- 1Biology Department, Research Group Plants and Ecosystems, University of Antwerp, Wilrijk, Belgium
- 2Natural Resource Management Unit, International Institute of Tropical Agriculture (CGIAR), Nairobi, Kenya
- 3Global Ecology Unit, Center for Ecological Research and Forestry Applications, Spanish National Research Council, Autonomous University of Barcelona, Barcelona, Spain
- 4Center for Ecological Research and Forestry Applications (CREAF), Cerdanyola del Valles, Spain
- 5School of Geography and the Environment, Environmental Change Institute, University of Oxford, Oxford, United Kingdom
- 6Ecosystem Services and Management Program, International Institute for Applied Systems Analysis, Laxenburg, Austria
- 7Department of Microbiology and Ecosystem Science, Centre for Microbiology and Environmental Systems Science, University of Vienna, Vienna, Austria
- 8Institute of Biological and Environmental Sciences, University of Aberdeen, Aberdeen, United Kingdom
Carbon dioxide removal (CDR) that increases the area of forest cover or bio-energy crops inherently competes for land with crop and livestock systems, compromising food security, or will encroach natural lands, compromising biodiversity. Mass deployment of these terrestrial CDR technologies to reverse climate change therefore cannot be achieved without a substantial intensification of agricultural output, i.e., producing more food on less land. This poses a major challenge, particularly in regions where arable land is little available or severely degraded and where agriculture is crucial to sustain people's livelihoods, such as the Global South. Enhanced silicate weathering, biochar amendment, and soil carbon sequestration are CDR techniques that avoid this competition for land and may even bring about multiple co-benefits for food production. This paper elaborates on the idea to take these latter CDR technologies a step further and use them not only to drawdown CO2 from the atmosphere, but also to rebuild fertile soils (negative erosion) in areas that suffer from pervasive land degradation and have enough water available for agriculture. This way of engineering topsoil could contribute to the fight against malnutrition in areas where crop and livestock production currently is hampered by surface erosion and nutrient depletion, and thereby alleviate pressure on intact ecosystems. The thrust of this perspective is that synergistically applying multiple soil-related CDR strategies could restore previously degraded soil, allowing it to come back into food production (or become more productive), potentially alleviating pressure on intact ecosystems. In addition to removing CO2 from the atmosphere, this practice could thus contribute to reducing poverty and hunger and to protection of biodiversity.
The conflict between using land to help solve the climate crisis or to produce more food for a growing human population
Across all Shared Socioeconomic Pathways (Riahi et al., 2017), a global increase in food demand of 35–56% is projected by 2050 relative to 2010 (van Dijk et al., 2021), implying an equally large food gap between food required in 2050 and food availability under a business-as-usual scenario (Ranganathan et al., 2018). Without drastic transformations of the agriculture and food sectors (Schmidt-Traub et al., 2019), producing 60% more food would require nearly 6 Mkm2 nature or forest land to be converted into cropland or pasture, i.e., a growth of the current agricultural area (50 Mkm2; Smith, 2016) by 12%. Evidently, such a dramatic land use change would have colossal adverse effects on biodiversity, environment, and climate (Ranganathan et al., 2018).
The yield per area and total production of major staple foods are globally on a decline owing to increased temperatures (Zhao et al., 2017). Especially strong impacts of climate change are experienced across the tropical belt where extreme heatwaves occur more frequently (Mbow et al., 2019) and severe droughts are already causing water stress (Porkka et al., 2016; Spinonii et al., 2021). In addition to the risk that climate change reduces productivity per unit land area, there is also a risk that the total land area available for agriculture will decline. Almost all IPCC scenarios compliant with a maximum of 1.5°C warming, which would dampen the increase in frequency and intensity of climate extremes (Hoegh-Guldberg et al., 2018), depend on large-scale deployment of carbon dioxide removal (CDR) technologies. Applying two of the most studied land-based CDR technologies, Bio-Energy with Carbon Capture and Storage (BECCS), which supports the energy transition, and afforestation/reforestation (AR), which is beneficial for biodiversity and Earth function, however, would require an estimated 4–7 Mkm2 of land (Smith et al., 2016; Mbow et al., 2019). Reducing the current 50 Mkm2 of agricultural land by 4–7 Mkm2 (-8 to−14%) to enable large-scale BECCS and AR deployment is unacceptable given that 820 million people are still undernourished (Mbow et al., 2019); likewise expanding the current agricultural area with an extra 6 Mkm2 to close the food gap would come at too large a cost for biodiversity and environment.
Food and nutritional security are furthermore threatened by decreases of soil organic matter stocks, compromising soil nutrient retention and water holding capacity (Tan et al., 2005; Ghimire et al., 2022), that moreover causes CO2 emissions that exacerbate the climate crisis. Food security is further threatened by soil contamination (Persson et al., 2022) and soil sealing, i.e., the creation of an impermeable layer (Gardi et al., 2015). Importantly, each year erosion strips 24 billion tons of topsoil from arable land worldwide (UNCCD, 2014; FAO and ITPS, 2015), diminishing the amount of food that can be produced for many generations to come. One third of global agricultural land is already subject to human-induced land degradation (FAO, 2021), and the pace at which this takes place is rising sharply due to population growth. Soil erosion and fertility loss affect a disproportionally large area of land where climate extremes decrease yields the most, aridity increases the fastest, and majority of people's livelihoods depend on agriculture (FAO, 2021). Halting soil degradation is thus a key priority to realize the zero-hunger development goal.
Avoiding competition for land
Multiple solutions have been proposed to overcome the trade-off between using land for CDR or for agriculture, and probably all will be needed if worse climate change impacts and food shortages are to be avoided. These proposed solutions are diverse and often not mutually exclusive. One group of solutions focuses on producing more food on less land. High-yield farming of croplands, with targeted protection of biodiversity (by setting aside 20% of agricultural land as the major pathway to improving biodiversity) and spatial optimization of crop selection and fertilizer inputs to current-day climate and soil conditions, was estimated to produce similar volumes of food on 40% less land (Folberth et al., 2020). Moreover, it would reduce global irrigation needs by 20% and maintained global fertilizer applications at current-day levels (Folberth et al., 2020). In many areas where rain-fed agriculture is currently possible, droughts occur with increased frequency and intensity, so ensuring water availability will be required to reduce the risk for crop failure during droughts. Hence, improved water management at landscape scale constitutes another important component of sustainable cropland intensification that could increase food production on less land (Rockström et al., 2010).
Besides maximizing yields, another group of solutions aims at optimizing the food chain, thus reducing the amount of food required to feed the world and enabling reductions in the agricultural land area. Reducing food wastage, for example, is one of the most low-hanging fruits: currently 30–50% of yields are wasted on their way from the field to the consumer (Lundqvist et al., 2008; FAO, 2011), implying that a drastic reduction in food wastage could substantially increase food availability, without requiring additional land. Another highly effective measure to reduce the land area needed to feed the world would be a global reduction in meat consumption. Roughly 75% of agricultural land is currently used to produce meat, which covers <25% of the calories and proteins required to feed the human population. Hence, also a reduction in global meat consumption would help reduce the land area needed to realize the zero-hunger objective and render land available for CDR (IPCC, 2022). Personal preferences and systemic behavioral patterns, however, pose major barriers to dietary change and more sustainable meat consumption (O'Riordan and Stoll-Kleemann, 2015). Moreover, livestock production also forms the backbone of pastoral communities in semi-arid zones where rainfall can only support grassland ecosystems, with approximately 200 million people worldwide depending on pure grazing (Kaufmann et al., 2019).
Another way to avoid competition for land between CDR and food production is to advance CDR techniques that can be implemented on agricultural land and therefore do not compete with food production, as is the case for enhanced weathering (EW) of silicate minerals (Beerling et al., 2018; Vicca et al., 2021; Ramos et al., 2022; Swoboda et al., 2022; Vienne et al., 2022), increased soil carbon storage (SCS; Smith, 2016; Smith et al., 2016; Rumpel et al., 2018; Amelung et al., 2020), or applying biochar (BC) from excess crop residues and other renewable biomass (Biederman and Harpole, 2013; Smith, 2016; Smith et al., 2016). While the estimated global CDR potentials of these three CDRs (EW about 1, SCS and BC both about 0.7 Gt C yr−1) are lower than those of BECCS (3.3 Gt C yr−1) and AR (up to 3.3 Gt C yr−1) (Smith, 2016; Smith et al., 2016), the agricultural and environmental co-benefits of these approaches help in neutralizing diverse adverse human impacts on the planet and on food security. For example, EW helps counteracting acidification (potentially replacing lime) and restores depleted pools of base cations, silica, and -depending on the source materials- also phosphorus and micronutrients (Beerling et al., 2018). Increasing soil organic matter stocks through SCS and/or BC improves soil aggregation and porosity, which combined with minimizing soil disturbance reduces erodibility, and enhances infiltration, water holding capacity and nutrient retention (Razzaghi et al., 2020; Panagea et al., 2021). Especially in areas with highly weathered or degraded soils like the Global South, these co-benefits help increase crop yields (Jeffery et al., 2017; Beerling et al., 2018).
One option that has rarely been considered so far (but see Horton et al., 2021) is that these latter CDRs may actually be combined, not only to maximize the CDR per unit agricultural land, but even to create new, fertile topsoils in landscapes where agriculture is no longer viable due to surface erosion and nutrient depletion (Figure 1). As mentioned earlier, roughly 25% of the land suited for agriculture is in a severe state of degradation or is rapidly degrading, a trend that must be halted and if possible reversed. The idea to create new fertile land using CDRs originated from studying chronosequences of volcanic islands south of Iceland (the Vestmannaeyjar islands; Leblans et al., 2014). The silicate-rich sediments on the youngest island, now 50 years old, contained all elements needed for plant growth, except nitrogen (N), and the island has remained largely unvegetated since its birth. However, one part of the island harbored a seabird colony where guano deposition catalyzed rapid development of dense vegetation. Microbes subsequently transformed the plant necromass into soil organic matter, which increased soil water-and nutrient retention that further enhanced vegetation productivity, reaching similar productivity values as vegetation on other islands with well-developed, well-managed soils (Leblans et al., 2014, 2017).
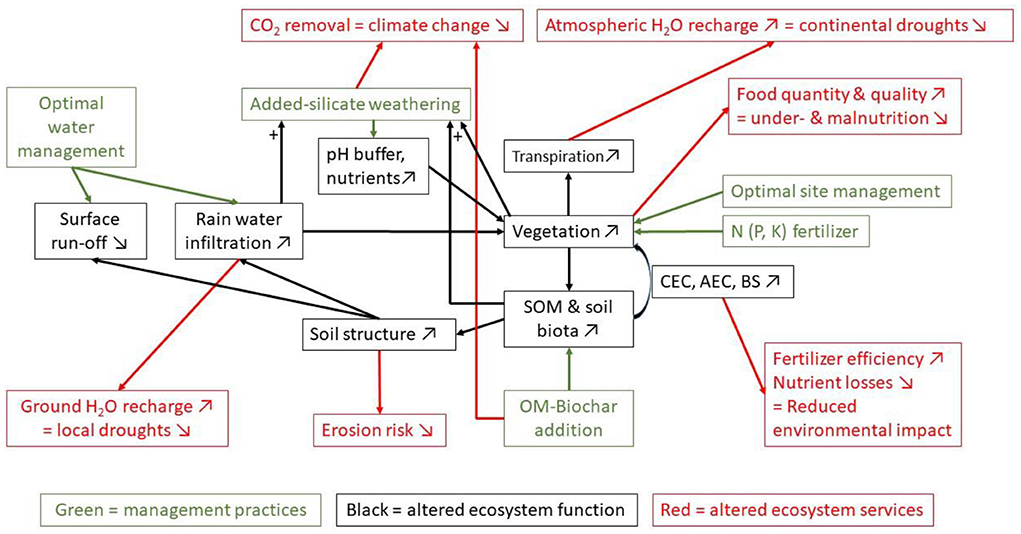
Figure 1. Overview of how combining CDR techniques to form new fertile soils would support functions and services in agricultural soils. CDRs and site management practices are given in green, effects on ecosystem services in red, and effects on ecosystem processes in black. Weathering of the added silicates releases a suite of nutrients and buffers soil pH, both benefiting vegetation growth. Plant growth must be supported by well-balanced addition of (in)organic fertilizers and site-specific management such as crop selection and engineered surfaces. Nutrient and water losses are best avoided through high inputs of organic matter (OM) or biochar from renewable biomass caches. These will increase cation- and anion exchange capacity (CEC and AEC) and base saturation (BS) of soils, as well as reinforce its biotic community leading to aggregate formation and improved soil structure. The latter not only protects against surface erosion, it also increases water infiltration, avoiding losses by overland flow and maximizing water retention, which stimulates plant growth. Optimal management of water through reservoirs, wells and irrigation systems further reduces water losses and increases plant-available water which helps to secure yields during extreme drought.
This natural development of fertile soil that supports high plant productivity thus occurred because of the combination of weatherable silicate minerals, nutrient-rich fertilizers (guano), and soil organic matter accumulation. In principle, this could be engineered on unproductive land where rain-fed agriculture is possible by applying a sufficient layer of weatherable silicate minerals (EW), mixing in substantial amounts of organic matter and kick-starting productivity by fertilizing with the nutrients that are limiting plant growth the most. Co-deployment of these CDR techniques could thus enable the formation of new fertile cropland and pasture where soils are degraded and not enough food is produced to sustain livelihoods. Because this method tackles the challenges of ending hunger and mitigating emissions at the same time it can foster a high level of support and investment which will promote widespread uptake.
Realizing high-yield, carbon-negative agriculture on degraded soil
Lessons learnt about the onset of plant productivity on volcanic islands suggest that all the following features are required to create fertile soils across degraded landscapes.
Weatherable silicate minerals provide the basis of soil formation and restoring agricultural land. Usually in applications of EW the silicates are dosed at 20–50 ton ha−1, i.e., a layer of a few mm, but this does not suffice for extremely degraded cases where topsoils have been lost. Because of the lack of empirical studies, we can only speculate about how deep the engineered topsoil should be to enable sufficient yields. Deeper soils provide more water and nutrient storage and better anchoring for the roots. However, assuming a density of 1.5 kg dm−3, every cm of engineered soil implies the transport of about 150 ton ha−1 of silicate minerals, that all needs to be ground and transported and requires a large financial investment and comes at an energy cost and (until the energy transition has been completed) also a CO2 cost. Based solely on expert judgment, we speculate that a soil depth of about 10 cm would be needed to sustain viable yields. Developing a new fertile, 10-cm surface layer, would thus imply the transport of roughly 1,500 ton ha−1, about 75 truckloads, and thus presents logistical and financial challenges, and comes with a large CO2 penalty, for real-world application. Organic matter and biochar from secondary biomass residues are the finishing coat and active ingredient of new fertile soil. How much of these resources would exactly be needed to build a 10-cm surface layer is not certain and will inherently vary. Some studies have suggested application rates of OM at an equivalent rate of 1 ton C per hectare, and of biochar at 30–60 ton dry matter per hectare (e.g., Smith et al., 2016).
The climate benefit of engineering soil on degraded land through a combination of EW, SCS and BC can be preliminary evaluated with a “back-of-the-envelope” CO2 balance. Emissions from grinding and transporting silicates reportedly amount to about 30% of the CO2 removed during the weathering for common rates of silicate amendment, i.e., 50 ton per hectare (Smith et al., 2019). Assuming mafic basalt is applied, such a quantity could sequester a total of 15 ton CO2/ha based on theoretical potential (i.e., 0.3 ton CO2 per ton rock; Strefler et al., 2018), and the supply-side emission would be 4.5 ton/ha. To create new fertile soil, we suggest applying 30 times more silicates, which will proportionally increase CO2 emission to 135 ton. However, this is compensated by different factors. If we consider that SCS and BC would realize an immediate CDR of 20 ton C or 73.5 ton CO2 per hectare, then the remaining emission is 61.5 ton CO2 per hectare. To achieve this offset with BC and cereal stover at a 3:1 ratio, landowners would have to apply about 25 ton/ha of BC with a low level of 50% fixed carbon (Morales et al., 2015) and about 52 ton/ha of dry cereal stover when 8% of C in the material is permanently retained in the soil (Xu et al., 2019). Further savings in CO2 emission can be achieved on the supply-side of EW by only using the fine fractions of available waste streams and reducing the distance between mines and soil restoration site. In this way, it is expected that the CO2 penalty of engineering soil with EW, SCS and BC could be reduced to 30 ton per hectare. Knowing that experiments with 30–50 ton silicates per hectare found a CO2 removal in the order of 1 ton C or 3.7 ton CO2 per hectare per year, it would take about 8 years to become CO2-neutral. Since much larger and longer lasting CO2 uptake is expected at rates of 1,500 ton EW per hectare, the engineered soils can become carbon neutral within fewer years, and likely carbon-negative during following decades. While these estimates are fraught with very large uncertainties, it is clear that engineering soils makes sense in terms of CO2 balance.
Depending on the type of applied silicate minerals, nutrients such as K, Ca, Mg, P, S may need to be supplied. Silicate minerals rich in non-acidic cations and P, and containing essential micronutrients are therefore to be preferred. However, the element that is lacking from these minerals is N. On the youngest of the Vestmannaeyjar islands, vegetation growth only took place when and where the non-geogenic nutrient, N, was deposited by the bird droppings. To estimate how much N would be needed to enable agriculture in engineered soils of 10 cm-depth, we assumed a target soil N concentration of 0.25% (average N concentration in arable soils; Nendel et al., 2019) and found that roughly 4 ton of N per hectare would be required to kick-start productivity for the 1,500 ton of silicate mineral added. We next estimated how much N would be removed with each harvest and thus needing to be resupplied to sustain high productivity in the long term. Taking maize as an example and assuming the mean global annual grain yield of 4.3 ton DM per hectare (FAO, 2009) and a N concentration of 1.2 % (Hirzel et al., 2020), an annual N supply of about 50 kg would be needed to sustain yields. Given the leakiness of the N cycle, this 50 kg is likely a low-end estimate. Replenishing stocks of N and the other nutrients missing from the applied silicate minerals is best achieved using organic fertilizers, such as manure, compost and other homologs, because also soil organic matter is needed (see below).
Supplying vegetation with ample nutrients only stimulates productivity when water is not limiting plant growth. In absence of infrastructures for water management, this restricts the engineering of new fertile soils to areas where rain-fed agriculture is possible. Advanced water conservation and application techniques, such as run-off collectors and irrigation, are therefore recommended, especially in view of extreme droughts becoming more frequent and more long-lived. Also, the greater the water infiltration rate and the higher the soil water retention capacity, the more water the soil can retain after rain events and the less vulnerable yields will be to drought. High water retention capacity could be realized by applying silt-sized silicate minerals. However, this would likely come at a great energy cost. Alternatively, infiltration rates and soil water retention capacity could also be enhanced by ensuring high soil organic matter contents. Maximizing the soil's organic matter content is crucial, not only because it contributes to CDR, but also because soil organic matter is key to almost every soil function, including nutrient retention and exchange (Figure 1). High soil organic matter inputs and stocks support active soil biota which stimulate the formation (and turnover) of soil aggregates, rich in micro- as well as macropores, that help avoid erosion. A proper balance between both pore size classes ensures high water infiltration, avoiding water losses by surface run-off, and maximizes water retention following rain events. Active soil biota also immobilize and slowly release nutrients, thereby helping to avoid nutrient losses from the system. In strongly degraded systems, microbial inoculants with beneficial microorganisms to enhance soil fertility and plant growth, i.e., bio-fertilizers, may be needed to jumpstart biological activity (D'Hondt et al., 2021).
Barriers and opportunities for real-world implementation
Where a degraded or shallow soil is present and sufficient rainfall occurs, we speculate that creating a 10-cm new topsoil layer with EW, SCS and BC may suffice to create a productive system because leaching and bioturbation tend to rapidly ameliorate subsoil conditions (e.g., buffer pH and increase organic matter content) without the need for additional deeper interventions. This 10-cm depth is a speculative prognosis based on expert opinion and has not been tested in modeling or empirical studies. A shallower depth (e.g., 5 cm) would come at lower financial and energy costs, and would thus be preferred, but may not suffice to retain sufficient rainwater and provide a healthy environment for roots and symbionts. Much deeper layers of newly created soil might be unrealistic in terms of the financial and energy requirements. Field experiments with representative rates of nutrient provision, including life cycle and economic analysis, are needed to draw sound conclusions on the optimal depth in true-life situations.
Covering earth surfaces with 10 cm of silicate minerals roughly requires 1,500 tons of rock material per hectare. Transporting such amounts is very costly and carbon-intensive if silicate minerals must be supplied from long distances by freight truck. Synthetic fertilizer inputs and water management infrastructures required to create high-yield carbon-negative agricultural land incur further production and transport costs, that all need to be assessed in life cycle assessment and—costing. While the upfront investments are large for applying EW, fertilization, SCS and BC at such high rates, a considerable part will be recovered from permanent increases of soil fertility and economic yields. Precisely for that reason farmers around the world use these practices to begin with. Furthermore, when the value of mitigated emissions and payments for ecosystem services (e.g., avoided deforestation and biodiversity loss) are considered, creating new fertile soil at large scale may become financially viable. To enhance the viability the following options should be considered.
Using nearby waste streams
In order to minimize the cost and required energy inputs, and maximize the CDR efficiency in economic and ecological terms, silicate minerals from waste streams are the preferred sources (Beerling et al., 2020). To minimize the financial investment and especially CO2 penalty, engineered soils must be created in the vicinity (the closer the better) of a free or cheap EW material, using whenever possible the finest fraction (<1 mm) of the silicate materials that does not require grinding (obtained e.g., by sieving). The billion tons of alkaline silicates that accumulate next to mines globally, and which are often pulverized, offer an obvious pool of substrates (Renforth et al., 2011; Renforth, 2019). Also industrial by-products such as steel slags and cement and concrete fines from demolition may provide suitable silicate substrates (Beerling et al., 2020). Yet, in as much as the economic cost of using these waste streams is relatively low, and weathering rates are typically high, their environmental and social impacts require careful investigation before they can be spread on earth surfaces. The composition of industrial by-products such as steel slags and concrete fines is notoriously heterogeneous and may require removal of pollutants to be suitable for EW applications in agriculture (e.g., Wang et al., 2018). Requirements on land accessibility by road could further restrict the area where rejuvenated soils can be constructed. This is the case in small-scale farming landscapes that are highly fragmented and have rugged topography, unless suitable logistical solutions and collective efforts are put in place.
Apply fertilizers
Research at the volcanic Vestmannaeyjar islands has indicated that on top of acquiring silicate minerals there is need for substantial N input to realize high plant productivity and, depending on the chemical composition and weathering rate of EW material that is used, other nutrients may have to be supplied. Once established, nutrient management must be tailored to the specific land use objective, i.e., food production or rangeland. The use of N2-fixing food crops, pasture grasses, fodder crops, or tree species can help minimize the need for inorganic N fertilizer input over the long term (Rosenstock et al., 2014). Nutrient supply requirements are a function of the balance between export via harvest and inputs via manure, compost or added biomass. Thus, industrial synthetic fertilizers may not be needed if sufficient manure or organic fertilizers are added to the soils. In any case, fertilizers add to the financial and environmental costs and must be included in the life cycle analysis and—costing.
Increasing and maintaining soil organic matter stocks
Next to EW, SCS and BC are two CDR techniques that are highly beneficial for soil function. The incorporation of crop residues alone generally does not suffice to maintain high soil nutrient availabilities, nor to increase soil organic matter stocks. Cultivating green manures, legume rotations, cover crops, perennials, or deep-rooting crops, and recycling manures, composts, and other organic amendments, are indispensable to maintain or increase soil organic matter stocks and build fertile soils (Smith, 2012; Smith et al., 2020). On the volcanic Vestmannaeyjar islands, the accumulation rate of soil organic matter was strongly coupled to the availability of N, the most limiting element for plant production in those soils (Leblans et al., 2017). Microorganisms that convert plant litter into soil organic matter are usually carbon-limited, even under conditions that are nutrient-limited for autotrophic communities (Soong et al., 2021). This will be specifically true for degraded and intensively cultivated agricultural soils, that are depleted in organic matter. Combining sufficient soil nutrient availability with high organic matter inputs will likely result in high microbial carbon use efficiency, converting a larger fraction of the plant litter into microbial biomass and ultimately in stabile soil organic matter (Cotrufo et al., 2013).
In parallel to these classic techniques for building up soil organic matter, adding biochar will also help maintaining or increasing soil organic matter stocks (Smith et al., 2016). The incorporation of carbonized plant biomass into agricultural land is a CDR with high permanence and potentially large co-benefits on soil nutrient and water retention, as well as on biotic communities (Jeffery et al., 2017). A study Kenya showed that biochar-producing gasifier cookstoves incentivized farmers to collect excess residues from crops which they otherwise burn in the field, thereby displacing firewood and decreasing CO2 emissions (Sundberg et al., 2020). Farm surveys in Uganda of available residues from common staple crops demonstrated that turning these into biochar could annually sequester 0.20 to 1.15 ton C ha−1, whereas the ambitious target of the “4 per mille” initiative is 0.6 ton C ha−1 (Rumpel et al., 2018; Roobroeck et al., 2019). Amendment of biochar to tropical soils was proven to increase crop yields for more than a decade, under favorable and unfavorable rainfall, as a result of improved soil pH and water holding capacity (Kätterer et al., 2019). Farmer households and agribusinesses thus profit from having low-cost energy and recurrent yield gains. Plans are being developed to scale this up, with farmers bringing their residues to nearby gasification plants for captive use of heat in drying processes and/or electricity generation for lowering costs of production or selling to provide income. In this closed-loop model, biochar will be added to inorganic fertilizers and then incorporated in the soils to increase crop productivity and nutrient use efficiency and reduce N2O emissions.
Water management
Even though the here-proposed practice of soil rejuvenation is best limited to areas where rain-fed agriculture is possible, water management is still preferred. Infrastructures, such as reservoirs that collect run-off water or irrigation systems, would in most cases further increase yields and reduce the risk for crop failure during anomalous droughts. Providing access to affordable renewable energy (solar or wind) would allow to pump irrigation water at little CO2 cost. In addition, in coastal areas or in areas with saline ground water, the water present is not suitable for irrigation purposes without treatment. Affordable renewable energy could enable desalinization of this water, thereby unlocking currently unavailable water resources that may increase or secure yields. These infrastructures would come at a high cost but have the benefit of climate-proofing the investment in engineered soils, as well as their yields.
Engineering new soils that are fertile and carbon sinks on degraded land through EW, SCS and BC has strong theoretic backing yet its real-world implementation with benefits on climate and economies is not straightforward. The CDR techniques in the proposed approach have a number of potential caveats including uncertainties due to knowledge gaps, barriers that limit implementation at scale, and risks of unwanted economic and ecological effects (Table 1). All these caveats must be addressed and countered before widespread deployment is financially viable and safe provides an expert assessment of the most important gaps, challenges, and threats that have large distortional effects on the proposed approach to rebuild degraded soils. Further details about potential caveats of EW, SCS and BC are provided by Fuss et al. (2018), Nemet et al. (2018), Lefebvre et al. (2019), Smith et al. (2019), Cao et al. (2020), Haque et al. (2020), Campbell et al. (2022), Swoboda et al. (2022), and Vienne et al. (2022), among others. While there is increasing attention to the individual CDR techniques, a key uncertainty that needs to be dealt with is how EW, SCS and BC interact. Will these techniques reinforce each other, or will antagonistic effects reduce their joint CDR and climate change mitigation potential?
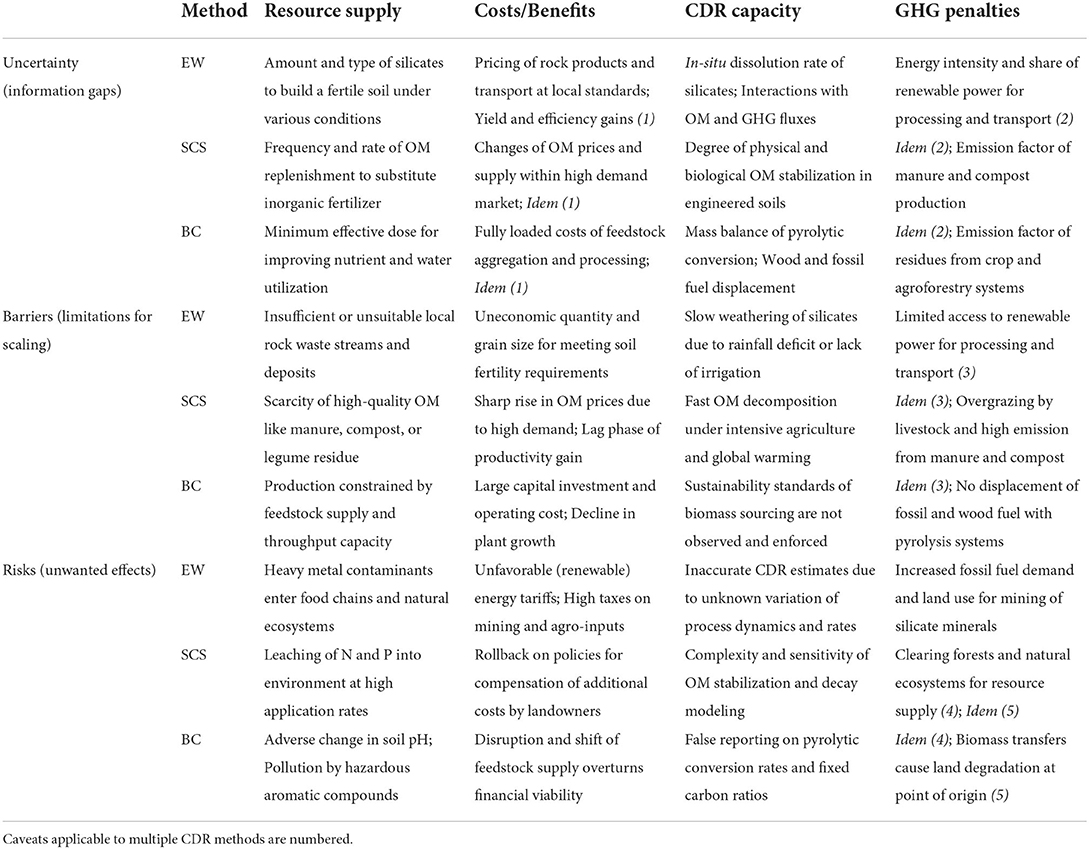
Table 1. Potential caveats for real-world deployment and climate impacts of the techniques in the proposed approach.
In conclusion, this perspective proposes to rebuild new soils in areas where climate allows food production, but where mismanagement in previous decades has severely degraded soils, rendering them unsuited for food production. This “negative erosion” can be realized by combining multiple CDR technologies that do not compete for land. Thus, these engineered soils would contribute to multiple sustainable development goals simultaneously.
Data availability statement
The original contributions presented in the study are included in the article/supplementary material, further inquiries can be directed to the corresponding author.
Author contributions
IJ proposed the original idea. All authors helped with elaborating the idea and with writing the text, contributed to the article, and approved the submitted version.
Funding
This research was supported by the Research Foundation—Flanders (FWO) and by the European Commissions (H2020 FET-open project Super Bio-Accelerated Mineral weathering: A new climate risk hedging reactor technology—“BAM”). JS was supported by Spanish Government Project PID2020115770RB-I.
Conflict of interest
The authors declare that the research was conducted in the absence of any commercial or financial relationships that could be construed as a potential conflict of interest.
Publisher's note
All claims expressed in this article are solely those of the authors and do not necessarily represent those of their affiliated organizations, or those of the publisher, the editors and the reviewers. Any product that may be evaluated in this article, or claim that may be made by its manufacturer, is not guaranteed or endorsed by the publisher.
References
Amelung, W., Bossion, D., de Vries, W., Kögel-Knabner, I., Lehmann, J., Amundson, R., et al. (2020). Towards a global-scale soil climate mitigation strategy. Nat. Commun. 11, 5427. doi: 10.1038/s41467-020-18887-7
Beerling, D. J., Kantzas, E. P., Lomas, M. R., Wade, P., Eufrasio, R. M., Renforth, P., et al. (2020). Potential for large-scale CO2 removal via enhanced rock weathering with croplands. Nature 583, 242–248. doi: 10.1038/s41586-020-2448-9
Beerling, D. J., Leake, J. R., Long, S. P., Scholes, J. D., Ton, J., Nelson, P. N., et al. (2018). Farming with crops and rocks to address global climate, food and soil security. Nat. Plants 4, 138–147. doi: 10.1038/s41477-018-0108-y
Biederman, L. A., and Harpole, W. S. (2013). Biochar and its effects on plant productivity and nutrient cycling: a meta-analysis. GCB Bioenergy 5, 202–214. doi: 10.1111/gcbb.12037
Campbell, J. S., Foteinis, S., Furey, V., Hawrot, O., Pike, D., Aeschlimann, S., et al. (2022). Geochemical negative emissions technologies: part I review. Front. Clim. 4:879133. doi: 10.3389/fclim.2022.879133
Cao, Y., Zhao, F., Zhang, Z., Zhu, T., and Xiao, H. (2020). Biotic and abiotic nitrogen immobilization in soil incorporated with crop residue. Soil Tillage Res. 202, 104664. doi: 10.1016/j.still.2020.104664
Cotrufo, M. F., Wallenstein, M. D., Boot, C. M., Denef, K., and Paul, E. (2013). The Microbial Efficiency-Matrix Stabilization (MEMS) framework integrates plant litter decomposition with soil organic matter stabilization: do labile plant inputs form stable soil organic matter? Glob. Change Biol. 19, 988–995. doi: 10.1111/gcb.12113
D'Hondt, K., Kostic, T., McDowell, R., Eudes, F., Singh, B. K., Sarkar, S., et al. (2021). Microbiome innovations for a sustainable future. Nat. Microbiol. 6, 138–142. doi: 10.1038/s41564-020-00857-w
FAO (2009). “Global cereal supply and demand brief” in Crop prospect and food situation, ed. FAO (Rome: FAO).
FAO (2021). The State of the world's land and water resources for food and agriculture – Systems at breaking point. Rome: FAO.
Folberth, C., Khabarov, N., Balkovič, J., Skalský, R., Visconti, P., Ciais, P., et al. (2020). The global cropland-sparing potential of high-yield farming. Nat. Sustain. 3, 281–289. doi: 10.1038/s41893-020-0505-x
Fuss, S., Lamb, W. F., Callaghan, M. W., Hilaire, J., Creutzig, F., Amann, T., et al. (2018). Negative emissions—Part 2: costs, potentials and side effects. Environ. Res. Lett. 13, 063002. doi: 10.1088/1748-9326/aabf9f
Gardi, C., Panagos, P., Van Liedekerke, M., Bosco, C., and de Brogniez, D. (2015). Land take and food security: assessment of land take on the agricultural production in Europe. J. Environ. Plan. Manag. 58, 898–912. doi: 10.1080/09640568.2014.899490
Ghimire, R., Bista, P., and Machado, S. (2022). “Crop yield limitation by soil organic matter decline: A Case Study from the US Pacific Northwest” in Advances in Understanding Soil Degradation. Innovations in Landscape Research, eds. E. Saljnikov, L. Mueller, A. Lavrishchev, and F. Eulenstein (Springer, Cham.), 609–621. doi: 10.1007/978-3-030-85682-3_27
Haque, F., Chiang, Y. W., and Santos, R. M. (2020). Risk assessment of Ni, Cr, and Si release from alkaline minerals during enhanced weathering. Open Agric. 5:166. doi: 10.1515/opag-2020-0016
Hirzel, J., Undurraga, P., León, L., Panichini, M., Carrasco, J., González, J., et al. (2020). Maize grain production, plant nutrient concentration and soil chemical properties in response to different residue levels from two previous crops. Acta Agric. Scand. B 70, 285–293. doi: 10.1080/09064710.2020.1725619
Hoegh-Guldberg, O., Jacob, D., Taylor, M., Bindi, M., Brown, S., Camilloni, I., et al. (2018). “Impacts of 1.5°C Global Warming on Natural and Human Systems” in Global Warming of 1.5°C. An IPCC Special Report on the impacts of global warming of 1.5°C above pre-industrial levels and related global greenhouse gas emission pathways, in the context of strengthening the global response to the threat of climate change, sustainable development, and efforts to eradicate poverty, eds. V. Masson-Delmotte, P. Zhai, H.-O. Pörtner, D. Roberts, J. Skea, P.R. Shukla, et al. (Cambridge and New York, NY: Cambridge University Press), 175–312.
Horton, P., Long, S. P., Smith, P., Banwart, S. A., and Beerling, D. J. (2021). Technologies to deliver food and climate security through agriculture. Nat. Plants 7, 250–255. doi: 10.1038/s41477-021-00877-2
IPCC. (2022). “Climate change 2022: Mitigation of climate change,” in Contribution of Working Group III to the Sixth Assessment Report of the Intergovernmental Panel on Climate Change, eds P. R. Shukla, J. Skea, R. Slade, A. Al Khourdajie, R. van Diemen, D. McCollum, M. Pathak, S. Some, P. Vyas, R. Fradera, M. Belkacemi, A. Hasija, G. Lisboa, S. Luz, and J. Malley, (Cambridge; New York, NY: Cambridge University Press). doi: 10.1017/9781009157926
Jeffery, S., Abalos, D., Prodana, M., Bastos, A. C., van Groenigen, J. W., Hungate, B. A., et al. (2017). Biochar boosts tropical but not temperate crop yields. Environ. Res. Lett. 12, 053001. doi: 10.1088/1748-9326/aa67bd
Kätterer, T., Roobroeck, D., Andrén, O., Kimutai, G., Karltun, E., Kirchmann, H., et al. (2019). Biochar addition persistently increased soil fertility and yields in maize-soybean rotations over 10 years in sub-humid regions of Kenya. Field Crops Res. 235, 18–26. doi: 10.1016/j.fcr.2019.02.015
Kaufmann, B. A., Hülsebusch, C. G., and Krätli, S. (2019). “Pastoral Livestock Systems” in Encyclopedia of Food Security and Sustainability, Vol. 3: Sustainable Food Systems and Agriculture, eds. P. Ferranti, E. M Berry, and J. R. Anderson (Elsevier), 354–360. doi: 10.1016/B978-0-08-100596-5.22179-3
Leblans, N. I. W., Sigurdsson, B. D., Aerts, R., Vicca, S., Magnússon, B., and Janssens, I. A. (2017). Icelandic grasslands as long-term C sinks under elevated organic N inputs. Biogeosci Discuss. 134, 1–21. doi: 10.1007/s10533-017-0362-5
Leblans, N. I. W., Sigurdsson, B. D., Roefs, P., Thuys, R., Magnússon, B., and Janssens, I. A. (2014). Effects of seabird nitrogen input on biomass and carbon accumulation after 50 years of primary succession on a young volcanic island, Surtsey. Biogeosciences 11, 6237–6250. doi: 10.5194/bg-11-6237-2014
Lefebvre, D., Goglio, P., Williams, A., Manning, D. A. C., de Azevedo, A. C., Bergmann, M., et al. (2019). Assessing the potential of soil carbonation and enhanced weathering through life cycle assessment: a case study for São Paulo State, Brazil. J. Clean. Prod. 233, 468–481. doi: 10.1016/j.jclepro.2019.06.099
Lundqvist, J., de Fraiture, C., and Molden, D. (2008). Saving Water: From Field to Fork – Curbing Losses and Wastage in the Food Chain. SIWI Policy Brief. SIWI.
Mbow, C., Rosenzweig, C., Barioni, L. G., Benton, T. G., Herrero, M., Krishnapillai, M., et al. (2019). “Food Security” in Climate Change and Land: an IPCC special report on climate change, desertification, land degradation, sustainable land management, food security, and greenhouse gas fluxes in terrestrial ecosystems, eds. P.R. Shukla, J. Skea, E. Calvo Buendia, V. Masson-Delmotte, H.-O. Pörtner, D.C. Roberts, et al. (Cambridge and New York, NY: Cambridge University Press), 437–550.
Morales, V. L., Pérez-Reche, F. J., Hapca, S. M., Hanley, K. L., Lehmann, J., and Zhang, W. (2015). Reverse engineering of biochar. Bioresour. Technol. 183, 163–174, doi: 10.1016/j.biortech.2015.02.043
Nemet, G. F., Callaghan, M. W., Creutzig, F., Fuss, S., Hartmann, J., Hilaire, J., et al. (2018). Negative emissions—Part 3: innovation and upscaling. Environ. Res. Lett. 13, 063003. doi: 10.1088/1748-9326/aabff4
Nendel, C., Melzer, D., and Thorburn, P. J. (2019). The nitrogen nutrition potential of arable soils. Sci. Rep. 9, 5851. doi: 10.1038/s41598-019-42274-y
O'Riordan, T., and Stoll-Kleemann, S. (2015). The challenges of changing dietary behavior toward more sustainable consumption. Environ. Sci. Policy Sustain. Develop. 57, 4–13. doi: 10.1080/00139157.2015.1069093
Panagea, I. S., Berti, A., Cermak, P., Diels, J., and Elsen, A., Kusá, H., et al. (2021). Soil water retention as affected by management induced changes of soil organic carbon: analysis of long-term experiments in Europe. Land 10, 1362. doi: 10.3390/land10121362
Persson, L., Almroth, B. M. C., Collins, C. D., Cornell, S., de Wit, C. A., Diamond, M. L., et al. (2022). Outside the safe operating space of the planetary boundary for novel entities. Environ. Sci. Technol. 56, 1510–1521. doi: 10.1021/acs.est.1c04158
Porkka, M., Gerten, D., Schaphoff, S., Siebert, S., and Kummu, M. (2016). Causes and trends of water scarcity in food production. Environ. Res. Lett. 11, 015001. doi: 10.1088/1748-9326/11/1/015001
Ramos, C. G., Hower, J. C., Blanco, E., Silva Oliveira, M. L., and Theodoro, S. H. (2022). Possibilities of using silicate rock powder: an overview. Geosci. Front. 13, 101185. doi: 10.1016/j.gsf.2021.101185
Ranganathan, J., Vennard, D., Waite, R., Lipinski, B., Searchinger, T., and Dumas, P. (2018). Shifting Diets for a Sustainable Food Future. Working Paper, Installment 11 of Creating a Sustainable Food Future. Washington, DC: World Resources Institute.
Razzaghi, F., Obour, P. B., and Arthur, E. (2020). Does biochar improve soil water retention? a systematic review and meta-analysis. Geoderma 361, 114055. doi: 10.1016/j.geoderma.2019.114055
Renforth, P. (2019). The negative emission potential of alkaline materials. Nat. Commun. 10, 1401. doi: 10.1038/s41467-019-09475-5
Renforth, P., Washbourne, C. -L., Taylder, J., and Manning, D. A. C. (2011). Silicate production and availability for mineral carbonation. Environ. Sci. Technol. 45, 2035–2041. doi: 10.1021/es103241w
Riahi, K., Van Vuuren, D. P., Kriegler, E., Edmonds, J., O'neill, B. C., Fujimori, S., et al. (2017). The shared socioeconomic pathways and their energy, land use, and greenhouse gas emissions implications: an overview. Glob. Environ. Chang. 42, 153–168. doi: 10.1016/j.gloenvcha.2016.05.009
Rockström, J., Karlberg, L., Wani, S. P., Barron, J., Hatibu, N., Oweis, T., et al. (2010). Managing water in rainfed agriculture—The need for a paradigm shift. Agric. Water Manag. 97, 543–550. doi: 10.1016/j.agwat.2009.09.009
Roobroeck, D., Hood-Nowotny, R., Nakubulwa, D., Tumuhairwe, J. B., Mwanjalolo, M., Ndawula, I., et al. (2019). Biophysical potential of crop residues for biochar carbon sequestration, and co-benefits, in Uganda. Ecol. Appl. 29:e01984. doi: 10.1002/eap.1984
Rosenstock, T. S., Tully, K. L., Arias-Navarro, C., Neufeldt, H., Butterbach-Bahl, K., and Verchot, L. V. (2014). Agroforestry with N2-fixing trees: sustainable development's friend or foe? Curr. Opin. Environ. Sustain. 6, 15–21. doi: 10.1016/j.cosust.2013.09.001
Rumpel, C., Amiraslani, F., Koutika, L. S., Smith, P., Whitehead, D., and Wollenberg, E. (2018). Put more carbon in soils to meet Paris climate pledges. Nature 564, 32–34. doi: 10.1038/d41586-018-07587-4
Schmidt-Traub, G., Obersteiner, M., and Mosnier, A. (2019). Fix the broken food system in three steps. Nature 569, 181–183. doi: 10.1038/d41586-019-01420-2
Smith, P. (2012). Soils and climate change. Curr. Opin. Environ. Sustain. 4, 539–544 doi: 10.1016/j.cosust.2012.06.005
Smith, P. (2016). Soil carbon sequestration and biochar as negative emission technologies. Glob. Change Biol. 22, 1315–1324. doi: 10.1111/gcb.13178
Smith, P., Adams, J., Beerling, D. J., Beringer, T., Calvin, K. V., Fuss, S., et al. (2019). Land-management options for greenhouse gas removal and their impacts on ecosystem services and the sustainable development goals. Annu. Rev. Environ. Resour. 44, 255–286. doi: 10.1146/annurev-environ-101718-033129
Smith, P., Calvin, K., Nkem, J., Campbell, D., Cherubini, F., and Grassi, G. (2020). Which practices co-deliver food security, climate change mitigation and adaptation, and combat land-degradation and desertification? Glob. Change Biol. 26, 1532–1575. doi: 10.1111/gcb.14878
Smith, P., Davis, S. J., Creutzig, F., Fuss, S., Minx, J., Gabrielle, B., et al. (2016). Biophysical and economic limits to negative CO2 emissions. Nat. Clim. Change 6, 42–50. doi: 10.1038/nclimate2870
Soong, J. L., Fuchslueger, L., Marañon-Jimenez, S., Torn, M. S., Janssens, I. A., Penuelas, J., et al. (2021). Microbial carbon limitation: the need for integrating microorganisms into our understanding of ecosystem carbon cycling. Glob. Change Biol. 26, 1953–1961. doi: 10.1111/gcb.14962
Spinonii, J., Barbosa, P., Cherlet, M., Forzieri, G., McCormick, N., Naumann, G., et al. (2021). How will the progressive global increase of arid areas affect population and land-use in the 21st century? Glob. Planet. Change 205, 103597. doi: 10.1016/j.gloplacha.2021.103597
Strefler, J., Amann, T., Bauer, N., Kriegler, E., and Hartmann, J. (2018). Potential and costs of carbon dioxide removal by enhanced weathering of rocks. Environ. Res. Lett. 13, 034010. doi: 10.1088/1748-9326/aaa9c4
Sundberg, C., Karltun, E., Gitau, J. K., Kätterer, T., Kimutai, G. M., Mahmoud, Y., et al. (2020). Biochar from cookstoves reduces greenhouse gas emissions from smallholder farms in Africa. Mitig. Adapt. Strateg. Glob. Change 25, 953–967. doi: 10.1007/s11027-020-09920-7
Swoboda, P., Döring, T. F., and Hamer, M. (2022). Remineralizing soils? the agricultural usage of silicate rock powders: a review. Sci. Total Environ. 807, 150976. doi: 10.1016/j.scitotenv.2021.150976
Tan, Z. X., Lal, R., and Wiebe, K. D. (2005). Global soil nutrient depletion and yield reduction. J. Sustain. Agric. 26, 123–146. doi: 10.1300/J064v26n01_10
UNCCD (2014). Land Degradation Neutrality: Resilience at Local, National and Regional Levels. Bonn: UNCCD.
van Dijk, M., Morley, T., Rau, M. L., and Saghai, Y. (2021). A meta-analysis of projected global food demand and population at risk of hunger for the period 2010–2050. Nat. Food 2, 494–501. doi: 10.1038/s43016-021-00322-9
Vicca, S., Goll, D. S., Hagens, M., Hartmann, J., Janssens, I. A., Neubeck, A., et al. (2021). Is the climate change mitigation effect of enhanced silicate weathering governed by biological processes? Glob. Change Biol. 28, 711–726. doi: 10.1111/gcb.15993
Vienne, A., Poblador, S., Portillo-Estrada, M., Hartmann, J., Ijiehon, S., Wade, P., et al. (2022). Enhanced weathering using basalt rock powder: Carbon sequestration, co-benefits and risks in a mesocosm study with Solanum tuberosum. Front. Clim. 4, 869456. doi: 10.3389/fclim.2022.869456
Wang, W., Zeng, C., Sardans, J., Zeng, D., Wang, C., Bartrons, M., et al. (2018). Industrial and agricultural wastes decreased greenhouse-gas emissions and increased rice grain yield in a subtropical paddy field. Exp. Agric. 54, 623–640. doi: 10.1017/S001447971700031X
Xu, H., Sieverding, H., Kwon, H., Clay, D., Stewart, C., Johnson, J. M. F., et al. (2019). A global meta-analysis of soil organic carbon response to corn stover removal. GCB Bioenergy 11, 1215–1233. doi: 10.1111/gcbb.12631
Keywords: enhanced weathering, biochar, soil carbon storage, food security, undoing soil degradation, engineering soils
Citation: Janssens IA, Roobroeck D, Sardans J, Obersteiner M, Peñuelas J, Richter A, Smith P, Verbruggen E and Vicca S (2022) Negative erosion and negative emissions: Combining multiple land-based carbon dioxide removal techniques to rebuild fertile topsoils and enhance food production. Front. Clim. 4:928403. doi: 10.3389/fclim.2022.928403
Received: 25 April 2022; Accepted: 18 August 2022;
Published: 07 September 2022.
Edited by:
Simona Liguori, Clarkson University, United StatesReviewed by:
Lyla Taylor, The University of Sheffield, United KingdomKyle S. Hemes, Amazon, United States
Copyright © 2022 Janssens, Roobroeck, Sardans, Obersteiner, Peñuelas, Richter, Smith, Verbruggen and Vicca. This is an open-access article distributed under the terms of the Creative Commons Attribution License (CC BY). The use, distribution or reproduction in other forums is permitted, provided the original author(s) and the copyright owner(s) are credited and that the original publication in this journal is cited, in accordance with accepted academic practice. No use, distribution or reproduction is permitted which does not comply with these terms.
*Correspondence: Ivan A. Janssens, aXZhbi5qYW5zc2Vuc0B1YW50d2VycGVuLmJl