- 1Department of Environmental Politics, Helmholtz Centre for Environmental Research – UFZ, Leipzig, Germany
- 2Department of Bioenergy, Helmholtz Centre for Environmental Research – UFZ, Leipzig, Germany
- 3Department of Economics, Helmholtz Centre for Environmental Research – UFZ, Leipzig, Germany
- 4Department of Environmental and Planning Law, Helmholtz Centre for Environmental Research – UFZ, Leipzig, Germany
- 5Biogeochemical Modelling, GEOMAR Helmholtz Centre for Ocean Research Kiel, Kiel, Germany
- 6Marine Evolutionary Ecology, GEOMAR Helmholtz Centre for Ocean Research Kiel, Kiel, Germany
- 7Deutsches Biomasseforschungszentrum gGmbH (DBFZ), Leipzig, Germany
Removal of carbon dioxide from the atmosphere will be required over the next decades to achieve the Paris Agreement goal of limiting global warming to well below 2°C aiming at not exceeding 1.5°C. Technological and ecosystem-based options are considered for generating negative emissions through carbon dioxide removal (CDR) and several nations have already included these in their Long-Term Low Greenhouse Gas Emission Development Strategies. However, strategies for development, implementation, and upscaling of CDR options often remain vague. Considering the scale at which CDR deployment is envisioned in emission pathways for limiting global warming to 1.5°C, significant environmental, social, and institutional implications are to be expected and need to be included in national feasibility assessments of CDR options. Following a multi-disciplinary and comprehensive approach, we created a framework that considers the environmental, technological, economic, social, institutional, and systemic implications of upscaling CDR options. We propose the framework as a tool to help guide decision-relevant feasibility assessments of CDR options, as well as identify challenges and opportunities within the national context. As such, the framework can serve as a means to inform and support decision makers and stakeholders in the iterative science-policy process of determining the role of CDR options in national strategies of achieving net-zero carbon emissions.
Introduction
All pathways for achieving the Paris Agreement (UNFCCC, 2015) goal of limiting the increase in global temperature to “well below 2°C” with aspirations to not exceed global warming by 1.5°C require carbon dioxide removal (CDR) from the atmosphere (IPCC, 2018). Accordingly, the Long-Term Low Greenhouse Gas Emission Development Strategies (LT-LEDS) proposed by national governments include CDR options (Thoni et al., 2020; Buylova et al., 2021). Hence, determining the feasibility of deployment of the envisioned CDR options within their respective national context is critical. Most LT-LEDS focus on strengthening natural carbon sinks including established strategies for tackling climate change such as afforestation/reforestation, wetland restoration and conservation, and options for the enhancement of soil carbon (Thoni et al., 2020). Few countries and the EU also propose the deployment of bioenergy with carbon capture and storage (BECCS) and direct air capture with carbon capture and storage (DACCS) (Schenuit et al., 2021).
CDR options differ considerably in terms of their technological maturity, carbon removal potentials, costs, co-benefits, risks, and trade-offs (IPCC, 2018; Minx et al., 2018; Schenuit et al., 2021). For example, afforestation/reforestation is not new, and thus it has a high readiness level. However, on the ground experience is mainly related to generating and accounting for mitigation benefits but not for negative emissions specifically and not at scale (Carton et al., 2020; Waller et al., 2020). The same goes for international and national climate policy, where enhancement of natural sinks has been discussed since the inception of the UNFCCC and widely included in national strategies, but the idea of generating net-negative emissions has only recently begun to appear in national strategies (Carton et al., 2020; Thoni et al., 2020). Other CDR options include technical approaches for capturing carbon dioxide (CO2) directly from the atmosphere, which are in different development stages and in some cases tested in pilot phase (e.g., Dittmeyer et al., 2019). Each of the considered CDR options require resources, for example, ecosystem-based CDR options require land and water (Heck et al., 2016, 2018; Brack and King, 2021) and technical options require the supply of renewable energy (Dittmeyer et al., 2019; Beerling et al., 2020).
CDR assessments have addressed critical technological, economic and environmental aspects related to CDR implementation (e.g., Fuss et al., 2018; Dooley et al., 2020). Also approaches for assessing the effectiveness, efficiency, scale, risk, and synergies of CDR options have been proposed and require to be tailored to the specific context (Fridahl et al., 2020). Hence it is pivotal to get a better understanding of the implications and feasibility of a large-scale deployment of diverse CDR options within the national context and as part of long-term strategies for achieving net-zero carbon emissions (Thoni et al., 2020; Schenuit et al., 2021). Significant challenges for the feasibility of CDR options are to be expected in particular if CDR were to be deployed at a scale required for achieving international climate targets (IPCC, 2018). Among others, the deployment and upscaling of CDR options can create a competition for resources such as land, water, and renewable energy, posing trade-offs with a variety of societal goals related to sustainable development (e.g., food security, biodiversity conservation, and renewable energy supply) (e.g., Dooley et al., 2018; Dittmeyer et al., 2019; Brack and King, 2021). While the scientific literature addresses selected questions about environmental, technological and economic feasibility of CDR options (e.g., Nemet et al., 2018; Fajardy et al., 2019; Dooley et al., 2020), aspects related to societal and institutional feasibility of the deployment and upscaling of CDR options are underrepresented (Thoni et al., 2020; Schenuit et al., 2021). For example, understanding societal aspects such as public acceptance are critical for the feasibility of deployment of CDR options, their design, and the upscaling of CDR options and related policies (Braun et al., 2018; Cox et al., 2020, 2021). This has also been shown, for example, by the public debates on the controversies related to the deployment of windmills, nuclear energy, energy crop production, and carbon capture and storage (CCS) pilot scale deployments (Lock et al., 2014; Dauber and Miyake, 2016; Jami and Walsh, 2017; Janhunen et al., 2018; Gough and Mander, 2019).
CDR deployment has to happen at multiple levels of governance and eventually has to take place at local scale within the institutional setting of federal states and municipalities involving public and private actors, land and infrastructure (Schenuit et al., 2021). There is the need for comprehensive feasibility assessments that consider the social and institutional realities in the specific context in which CDR options potentially have to operate (Thoni et al., 2020; Schenuit et al., 2021). Honegger et al. (2021b) provides an assessment of possible synergies and trade-offs of CDR deployment with the sustainable development goals (SDGs). There is also the need to translate such assessments to the national and sub-national context (e.g., by focusing on indicators of national relevance). This is in particular important for supporting participatory processes, including public and private stakeholder engagement, in identifying, developing and deploying CDR options, which are required to ensure that stakeholder perspectives are adequately taken into account (cf. Winickoff and Mondou, 2017; Bellamy et al., 2021).
Participatory processes are also considered to be key to ensure public acceptance (Dütschke, 2011; Honegger et al., 2021a), and strategies of co-producing the design and deployment of CDR options important for adequate consideration of sustainable development broadly (Dooley et al., 2018). At an international scale, more comprehensive assessment frameworks for a scientific assessment of CDR options have been proposed (e.g., Dooley et al., 2020; Forster et al., 2020, etc.). However, policies and strategies for CDR remain very broad with significant knowledge gaps when it comes to implementation (Thoni et al., 2020; Schenuit et al., 2021). There is the need to bridge this gap between science and policy and provide tools that can inform participatory science-policy processes.
For example, Germany's national long-term climate strategy lays out possible CDR options, but vaguely discusses the feasibility of these options, and the challenges and opportunities they pose to the nation. The plan also acknowledges that there are industrial and agricultural emissions that are unavoidable (e.g., emissions related to cement and steel production or agricultural activities), which will require the implementation of technological CDR options to compensate for these emissions (BMUB, 2016). There is a heavy emphasis in the plan on ecosystem-based CDR options with a focus on enhancing carbon sinks through sustainable forest management, the use of wood as construction material, as well as the conservation of grassland and peatlands (BMUB, 2016). However, it acknowledges that ecosystems not only serve as carbon sinks but also offer other societal benefits and possible trade-offs are mentioned (e.g., land competition, impacts on biodiversity and ecosystem services). However, it remains unclear how these trade-offs should be addressed.
There is the need for approaches that can help to elicit and synthesize knowledge on CDR options in a transparent, comprehensive, and inclusive manner in order to support participatory and deliberative processes for defining the governance of CDR within the national context (Borth and Nicholson, 2021). Lessons learned from the assessment of bioenergy options in Germany suggest that using indicators tailored to specific decision-making processes can enable co-design in collaboration with key stakeholder groups (e.g., Thrän et al., 2020). Herein we propose a comprehensive framework as a tool to assess the feasibility of deploying CDR options in order to support and inform science-policy processes on CDR deployment within the national and local contexts of Germany. We consider a CDR option to be feasible if key indicators related to implementation are deemed to pose no or few hurdles (see Sections Environmental dimension, Technological dimension, Economic dimension, Social dimension, Institutional dimension, and System utility on key assessment dimensions and Section Traffic light system on the traffic light system for assessing if an indicator is likely to pose a hurdle to implementation). The framework is not intended for the purpose of assessing whether or not different CDR options are desirable mitigation options or for enhancing their acceptance. That being said, desirability and feasibility often overlap. For instance, for a CDR option to be seen as politically and socially acceptable it needs to be deemed desirable and not encounter too much opposition. Hence we use a conditional understanding of feasibility: if hurdles to implementation of key indicators are considered to be low then the CDR option is likely to be more feasible. In our assessment framework, indicators important for feasibility are defined based on recent literature and expert elicitation involving an iterative peer review (see also Singh et al., 2020).
Objectives of the proposed assessment framework for CDR options:
• Provide a comprehensive framework to assess the feasibility of CDR options including challenges and opportunities along six dimensions: environmental, technological, economic, social, institutional, and systemic dimensions;
• Identify co-benefits and trade-offs involved in the implementation of CDR options, as well as interlinkages across the assessment dimensions;
• Provide a flexible tool that will support inclusive, participatory, adaptive and iterative science-policy processes on the design, implementation and upscaling of CDR options in Germany;
Materials and Methods
The comprehensive framework presented herein to assess the feasibility of CDR options in Germany is based on recent literature and expert elicitation (Singh et al., 2020) involving experts of the Helmholtz Climate Initiative (https://www.helmholtz-klima.de/en/about-us). The initiative brought together expertise on CDR options including biomass production for bioenergy (BE), BE combined with carbon capture and storage (BECCS), direct air carbon capture and storage (DACCS), and anthropogenic actions for enhancing natural carbon sinks (nature-based solutions) (Figure 1). There is ongoing research on these CDR options and nature-based solutions are already being considered within Germany's long-term climate strategy (BMUB, 2016; Thoni et al., 2020). Besides involving experts on the technical aspects of each CDR option, experts from the social sciences with expertise in laws and regulations, stakeholder participation and science-policy processes were part of this multidisciplinary effort to ensure that the framework would include the most relevant aspects for assessing the feasibility of available CDR options (see Supplementary Material 1 for more information on the experts involved).
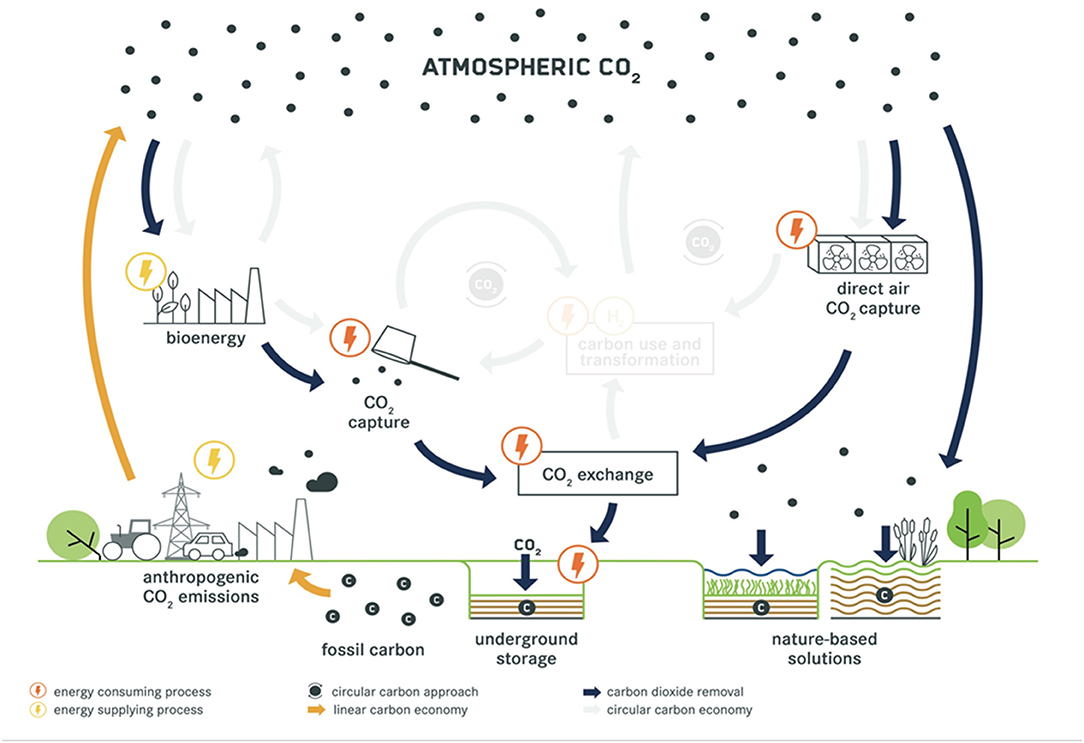
Figure 1. Illustration of the historical burning of fossil carbon (orange arrows), and novel approaches allowing for a more circular carbon economy (light blue arrows, blurred) and carbon dioxide removal (dark blue arrows). Carbon dioxide removal includes point capture at the source of carbon dioxide from bioenergy production, direct air capture and nature-based solutions. Circular carbon approaches have been faded as the framework presented herein focuses on assessing options for carbon dioxide removal (CDR) (source: Helmholtz Climate Initiative // Tanja Hildebrandt, Creative Commons CC-BY NC 4.0 license).
For determining the thematic dimensions relevant for the feasibility assessment of CDR options, the feasibility assessment of mitigation options designed by the IPCC Special Report on Global Warming of 1.5°C (Table 4.10 in de Coninck et al., 2018a) served as a starting point. This was complemented by dimensions identified for assessing the feasibility of bioenergy strategies in Germany (Thrän et al., 2020). Because no CDR option has yet been deployed at a large scale, the construction of the assessment framework was also informed by literature from other fields, such as new technologies and large infrastructure projects (e.g., wind energy, fossil CCS, and nuclear energy) (Lock et al., 2014; L'Orange Seigo et al., 2014; Jami and Walsh, 2017; Winickoff and Mondou, 2017; Janhunen et al., 2018), as well as by expert consultations.
As our assessment framework focuses on Germany, we aimed to include indicators that are relevant at the national level, which might not be applicable and too fine grained for a global assessment. We were therefore drawing on insights from assessments of national Long-Term Low Greenhouse Gas Emission Development Strategies (Thoni et al., 2020), previous CDR assessment studies (Fuss et al., 2018), assessments of economic barriers to the deployment of new technologies (Agora Verkehrswende, 2020a,b) and assessments related to the bioenergy system in Germany (Thrän et al., 2020). Where possible, indicators with relevance to the German national level were selected, for example, German-based environmental impact assessments (UBA, 2020a).
The dimensions of the assessment framework were thus adjusted to include criteria and indicators that address information needs for national-scale decision-making. Criteria and indicators already used within established planning and assessment processes (e.g., regulatory impact assessments) were preferred in order to ensure useful information transfer to decision makers in Germany (Fridahl et al., 2020; Thrän et al., 2020; see also Table 4.10 in de Coninck et al., 2018a).
Following the approach used by Thrän et al. (2020), a traffic light system was introduced for each indicator in order to evaluate whether or not it would likely pose a hurdle to a CDR option. We chose a traffic light system mainly for communication purposes. In general, red refers to an indicator that is likely to pose a large hurdle to implementation, while green poses no hurdle (see also similar approaches used by e.g. Boehm et al., 2021 and Climate Action Tracker, 2021). In addition, the color code of the traffic light system of each indicator is complemented with a description of the ranking and thus the system is not only dependent on this red/green color coding.
To ensure the plausibility of the selected dimensions, criteria, indicators and the definition of the traffic light system, we organized an internal review process involving 32 interdisciplinary experts of the Helmholtz Climate Initiative (further information on the review process is provided in the Supplementary Material 1). During a workshop the assessment framework, the selection of criteria, indicators and the respective traffic light system were reviewed by groups of experts. These involved experts with knowledge on the technological and biophysical processes involved in the aforementioned CDR options (see Figure 1), experts with knowledge on and experiences with stakeholder participation and science-policy processes, and experts on the laws and regulations related to climate policies in Germany. Overall, the review process included experts from a broad range of disciplines including environmental science (including agricultural science, climate modeling, climate physics, ecology, geology, meteorology and physics), social science (including economics, political science, and law), engineering as well as business and management, geography, resource management, infrastructure planning, and sustainability studies.
Results
The developed framework (Table 1) includes key dimensions for assessing the feasibility of climate change mitigation options proposed by the IPCC (de Coninck et al., 2018b) (Figure 2), complemented with criteria and indicators as described in the section above. The complete framework for assessing the feasibility of CDR options with references for the selected criteria and indicators is included in Supplementary Material 2.
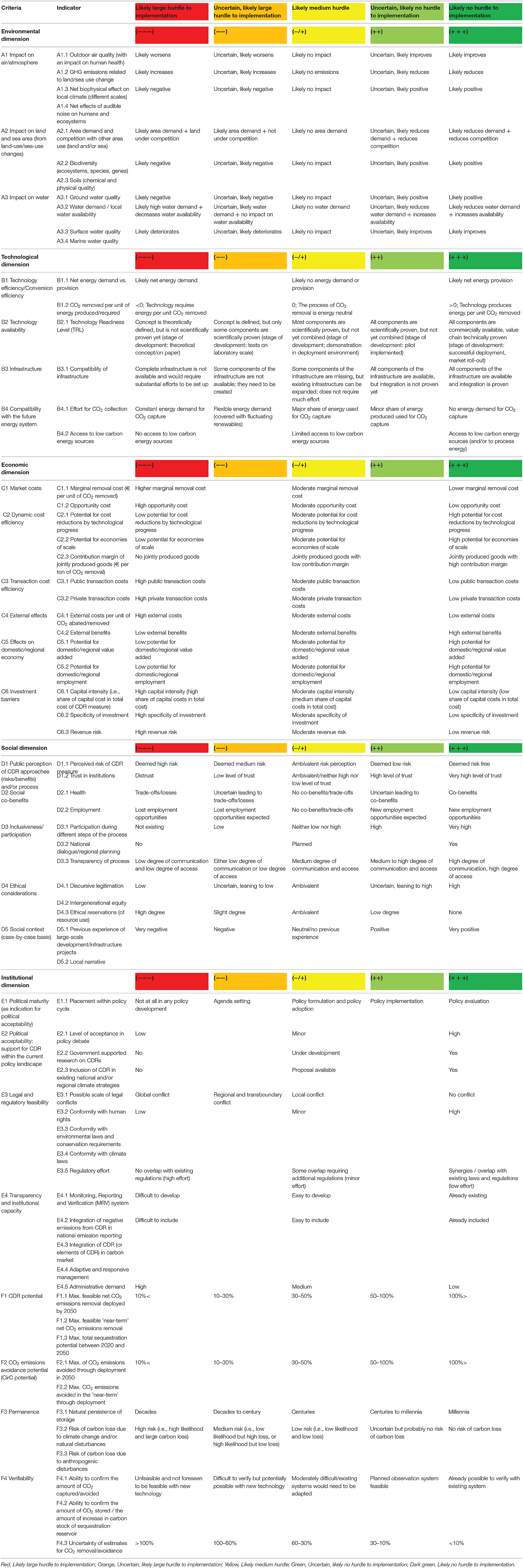
Table 1. Overview of criteria and indicators included in the assessment framework, including the traffic light system.
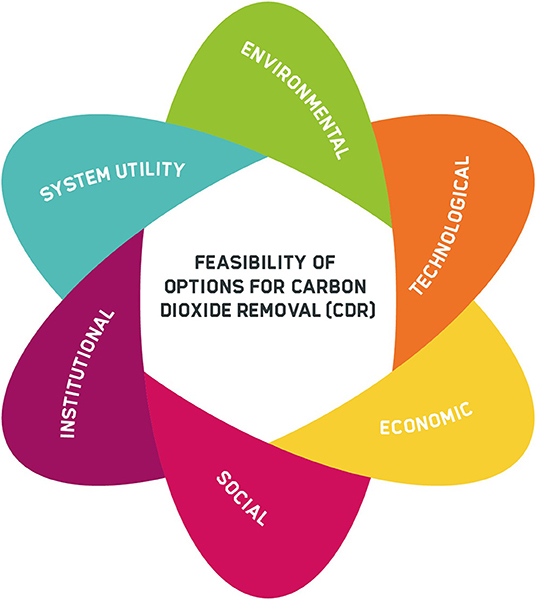
Figure 2. Overview of thematic dimensions included in the feasibility assessment framework of carbon dioxide removal (CDR) options (source: UFZ/Conor Ó Beoláin, Helmholtz Climate Initiative // Julia Blenn, Creative Commons CC-BY NC 4.0 license).
The framework aims to support actors and decision makers working at the science-policy interface (e.g., actors from scientific organizations and government agencies) by providing (a) guidance on relevant criteria and indicators to be considered and included when addressing the feasibility of CDR options and (b) a traffic light ranking system for assessing whether or not the topics addressed by the criteria and indicators could pose a hurdle to CDR implementation. The developed framework can be regarded as a tool for better understanding and navigating the feasibility of CDR options. Furthermore, the assessment framework can be adapted and complemented with indicators in accordance with user needs such as including more fine-grained indicators and data if needed. The assessment framework presented herein may serve as a starting point to then be adapted to meet the needs of particular stakeholders and national circumstances. For example, besides applying the framework to assess the feasibility of CDR options at a national scale, the criteria and indicators can be adapted to assess pilot-sized projects. Thereby, the focus of the assessment framework can also be adapted to the specific local context depending on the respective social-ecological, economic, legal and political situation and the information needed by stakeholders (Fridahl et al., 2020). A case specific adaptation of the assessment framework can address particular information needs of stakeholders and provide more relevant information for decision-making processes to make CDR assessments fit-for-purpose.
Environmental Dimension
The potential impacts of deploying a CDR option on air/atmosphere, land and sea, and freshwater bodies is explored in the environmental dimension. The selection of environmental criteria and related indicators is partly based on established environmental impact assessments used in Germany (UBA, 2020a).
Impact on the air/atmosphere (A1) includes an air quality indicator that considers air changes that affect human health, GHG emissions related to land/sea use changes, net biophysical effects on local climate, and net effects of audible noise on humans and ecosystems. For the air quality indicator, any impacts that have implications for human health should be considered, including changes in ground level ozone, particle pollution (also known as particulate matter), sulfur dioxide and nitrogen dioxide. GHG emissions related to changes in land/sea use indicates whether the CDR measure is likely to cause or avoid any non-CO2 GHG emissions, including methane, nitrous oxide, or fluorinated GHGs. Changes in the use of land and sea can cause emissions of a range of GHGs, for comparability the indicator refers to CO2 equivalents using global warming potential over 100 years (GWP100). Due to time lags in the impact of CDR measures on GHG emissions (e.g., forest, seagrass or peatland restoration can take decades before reaching their full potential in GHG uptake) this indicator considers GHG effects starting from implementation until 2050. Net biophysical effects indicate impacts of CDR implementation on local climate conditions due to changes in albedo, water and heat fluxes caused by e.g., land cover changes. The indicator on noise effects is referring to any impacts on humans and ecosystems from audible noise caused by the CDR option.
Impacts on land and sea (A2) include indicators for assessing area demand resulting from CDR implementation and related competition, impacts on biodiversity (ecosystems, species, genes), and impacts on soil quality. While the IPCC (de Coninck et al., 2018a) includes land use under the geophysical dimension, we follow Thrän et al. (2020) and include it under the environmental dimension as land/sea use has implications for multiple environmental impact categories. Area demand assesses whether the implementation of the CDR measure requires land/sea area and if the area is under competition for alternative uses (e.g., food production, conservation, reforestation). This includes indirect land use impacts, with area demand being shifted to other regions (teleconnections), which requires also to account for related indirect emissions (Fridahl et al., 2020). Most area demand caused by CDR options in Germany is likely to increase land competition. However, some CDR options can reduce competition by introducing multi-purpose use of the affected area (e.g., paludiculture on rewetted peatlands and restored seagrass meadows can allow alternative use of the same area). The biodiversity indicator refers to expected changes in ecosystems (genes to species) as a result of the implementation of a CDR option. Impacts on biodiversity can be assessed by e.g., changes in species richness of an ecosystem (alpha diversity), changes in species turnover (beta diversity) or mean species abundance. We suggest using a biotope valuation point system established for Germany that allows assessing habitat quality related to biodiversity and which is also applied for determining restoration costs (Schweppe-Kraft et al., 2020). Impacts on soil quality include changes in chemical and physical soil characteristics including nutrients (e.g., nitrate or phosphate), heavy metal concentrations, soil erosion and compaction/consolidation.
Assessing impacts on water (A3) includes indicators on the quality of groundwater, surface water, and sea water, as well as indicators on water demand and consequences for its availability. In general, the indicators follow the EU Water Framework Directive and the EU Groundwater Directive (EC, 2000, 2006). Indicators on quality of surface water and groundwater consider impacts on chemical composition, such as nutrient content (in particular nitrate and phosphate), pesticides and heavy metal concentrations (Geidel et al., 2021).
Technological Dimension
Technological performance is the key to deploying and upscaling CDR options, as well as determining CDR efficiency (e.g., see also Fridahl et al., 2020). This performance is evaluated under the technical dimension. It accounts for efficiency (i.e., energetic and CO2 removal capability), market maturity, infrastructural requirements and integration into the future energy system. The criteria and indicators have been adapted based on the integrated assessment framework of bioenergy strategies by Thrän et al. (2020) and contribute to operationalizing indicators on efficiency and scale (e.g., proposed by Fridahl et al., 2020) within the national context of Germany.
Technology efficiency (B1) addresses two aspects: (1) the net energy balance of the CDR option, which can either be positive (net energy provision), neutral or negative (net energy demand); and (2) the CO2 reduction and removal efficiency per energy unit used or produced, which describes how energy intensive a CO2 removal process is and hence the leverage potential for negative emission generation. Availability of the technology (B2) is used to assess the extent to which a technology is commercially available on the market. It is described by the technology readiness level (TRL) in terms of its stage of implementation (laboratory, demonstration, pilot, market rollout) (DOE, 2011; as defined by EC in HORIZON 2020 Work Programme). Another relevant aspect for the feasibility of a CDR option is the compatibility of the already existing infrastructure with that needed to implement the CDR option (B3), such as availability of plants/installations (e.g., pipelines for natural gas, hydrogen, or CO2 transportation) or the operation of ecosystem-based options (e.g., materials and machinery for habitat restoration, ease of access for harvesting biomass). This criterion specifically addresses whether a suitable infrastructure already exists, or if it has to be created before implementing the CDR option. Finally, the compatibility with the future energy system (B4) is assessed, which includes the operation effort of the CO2 collection and also the possibility to access low carbon energy carriers on different stages of the option's life cycle (Fajardy et al., 2018).
Economic Dimension
The economic criteria focus on costs related to the deployment of CDR measures, effects of CDR deployment on the domestic economy, as well as on potential investment barriers that might hamper the deployment of CDR measures.
The criterion market costs (C1) analyzes the private cost for CO2 removal from the atmosphere (Minx et al., 2018). A first indicator are today's marginal removal cost, i. e. the cost that needs to be spent in order to remove one additional metric ton of CO2 from the atmosphere under this CDR option. All else being equal, a higher marginal removal cost means a higher cost for reaching a given CO2 removal target and hence a lower cost-effectiveness from a static point of view as also stressed by Fridahl et al. (2020). Additionally, a second indicator analyzes the opportunity cost of applying a CDR measure, meaning that it looks at forgone and/or restricted other economic uses of the deployed production factors, e.g., land use (United Nations Environment Programme, 2020). As Fridahl et al. (2020) point out, CDR options, particularly land based ones, often compete with alternative uses, and thus can come along with considerable opportunity cost.
However, the cost of a CDR option might change over time and thus can alter the relative cost-effectiveness when compared with other CDR options. This is mirrored in the criterion dynamic cost efficiency (C2) which aims to assess the potential for future cost discrepancies (e.g., cost reductions) and depends on various influencing factors. This criterion provides an indication for future subsidy needs (Yao et al., 2020). A first indicator for this criterion is the potential for future cost reductions due to technological advancements of a CDR option. Another source for future cost reductions is a potential decrease of the average production cost per unit of CDR when the overall production of the respective CDR measure is increased (potential for economies of scale). However, average production cost can also increase, e.g., if increasing the scale means higher opportunity costs of land use. A third indicator is the contribution margin of marketable, jointly produced goods produced by a CDR option which allows for reducing the cost for the CDR service. The contribution margin describes the share that the revenue of a certain product contributes to the coverage of the fixed costs. By covering a part of the fixed costs, the contribution margin of jointly produced goods thus reduces the (fixed) costs that need to be refinanced by the CDR service.
Like all environmental policy instruments the deployment of CDR options do not only come along with production costs, but also generates transaction costs (C3), i.e., costs that accrue for transactions that are related to the deployment of a CDR measure, such as for using the market, for insuring against risks, or for regulating the deployment of a CDR option (Krutilla, 2011). High transaction costs can decrease the relative economic efficiency of CDR measures, e.g., if a large number of actors is involved in transactions or permanent and long-lasting regulatory control of retention of removed CDR is necessary. Transaction costs accrue both on the side of regulators as well as on the side of private actors who apply CDR measures. On the side of public actors transaction costs encompass for instance the costs of legislative procedures or the enforcement of laws and regulations. On the private side transaction costs can accrue due to, for example, the compliance with laws and regulations or the usage of the market (e.g., finding transaction partners, settlement of trade disputes, etc.). Both higher public and private transaction costs increase the cost of deploying the respective CDR option.
External effects (C4) of CDR options describe negative effects or benefits to third party actors who did not choose to incur that damage or benefit and that accrue due to the deployment of the respective measure. Classic examples include environmental damages or health impacts. External effects constitute a major economic cost and play an important role for the economic assessment of a CDR option (Fuss et al., 2018).
Besides economic efficiency reasons, policy decisions for or against the deployment of a certain technology are often also based on economic policy, which is considered in the effects on domestic/regional economy (C5) criterion. In this regard, effects on domestic value added and employment are often analyzed in multicriteria assessments of technology options (cf. Thrän et al., 2020) as well as in the context of CDR options in particular (Honegger et al., 2021a).
In a sixth criterion, the economic dimension examines investment barriers (C6) as these might impede the implementation of (potentially cost-effective) CDR options. This criterion encompasses three indicators. The first indicator looks at the capital intensity of CDR options, i.e., the share of capital cost in the total cost of the CDR option which can differ significantly between different CDR options (Fridahl et al., 2020). If capital markets are imperfect, e.g., due to incomplete information, a high share of capital intensity can restrain investments in the respective measure (cf. Hu et al., 2018). A second indicator is the specificity of investment, asking for other potential uses of the investment than the envisaged one. If other applications for the investment are absent or significantly reduce its value then the investment is highly specific and means a high financial loss to the investor in case of failure, i.e., the CDR investment might end up as a stranded asset (Minx et al., 2018). Accordingly, a highly specific investment bears a high risk and hence might discourage investors from investing. A third indicator is the revenue risk of a CDR investment (cf. Hu et al., 2018). This indicator considers the risk that revenues fail to accrue once the investment is made. For instance, some CDR measures may rely greatly on regulated revenues or state subsidies, which are subject to the discretion of political actors, while others have to cover their costs by market revenues (Fridahl et al., 2020). A higher revenue risk indicates a larger investment barrier. However, it is important to consider interactions between these indicators because, for instance, a highly specific investment might be less of an investment barrier if the revenue risk is low.
Social Dimension
The social dimension focuses on understanding social acceptance in a specific setting, informed by the following criteria: public perception of CDR options, social co-benefits or costs, inclusiveness and participation, ethical considerations, and social context. In addition to consideration of previous (global) assessments, as discussed above, the selection of the criteria for the social dimension were informed by literature on social acceptance, while recognizing that complex social processes are not easily captured by fixed criteria and indicators. For instance, while the assessment framework has been developed for the national level, the criterion ‘social context’ points to the relevance of even more fine-grained analyses (cf. Bellamy et al., 2019). As much research on CDR options focuses on modeled or projected policy outcomes (‘supply-side’ of knowledge production), another consideration going into the social dimension was to include actors' expectations projected to these emerging technologies and governance. In practice, this means that we have included a broad range of criteria and indicators, such as consideration of perceived, absolute, and/or anticipated risks and benefits, based on input from various actors.
While public perception (D1) and social acceptance are sometimes used synonymously in the literature, for the purpose of this assessment framework, we treat social acceptance as an overarching theme, for which all criteria under the social dimension are relevant. This differentiation marks an important methodological distinction, reflecting the fact that asking people what they think about CDR today is not necessarily a good indicator for how they will feel about it in the future (cf. Dowd et al., 2015; Winickoff and Mondou, 2017; Bellamy et al., 2019). Social acceptance can instead be understood as something that is built over time, associated with creating trust in the process (e.g., Mabon et al., 2013; Braun et al., 2018; Gough and Mander, 2019; Waller et al., 2020). Moreover, emerging research on social acceptance in the context of CDR options shows that rather than that the public lacks information, resistance to technology deployment often stems from basic value conflicts, distrust in authorities and institutions and perceived injustice (Winickoff and Mondou, 2017; Markusson et al., 2020; Waller et al., 2020). This research highlights the need for qualitative-procedural based research on politics—the input into the policy process—as much focus has already been given to quantitative-distributional studies on outcomes of policies. The social dimension covers both these aspects—input into the policy process through considerations of inclusiveness and participation, and output of policies through considerations of co-benefits and risks. The proposed indicators are therefore a contribution to translating more general indicators of CDR acceptance (e.g., as proposed by Fridahl et al., 2020) to more specific indicators for application within the national context of Germany.
One criterion that has been linked to positive public perception is perceived benefits. The criterion social co-benefits (D2) is thus linked to public perception. However, perceived benefits and absolute or anticipated benefits are not the same (L'Orange Seigo et al., 2014). Given that CDR is not yet implemented at large scale, anticipated social co-benefits such as positive health effects or new jobs are relevant to give an idea regarding acceptance in the future. While such social benefits are critical for determining public acceptance, it should also be noted that the selected indicators represent common discussions in the literature, and that other social co-benefits exist (e.g., enhancing climate resilience through ecosystem-based CDR options). When the assessment framework is applied, it would therefore be beneficial to consider the most important co-benefits and risks for the specific context.
The degree of public trust has been linked to inclusiveness and participation, transparency, and perceived fairness of processes, but previous research also highlights that the picture is complex and context specific (Lock et al., 2014; Jami and Walsh, 2017; Janhunen et al., 2018). Consequently, trust is here assessed as both public perception of trust in the process, as well as indirectly through assessments of inclusiveness and participation (D3) in the knowledge and decision-making process. By way of comparison, public perception is a criterion where we consider—at a given moment in time—risk perception on the one hand, and trust in the process on the other. This is also in line with literature on CCS where perceived risks/benefits and trust in stakeholders are the most common indicators for public perception (L'Orange Seigo et al., 2014).
The criterion ethical considerations (D4) goes beyond the evaluation of direct and personal benefits to include more general notions of what is regarded as right or good. For instance, perceived interference with nature has been linked to negative attitudes toward CO2-storage (Wallquist et al., 2012; Wolske et al., 2019). The criterion also includes the linkage between discursive legitimation and social acceptance (cf. Bäckstrand and Lövbrand, 2019). Research indicates that negative perceptions of fracking may conjure negative feelings toward CDR (Cox et al., 2021), and that associating CCS with bioenergy and negative emissions could positively affect attitudes toward CCS compared to when CCS is associated with the fossil fuel industry, for example (e.g., Wallquist et al., 2012; Haikola et al., 2019).
Overall, an important consideration for the choice of criteria and indicators selected for the social dimension has been to highlight that the socio-technical systems that CDR options are embedded in, and the social context in which citizens decide, matter for social acceptance. For instance, in a study about perceptions of BECCS, Bellamy et al. (2019) show that the support or resistance for BECCS cannot be well-understood merely by looking at technological characteristics. Instead, public perception is affected by the type of policy instrument (e.g., taxes, funding, standards) used to incentivize it. Social acceptance has also been linked to much more local/social context-specific factors than the more generic ones discussed above, such as previous experience with similar projects (e.g., Braun, 2017; Gough et al., 2018; Cox et al., 2021). It is also important to note that “the public” cannot be well-understood as one actor and that public reaction is context specific (Dowd et al., 2015; OECD, 2017).
Institutional Dimension
The institutional dimension evaluates the political and legal conditions for the development and deployment of CDR options. Four criteria have been selected for this purpose: political and institutional maturity, support for CDR within the current policy landscape, legal and regulatory feasibility, and transparency and institutional capacity. As highlighted by Fridahl et al. (2020), it is important to assess the juridical compatibility of CDR options within their respective political context and our proposed indicators allow for operationalizing this assessment within the German national context.
Political (and institutional) maturity (E1) can help to locate CDR approaches in the different phases of the policy cycle, ranging from agenda setting to policy evaluation. This criterion could also give an indication of political acceptance, since if a specific CDR option faces opposition, it will be less likely to advance in the policy cycle than CDR options that are widely accepted (Geden, 2016; Zelli et al., 2017; Pye et al., 2021).
The second criterion, political acceptability (E2), is the institutional and public support for the different approaches that seek to generate negative emissions within the current political landscape. It allows for the level of acceptance to be assessed in the political debate as indicated, for example, by the inclusion of CDR options in national and/or regional climate strategies (Geden and Schenuit, 2020; Thoni et al., 2020). Political acceptability can be broadly understood as support for a policy or measure (including a lack of opposition). This criterion is also closely related to the policy cycle. For example, if a specific CDR approach is a taboo topic, it means that it may not even reach the agenda setting phase and therefore not be assessed with respect to the policy cycle. To date, it is still difficult to assess the political acceptability of specific CDR approaches, because of its early stage of development and the need to anticipate governance needs and challenges.
Legal and regulatory feasibility (E3) addresses the questions whether CDR approaches will generate legal conflicts, i.e., with a view to existing laws at different levels, i.e., at the global, regional or local level and/or presents any conflicts with legal requirements (Brent et al., 2018; Geden et al., 2019; Markus et al., 2021a). Any CDR approach to be deployed must conform with international human rights instruments (e.g., regarding the restriction of free use of property rights) and various environmental laws, including general principles (e.g., prevention and precautionary principles), as well as rules set out in specific legislation (e.g., at the European level: RED II, EU-Emissions trading system) (Creutzig et al., 2013; Burns and Nicholson, 2017; Brent et al., 2018; IPCC, 2018; Markus et al., 2020, 2021b). This criterion is used to assess whether the development or deployment of the CDR approach is adequately regulated or requires the creation of a new regulation, either at the European or national level or whether it can be integrated into existing legal instruments (Hester, 2019; Honegger et al., 2019; Markus et al., 2020, 2021a).
Finally, the criterion on transparency and institutional capacity (E4) examines whether a monitoring, reporting, and verification (MRV) system is in place to evaluate the CO2 sequestered, and report on compliance with social and environmental safeguards related to CDR options (Lin, 2018; Royal Society and Royal Academy of Engineering, 2018; UBA, 2020b). To avoid introducing too much complexity, we have not included further indicators for the quality of the MRV-system. It is more of a first step, rather than a complete picture. That being said, the criterion verifiability (F4) in the system utility dimension looks at scientific and technological advancements and uncertainty ranges of measuring systems for CDR options, which in turn could inform MRV-assessments. This criterion of transparency and institutional capacity (E4) also addresses the integration of negative emissions from CDR approaches in national emission reports and the integration of CDR approaches in the carbon market (Geden and Schenuit, 2020). With regard to institutional capacity, this criterion seeks to determine whether there is adaptive and responsive management in place to evaluate and possibly adapt mechanisms and procedures in a transparent manner for the governance of the deployment of CDR technologies (Armeni and Redgewell, 2015; Forster et al., 2020. It may be concluded whether the existing capacity is sufficient or whether new institutions or new institutional arrangements are required. For example, potential administrative or institutional demands for specific CDR approaches may arise with a view to establishing and implementing permitting regimes, monitoring requirements, standards, control and enforcement schemes, as well as participation in planning measures (Lin, 2018; Hester, 2019; Markus et al., 2021a). The indicator on administrative demand addresses the effort related to building such institutional capacity and includes aspects such as costs, time, capacity building, etc.
System Utility
The system utility dimension describes the CDR option's potential for CO2 removal, which relates to the effectiveness of CDR options (e.g., addressed by Fridahl et al., 2020). Removing CO2 can have a two-fold system utility. First, removing CO2 during periods of net positive emissions can compensate for remaining gross positive emissions and therefore close the gap to net-zero CO2. And second, removing CO2 can enable net-negative CO2 emissions and therefore would enable the mitigation of a carbon budget overshoot. The CDR potential (F1) criterion describes the maximum negative emissions potential in the short and longer term (2025 and 2050), including both annual capacities and cumulative effects over time. In addition, we include the CO2 emissions avoidance potential (F2) showing possible co-benefits of additionally avoiding current emissions to the system. For example, emissions from agricultural soils can be avoided by rewetting previously drained organic soils, and in addition to that, these ecosystems will sequester carbon. We here explicitly exclude future avoided emissions (e.g., future anthropogenic disturbances of existing carbon stocks), as this assessment would require additional scenario assumptions about a future counterfactual scenario. Permanence of CO2 storage (F3) explores the risks and efforts associated with maintaining an intact carbon stock. This includes the natural persistence of the chosen storage reservoir over time scales of decades, centuries to millennia, as well as the level of risk associated with natural and anthropogenic disturbances of a carbon stock.
Finally, verifiability (F4) indicates if adequate measuring systems exist and are in place to confirm the amount of CO2 sequestered or captured and stored. This can be assessed by the scientific community, for example, by verifying the amount of carbon added to the overall carbon stock or measuring CO2 fluxes. Where possible, uncertainty estimates for CO2 removal or avoidance can be added to this criterion. The focus here is on scientific and technological certainty, whereas the MRV-indicator (E4.1) assesses whether on an institutional level MRV-systems are in place as a means for creating transparency. For instance, the measuring systems for verifying carbon dioxide removal by a CDR option with adequate accuracy might exist (F4.3), but there is no MRV-system in place (E4.1) to ensure transparent accounting of the removed carbon dioxide as part of a national accounting scheme.
Interlinkages of Dimensions
Interlinkages arise between the dimensions due to the complexity of the reality in which CDR options operate and resulting overlaps in the dimensions (Table 2). Here, we identify and discuss some of these interlinkages that consequently might cause synergies or trade-offs between objectives when CDR options are implemented or scaled up. This can provide useful information for decision-makers on the expected added value or unintended side effects from CDR implementation and identify where effort is required to harness synergies and address trade-offs.
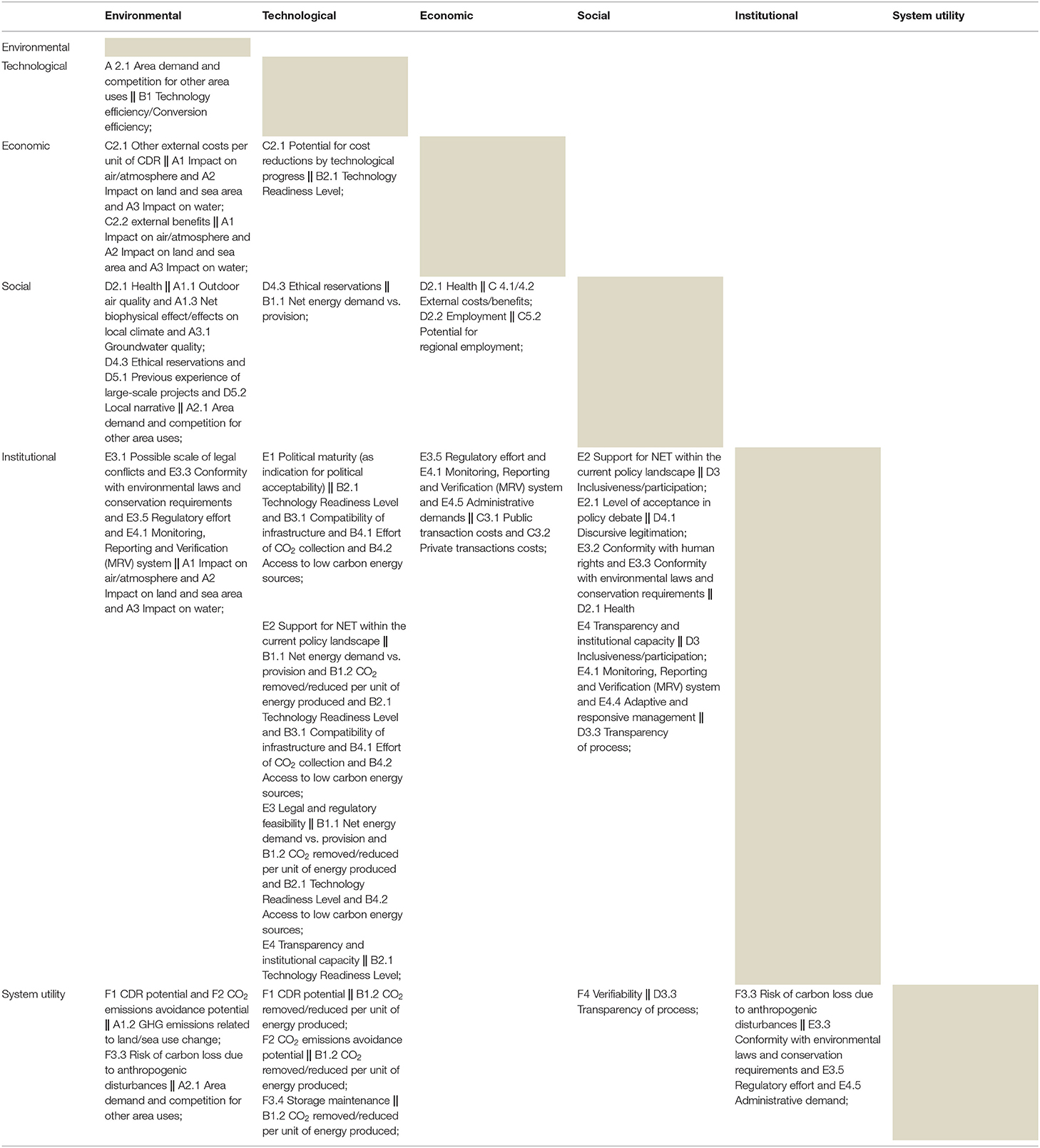
Table 2. Interlinkages between the dimensions of the feasibility assessment framework of carbon dioxide removal (CDR) options (interlinkages between dimensions are idicated by “||”.
For example, area demand (A2.1) of a CDR option, assessed within the environmental dimension, has wide ranging environmental (A1 to A3) and social (D2 and D4) implications, with positive or negative externalities accounted for in the economic dimension (C4). These environmental impacts are also subject to laws and regulations (E3) being assessed under the institutional dimension. If the implementation and upscaling of CDR options requires compliance with multiple environmental laws and regulations, then CDR deployment would likely involve a high regulatory effort (E3.5) and a need for transparency with implications for the administrative demand (E4).
There are also overlaps between the environmental and economic dimensions with regards to externalities (C4) (i.e., negative and positive impacts related to CDR deployment). For selected environmental impacts, economic cost estimates have been established for Germany, for example, to inform regulatory impact assessments (UBA, 2020a). They can be used to determine negative and positive externalities related to environmental impacts resulting from the implementation of CDR options. Cost estimates are also available for carbon emissions resulting from land-use change (based on damage cost of carbon emissions), air pollution, noise pollution, the sealing of soils with impermeable surfaces, and the release of nutrients to surface water, groundwater and coastal waters (UBA, 2020a). Where CDR implementation is having positive environmental impacts (e.g., reduce carbon emissions, enhance carbon uptake, reduce nutrients, air and noise pollution, and reverse the sealing of surfaces), the same cost estimates can be used to determine the positive externalities.
Interlinkages can reveal potential correlations between indicators as well as highlight those that bear the risk of being considered twice in the assessment. While the former might apply, for example, to the interlinkage of environmental impacts and the resulting need for environmental regulation, the latter is inherent for instance in the economic evaluation of external effects, such as environmental damages or health impacts. This is an important limitation of the assessment framework when weighing different CDR options against one another. As double consideration should be avoided in multi-criteria assessments to prevent overweighting of particular aspects, the respective feature should only be evaluated in one of the dimensions concerned.
The presented framework can provide an important tool to systematically assess interlinkages in order to better understand effort, risks, and opportunities involved in the development and potential large-scale implementation of CDR options. This analysis also helps identify possible stakeholder groups impacted by CDR implementation and related consequences that require participatory processes.
Traffic Light System
To assess the implications of CDR options via the identified indicators, a traffic light system was developed. The general idea behind the traffic light system is to provide a systematic overview of and communicate challenges and opportunities for CDR options. It also indicates where efforts are needed to overcome hurdles for the implementation and deployment of CDR options, in line with previous efforts to develop similar systems (e.g., Thrän et al., 2020). Our traffic light system combined with the proposed indicators are adapted to the German national context and the framework is, to the best of our knowledge, the only one with this scope. There are other color coded assessments in the field of CDR (e.g., de Coninck et al., 2018b; Honegger et al., 2019), and other traffic light assessment systems in the field of climate governance, for instance the Boehm et al. (2021) and Climate Action Tracker (2021).
We regard the combination of a descriptive ranking system together with a traffic light color coding system to be an effective tool to support science-policy processes on CDR development and deployment as it helps structure and evaluate the available information on the feasibility of CDR options. The ranking can serve as an input to and be informed by participatory science-policy processes involving relevant stakeholders, but it cannot replace decision making processes. The assessment is also not meant to be policy prescriptive. For example, where an indicator for a CDR option has been ranked with a red traffic light color code (which means that the issue addressed by the indicator is likely to represent a high hurdle to implementation), the assessment does not imply that this CDR option should be increased or abandoned. Such conclusions will have to be drawn within participatory stakeholder processes and under consideration of multiple decision-relevant criteria, which can be supported but not replaced by such an assessment.
The color code of the traffic light system ranges from green colors (no or little hurdle/effort for implementation) to yellow color (medium hurdle/effort for implementation) to red colors (large hurdle/effort for implementation) with five different codes for most indicators and three (green-yellow-red) for some, depending on the characteristics of the indicator.
In general, green traffic lights represent a high feasibility with no or little effort/hurdles for implementation. In other words, implementation would be possible under current conditions. In contrast, red traffic lights indicate the need for high efforts, or existence of large hurdles, for the implementation of the CDR option and/or for the prevention of side effects. Yellow traffic lights consequently indicate medium effort needed. Due to the different nature of the dimensions, the specific logic behind the traffic light system varies between indicators. More specifically, the traffic light system covers three overarching logics:
Traffic light based on advancement within a given process: Several dimensions (e.g., for E1: Political maturity) have indicators coupled with a traffic light system representing steps in a process, where green represents that the development has come a long way through the process, red that it has not even or barely started. This type of logic is obvious for the technological dimension, where the rate of technological development is assessed, for instance based on the Technology Readiness Level (TRL). Other examples include the policy process, included as an indicator under the institutional dimension.
Traffic light based on improvement or worsening of the current condition: For most indicators, current conditions (unless specified otherwise) comprise the reference system or baseline of the assessment and all indicators are assessed relative to this. For example, the environmental dimension is assessed against current environmental conditions so yellow, green, red light classifications represent no change, improving and worsening conditions, respectively. In contrast, indicators of the systemic dimension assess how much CO2 could be removed by certain CDR options along different time frames and the ranking follows order of magnitude of removal from zero/low (red) to high (green). Many indicators of the institutional dimension also use current conditions as the starting point, assessing for instance the applicability of existing regulatory frameworks and institutions. Similarly, indicators of the social dimension assess possibilities to participate in the decision-making process against current institutional settings, as well as trade-offs and synergies compared with today's world.
Traffic light based on assessment against expectations/what is deemed low vs. high effort: For some dimensions, the expected effort for deployment is not meaningfully assessed against current conditions. For instance, for the economic dimension, all interventions imply some cost. Hence, it would not be informative to apply the same logic to the economic dimension as that of the environmental one, since using current conditions as a baseline would imply that all approaches would get a red light due to associated costs. Instead, the traffic light system for the economic dimension represents a qualitative assessment based on what can be considered a high or low cost for different economic indicators. Similarly, most of the indicators of the systemic dimension have a traffic light system that corresponds to what would arguably be deemed low vs. high effort for deployment. For instance, high maintenance costs get a red light, low costs a green light, and moderate costs a yellow light. The social dimension also includes indicators that instead of current conditions need to be assessed against what is deemed acceptable or not, which in turn is subjective and depends on who is deciding what is acceptable or not. For instance, what is deemed high or low risk may differ between an expert-based impact assessment and an assessment by the people at risk of being adversely affected. Hence there is a need to contextualize the assessment and to specify the underlying assumptions including whose perspective the evaluation represents (e.g., the perspective of technical experts vs. science vs. policy vs. practice vs. civil society vs. etc.), and the degree of participation in and transparency of the assessment process.
Where applicable, quantitative values are used for defining the different colors of the traffic light system, for instance related to emissions removed or avoided in the systemic dimension. In most cases however, a qualitative approach to the traffic light system is used (cf. Thrän et al., 2020). An advantage of the traffic light system is to allow for flexibility in the use of qualitative and quantitative information as long as the underlying assumptions are transparent. What is a high cost or risk in one place can be medium cost or risk in another place, depending on circumstances. A region that wants to reach net-zero carbon emissions and has access to a range of natural carbon sinks may judge investments in DAC too costly, while regions that do not have any natural carbon sinks may find the costs of DAC reasonable. Moreover, for the application of the assessment framework, actors applying the framework to a specific case may choose to specify the traffic lights in more detail. For example, the indicator ‘transparency of process’ (D3.3) only specifies the degree of communication and stakeholder access to the process. Therefore, users applying the assessment framework to a specific case have to define what a high or low degree means for their context and purposes (e.g., a high/low degree of access means that a certain number of meetings per year are open to the public, etc.). Hence, when the assessment framework is applied to a different national or regional context, the traffic light system needs to be specified in more detail.
An alternative approach to assessing indicators against a baseline or reference is to assess CDR options against one another (comparative analysis of CDR options). For instance, for the economic dimension, a comparative analysis of CDR options would result in identifying options with lower or higher costs. However, comparing CDR options against one another only provides information about which ones require more or less effort relative to one another, not the effort needed in absolute terms. Another alternative would be to assess CDR options against a reference system (cf. Thrän et al., 2020), such as paying for CO2 removal from verified CDR services, such as the Climeworks DACCS-project on Iceland1.
However, if the reference system, as in the example given, requires only limited land, then all land-based CDR options would require more effort relative to the reference system, but we would not know the expected level of effort in absolute terms. Moreover, including a reference system outside the national context (e.g., establishing an international trading system for emission removal from CDR measures) would add another layer of uncertainty, not least given the uncertainties around international climate policies, transparency with regards to monitoring, reporting and verification (MRV) and disagreement around Article 6 of the Paris Agreement on internationally transferable mitigation outcomes. Moreover, CDR potential is limited worldwide, and not all countries that want to compensate for residual emissions will be able to do so abroad.
Discussion
Although many Long-Term Low Greenhouse Gas Emission Development Strategies (LT-LEDS) of Parties under the UNFCCC already include options for carbon dioxide removal (CDR), there are still considerable uncertainties regarding the feasibility of deploying CDR options at a large scale (IPCC, 2018). Without an understanding of the feasibility of such CDR options the inclusion of CDR options in current national strategies for reaching net-zero carbon emissions can involve the risk of misleading current climate strategies. Previous research has raised concerns about the risk of mitigation deterrence, meaning that alone the anticipation of generating future carbon removal could slow down action on climate change today (e.g., Carton, 2019; Low and Schäfer, 2020; McLaren, 2020; Waller et al., 2020). In models, the greater the contribution from CDR options is, the slower the rate of decarbonization becomes (Holz et al., 2018; Butnar et al., 2020). This in turn can make it difficult to understand the magnitude of societal transformation needed, if CDR options do not deliver as assumed in emission pathways (Larkin et al., 2018).
There is a growing literature focusing on the feasibility of deploying CDR options under current conditions instead of focusing on the theoretical, technical, maximum potential in the future (Boysen et al., 2017; Geden et al., 2018; Vaughan et al., 2018; Asayama and Hulme, 2019; Fridahl et al., 2020; Wieding et al., 2020; Brack and King, 2021). Assessments also highlight the importance of policy design for CDR in order to address potential trade-offs with other policy objectives such as achieving the Sustainable Development Goals (e.g., Honegger et al., 2021b).
Our assessment framework contributes to this effort by providing a comprehensive set of criteria to explore the feasibility of CDR options within the national context of Germany. Where possible, criteria and indicators were selected with a specific focus on Germany in order to ensure their relevance for and compatibility with established assessment and decision-making processes. This includes, for example, indicators used in national environmental and regulatory impact assessments (UBA, 2020a) and in assessments of renewable energies (Thrän et al., 2020). This contextualization of the assessment framework to the national level is a first step of adapting CDR assessments to specific decision-making contexts. We include previously underexplored criteria in particular related to the social and institutional dimensions that are relevant for assessing opportunities for and barriers to the deployment of CDR options within the current political landscape. This complements previous CDR assessments with a more global perspective (e.g., Fridahl et al., 2020; Honegger et al., 2021b) and assessments with focus on technical or environmental implications (cf. Forster et al., 2020; Waller et al., 2020). Our national level framework, adapted to Germany, can also function as a starting point for discussion of what indicators and criteria are relevant for other countries or regions.
Assessing the numerous indicators of the proposed framework poses a challenge and requires the involvement of experts and stakeholders from multiple disciplines and backgrounds related to both the thematic dimensions and CDR options. This can make the assessment process a complex and demanding task, including the challenge of identifying adequate information and data for each indicator. In order to address these challenges, the rating combined with the traffic light system facilitates the assessment of indicators in both quantitative and qualitative terms (e.g., expert judgement in case no primary or secondary data exists). While this system can support the assessment of complex information from a diversity of sources (e.g., publications, gray literature, expert evaluation), the ranking of indicators also involves the simplification of information with the risk of information loss (Barnett et al., 2008; Fridahl et al., 2020). Therefore, it is critical to ensure the transparency of underlying information and assumptions in order to gauge their validity and the uncertainties involved in the assessment outcome.
Users applying the framework need to have experience in synthesizing knowledge (e.g., working with multi-criteria analysis) and in facilitating multidisciplinary assessment processes. This can include users from academia, as well as public and private agencies working at the science-policy interface for informing the design of CDR options and policies. Given that the ranking of indicators using the traffic light system involves interpretation of the underlying information based on the judgement of experts or stakeholders from different disciplines and backgrounds, different actors may disagree in the interpretation and ranking of indicators. Therefore, to build credibility, relevance and legitimacy of assessment outcomes, it is also important to be transparent about the actors involved in the assessment process.
Experts, stakeholders, and decision makers might have different opinions on the ranking and relevance of criteria and indicators for determining the feasibility of a CDR option. For example, from a technical perspective CDR options involving CCS technology might be considered feasible by technical experts. However, from a social perspective a lack of public acceptance of CCS due to a different perception of the risks involved could potentially block the implementation of CDR options with CCS (Wallquist et al., 2012). It would arguably be easier for DAC to find public acceptance as part of industrial processes or integrated into air conditioning systems together with the recycling and use of carbon compared to implementation in large scale plants with CCS. However, high demand for renewable energy could undermine energy efficiency and thereby pose a trade-off for the feasibility of DAC technology (Dittmeyer et al., 2019). Hence the assessment framework can help to elicit such trade-offs and open up the policy debate on the feasibility CDR options.
Furthermore, some indicators can be conceptually challenging involving a simplification of the actual underlying processes. For example, determining the placement of the implementation of CDR policies within the policy cycle (E1.1) can be ambiguous as the evolution of decisions leading to policies is often complex with multiple policy processes taking place in parallel, at different policy levels, involving networks and coalitions with a diversity of interests, with policy development taking different paths over time (Wellstead et al., 2018). Hence the evolution of CDR policies is not a linear process as a placement within the policy cycle might suggest. When applying the assessment framework, it is critical to be aware of such ambiguities in order to avoid simplistic conclusions. The indicators allow structuring complex information, eliciting different perspectives and discussing ambiguities within the larger context of the multiple dimensions. Therefore, we understand the framework to be an approach for structuring complex information, bringing together different expertise, perspectives and knowledge types. This can help elicit and reduce ambiguities and thereby support an iterative science-policy process on the development and implementation of CDR.
Starting with a comprehensive assessment framework and using participatory and iterative processes can help to identify and narrow down the criteria and indicators requiring closer attention in decision making on CDR development and implementation (e.g., indicators associated with hurdles to implementation). Hence the process of conducting such multi-criteria assessment is in itself an important part of generating decision relevant information. For example, it could help to better understand which aspects are of particular relevance for stakeholder groups and how priorities differ. It would also help to elicit the underlying assumptions and information sources the different stakeholder groups use for justifying their evaluation of CDR options and assess their relevance, credibility and legitimacy. This benefit could potentially be lost when aggregating the ranking of indicators into a single index (Barnett et al., 2008; Fridahl et al., 2020).
While working with such a comprehensive assessment framework can be challenging, it can support the identification of expertise and stakeholder perspectives that need to be considered in the feasibility assessment. This helps to elicit the various perspectives of actors who are involved in or impacted by the development and implementation of CDR options, such as experts from academia, engineering and practice, as well as representatives of public and private stakeholder groups. Ensuring inclusiveness also helps build the credibility, relevance and legitimacy of the assessment process and its outcomes for informing decision making on CDR options (Sarkki et al., 2015). As the framework includes indicators on assessing the inclusiveness and transparency of processes (D3) related to CDR development and deployment, these indicators could change and potentially improve over time as a result of a participatory assessment process.
In order to avoid being overwhelmed by the complexity of information involved, the level of detail in the application of the assessment framework can be adapted to particular information needs and data availability, ranging from a coarse scoping of CDR options to more detailed assessments of selected indicators. When applying the framework to pilot projects or other national contexts, the choice of criteria and indicators can be adapted and specified further according to environmental and societal conditions and particular information needs. Depending on the information needed within a specific decision-making context, categories of the framework can be prioritized over others. For instance, some indicators could be set as a minimum requirement, and the full assessment will only be carried out if these indicators have been fulfilled (e.g., only if removal potential is deemed to be high enough). Thereby, the traffic light system can help identify trends in indicators, expected impacts and related trade-offs and synergies with particular relevance for informing decision making. However, further in-depth analysis might be required in order to enhance the accuracy and thereby the credibility of such findings for informing decision making.
Informing decision-making processes is a dynamic process, which requires different levels of detail of information at different points in time. Therefore, defining the information needed and the level of detail of the assessment should be part of an iterative science-policy process. While this can help to reduce complexity and resource needs for conducting the assessment, the framework allows for keeping track of outstanding knowledge gaps for achieving a comprehensive assessment of CDR options. Keeping track of criteria and indicators that might have been overlooked in more technical assessments can also facilitate the inclusion of more diverse stakeholder perspectives within CDR assessments, leading to a broader and more comprehensive debate on the feasibility of CDR options (e.g., stakeholder perspectives on social acceptance). As such, the framework is flexible in its application as a tool for guiding the design of comprehensive assessment processes of CDR options.
However, the assessment framework has its limitations in particular in understanding systemic implications, trade-offs and synergies between the assessed criteria, effects related to the upscaling of potential CDR options, and indirect impacts within and outside the national boundaries (e.g., so-called teleconnections). It is important to note that the assessment outcome does not prescribe conclusions for decision making on CDR policies or implementation. For example, if societal acceptance of a CDR option is low and rated as a hurdle to its implementation, the consequence of this information can include finding ways to increase its acceptance or abandoning its implementation altogether. Such conclusions need to be taken as part of an informed and inclusive decision making process, which the assessment framework can support but not replace. Ambiguities are inherent in feasibility assessments and we hope that the proposed framework can help in making the processes of navigating opportunities and challenges related to CDR options more transparent. Furthermore, the presented assessment framework is not a panacea, decision makers and actors involved in assessing the feasibility of CDR options might prefer different assessment approaches and processes. This has to be taken into account when designing CDR feasibility assessments with outcomes that ought to generate credible, relevant, and legitimate information for decision making (Sarkki et al., 2015).
Conclusions
Given the tremendous challenges involved in achieving net-zero carbon emissions at a national scale and the uncertainties prevailing in the feasibility of CDR options, it is critical to assess the challenges and opportunities of CDR options with decision-relevant indicators tailored for specific national contexts. We believe that the proposed assessment framework for CDR options can be an appropriate tool to help navigate this process. The process of adapting the assessment framework and discussing evaluations of the traffic light system in a transdisciplinary effort, together with scientific experts and stakeholders, becomes part of a participatory and iterative approach to better understand the effort involved in developing and implementing CDR options that will be required to reach the goal of the Paris Agreement.
Data Availability Statement
The original contributions presented in the study are included in the article/Supplementary Material, further inquiries can be directed to the corresponding author.
Author Contributions
SB, MB, JF, KK, TM, NM, RS, AS, TT, and DT conceived of and designed the study and reviewed the assessment framework based on an interdisciplinary expert workshop. SB, MB, JF, EG, KK, TM, NM, RS, AS, TT, and DT developed the dimensions of the assessment framework including criteria, indicators, and traffic light system. JF designed Table 1 and led the drafting of the article, with contributions from all authors. NM designed Table 2. AS and AO edited and revised the text. All authors contributed to the article and approved the submitted version.
Funding
The Helmholtz Climate Initiative enabled the development of this publication, which was funded by the Helmholtz Associations Initiative and Networking Fund.
Conflict of Interest
The authors declare that the research was conducted in the absence of any commercial or financial relationships that could be construed as a potential conflict of interest.
Publisher's Note
All claims expressed in this article are solely those of the authors and do not necessarily represent those of their affiliated organizations, or those of the publisher, the editors and the reviewers. Any product that may be evaluated in this article, or claim that may be made by its manufacturer, is not guaranteed or endorsed by the publisher.
Acknowledgments
We would like to thank all participants of the expert workshop for contributing to the review and improvement of the assessment framework and Conor Ó. Beoláin for contributing to the design of Figure 2. We would also like to thank the two reviewers for the constructive comments and suggestions.
Supplementary Material
The Supplementary Material for this article can be found online at: https://www.frontiersin.org/articles/10.3389/fclim.2022.758628/full#supplementary-material
Footnotes
1. ^URL: https://climeworks.com/orca
References
Agora Verkehrswende (2020a). Technologieneutralität im Kontext der Verkehrswende. Kritische Beleuchtung eines Postulats. Available online at: https://www.agora-verkehrswende.de/fileadmin/Projekte/2019/Technologieneutralitaet/33_Technologieneutralitaet_LANGFASSUNG_WEB_20-04-20.pdf (accessed August 13 2021).
Agora Verkehrswende (2020b). Technology Neutrality for Sustainable Transport. Critical Assessment of a Postulate - Summary. Available online at: https://www.agora-verkehrswende.de/fileadmin/Projekte/2019/Technologieneutralitaet/Agora-Verkehrswende_Technology-Neutrality-for-Sustainable-Transport.pdf (accessed August 13 2021).
Armeni, C., and Redgewell, C. (2015). Assessment of International Treaties Applicable, or at Least Adaptable, to Geoengineering-Related Activities through Indicators. CGG Working Paper no. 21. Available online at: http://www.jura.uni-freiburg.de/de/institute/ioeffr2/downloads/lger/armeni-redgwell-international-legal-and-regulatory-issues-of-climate-geoengineering-governance.pdf (accessed August 13, 2021).
Asayama, S., and Hulme, M. (2019). Engineering climate debt: temperature overshoot and peak-shaving as risky subprime mortgage lending. Clim.ate Policy 19, 937–946. doi: 10.1080/14693062.2019.1623165
Bäckstrand, K., and Lövbrand, E. (2019). The road to paris: contending climate governance discourses in the post-copenhagen era. J. Environ. Policy Plann. 21, 519–532. doi: 10.1080/1523908X.2016.1150777
Barnett, J., Lambert, S., and Fry, I. (2008). The hazards of indicators: insights from the environmental vulnerability index. Ann. Assoc. Am. Geogr. 98, 102–119. doi: 10.1080/00045600701734315
Beerling, D. J., Kantzas, E. P., Lomas, M. R., Wade, P., Eufrasio, R. M., Renforth, P., et al. (2020). Potential for large-scale CO2 removal via enhanced rock weathering with croplands. Nature 583, 242–248. doi: 10.1038/s41586-020-2448-9
Bellamy, R., Fridahl, M., Lezaun, J., Palmer, J., Rodriguez, E., Lefvert, A., et al. (2021). Incentivising bioenergy with carbon capture and storage (BECCS) responsibly: comparing stakeholder policy preferences in the United Kingdom and Sweden. Environ. Sci. Policy 116, 47–55. doi: 10.1016/j.envsci.2020.09.022
Bellamy, R., Lezaun, J., and Palmer, J. (2019). Perceptions of bioenergy with carbon capture and storage in different policy scenarios. Nat. Commun. 10, 743. doi: 10.1038/s41467-019-08592-5
BMUB (2016). Climate Action Plan 2050. Principles and Goals of the German Government's Climate Policy. Federal Ministry for the Environment, Nature Conservation, Building and Nuclear Safety (BMUB), 92. Available online at: https://www.bmu.de/fileadmin/Daten_BMU/Pools/Broschueren/klimaschutzplan_2050_en_bf.pdf (accessed August 13, 2021).
Boehm, S., Lebling, K., Levin, K., Fekete, H., Jaeger, J., Waite, R., et al. (2021). State of climate action 2021: systems transformations required to limit global warming to 1.5°C. WRIPUB. Washington, DC: World Resources Institute. 249. doi: 10.46830/wrirpt.21.00048
Borth, A. C., and Nicholson, S. (2021). A deliberative orientation to governing carbon dioxide removal: actionable recommendations for national-level action. Front. Clim. 3, 684209. doi: 10.3389/fclim.2021.684209
Boysen, L. R., Lucht, W., Gerten, D., Heck, V., Lenton, T. M., and Schellnhuber, H. J. (2017). The limits to global-warming mitigation by terrestrial carbon removal: the limits of terrestrial carbon removal. Earths Fut. 5, 463–474. doi: 10.1002/2016EF000469
Brack, D., and King, R. (2021). Managing land-based CDR: BECCS, forests and carbon sequestration. Glob. Policy 12, 45–56. doi: 10.1111/1758-5899.12827
Braun, C. (2017). Not in my backyard: CCS sites and public perception of CCS. Risk Anal. 37, 2264–2275. doi: 10.1111/risa.12793
Braun, C., Merk, C., Pönitzsch, G., Rehdanz, K., and Schmidt, U. (2018). Public perception of climate engineering and carbon capture and storage in Germany: survey evidence. Clim. Policy 18, 471–484. doi: 10.1080/14693062.2017.1304888
Brent, K., McGee, J., McDonald, J., and Rohling, E. J. (2018). International law poses problems for negative emissions research. Nat. Clim. Change 8, 451–453. doi: 10.1038/s41558-018-0181-2
Burns, W., and Nicholson, S. (2017). Bioenergy and carbon capture with storage (BECCS): the prospects and challenges of an emerging climate policy response. J. Environ. Stud. Sci. 7, 527–534. doi: 10.1007/s13412-017-0445-6
Butnar, I., Broad, O., Solano Rodriguez, B., and Dodds, P. E. (2020). The role of bioenergy for global deep decarbonization: CO2 removal or low-carbon energy? GCB Bioenergy 12, 198–212. doi: 10.1111/gcbb.12666
Buylova, A., Fridahl, M., Nasiritousi, N., and Reischl, G. (2021). Cancel (out) emissions? The envisaged role of carbon dioxide removal technologies in long-term national climate strategies. Front. Clim. 3, 675499. doi: 10.3389/fclim.2021.675499
Carton, W. (2019). “Fixing” climate change by mortgaging the future: negative emissions, spatiotemporal fixes, and the political economy of delay. Antipode 51, 750–769. doi: 10.1111/anti.12532
Carton, W., Asiyanbi, A., Beck, S., Buck, H. J., and Lund, J. F. (2020). Negative emissions and the long history of carbon removal. WIREs Clim. Change 11, e671. doi: 10.1002/wcc.671
Climate Action Tracker (2021). CAT Rating Methodology. Available online at: https://climateactiontracker.org/methodology/cat-rating-methodology (accessed October 28, 2021).
Cox, E., Pidgeon, N., and Spence, E. (2021). But they told us it was safe! Carbon dioxide removal, fracking, and ripple effects in risk perceptions. Risk Anal. doi: 10.1111/risa.13717. [Epub ahead of print].
Cox, E., Spence, E., and Pidgeon, N. (2020). Public perceptions of carbon dioxide removal in the United States and the United Kingdom. Nat. Clim. Change 10, 744–749. doi: 10.1038/s41558-020-0823-z
Creutzig, F., Corbera, E., Bolwig, S., and Hunsberger, C. (2013). Integrating place-specific livelihood and equity outcomes into global assessments of bioenergy deployment. Environ. Res. Lett. 8, 035047. doi: 10.1088/1748-9326/8/3/035047
Dauber, J., and Miyake, S. (2016). To integrate or to segregate food crop and energy crop cultivation at the landscape scale? Perspectives on biodiversity conservation in agriculture in Europe. Energy Sust. Soc. 6, 25. doi: 10.1186/s13705-016-0089-5
de Coninck, H., Revi, A., Babiker, M., Bertoldi, P., Buckeridge, M., Cartwright, A., et al. (2018a). “Strengthening and implementing the global response,” in Global Warming of 1.5°C. An IPCC Special Report on the Impacts of Global Warming of 1.5°C above Pre-Industrial Levels and Related Global Greenhouse Gas Emission Pathways, in the Context of Strengthening the Global Response to the Threat of Climate Change, Sustainable Development, and Efforts to Eradicate Poverty, eds V. Masson-Delmotte, P. Zhai, H. O. Pörtner, D. Roberts, J. Skea, P. R. Shukla, et al. 313–443. Available online at: https://www.ipcc.ch/sr15/chapter/chapter-4/#article-citation-chapter-4 (accessed August 13, 2021).
de Coninck, H., Revi, A., Babiker, M., Bertoldi, P., Buckeridge, M., Cartwright, A., et al. (2018b). “Strengthening and implementing the global response: supplementary material,” in Global Warming of 1.5°C. An IPCC Special Report on the Impacts of Global Warming of 1.5°C above Pre-Industrial Levels and Related Global Greenhouse Gas Emission Pathways, in the Context of Strengthening the Global Response to the Threat of Climate Change, Sustainable Development, and Efforts to Eradicate Poverty, eds V. MassonDelmotte, P. Zhai, H. O. Pörtner, D. Roberts, J. Skea, P. R. Shukla, et al. 313–443. Available online at: https://www.ipcc.ch/sr15/chapter/chapter-4/#article-citation-chapter-4 (accessed August 13, 2021).
Dittmeyer, R., Klumpp, M., Kant, P., and Ozin, G. (2019). Crowd oil not crude oil. Nat. Commun. 10, 1818. doi: 10.1038/s41467-019-09685-x
DOE (2011). Technology Readiness Assessment Guide. Washington, DC: U.S. Department of Energy. Available online at: https://www.directives.doe.gov/directives-documents/400-series/0413.3-EGuide-04 (accessed March 28, 2022).
Dooley, K., Christoff, P., and Nicholas, K. A. (2018). Co-producing climate policy and negative emissions: trade-offs for sustainable land-use. Glob. Sust. 1, e3. doi: 10.1017/sus.2018.6
Dooley, K., Harrould-Kolieb, E., and Talberg, A. (2020). Carbon-dioxide removal and biodiversity: a threat identification framework. Glob. Policy 12, 34–44. doi: 10.1111/1758-5899.12828
Dowd, A.-M., Rodriguez, M., and Jeanneret, T. (2015). Social science insights for the BioCCS industry. Energies 8, 4024–4042. doi: 10.3390/en8054024
Dütschke, E. (2011). What drives local public acceptance–Comparing two cases from Germany. Energy Proc. 4, 6234–6240. doi: 10.1016/j.egypro.2011.02.636
EC (2000). Directive 2000/60/EC of the European Parliament and of the Council establishing a framework for Community action in the field of water policy. OJ L327, 22.12.2000. Available online at: https://eur-lex.europa.eu/legal-content/en/TXT/?uri=CELEX:32000L0060 (accessed August 13, 2021).
EC (2006). Directive 2006/118/EC of the European Parliament and of the Council of 12 December 2006 on the protection of groundwater against pollution and deterioration. Available online at: https://eur-lex.europa.eu/legal-content/EN/TXT/?uri=celex%3A32006L0118 (accessed August 13, 2021).
Fajardy, M., Chiquier, S., and Mac Dowell, N. (2018). Investigating the BECCS resource nexus: delivering sustainable negative emissions. Energy Environ. Sci. 11, 3408–3430. doi: 10.1039/C8EE01676C
Fajardy, M., Patrizio, P., Daggash, H. A., and Mac Dowell, N. (2019). negative emissions: priorities for research and policy design. Front. Clim. 1, 6. doi: 10.3389/fclim.2019.00006
Forster, J., Vaughan, N. E., Gough, C., Lorenzoni, I., and Chilvers, J. (2020). Mapping feasibilities of greenhouse gas removal: key issues, gaps and opening up assessments. Glob. Environ. Change 63, 102073. doi: 10.1016/j.gloenvcha.2020.102073
Fridahl, M., Hansson, A., and Haikola, S. (2020). ‘Towards Indicators for a negative emissions climate stabilisation index: problems and prospects. Climate 8, 75. doi: 10.3390/cli8060075
Fuss, S., Lamb, W. F., Callaghan, M. W., Hilaire, J., Creutzig, F., Amann, T., et al. (2018). Negative emissions—part 2: costs, potentials and side effects. Environ. Res. Lett. 13, 063002. doi: 10.1088/1748-9326/aabf9f
Geden, O. (2016). The Paris Agreement and the inherent inconsistency of climate policymaking. WIREs Clim. Change 7, 790–797. doi: 10.1002/wcc.427
Geden, O., Peters, G. P., and Scott, V. (2019). Targeting carbon dioxide removal in the European Union. Clim. Policy 19, 487–494. doi: 10.1080/14693062.2018.1536600
Geden, O., and Schenuit, F. (2020). Unconventional Mitigation. Carbon Dioxide Removal as a New Approach in EU Climate Policy. German Institute for International and Security Affairs. SWP Research Paper 8, 1–38. Available online at: https://www.swp-berlin.org/publications/products/research_papers/2020RP08_ClimateMitigation.pdf (accessed August 13, 2021).
Geden, O., Scott, V., and Palmer, J. (2018). Integrating carbon dioxide removal into EU climate policy: prospects for a paradigm shift. WIREs Clim. Change 9, e521. doi: 10.1002/wcc.521
Geidel, T., Dworak, T., Schmidt, G., Rogger, M., Matauschek, C., Völker, J., et al. (2021). Ausgewählte Fachinformationen zur Nationalen Wasserstrategie. Abschlussbericht. Umweltbundesamt (UBA), Dessau-Roßlau. TEXTE 86/2021, 105. Available online at: https://www.umweltbundesamt.de/sites/default/files/medien/5750/publikationen/2021-06-03_texte_86-2021_fachinformation_wasserstrategie_0.pdf (accessed August 13, 2021).
Gough, C., Cunningham, R., and Mander, S. (2018). Understanding key elements in establishing a social license for CCS: an empirical approach. Int. J. Greenhouse Gas Control 68, 16–25. doi: 10.1016/j.ijggc.2017.11.003
Gough, C., and Mander, S. (2019). Beyond social acceptability: applying lessons from CCS social science to support deployment of BECCS. Curr. Sust. Renew. Energy Rep. 6, 116–123. doi: 10.1007/s40518-019-00137-0
Haikola, S., Hansson, A., and Anshelm, J. (2019). From polarization to reluctant acceptance-bioenergy with carbon capture and storage (BECCS) and the post-normalization of the climate debate. J. Integr. Environ. Sci. 16, 45–69. doi: 10.1080/1943815X.2019.1579740
Heck, V., Gerten, D., Lucht, W., and Boysen, L. R. (2016). Is extensive terrestrial carbon dioxide removal a ‘green' form of geoengineering? A global modelling study. Glob. Planet. Change 137, 123–130. doi: 10.1016/j.gloplacha.2015.12.008
Heck, V., Gerten, D., Lucht, W., and Popp, A. (2018). Biomass-based negative emissions difficult to reconcile with planetary boundaries. Nat. Clim. Change 8, 151–155. doi: 10.1038/s41558-017-0064-y
Hester, T. (2019). “Negative emissions technologies and direct air capture,” in: Legal Pathways to Deep Decarbonization in the United States, eds M. Gerrard and J. Dernbach (Washington DC: ELI Press), 749–771.
Holz, C., Siegel, L. S., Johnston, E., Jones, A. P., and Sterman, J. (2018). Ratcheting ambition to limit warming to 1.5 °C–trade-offs between emission reductions and carbon dioxide removal. Environ. Res. Lett. 13, 064028. doi: 10.1088/1748-9326/aac0c1
Honegger, M., Michaelowa, A., and Joyashree, R. (2021b). Potential implications of carbon dioxide removal for the sustainable development goals. Clim. Policy 21, 678–698. doi: 10.1080/14693062.2020.1843388
Honegger, M., Michaelowa, A., and Poralla, M. (2019). Net-zero emissions: the role of carbon dioxide removal in the paris agreement. NET-Rapido & Perspectives Climate Research, Freiburg. 42. Available online at: https://www.perspectives.cc/public/fileadmin/Publications/Situating_NETs_under_the_PA.pdf (accessed February 18, 2021).
Honegger, M., Poralla, M., Michaelowa, A., and Ahonen, H.-M. (2021a). Who is paying for carbon dioxide removal? Designing policy instruments for mobilizing negative emissions technologies. Front. Clim. 3,672996. doi: 10.3389/fclim.2021.672996
Hu, J., Harmsen, R., Crijns-Graus, W., and Worrell, E. (2018). Barriers to investment in utility-scale variable renewable electricity (VRE) generation projects. Renew. Energy 121, 730–744. doi: 10.1016/j.renene.2018.01.092
IPCC (2018). “Global warming of 1.5°C,” in An IPCC Special Report on the Impacts of Global Warming of 1.5° C Above Pre-industrial Levels and Related Global Greenhouse Gas Emission Pathways, in the Context of Strengthening the Global Response to the Threat of Climate Change, Sustainable Development, and Efforts to Eradicate Poverty, eds V. Masson-Delmotte, P. Zhai, H. O. Pörtner, D. Roberts, J. Skea, P. R. Shukla, et al. 1–562. Available online at:https://www.ipcc.ch/sr15/ (accessed August 13, 2021).
Jami, A. A., and Walsh, P. R. (2017). From consultation to collaboration: a participatory framework for positive community engagement with wind energy projects in Ontario, Canada. Energy Res. Soc. Sci. 27, 14–24. doi: 10.1016/j.erss.2017.02.007
Janhunen, S., Hujala, M., and Pätäri, S. (2018). The acceptability of wind farms: the impact of public participation. J. Environ. Policy Plann. 20, 214–235. doi: 10.1080/1523908X.2017.1398638
Krutilla, K. (2011). Transaction Costs and Environmental Policy: An Assessment Framework and Literature Review. International Review of Environmental and Resource Economics, 4(3–4), 261–354. doi: 10.1561/101.00000035
Larkin, A., Kuriakose, J., Sharmina, M., and Anderson, K. (2018). What if negative emission technologies fail at scale? Implications of the Paris Agreement for big emitting nations. Clim. Policy 18, 690–714. doi: 10.1080/14693062.2017.1346498
Lin, A. C. (2018). Carbon dioxide removal after Paris, Ecology Law Quart. 45, 533–582. doi: 10.15779/Z386M3340F
Lock, S. J., Smallman, M., Lee, M., and Rydin, Y. (2014). “Nuclear energy sounded wonderful 40 years ago”: UK citizen views on CCS. Energy Policy 66, 428–435. doi: 10.1016/j.enpol.2013.11.024
L'Orange Seigo, S., Dohle, S., and Siegrist, M. (2014). Public perception of carbon capture and storage (CCS): a review. Renew. Sust. Energy Rev. 38, 848–863. doi: 10.1016/j.rser.2014.07.017
Low, S., and Schäfer, S. (2020). Is bio-energy carbon capture and storage (BECCS) feasible? The contested authority of integrated assessment modeling. Energy Res. Soc. Sci. 60, 101326. doi: 10.1016/j.erss.2019.101326
Mabon, L., Vercelli, S., Shackley, S., Anderlucci, J., Battisti, N., Franzese, C., et al. (2013). ‘Tell me what you Think about the Geological Storage of Carbon Dioxide': towards a fuller understanding of public perceptions of CCS. Energy Proc. 37, 7444–7453. doi: 10.1016/j.egypro.2013.06.687
Markus, T., Schaller, R., Gawel, E., and Korte, K. (2021a). Negativemissionstechnologien als neues Instrument der Klimapolitik: charakterisiken und klimapolitische Hintergründe. Natur und Recht 43, 90–99. doi: 10.1007/s10357-021-3804-8
Markus, T., Schaller, R., Gawel, E., and Korte, K. (2021b). Negativemissionstechnologien und ihre Verortung im Regelsystem internationaler Klimapolitik. Natur und Recht 43, 153–158. doi: 10.1007/s10357-020-3755-5
Markus, T., Schaller, R., Korte, K., and Gawel, E. (2020). Zum regulatorischen Rahmen direkter Abscheidung von Kohlendioxid aus der Luft (Direct Air Capture - DAC). Available online at: https://www.netto-null.org/imperia/md/assets/net_zero/dokumente/2020_netto-null-2050_deliverable_m-p2.1_web.pdf (accessed August 13, 2021).
Markusson, N., Balta-Ozkan, N., Chilvers, J., Healey, P., Reiner, D., and McLaren, D. (2020). Social science sequestered. Front. Clim. 2, 2. doi: 10.3389/fclim.2020.00002
McLaren, D. (2020). Quantifying the potential scale of mitigation deterrence from greenhouse gas removal techniques. Clim. Change 162, 2411–2428. doi: 10.1007/s10584-020-02732-3
Minx, J. C., Lamb, W. F., Callaghan, M. W., Fuss, S., Hilaire, J., Creutzig, F., et al. (2018). Negative emissions—Part 1: research landscape and synthesis. Environ. Res. Lett. 13, 063001. doi: 10.1088/1748-9326/aabf9b
Nemet, G. F., Callaghan, M. W., Creutzig, F., Fuss, S., Hartmann, J., Hilaire, J., et al. (2018). Negative emissions—Part 3: innovation and upscaling. Environ. Res. Lett. 13, 063003. doi: 10.1088/1748-9326/aabff4
OECD (2017). “Public acceptance and emerging production technologies,” in The Next Production Revolution (OECD Publishing), 277–298. doi: 10.1787/9789264271036-12-en
Pye, S., Broad, O., Bataille, C., Brockway, P., Daly, H. E., Freeman, R., et al. (2021). Modelling net-zero emissions energy systems requires a change in approach. Clim. Policy 21, 222–231. doi: 10.1080/14693062.2020.1824891
Royal Society and Royal Academy of Engineering (2018). Greenhouse Gas Removal. ISBN: 978-1-78252-349-9 Available online at: https://royalsociety.org/-/media/policy/projects/greenhouse-gas-removal/royal-society-greenhouse-gas-removal-report-2018.pdf (accessed August 13, 2021).
Sarkki, S., Tinch, R., Niemelä, J., Heink, U., Waylen, K., Timaeus, J., et al. (2015). Adding ‘iterativity' to the credibility, relevance, legitimacy: a novel scheme to highlight dynamic aspects of science–policy interfaces. Environ. Sci. Policy 54, 505–512. doi: 10.1016/j.envsci.2015.02.016
Schenuit, F., Colvin, R., Fridahl, M., McMullin, B., Reisinger, A., Sanchez, D. L., et al. (2021). Carbon dioxide removal policy in the making: assessing developments in 9 OECD cases. Front. Clim. 3, 638805. doi: 10.3389/fclim.2021.638805
Schweppe-Kraft, B., Syrbe, R.-U., Meier, S., and Grunewald, K. (2020). “Datengrundlagen für einen Biodiversitätsflächenindikator auf Bundesebene,” in: Flächennutzungsmonitoring XII mit Beiträgen zum Monitoring von Ökosystemleistungen und SDGs, eds G. Meinel, U. Schumacher, M. Behnisch, and T. Krüger (Berlin: Rhombos-Verlag, [IÖR-Schriften; 78)], 191–204.
Singh, C., Ford, J., Ley, D., Bazaz, A., and Revi, A. (2020). Assessing the feasibility of adaptation options: methodological advancements and directions for climate adaptation research and practice. Climatic Change, 162, 255–77. doi: 10.1007/s10584-020-02762-x
Thoni, T., Beck, S., Borchers, M., Förster, J., Görl, K., Hahn, A., et al. (2020). Deployment of negative emissions technologies at the national level: a need for holistic feasibility assessments. Front. Clim. 2, 590305. doi: 10.3389/fclim.2020.590305
Thrän, D., Bauschmann, M., Dahmen, N., Erlach, B., Heinbach, K., Hirschl, B., et al. (2020). Bioenergy beyond the German “Energiewende”–Assessment framework for integrated bioenergy strategies. Biomass Bioenergy 142, 105769. doi: 10.1016/j.biombioe.2020.105769
UBA (2020a). Methodenkonvention 3.1 zur Ermittlung von Umweltkosten. Kostensätze. Stand 12/2020. Umweltbundesamt (UBA), Dessau-Roßlau. Available online at: https://www.umweltbundesamt.de/sites/default/files/medien/1410/publikationen/2020-12-21_methodenkonvention_3_1_kostensaetze.pdf (accessed August 13, 2021).
UBA (2020b). Submission under the United Nations Framework Convention on Climate Change and the Kyoto Protocol 2020. National Inventory Report for the German Greenhouse Gas Inventory 1990 – 2018. Available online at: https://www.umweltbundesamt.de/sites/default/files/medien/1410/publikationen/2020-04-15-climate-change_23-2020_nir_2020_en_0.pdf (accessed August 13, 2021).
UNFCCC (2015). Adoption of the Paris Agreement. FCCC/CP/2015/L.9/Rev.1. Available online at: https://unfccc.int/resource/docs/2015/cop21/eng/l09r01.pdf (accessed August 13, 2021).
United Nations Environment Programme (2020). The Economics of Nature-based Solutions: Current Status and Future Priorities. United Nations Environment Programme Nairobi. Nairobi, Kenya. Available online at: https://www.un.org/sites/un2.un.org/files/economics_of_nbs_0.pdf (accessed February 09, 2022).
Vaughan, N. E., Gough, C., Mander, S., Littleton, E. W., Welfle, A., Gernaat, D. E. H. J., et al. (2018). Evaluating the use of biomass energy with carbon capture and storage in low emission scenarios. Environ. Res. Lett.13, 044014. doi: 10.1088/1748-9326/aaaa02
Waller, L., Rayner, T., Chilvers, J., Gough, C. A., Lorenzoni, I., Jordan, A., et al. (2020). Contested framings of greenhouse gas removal and its feasibility: social and political dimensions. WIREs Clim. Change 11, e649. doi: 10.1002/wcc.649
Wallquist, L., Seigo, S. L., Visschers, V. H. M., and Siegrist, M. (2012). Public acceptance of CCS system elements: a conjoint measurement. Int. J. Greenhouse Gas Control 6, 77–83. doi: 10.1016/j.ijggc.2011.11.008
Wellstead, A., Cairney, P., and Oliver, K. (2018). Reducing ambiguity to close the science-policy gap. Policy Design Pract. 1, 115–125. doi: 10.1080/25741292.2018.1458397
Wieding, J., Stubenrauch, J., and Ekardt, F. (2020). human rights and precautionary principle: limits to geoengineering, SRM, and IPCC scenarios. Sustainability 12, 1–23. doi: 10.3390/su12218858
Winickoff, D. E., and Mondou, M. (2017). The problem of epistemic jurisdiction in global governance: the case of sustainability standards for biofuels. Soc. Stud. Sci. 47, 7–32. doi: 10.1177/0306312716667855
Wolske, K. S., Raimi, K. T., Campbell-Arvai, V., and Hart, P. S. (2019). Public support for carbon dioxide removal strategies: the role of tampering with nature perceptions. Clim. Change 152, 345–361. doi: 10.1007/s10584-019-02375-z
Yao, X., Fan, Y., Zhu, L., and Zhang, X. (2020). Optimization of dynamic incentive for the deployment of carbon dioxide removal technology: a nonlinear dynamic approach combined with real options. Energy Econ. 86, 104643. doi: 10.1016/j.eneco.2019.104643
Keywords: carbon dioxide removal (CDR), net-zero, climate change mitigation action, feasibility assessment, integrated assessment (IA) frameworks, national climate strategies
Citation: Förster J, Beck S, Borchers M, Gawel E, Korte K, Markus T, Mengis N, Oschlies A, Schaller R, Stevenson A, Thoni T and Thrän D (2022) Framework for Assessing the Feasibility of Carbon Dioxide Removal Options Within the National Context of Germany. Front. Clim. 4:758628. doi: 10.3389/fclim.2022.758628
Received: 14 August 2021; Accepted: 07 February 2022;
Published: 02 May 2022.
Edited by:
Keith Paustian, Colorado State University, United StatesReviewed by:
Matthias Honegger, Perspectives Climate Research gGmbH, GermanyMathias Fridahl, Linköping University, Sweden
Copyright © 2022 Förster, Beck, Borchers, Gawel, Korte, Markus, Mengis, Oschlies, Schaller, Stevenson, Thoni and Thrän. This is an open-access article distributed under the terms of the Creative Commons Attribution License (CC BY). The use, distribution or reproduction in other forums is permitted, provided the original author(s) and the copyright owner(s) are credited and that the original publication in this journal is cited, in accordance with accepted academic practice. No use, distribution or reproduction is permitted which does not comply with these terms.
*Correspondence: Johannes Förster, am9oYW5uZXMuZm9lcnN0ZXJAdWZ6LmRl