carbon dioxide and hydrogen sulphide at Hellisheidi power plant in 2014–2017 and the role of CarbFix in achieving the 2040 Iceland climate goals. Energy Procedia 146, 135–145. doi: 10.1016/j.egypro.2018.07.018
Smith, P., Davis, S., Creutzig, F., Fuss, S., Minx, J., Gabrielle, B., et al. (2015). Biophysical and economic limits to negative CO2 emissions. Nat. Clim. Change 6, 42–50. doi: 10.1038/nclimate2870
Strefler, J., Bauer, N., Kriegler, E., Popp, A., Giannousakis, A., and Edenhofer, O. (2018). Between Scylla and Charybdis: delayed mitigation narrows the passage between large-scale CDR and high costs. Environ. Res. Lett. 13:4. doi: 10.1088/1748-9326/aab2ba
United Nations (2015). United Nations Framework Convention on Climate Change. Adoption of the Paris Agreement. FCC/CP/2015/L.9. Paris: UN. Available online at: https://treaties.un.org/doc/Treaties/2016/02/20160215%2006-03%20PM/Ch_XXVII-7-d.pdf
Verdegaal, W., Becker, S., and von Olshausen, C. (2015). Power-to-liquids: synthetic crude oil from CO2, water and sunshine. Chem. Ing. Tech. 87, 340–346. doi: 10.1002/cite.201400098
von der Assen, N. (2015). From Life-Cycle Assessment towards Life-Cycle Design of Carbon Dioxide Capture and Utilitzation. Aachener Beiträge zur Technischen Thermodynamik. Available online at: http://publications.rwth-aachen.de/record/570980/files/570980.pdf
von der Assen, N. V., Jung, J., and Bardow, A. (2013). Life-cycle assessment of carbon dioxide capture and utilization: avoiding the pitfalls. Energy Environ. Sci. 6:2721. doi: 10.1039/C3EE41151F
World Meteorological Organization (2019). State of the Climate in 2018 Shows Accelerating Climate Change Impacts. Abgerufen: World Meteorological organization. Available online at: https://public.wmo.int/en/media/press-release/state-of-climate-2018-shows-accelerating-climate-change-impacts (accessed June 30, 2019).
Keywords: direct air capture, DACS, negative emissions, NETs, mitigation, climate policy, climate change
Citation: Beuttler C, Charles L and Wurzbacher J (2019) The Role of Direct Air Capture in Mitigation of Anthropogenic Greenhouse Gas Emissions. Front. Clim. 1:10. doi: 10.3389/fclim.2019.00010
Received: 01 May 2019; Accepted: 25 October 2019;
Published: 21 November 2019.
Edited by:
Jennifer Wilcox, Worcester Polytechnic Institute, United StatesReviewed by:
Peter Campbell Psarras, Colorado School of Mines, United StatesPhillip Williamson, Natural Environment Research Council (NERC), United Kingdom
Copyright © 2019 Beuttler, Charles and Wurzbacher. This is an open-access article distributed under the terms of the Creative Commons Attribution License (CC BY). The use, distribution or reproduction in other forums is permitted, provided the original author(s) and the copyright owner(s) are credited and that the original publication in this journal is cited, in accordance with accepted academic practice. No use, distribution or reproduction is permitted which does not comply with these terms.
*Correspondence: Christoph Beuttler, Y2hiQGNsaW1ld29ya3MuY29t