Siberian Ecosystems as Drivers of Cryospheric Climate Feedbacks in the Terrestrial Arctic
- 1Department of Geography, Colgate University, Hamilton, NY, United States
- 2School of Forestry and Wildlife Sciences, Auburn University, Auburn, AL, United States
- 3Environmental Studies Program, Hamilton College, Clinton, NY, United States
- 4School of Natural Resources and Environment, University of Florida, Gainesville, FL, United States
Climate warming is altering the persistence, timing, and distribution of permafrost and snow cover across the terrestrial northern hemisphere. These cryospheric changes have numerous consequences, not least of which are positive climate feedbacks associated with lowered albedo related to declining snow cover, and greenhouse gas emissions from permafrost thaw. Given the large land areas affected, these feedbacks have the potential to impact climate on a global scale. Understanding the magnitudes and rates of changes in permafrost and snow cover is therefore integral for process understanding and quantification of climate change. However, while permafrost and snow cover are largely controlled by climate, their distributions and climate impacts are influenced by numerous interrelated ecosystem processes that also respond to climate and are highly heterogeneous in space and time. In this perspective we highlight ongoing and emerging changes in ecosystem processes that mediate how permafrost and snow cover interact with climate. We focus on larch forests in northeastern Siberia, which are expansive, ecologically unique, and studied less than other Arctic and subarctic regions. Emerging fire regime changes coupled with high ground ice have the potential to foster rapid regional changes in vegetation and permafrost thaw, with important climate feedback implications.
Introduction
Amplified climate warming across the Arctic is causing widespread terrestrial cryospheric change in the form of altered snow cover dynamics and permafrost thaw, both with the potential to act as globally important climate feedbacks (Flanner et al., 2011; Schuur et al., 2015; Meredith et al., 2019). Reduced albedo associated with declines in snow cover duration and increased snow masking by vegetation canopies protruding above the snowpack acts as a positive climate feedback (Bonan et al., 1992; Chapin, 2005). Permafrost thaw makes previously inaccessible organic carbon available for microbial decomposition and subsequent release to the atmosphere as greenhouse gases, also acting as a positive feedback to climate warming (Schuur et al., 2015). These processes are not independent; increases in snow depth insulate permafrost soils from cold winter air temperatures, further increasing greenhouse gas efflux and the likelihood of thaw (Stieglitz et al., 2003; Anisimov and Zimov, 2020). Consequently, concurrent changes in snow cover and permafrost thaw across the terrestrial Arctic and subarctic will dictate the strength of terrestrial cryospheric climate feedbacks. However, quantifying these changes remains a key challenge in climate research because they are affected by spatially heterogeneous interactions between climatic, ecological, and geomorphic conditions, as well as disturbances such as fire (Loranty et al., 2018).
Among ecological factors, vegetation is a key determinant of cryospheric feedback strengths because of the role it plays in surface energy partitioning (Loranty et al., 2018). Variability in vegetation canopy cover, height, and leaf habit within and between vegetation functional types at local and regional scales drives differences in land surface albedo that are important in the context of snow-albedo-feedback magnitudes (Sturm et al., 2005; Loranty et al., 2014). Similarly, vegetation canopy influences on snow redistribution impact the timing and duration of melt-out (Pomeroy et al., 2006; Marsh et al., 2010; Sweet et al., 2014) and soil thermal dynamics (Kropp et al., 2020). Without snow cover, vegetation canopy shading may reduce permafrost soil temperatures, while plant water use and soil properties interact to affect ground heat flux (Blok et al., 2010; Loranty et al., 2018). Taken together, these processes highlight the important fact that an accurate predictive understanding of terrestrial cryospheric change across the northern hemisphere requires detailed knowledge of ongoing vegetation change. In particular, changes that involve transitions from prostrate to erect shrub growth forms, transitions in leaf habit (e.g., deciduous vs. evergreen), or substantial changes in shrub and forest canopy cover are especially important.
Vegetation structure and distribution are changing in direct response to warming temperatures, with the most prominent example being shrub expansion into graminoid dominated tundra ecosystems (Tape et al., 2006; Forbes et al., 2010; Frost and Epstein, 2014). Here, the associated reduced albedo (Loranty et al., 2011), snow trapping by canopies (Sturm et al., 2001), and increased ground temperatures (Myers-Smith and Hik, 2013; Kropp et al., 2020) are well documented. Wildfire disturbance is also an important driver of vegetation change, with altered post-fire succession as a consequence of climate-driven fire regime shifts in boreal North America forming the primary basis of current knowledge (Johnstone et al., 2010; Alexander and Mack, 2016). Associated post-fire albedo recovery (Amiro et al., 2006; Randerson et al., 2006), albedo associated with deciduous dominance (Beck et al., 2011), and elevated ground temperature (Fisher et al., 2016; Way and Lapalme, 2021) are among the key consequences. Notably, however, there has been less work focused on boreal canopy-snow interactions, and previous fire focused work occurs mostly in the discontinuous and sporadic permafrost zones where permafrost may not be present. Conspicuously absent from this emerging body of research are analyses focused on ecosystem-cryosphere interactions in the Siberian Arctic and subarctic (Metcalfe et al., 2018), despite a unique combination of ecological and cryospheric conditions and extensive areal extent that makes it regionally important. In this perspective, we highlight and synthesize recent research and emerging evidence illustrating the potential for ecosystem processes to drive rapid and widespread cryospheric change across central and eastern Siberia, with particular focus on larch (Larix spp.) dominated forests.
The Siberian Climatic and Ecological Setting
Eastern Siberia is characterized by winters with extreme low air temperatures with thin to moderate snow accumulation that reaches maximum depths of 20–100 cm between January and March (Grippa et al., 2004; Zhong et al., 2018; Mortimer et al., 2020). Maximum snow depth increased throughout much of Siberia over recent decades (Bulygina et al., 2009). Snow accumulation typically begins around October, and snow extent is largely diminished by June (Grippa et al., 2004). The duration of total days of snow cover is decreasing in northern and central regions (Bulygina et al., 2009; Bormann et al., 2018) coinciding with below average May and June snow cover extent in recent decades (Mudryk et al., 2017, 2020). On the ecosystem and landscape scales, patterns of snow depth and timing of snowmelt can be varied, influenced by localized factors including topography, vegetation canopy characteristics, and tree density (Sturm et al., 2001; Todt et al., 2018).
Continuous permafrost occurs at subarctic latitudes (~60°N) in central and eastern Siberia, owing to the harsh continental climate. Thick, carbon and ice-rich permafrost deposits (termed Yedoma) are prevalent in Northern Siberia, particularly in lowlands and foothills (Grosse, 2013; Strauss et al., 2017). The high ice content (~80% by volume) of Yedoma deposits means they are highly vulnerable to temperature-induced permafrost degradation, and thermokarst lakes are prevalent throughout the region (Olefeldt et al., 2016; Nitze et al., 2018). Permafrost thaw has also been documented in the more mountainous areas of Siberia, where hill slope thermokarst features such as retrogressive thaw slumps are common (Olefeldt et al., 2016; Nitze et al., 2018).
Siberia is characterized primarily by boreal forests and wetlands with a transition to tundra ecosystems a few degrees of latitude north of the Arctic circle. Considerable landscape heterogeneity is driven by local variation in topoedaphic and hydrologic conditions (Sulla-Menashe et al., 2011). Tundra vegetation communities are comparable to those found in North America, with similar climatic and geomorphic controls on distribution (Walker et al., 2005). Relative to North America, the forest-tundra ecotone generally occurs further north and is more diffuse in Siberia (Ranson et al., 2011) owing to differences in geomorphology and glacial history. Siberian boreal forests differ in several key ways from those in North America. Eastern Siberian boreal forests are dominated by larch (Larix spp.), a deciduous needle-leaf tree. Larch forests tolerate cold soil conditions, and cover more than 3 M km2 with in the permafrost zone (Loranty et al., 2016). By contrast, boreal forests in North America and western Siberia are composed of evergreen conifers and deciduous broadleaf trees and are underlain by sporadic or isolated permafrost.
The deciduous, needle-leaf larch (Larix spp.) forests that cover vast expanses of Siberia's boreal biome require periodic fire disturbances to perpetuate their long-term persistence in this region (Kharuk et al., 2011). Fires, ignited by lightning or humans during the warm and relatively dry growing season (Kharuk et al., 2021), occur every 80–350 years, with fire return intervals increasing with latitude (Kharuk et al., 2011; Berner et al., 2012). Fires are typically surface fires (>90%) that creep along the forest floor, fueled by consumption of the thick soil organic layer (SOL) that accumulates during the fire free intervals (Kharuk et al., 2021). Fire removal of the SOL exposes high-quality mineral soil seed beds that promote seed germination (Sofronov and Volokitina, 2010; Alexander et al., 2018). Fire also consumes shrubs and other vegetation, decreasing competition, and improving conditions for early seedling establishment (Kharuk et al., 2011, 2021). Without fire to prepare the seedbed and remove competition, larch recruitment is often limited to microsites provided by tip-up mounds or other small-scale disturbances (Shirota et al., 2006).
Fire activity varies across Siberia, and much of what is known comes from research in western and central Siberia with a limited understanding of fire activity in eastern Siberia. Across Siberia, the area burned and the number of large fire seasons (i.e., over 50% area burned) has increased between 1995 and 2005 (Soja et al., 2007), but the full extent of area burned is often underestimated by coarse resolution satellites (Shvidenko et al., 2011; Berner et al., 2012) e.g., MODIS ~500 m; (Shvidenko et al., 2011; Berner et al., 2012). It is predicted that greater combustion losses and permafrost thaw during more extreme fire seasons will increase carbon emissions (Soja et al., 2004). In northwestern Siberia, warmer and drier conditions (Soja et al., 2007; Ponomarev et al., 2016) as well as human activity (Sizov et al., 2021) have contributed to increased fire activity. In northeastern Siberia fire regimes are less well understood (Berner et al., 2012) because long-term ground-based records are limited and difficult to access (Soja et al., 2007). Improved quantification of regional fire dynamics is crucial to identifying fire-induced vegetation shifts and altered soil conditions (Holloway et al., 2020) that act as catalysts for permafrost degradation (Loranty et al., 2016), and contribute further to ongoing climate feedbacks.
Larch forest recovery after fire requires a co-occurring suite of conditions. Fires often damage larch roots (Kharuk et al., 2021), which are mostly restricted to the shallow, seasonally-thawed ground layer above the permafrost (Abaimov et al., 1998). Consequently, many mature, seed-bearing larch die soon after fire, resulting in stand-replacing fires. Thus, a critical primary filter for larch forest recovery after fire is seed availability, which depends on several factors. Larch annually produce small, wind-dispersed seeds that can travel ~100 m (Cai et al., 2018), with masting events (large seed crops) every 3–5 years (Abaimov, 2010). As such, small seed crops in non-mast years can limit post-fire larch recovery despite fire's creation of appropriate seedbed conditions. However, if the seed crop is substantial, and fires do not propagate into the larch canopy, already ripe seeds on fire-killed trees can provide seed sources for forest recovery (Kharuk et al., 2021). If canopy fires damage seeds, recovery depends on seeds from nearby unburned forests or islands of unburned trees. Thus, seed availability may be low within the interior of large burn patches whose size exceeds that of larch seed dispersal distances (Cai et al., 2013), especially in low seed crop years, unless residual seed crops persist within the burn (Cai et al., 2018). Recruitment failure may occur in areas with ice-rich yedoma permafrost where charring and vegetation removal triggers ground subsidence and pooling of water on the ground surface inhibits larch seed germination (Alexander et al., 2018). Ultimately, low seed availability or failure to germinate after fire can result in larch recruitment failure and forest transition to alternative vegetation cover types, including shrublands and grasslands (Cai et al., 2013, 2018; Chu et al., 2017).
Discussion of Emerging Ecosystem Changes With Implications for Cryospheric Climate Feedbacks
Vegetation cover is an important determinant of the snow albedo feedback (Thackeray et al., 2019). As with elsewhere in the Arctic (Beck and Goetz, 2011), tree and shrub cover are increasing across Siberia in response to rising temperatures (Frost and Epstein, 2014; Shevtsova et al., 2020, 2021). The prevalence of canopy infilling in larch forests (Shevtsova et al., 2020) combined with relatively low canopy cover (Loranty et al., 2014) and fragmented nature of the forest-tundra ecotone (Ranson et al., 2011) in the region create a potential for changes in forest cover over large areas. These vegetation changes will act as a positive climate feedback when increased canopy cover masks the underlying snowpack thereby reducing albedo. However, when vegetation masks snow the difference between snow-on and snow-off albedo is lower, and the strength of climate feedbacks associated with changes in the duration of seasonal snow cover is reduced (Euskirchen et al., 2016; Potter et al., 2020). Accurate representation of ongoing vegetation change is therefore integral to observational quantification and predictive modeling of the snow albedo feedback strength.
Fire is an important driver of vegetation change, and though it is common in the region, the past several years have been exceptional both in terms of total area burned, as well as the proportion of area burned above the Arctic Circle. In northeastern Siberia, the 2020 fire season alone constitutes nearly 30% of the cumulative area burned over the past two decades. During the 2019 and 2020 fire seasons the area burned in the Arctic (3.4 and 5.4 million hectares, respectively) was much greater than the average over the previous two decades (0.7 million hectares) (Figure 1A; Talucci, in review). Additionally, recent albedo trends suggest a fire regime shift may be underway (Webb et al., 2021). If these fire regime shifts are sustained, the potential impacts on forest cover associated with recruitment dynamics (Alexander et al., 2012, 2018; Paulson et al., 2021) have important implications for cryospheric climate feedbacks. The extent to which increases in winter albedo immediately postfire (Chen et al., 2018; Stuenzi and Schaepman-Strub, 2020) are reversed during post-fire succession will depend on the density of forest regrowth (Talucci et al., 2020; Paulson et al., 2021; Walker et al., 2021). Similarly, forest impacts on surface energy exchange exert a series of interrelated controls on permafrost and snow thermal dynamics (Loranty et al., 2018; Stuenzi et al., 2021). Variation in forest recovery within a single fire perimeter near Cherskiy has resulted in even-aged stands with canopy cover ranging from ~10–95% (Paulson et al., 2021), and winter albedos of ~0.3 and ~0.7 at representative high- and low- density stands (Kropp and Loranty, 2018; Kropp et al., 2019). Corresponding to post-fire tree density, summer and winter soil temperatures are warmer at sites with lower tree cover (Figure 1B) as a result of lower canopy shading and insulation from organic soils, and snow redistribution, respectively. Fire-induced vegetation change will exert strong control on albedo and permafrost climate feedbacks if this type of variation in post-fire larch regrowth becomes more common. Consequently, accurate model predictions of these cryospheric climate feedbacks requires improved observational understanding of fire impacts on vegetation-snow-permafrost interactions in the region.
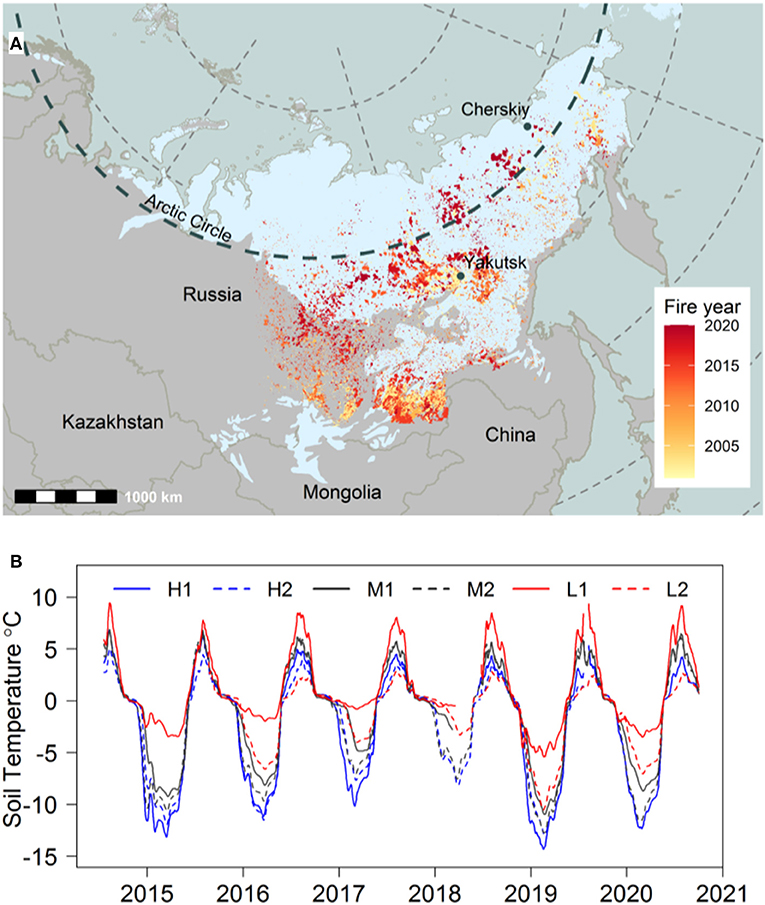
Figure 1. Area burned (A) in eastern Siberia from 2001–2020 derived from Landsat data using Google Earth Engine (Talucci et al., 2021) with areas of continuous permafrost delineated in light blue. Note more area has burned (in red) in recent years above the Arctic circle (gray dashed line). Soil temperature (B) across a forest density gradient associated with post-fire regrowth within a burn perimeter near Cherskiy where H1 and H2 represent high-density stands (> 75% canopy cover), M1 and M2 represent medium density stands (75% > canopy cover > 25%), and L1 and L2 represent low density stands (<25% canopy cover) (Loranty and Alexander, 2021).
Changes in snow cover, and snow-vegetation interactions, are likely to influence the distribution and rates of permafrost thaw. Snow is an effective insulator that decouples permafrost soils from frigid winter air temperatures (Jorgenson et al., 2010; Park et al., 2015), and numerous manipulation experiments have illustrated that increases in snow depth can lead to permafrost thaw and increased greenhouse gas emissions (Walker et al., 1999; Natali et al., 2014; Webb et al., 2016). For example, in northeastern Siberia near Cherskiy, snow depths were nearly double the long-term average in the winters beginning in 2016 and 2017, resulting in exceptionally deep seasonally thawed active layers that did not fully re-freeze in subsequent winters (Anisimov and Zimov, 2020). While these findings are consistent with model projections (Räisänen, 2008; Mankin and Diffenbaugh, 2015), and the relationship between snow cover and permafrost soil temperature is not unique to Siberia (Lawrence and Slater, 2010), the high ground ice and carbon content of Siberian permafrost mean that ground thaw in the region will be irreversible once started and lead to high carbon emissions (Strauss et al., 2017). The observations reported by Anisimov and Zimov (2020) highlight the following important points regarding the impacts of snow cover on permafrost climate feedbacks. First, changes in snow cover can initiate permafrost thaw even in regions such as northeastern Siberia where air and permafrost temperatures are low (Anisimov and Zimov, 2020). Additionally, the relative impact of increased snow depth on permafrost varies with vegetation and disturbance history (Shur and Jorgenson, 2007). This highlights the importance of vegetation snow interactions, which are well documented at the local scale (Essery and Pomeroy, 2004), and appear to be important yet less well understood at the pan-Arctic scale (Kropp et al., 2020). Lastly, Anisimov and Zimov (2020) noted the greatest impacts of increased snow cover on permafrost in recently burned locations devoid of vegetation and organic soils, which is in line with observations of an inverse relationship between seasonally thawed active layer depth and time since fire for forests in the region (Webb et al., 2017; Alexander et al., 2018; Kharuk et al., 2021). Taken together, this evidence indicates that vegetation-snow interactions are of crucial importance for albedo and permafrost related climate feedbacks. The ability of fire to foster rapid vegetation change over large areas in relatively short time spans means that changing fire regimes may exert very strong influences on regional cryospheric feedbacks in the coming decades.
Wildfire, and climate warming more generally, thaw permafrost and cause ground subsidence or thermokarst formation (Jones et al., 2015; Yanagiya and Furuya, 2020). Thermokarst features, especially lakes, are common in Siberia, and surface water extent (e.g., lake size, river discharge, and moisture stored in the active layer) is changing across the region—increasing in some areas and decreasing in others—likely due to climate-change induced shifts in precipitation and permafrost thaw (Iijima et al., 2010; Karlsson et al., 2012; Vey et al., 2013; Boike et al., 2016; Nitze et al., 2017; Wang et al., 2021). Changes in the areal extent of surface waters have important implications for localized albedo-mediated heat transfer (that is, the presence of water can increase near surface temperatures by as much as 10°C; (Jorgenson et al., 2010) as well as regional warming and cooling through albedo-induced changes in top-of-the atmosphere radiative forcing (Webb et al., 2021). Overall, changes in Siberian surface water over the past two decades have led to regional albedo-induced warming (Webb et al., 2021), although the pattern is heterogeneous across the region (Figure 2). Thermokarst lakes are also CH4 emission hotspots (Heslop et al., 2020) that effectively increase global warming potential of greenhouse gas emissions related to permafrost thaw.
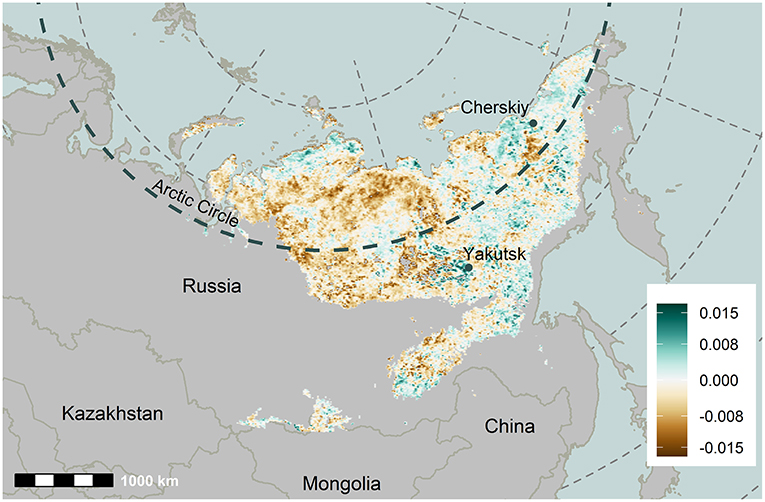
Figure 2. Trends in albedo change (April–September of 2000-2019) attributed to surface water change derived using MODIS data. The standard MODIS albedo product was used to characterize albedo trends using linear regression, and the surface water change was quantified using the Superfine Water Index calculated from MODIS data. A generalized additive model (GAM) was used to attribute albedo trends to surface water change. Adapted from Webb et al. (2021).
Conclusions
Eastern Siberia contains a unique combination of fire-prone larch forests and ice-rich yedoma permafrost. The ability of fire to alter vegetation and permafrost conditions over relatively short time scales has the potential to influence climate feedbacks associated with albedo change and permafrost thaw. However, our understanding of ongoing change in the region is lacking relative to other areas of the Arctic, thus highlighting a need for additional work in the region. Specifically, there is a crucial need to understand how emerging changes in the fire regime are likely to impact post-fire vegetation recovery, and how this in turn will affect permafrost resilience. Variation of vegetation-snow interactions, and associated impacts on permafrost stability also requires closer examination. The latter is particularly important in the context of surface water induced albedo trends. Accurately predicting the timing and magnitude of the cryospheric climate feedbacks arising from terrestrial ecosystem change in the region requires improved understanding of key processes achieved through continued observational and model investigation. For example, a recent study in northern Siberia conducted using a model with explicit representation of ice-rich permafrost dynamics, informed with field observations, predicted a much greater degree of permafrost thaw than previous projections that lacked these process representations (Nitzbon et al., 2020). Similar research utilizing observations and models to investigate interactions between fire, vegetation, and permafrost will help improve forecasts of ecological change in the region and identify key processes necessary to help constrain feedback processes in land surface and climate models. It is also important to note that a great deal of research on this region has been published in the Russian literature, and so translation of Russian papers and data offers a means to improve understanding of the region. Additionally, eastern Siberia includes large indigenous populations, who can provide additional insight to cryospheric change given their reliance on cryospheric resources for a range of basic needs (Fedorov, 2019), and through their long-term landscape-based perspectives that may be missed by western scientific research (Crate et al., 2017).
Data Availability Statement
The datasets shown in Figure 1 can be found at the Arctic Data Center (Figure 1A; https://doi.org/10.18739/A2N87311N, Figure 1B; https://doi.org/10.18739/A24B2X59C). Data from Figure 2 are publicly available MODIS data as described by Webb et al. (2021).
Author Contributions
ML, AT, and EW prepared the figures. All authors contributed to conception and design of the paper, and wrote sections of the manuscript, contributed to manuscript revisions, read, and approved the submitted version of the manuscript.
Funding
Support for this work was provided by National Science Foundation Grants OPP-1708322 to ML, OPP-1708307 and OPP-2100773 to HA, and from the Colgate University Research Council to ML.
Conflict of Interest
The authors declare that the research was conducted in the absence of any commercial or financial relationships that could be construed as a potential conflict of interest.
Publisher's Note
All claims expressed in this article are solely those of the authors and do not necessarily represent those of their affiliated organizations, or those of the publisher, the editors and the reviewers. Any product that may be evaluated in this article, or claim that may be made by its manufacturer, is not guaranteed or endorsed by the publisher.
Acknowledgments
The research was conducted on the homelands of many peoples, including the Chukchi and Sakha people. We pay respect to the Chukchi and Sakha people, and endeavor to use our science as way to honor their continuing presence on the land.
References
Abaimov, A. P. (2010). “Geographical distribution and genetics of siberian larch species,” in Permafrost Ecosystems: Siberian Larch Forests, eds A. Osawa, O. A. Zyryanova, Y. Matsuura, T. Kajimoto, and R. W. Wein (Netherlands: Springer), 41–58.
Abaimov, A. P., Lesinski, J. A., Martinsson, O., and Milyutin, L. I. (1998). Variability and Ecology of Siberian Larch Species, 126.
Alexander, H. D., and Mack, M. C. (2016). A canopy shift in interior alaskan boreal forests: consequences for above- and belowground carbon and nitrogen pools during post-fire succession. Ecosystems 19, 98–114. doi: 10.1007/s10021-015-9920-7
Alexander, H. D., Mack, M. C., Goetz, S., Loranty, M. M., Beck, P. S. A., Earl, K., et al. (2012). Carbon accumulation patterns during post-fire succession in cajander larch (Larix cajanderi) forests of Siberia. Ecosystems 15, 1065–1082. doi: 10.1007/s10021-012-9567-6
Alexander, H. D., Natali, S. M., Loranty, M. M., Ludwig, S. M., Spektor, V. V., Davydov, S., et al. (2018). Impacts of increased soil burn severity on larch forest regeneration on permafrost soils of far northeastern Siberia. For. Ecol. Manage. 417, 144–153. doi: 10.1016/j.foreco.2018.03.008
Amiro, B. D., Orchansky, A. L., Barr, A. G., Black, T. A., Chambers, S. D., Chapin, I. I. I., et al. (2006). The effect of post-fire stand age on the boreal forest energy balance. Agricult. For. Meteorol. 140, 41–50. doi: 10.1016/j.agrformet.2006.02.014
Anisimov, O., and Zimov, S. (2020). Thawing permafrost and methane emission in Siberia: synthesis of observations, reanalysis, and predictive modeling. Ambio 20:1392. doi: 10.1007/s13280-020-01392-y
Beck, P. S. A., and Goetz, S. J. (2011). Satellite observations of high northern latitude vegetation productivity changes between 1982 and 2008: Ecological variability and regional differences. Environ. Res. Lett. 6:045501. doi: 10.1088/1748-9326/6/4/045501
Beck, P. S. A., Goetz, S. J., Mack, M. C., Alexander, H. D., Jin, Y., Randerson, J. T., et al. (2011). The impacts and implications of an intensifying fire regime on Alaskan boreal forest composition and albedo: FIRE REGIME EFFECTS ON BOREAL FORESTS. Glob. Chang. Biol. 17, 2853–2866. doi: 10.1111/j.1365-2486.2011.02412.x
Berner, L. T., Beck, P. S. A., Loranty, M. M., Alexander, H. D., Mack, M. C., and Goetz, S. J. (2012). Cajander larch (Larix cajanderi) biomass distribution, fire regime and post-fire recovery in northeastern Siberia. Biogeosciences 9, 3943–3959. doi: 10.5194/bg-9-3943-2012
Blok, D., Heijmans, M. M. P. D., Schaepman-Strub, G., Kononov, A. V., Maximov, T. C., and Berendse, F. (2010). Shrub expansion may reduce summer permafrost thaw in Siberian tundra. Glob. Chang. Biol. 16, 1296–1305. doi: 10.1111/j.1365-2486.2009.02110.x
Boike, J., Grau, T., Heim, B., Günther, F., Langer, M., Muster, S., et al. (2016). Satellite-derived changes in the permafrost landscape of central Yakutia, 2000–2011: Wetting, drying, and fires. Glob. Planet. Change 139, 116–127. doi: 10.1016/j.gloplacha.2016.01.001
Bonan, G. B., Pollard, D., and Thompson, S. L. (1992). Effects of boreal forest vegetation on global climate. Nature 359, 716–718. doi: 10.1038/359716a0
Bormann, K. J., Brown, R. D., Derksen, C., and Painter, T. H. (2018). Estimating snow-cover trends from space. Nat. Clim. Chang. 8, 924–928. doi: 10.1038/s41558-018-0318-3
Bulygina, O. N., Razuvaev, V. N., and Korshunova, N. N. (2009). Changes in snow cover over Northern Eurasia in the last few decades. Environ. Res. Lett. 4:045026. doi: 10.1088/1748-9326/4/4/045026
Cai, W. H., Liu, Z., Yang, Y. Z., and Yang, J. (2018). Does environment filtering or seed limitation determine post-fire forest recovery patterns in boreal larch forests? Front. Plant Sci. 9:1318. doi: 10.3389/fpls.2018.01318
Cai, W. H., Yang, J., Liu, Z., Hu, Y., and Weisberg, P. J. (2013). Post-fire tree recruitment of a boreal larch forest in Northeast China. For. Ecol. Manage. 307, 20–29. doi: 10.1016/j.foreco.2013.06.056
Chapin, F. S. (2005). Role of land-surface changes in arctic summer warming. Science 310, 657–660. doi: 10.1126/science.1117368
Chen, D., Loboda, T. V., He, T., Zhang, Y., and Liang, S. (2018). Strong cooling induced by stand-replacing fires through albedo in Siberian larch forests. Sci. Rep. 8:4821. doi: 10.1038/s41598-018-23253-1
Chu, T., Guo, X., and Takeda, K. (2017). Effects of burn severity and environmental conditions on post-fire regeneration in Siberian larch forest. Forests 8:76. doi: 10.3390/f8030076
Crate, S., Ulrich, M., Habeck, J. O., Desyatkin, A. R., Desyatkin, R. V., Fedorov, A. N., et al. (2017). Permafrost livelihoods: A transdisciplinary review and analysis of thermokarst-based systems of indigenous land use. Anthropocene 18, 89–104. doi: 10.1016/j.ancene.2017.06.001
Essery, R., and Pomeroy, J. (2004). Vegetation and topographic control of wind-blown snow distributions in distributed and aggregated simulations for an arctic tundra basin. J. Hydrometeorol. 5:11. doi: 10.1175/1525-7541(2004)005<0735:VATCOW>2.0.CO;2
Euskirchen, E. S., Bennett, A. P., Breen, A. L., Genet, H., Lindgren, M. A., Kurkowski, T. A., et al. (2016). Consequences of changes in vegetation and snow cover for climate feedbacks in Alaska and northwest Canada. Environ. Res. Lett. 11:105003. doi: 10.1088/1748-9326/11/10/105003
Fedorov, R. (2019). Cryogenic resources: ice, snow, and permafrost in traditional subsistence systems in Russia. Resources 8:17. doi: 10.3390/resources8010017
Fisher, J. P., Estop-Aragonés, C., Thierry, A., Charman, D. J., Wolfe, S. A., Hartley, I. P., et al. (2016). The influence of vegetation and soil characteristics on active-layer thickness of permafrost soils in boreal forest. Glob. Chang. Biol. 22, 3127–3140. doi: 10.1111/gcb.13248
Flanner, M. G., Shell, K. M., Barlage, M., Perovich, D. K., and Tschudi, M. A. (2011). Radiative forcing and albedo feedback from the Northern Hemisphere cryosphere between 1979 and 2008. Nat. Geosci. 4, 151–155. doi: 10.1038/ngeo1062
Forbes, B. C., Fauria, M. M., and Zetterberg, P. (2010). Russian Arctic warming and ‘greening' are closely tracked by tundra shrub willows. Glob. Chang. Biol. 16, 1542–1554. doi: 10.1111/j.1365-2486.2009.02047.x
Frost, G. V., and Epstein, H. E. (2014). Tall shrub and tree expansion in Siberian tundra ecotones since the 1960s. Glob. Chang. Biol. 20, 1264–1277. doi: 10.1111/gcb.12406
Grippa, M., Mognard, N., Le Toan, T., and Josberger, E. G. (2004). Siberia snow depth climatology derived from SSM/I data using a combined dynamic and static algorithm. Remote Sens. Environ. 93, 30–41. doi: 10.1016/j.rse.2004.06.012
Grosse, G. (2013). Distribution of Late Pleistocene Ice-Rich Syngenetic Permafrost of the Yedoma Suite in East and Central Siberia, Russia (USGS Open-File Report No. 2013–1078), 1–37. Available online at: https://pubs.usgs.gov/of/2013/1078/8
Heslop, J. K., Walter Anthony, K. M., Winkel, M., Sepulveda-Jauregui, A., Martinez-Cruz, K., Bondurant, A., et al. (2020). A synthesis of methane dynamics in thermokarst lake environments. Earth-Sci. Rev. 210:103365. doi: 10.1016/j.earscirev.2020.103365
Holloway, J. E., Lewkowicz, A. G., Douglas, T. A., Li, X., Turetsky, M. R., Baltzer, J. L., et al. (2020). Impact of wildfire on permafrost landscapes: a review of recent advances and future prospects. Permafrost Periglacial Processes 31, 371–382. doi: 10.1002/ppp.2048
Iijima, Y., Fedorov, A. N., Park, H., Suzuki, K., Yabuki, H., Maximov, T. C., et al. (2010). Abrupt increases in soil temperatures following increased precipitation in a permafrost region, central Lena River basin, Russia. Permafrost Periglacial Processes 21, 30–41. doi: 10.1002/ppp.662
Johnstone, J. F., Hollingsworth, T. N., Chapin, F. S., and Mack, M. C. (2010). Changes in fire regime break the legacy lock on successional trajectories in Alaskan boreal forest. Glob. Chang. Biol. 16, 1281–1295. doi: 10.1111/j.1365-2486.2009.02051.x
Jones, B. M., Grosse, G., Arp, C. D., Miller, E., Liu, L., Hayes, D. J., et al. (2015). Recent Arctic tundra fire initiates widespread thermokarst development. Sci. Rep. 5:15865. doi: 10.1038/srep15865
Jorgenson, M. T. J. T., Romanovsky, V. R., Harden, J. H., Shur, Y. S., O'Donnell, J. O., Schuur, E. A. G. S. A. G., et al. (2010). Resilience and vulnerability of permafrost to climate changethis article is one of a selection of papers from the dynamics of change in Alaska's Boreal forests: resilience and vulnerability in response to climate warming. Canad. J. For. Res. 10:60. doi: 10.1139/X10-060
Karlsson, J. M., Lyon, S. W., and Destouni, G. (2012). Thermokarst lake, hydrological flow and water balance indicators of permafrost change in Western Siberia. J. Hydrol. 12, 459–466. doi: 10.1016/j.jhydrol.2012.07.037
Kharuk, V. I., Ponomarev, E. I., Ivanova, G. A., Dvinskaya, M. L., Coogan, S. C. P., and Flannigan, M. D. (2021). Wildfires in the Siberian taiga. Ambio 20:1490. doi: 10.1007/s13280-020-01490-x
Kharuk, V. I., Ranson, K. J., Dvinskaya, M. L., and Im, S. T. (2011). Wildfires in northern Siberian larch dominated communities. Environ. Res. Lett. 6:045208. doi: 10.1088/1748-9326/6/4/045208
Kropp, H., and Loranty, M. (2018). Siberian boreal forest energy balance (ViPER (Vegetation Impacts on Permafrost) project), Cherskiy, Sakha Republic, Russia, 2016-−2017 [Text/xml]. Arctic Data Center.
Kropp, H., Loranty, M. M., Natali, S. M., Kholodov, A. L., Alexander, H. D., Zimov, N. S., et al. (2019). Tree density influences ecohydrological drivers of plant–water relations in a larch boreal forest in Siberia. Ecohydrology 12:e2132. doi: 10.1002/eco.2132
Kropp, H., Loranty, M. M., Natali, S. M., Kholodov, A. L., Rocha, A. V., Myers-Smith, I., et al. (2020). Shallow soils are warmer under trees and tall shrubs across Arctic and Boreal ecosystems. Environ. Res. Lett. 16:015001. doi: 10.1088/1748-9326/abc994
Lawrence, D. M., and Slater, A. G. (2010). The contribution of snow condition trends to future ground climate. Clim. Dynam. 34, 969–981. doi: 10.1007/s00382-009-0537-4
Loranty, M., and Alexander, H. (2021). Understory micrometorology across a larch forest density gradient in northeastern Siberia 2014-2020. Arctic Data Center.
Loranty, M. M., Abbott, B. W., Blok, D., Douglas, T. A., Epstein, H. E., Forbes, B. C., et al. (2018). Reviews and syntheses: Changing ecosystem influences on soil thermal regimes in northern high-latitude permafrost regions. Biogeosciences 15, 5287–5313. doi: 10.5194/bg-15-5287-2018
Loranty, M. M., Berner, L. T., Goetz, S. J., Jin, Y., and Randerson, J. T. (2014). Vegetation controls on northern high latitude snow-albedo feedback: observations and CMIP5 model simulations. Glob. Chang. Biol. 20, 594–606. doi: 10.1111/gcb.12391
Loranty, M. M., Goetz, S. J., and Beck, P. S. A. (2011). Tundra vegetation effects on pan-Arctic albedo. Environ. Res. Lett. 6:024014. doi: 10.1088/1748-9326/6/2/024014
Loranty, M. M., Lieberman-Cribbin, W., Berner, L. T., Natali, S. M., Goetz, S. J., Alexander, H. D., et al. (2016). Spatial variation in vegetation productivity trends, fire disturbance, and soil carbon across arctic-boreal permafrost ecosystems. Environ. Res. Lett. 11:095008. doi: 10.1088/1748-9326/11/9/095008
Mankin, J. S., and Diffenbaugh, N. S. (2015). Influence of temperature and precipitation variability on near-term snow trends. Clim. Dynam. 45, 1099–1116. doi: 10.1007/s00382-014-2357-4
Marsh, P., Bartlett, P., MacKay, M., Pohl, S., and Lantz, T. (2010). Snowmelt energetics at a shrub tundra site in the western Canadian Arctic. Hydrol. Process. 24, 3603–3620. doi: 10.1002/hyp.7786
Meredith, M., Sommerkorn, M., Cassotta, S., Derksen, C., Ekaykin, A., Hollowed, A., Kofinas, A., et al. (2019). “Polar regions,” in IPCC Special Report on the Ocean and Cryosphere in a Changing Climate. 1–12.
Metcalfe, D. B., Hermans, T. D. G., Ahlstrand, J., Becker, M., Berggren, M., Björk, R. G., et al. (2018). Patchy field sampling biases understanding of climate change impacts across the Arctic. Nat. Ecol. Evol. 2, 1443–1448. doi: 10.1038/s41559-018-0612-5
Mortimer, C., Mudryk, L., Derksen, C., Luojus, K., Brown, R., Kelly, R., et al. (2020). Evaluation of long-term Northern Hemisphere snow water equivalent products. Cryosphere 14, 1579–1594. doi: 10.5194/tc-14-1579-2020
Mudryk, L. R., Chereque, A., Brown, R., Derksen, C., Luojus, K., and Decharme, B. (2020). Arctic Report Card 2020: Terrestrial Snow Cover.
Mudryk, L. R., Kushner, P. J., Derksen, C., and Thackeray, C. (2017). Snow cover response to temperature in observational and climate model ensembles. Geophys. Res. Lett. 44, 919–926. doi: 10.1002/2016GL071789
Myers-Smith, I. H., and Hik, D. S. (2013). Shrub canopies influence soil temperatures but not nutrient dynamics: An experimental test of tundra snow–shrub interactions. Ecol. Evol. 3, 3683–3700. doi: 10.1002/ece3.710
Natali, S. M., Schuur, E. A. G., Webb, E. E., Pries, C. E. H., and Crummer, K. G. (2014). Permafrost degradation stimulates carbon loss from experimentally warmed tundra. Ecology 95, 602–608. doi: 10.1890/13-0602.1
Nitzbon, J., Westermann, S., Langer, M., Martin, L. C. P., Strauss, J., Laboor, S., et al. (2020). Fast response of cold ice-rich permafrost in northeast Siberia to a warming climate. Nat. Commun. 11:2201. doi: 10.1038/s41467-020-15725-8
Nitze, I., Grosse, G., Jones, B. M., Arp, C. D., Ulrich, M., Fedorov, A., et al. (2017). Landsat-based trend analysis of lake dynamics across Northern Permafrost Regions. Rem. Sens. 9:640. doi: 10.3390/rs9070640
Nitze, I., Grosse, G., Jones, B. M., Romanovsky, V. E., and Boike, J. (2018). Remote sensing quantifies widespread abundance of permafrost region disturbances across the Arctic and Subarctic. Nat. Commun. 9:5423. doi: 10.1038/s41467-018-07663-3
Olefeldt, D., Goswami, S., Grosse, G., Hayes, D., Hugelius, G., Kuhry, P., et al. (2016). Circumpolar distribution and carbon storage of thermokarst landscapes. Nat. Commun. 7:13043. doi: 10.1038/ncomms13043
Park, H., Fedorov, A. N., Zheleznyak, M. N., Konstantinov, P. Y., and Walsh, J. E. (2015). Effect of snow cover on pan-Arctic permafrost thermal regimes. Clim. Dynam. 44, 2873–2895. doi: 10.1007/s00382-014-2356-5
Paulson, A. K. III., Alexander, H. D., Davydov, S. P., Loranty, M. M., Mack, M. C., and Natali, S. M. (2021). Understory plant diversity and composition across a postfire tree density gradient in a Siberian Arctic boreal forest. Canad. J. For. Res. 20:483. doi: 10.1139/cjfr-2020-0483
Pomeroy, J. W., Bewley, D. S., Essery, R. L. H., Hedstrom, N. R., Link, T., Granger, R. J., et al. (2006). Shrub tundra snowmelt. Hydrol. Process. 20, 923–941. doi: 10.1002/hyp.6124
Ponomarev, E. I., Kharuk, V. I., and Ranson, K. J. (2016). Wildfires dynamics in Siberian larch forests. Forests 7:125. doi: 10.3390/f7060125
Potter, S., Solvik, K., Erb, A., Goetz, S. J., Johnstone, J. F., Mack, M. C., et al. (2020). Climate change decreases the cooling effect from postfire albedo in boreal North America. Glob. Chang. Biol. 26, 1592–1607. doi: 10.1111/gcb.14888
Räisänen J. (2008). Warmer climate: less or more snow? Clim. Dynam. 30, 307–319. doi: 10.1007/s00382-007-0289-y
Randerson, J. T., Liu, H., Flanner, M. G., Chambers, S. D., Jin, Y., Hess, P. G., et al. (2006). The impact of boreal forest fire on climate warming. Science 314, 1130–1132. doi: 10.1126/science.1132075
Ranson, K. J., Montesano, P. M., and Nelson, R. (2011). Object-based mapping of the circumpolar taiga–tundra ecotone with MODIS tree cover. Remote Sens. Environ. 115, 3670–3680. doi: 10.1016/j.rse.2011.09.006
Schuur, E., McGuire, A. D., Schädel, C., Grosse, G., Harden, J. W., et al. (2015). Climate change and the permafrost carbon feedback. Nature 520, 171–179. doi: 10.1038/nature14338
Shevtsova, I., Heim, B., Kruse, S., Schröder, J., Troeva, E. I., Pestryakova, L. A., et al. (2020). Strong shrub expansion in tundra-taiga, tree infilling in taiga and stable tundra in central Chukotka (north-eastern Siberia) between 2000 and 2017. Environ. Res. Lett. 15:085006. doi: 10.1088/1748-9326/ab9059
Shevtsova, I., Herzschuh, U., Heim, B., Schulte, L., Stünzi, S., Pestryakova, L. A., et al. (2021). Recent above-ground biomass changes in central Chukotka (Russian Far East) using field sampling and Landsat satellite data. Biogeosciences 18, 3343–3366. doi: 10.5194/bg-18-3343-2021
Shirota, T., Saito, H., Maximov, T. C., Isaev, A. P., and Takahashi, K. (2006). “Safe sites of larch seedlings in the lightly burnt forest in Eastern Siberia,” in Symptom of Environmental Change in Siberian Permafrost Region (Hokkaido: Hokkaido University Press), 159–162.
Shur, Y. L., and Jorgenson, M. T. (2007). Patterns of permafrost formation and degradation in relation to climate and ecosystems. Permafrost Periglacial Processes 18, 7–19. doi: 10.1002/ppp.582
Shvidenko, A. Z., Shchepashchenko, D. G., Vaganov, E. A., Sukhinin, A. I., Maksyutov, S.h., et al. (2011). Impact of wildfire in Russia between 1998–2010 on ecosystems and the global carbon budget. Doklady Earth Sci. 441, 1678–1682. doi: 10.1134/S1028334X11120075
Sizov, O., Ezhova, E., Tsymbarovich, P., Soromotin, A., Prihod'ko, N., Petäj,ä, T., et al. (2021). Fire and vegetation dynamics in northwest Siberia during the last 60 years based on high-resolution remote sensing. Biogeosciences 18, 207–228. doi: 10.5194/bg-18-207-2021
Sofronov, M. A., and Volokitina, A. V. (2010). “Wildfire ecology in continuous permafrost zone,” in Permafrost Ecosystems: Siberian Larch Forests, eds A. Osawa, O. A. Zyryanova, Y. Matsuura, T. Kajimoto, and R. W. Wein (Netherlands: Springer), 59–82.
Soja, A. J., Cofer, W. R., Shugart, H. H., Sukhinin, A. I., Stackhouse, P. W., McRae, D. J., et al. (2004). Estimating fire emissions and disparities in boreal Siberia (1998–2002). J. Geophysic. Res. Atmosph. 109:D14. doi: 10.1029/2004JD004570
Soja, A. J., Tchebakova, N. M., French, N. H. F., Flannigan, M. D., Shugart, H. H., Stocks, B. J., et al. (2007). Climate-induced boreal forest change: predictions versus current observations. Glob. Planet. Change 56, 274–296. doi: 10.1016/j.gloplacha.2006.07.028
Stieglitz, M., Déry, S. J., Romanovsky, V. E., and Osterkamp, T. E. (2003). The role of snow cover in the warming of arctic permafrost. Geophys. Res. Lett. 30:13. doi: 10.1029/2003GL017337
Strauss, J., Schirrmeister, L., Grosse, G., Fortier, D., Hugelius, G., Knoblauch, C., et al. (2017). Deep Yedoma permafrost: a synthesis of depositional characteristics and carbon vulnerability. Earth-Sci. Rev. 172, 75–86. doi: 10.1016/j.earscirev.2017.07.007
Stuenzi, S. M., Boike, J., Cable, W., Herzschuh, U., Kruse, S., Pestryakova, L. A., et al. (2021). Variability of the surface energy balance in permafrost-underlain boreal forest. Biogeosciences 18, 343–365. doi: 10.5194/bg-18-343-2021
Stuenzi, S. M., and Schaepman-Strub, G. (2020). Vegetation trajectories and shortwave radiative forcing following boreal forest disturbance in Eastern Siberia. J. Geophysic. Res. Biogeosci. 125:e2019JG005395. doi: 10.1029/2019JG005395
Sturm, M., Douglas, T., Racine, C., and Liston, G. E. (2005). Changing snow and shrub conditions affect albedo with global implications. J. Geophysic. Res. Biogeosci. 110:G1. doi: 10.1029/2005JG000013
Sturm, M., Holmgren, J., McFadden, J. P., Liston, G. E., Chapin, F. S., and Racine, C. H. (2001). Snow–shrub interactions in arctic tundra: a hypothesis with climatic implications. J. Clim. 14, 336–344. doi: 10.1175/1520-0442(2001)014andlt;0336:SSIIATandgt;2.0.CO;2
Sulla-Menashe, D., Friedl, M. A., Krankina, O. N., Baccini, A., Woodcock, C. E., Sibley, A., et al. (2011). Hierarchical mapping of Northern Eurasian land cover using MODIS data. Remote Sens. Environ. 115, 392–403. doi: 10.1016/j.rse.2010.09.010
Sweet, S. K., Gough, L., Griffin, K. L., and Boelman, N. T. (2014). Tall deciduous shrubs offset delayed start of growing season through rapid leaf development in the alaskan arctic tundra. Arct. Antarct. Alp. Res. 46, 682–697. doi: 10.1657/1938-4246-46.3.682
Talucci, A. C., Forbath, E., Kropp, H., Alexander, H. D., DeMarco, J., Paulson, A. K., et al. (2020). Evaluating post-fire vegetation recovery in Cajander Larch Forests in Northeastern Siberia Using UAV Derived Vegetation Indices. Rem. Sens. 12:2970. doi: 10.3390/rs12182970
Talucci, A. C., Loranty, M. M., and Alexander, H. (2021). Fire perimeters for eastern Siberia taiga and tundra from 2001–2020. Arctic Data Center.
Tape, K., Sturm, M., and Racine, C. (2006). The evidence for shrub expansion in Northern Alaska and the Pan-Arctic. Glob. Chang. Biol. 12, 686–702. doi: 10.1111/j.1365-2486.2006.01128.x
Thackeray, C. W., Derksen, C., Fletcher, C. G., and Hall, A. (2019). Snow and climate: feedbacks, drivers, and indices of change. Curr. Clim. Change Rep. 5, 322–333. doi: 10.1007/s40641-019-00143-w
Todt, M., Rutter, N., Fletcher, C. G., Wake, L. M., Bartlett, P. A., Jonas, T., et al. (2018). Simulation of longwave enhancement in boreal and montane forests. J. Geophysic. Res. Atmosph. 123, 731–747. doi: 10.1029/2018JD028719
Vey, S., Steffen, H., Müller, J., and Boike, J. (2013). Inter-annual water mass variations from GRACE in central Siberia. J. Geodesy 87, 287–299. doi: 10.1007/s00190-012-0597-9
Walker, D. A., Raynolds, M. K., Daniëls, F. J. A., Einarsson, E., Elvebakk, A., Gould, W. A., et al. (2005). The Circumpolar Arctic vegetation map. J. Vegetat. Sci. 16, 267–282. doi: 10.1111/j.1654-1103.2005.tb02365.x
Walker, M. D., Walker, D. A., Welker, J. M., Arft, A. M., Bardsley, T., Brooks, P. D., et al. (1999). Long-term experimental manipulation of winter snow regime and summer temperature in arctic and alpine tundra. Hydrol. Processes 13, 2315–2330. doi: 10.1002/(SICI)1099-1085(199910)13:14/15andlt;2315::AID-HYP888andgt;3.0.CO;2-A
Walker, X., Alexander, H. D., Berner, L., Boyd, M. A., Loranty, M. M., Natali, S., et al. (2021). Positive response of tree productivity to warming is reversed by increased tree density at the Arctic tundra-taiga ecotone. Canad. J. For. Res. 2020:0466. doi: 10.1139/cjfr-2020-0466
Wang, P., Huang, Q., Pozdniakov, S. P., Liu, S., Ma, N., Wang, T., et al. (2021). Potential role of permafrost thaw on increasing Siberian river discharge. Environ. Res. Lett. 16:034046. doi: 10.1088/1748-9326/abe326
Way, R. G., and Lapalme, C. M. (2021). Does tall vegetation warm or cool the ground surface? constraining the ground thermal impacts of upright vegetation in northern environments. Environ. Res. Lett. 16:054077. doi: 10.1088/1748-9326/abef31
Webb, E. E., Heard, K., Natali, S. M., Bunn, A. G., Alexander, H. D., Berner, L. T., et al. (2017). Variability in above- and belowground carbon stocks in a Siberian larch watershed. Biogeosciences 14, 4279–4294. doi: 10.5194/bg-14-4279-2017
Webb, E. E., Loranty, M. M., and Lichstein, J. W. (2021). Surface water, vegetation, and fire as drivers of the terrestrial Arctic-boreal albedo feedback. Environ. Res. Lett. 16:084046. doi: 10.1088/1748-9326/ac14ea
Webb, E. E., Schuur, E. A. G., Natali, S. M., Oken, K. L., Bracho, R., Krapek, J. P., et al. (2016). Increased wintertime CO2 loss as a result of sustained tundra warming. J. Geophysic. Res. Biogeosci. 121, 249–265. doi: 10.1002/2014JG002795
Yanagiya, K., and Furuya, M. (2020). Post-wildfire surface deformation near batagay, eastern siberia, detected by L-band and C-band InSAR. J. Geophysic. Res. Earth Surface 125:e2019JF005473. doi: 10.1029/2019JF005473
Keywords: Siberia, larch, permafrost, wildfire, snow—vegetation interactions, ecosystems, arctic and boreal ecosystems, Arctic
Citation: Loranty MM, Alexander HD, Kropp H, Talucci AC and Webb EE (2021) Siberian Ecosystems as Drivers of Cryospheric Climate Feedbacks in the Terrestrial Arctic. Front. Clim. 3:730943. doi: 10.3389/fclim.2021.730943
Received: 25 June 2021; Accepted: 06 October 2021;
Published: 04 November 2021.
Edited by:
Chris Derksen, Environment and Climate Change, CanadaReviewed by:
Chad Thackeray, University of California, Los Angeles, United StatesBernardo Teufel, McGill University, Canada
Copyright © 2021 Loranty, Alexander, Kropp, Talucci and Webb. This is an open-access article distributed under the terms of the Creative Commons Attribution License (CC BY). The use, distribution or reproduction in other forums is permitted, provided the original author(s) and the copyright owner(s) are credited and that the original publication in this journal is cited, in accordance with accepted academic practice. No use, distribution or reproduction is permitted which does not comply with these terms.
*Correspondence: Michael M. Loranty, mloranty@colgate.edu