- 1Department of Medicine, University of California, Los Angeles, Los Angeles, CA, United States
- 2Department of Physiology, University of California, Los Angeles, Los Angeles, CA, United States
- 3Department of Orthopaedic Surgery, University of California, Los Angeles, Los Angeles, CA, United States
- 4Department of Bioengineering, University of California, Los Angeles, Los Angeles, CA, United States
Previously considered a degenerative process, cardiovascular calcification is now established as an active process that is regulated in several ways by lipids, phospholipids, and lipoproteins. These compounds serve many of the same functions in vascular and valvular calcification as they do in skeletal bone calcification. Hyperlipidemia leads to accumulation of lipoproteins in the subendothelial space of cardiovascular tissues, which leads to formation of mildly oxidized phospholipids, which are known bioactive factors in vascular cell calcification. One lipoprotein of particular interest is Lp(a), which showed genome-wide significance for the presence of aortic valve calcification and stenosis. It carries an important enzyme, autotaxin, which produces lysophosphatidic acid (LPA), and thus has a key role in inflammation among other functions. Matrix vesicles, extruded from the plasma membrane of cells, are the sites of initiation of mineral formation. Phosphatidylserine, a phospholipid in the membranes of matrix vesicles, is believed to complex with calcium and phosphate ions, creating a nidus for hydroxyapatite crystal formation in cardiovascular as well as in skeletal bone mineralization. This review focuses on the contributions of lipids, phospholipids, lipoproteins, and autotaxin in cardiovascular calcification, and discusses possible therapeutic targets.
Significance of Cardiovascular Calcification
Previously considered a degenerative process, cardiovascular calcification is now established as a regulated process (1–3) in which vascular and valvular mesenchymal cells undergo osteogenic differentiation (4–8). Clinically, vascular calcification is considered pathognomonic of atherosclerosis. In the coronary arteries, the degree of calcification has been shown to correlate closely with the degree of atherosclerotic plaque burden (9). The presence of calcium deposits in atherosclerotic lesions also appears to increase the risk of intraplaque hemorrhage (10), in which mechanical disruption within a lesion tears microvessels, causing bleeding. The bleeding expands the lesion, so that it may abruptly encroach on the artery lumen causing stenosis or occlusion, which result in ischemia or infarction.
In the aortic valve, calcification carries especially high risk of mortality. Calcific aortic valve disease (CAVD) affects 13% of the population over 65 years of age in the US. Of those who develop symptoms, half die within 2 years (11). Even in the absence of hemodynamically significant obstruction of left ventricular outflow, CAVD is associated with greater risk of cardiovascular events (12). The high morbidity and mortality are due to leaflet stiffening, retraction, and stenosis, which limit valve opening and closing, increasing outflow resistance and oxygen demand, while impairing myocardial perfusion and oxygen supply. Currently, the only options are surgical or trans-catheter interventional replacement of the valve. A wide range of factors and mechanisms are now known to also mediate CAVD, including those in the general categories of hyperlipidemia, inflammation, oxidation, diabetes, apoptosis, hyperphosphatemia, and mechanical forces (13–20). Many of these may act in sequence or in concert, but others are independent (21). This review focuses on the contributions of lipids and the interconnections among lipid metabolism, inflammation, and osteogenesis.
Biomechanical Considerations
Although the mechanisms are not entirely clear, mechanical stresses on the cardiac valves and artery walls, including oscillatory shear on the endothelium and cyclic strain on the valvular interstitial cells and vascular smooth muscle cells, have been implicated in the pathogenesis of cardiovascular calcification (22–24). Cumulatively, these stresses may promote cardiovascular calcification, as they do in skeletal bone, whether through cellular injury leading to dystrophic calcification, or mechanotransduction-related osteogenic differentiation of cells. With regard to lipids, biomechanical stresses may lead to endothelial injury or increased permeability where low shear and oscillatory flows have the capacity to promote accumulation of plasma lipoproteins, such as low-density lipoprotein (LDL) and lipoprotein(a) [Lp(a)]. The severity of calcification in the mitral valve does not correspond with that in the aortic valve, consistent with early work showing that the aortic valve calcifies 10 years earlier than the mitral valve (25). The reason for the mismatch remains unclear, but it suggests a relationship to shear stress or pressure, given that they are exposed to the same oxygen levels and flow volume. Once valve disease begins, the mechanical triggers are likely to worsen. Merryman and Schoen have clarified this reciprocal nature of the interaction between mechanical forces and tissue: while hemodynamic forces affect tissue properties, changes in tissue properties also affect hemodynamic forces (24). Computational models of the valve have been used successfully to predict these interactions (26).
Whether calcium deposits promote plaque rupture or stability is controversial. While plaque rupture into the lumen has been found to occur more often in non-calcified areas of plaque in patients who died from coronary obstruction, the sectioning process itself may disrupt the plaque, especially in areas of lipid pools, where the histologic processing usually removes the lipid. As an alternative, magnetic resonance imaging can detect intraplaque rupture in living patients. Lin et al. performed MRI of carotid arteries in over 100 living patients, and found a strong link (O.R. ≥ 10) between the presence of any type of calcium deposits (whether multiple, surface, or mixed) and intraplaque hemorrhage, after adjusting for age, LDL, maximum wall thickness, and maximum soft plaque thickness, suggesting that calcium mineral deposits promote biomechanical rupture (10). An engineering analysis suggests that the rupture stress is highly concentrated at calcium deposits on the edges that face the direction of stress (27).
In clinical discussions of calcification, the concept of stability is widely used, without careful distinction between its two meanings. Lesion stability may be clinical or biomechanical. Clinical stability refers to the presence and time course of patient signs and symptoms of vascular stenosis or occlusion, such as ischemia, angina, transient neurological symptoms, or infarcts. Biomechanical stability refers to the relative values of tissue mechanical strength vs. tissue mechanical stresses, which is often indirectly inferred from images showing distribution of lesion components, which, as an aside, may improve with machine learning techniques (28). Although these two meanings of stability relate in that biomechanical instability often leads to clinical instability, in investigations pertaining to the effects of treatments on lesions, the two should be clearly distinguished.
Lipids
The association of serum LDL-cholesterol levels with vascular calcification in patients is well-established (29), and the association is even stronger when the average cholesterol levels are integrated over many years (30). In mice, hyperlipidemia consistently leads to calcification of the aortic root within a few weeks (31). Serum lipidomic analysis in humans has identified fatty acid metabolic markers for cardiovascular calcification. For instance, patients with high coronary calcium scores have more 20:4 fatty acyl chain lipid species and less 18-carbon fatty acyl chain phosphatidylcholines in their serum (32). Bioinformatics approaches have identified phospholipid phosphatase 3 as a key gene in calcific valve disease (33). In vascular cell culture, oxidized lipids induce rapid mineralization (34). As evidence for their importance, use of lipoprotein-deficient serum in the culture medium prevented formation of calcified nodules in the in vitro model of vascular cell calcification (35). Neutral lipids accumulate in the sub-endothelial layer of arteries (36). With time, these lipids undergo non-enzymatic modification by the action of products of cellular metabolism (37), such as mildly oxidized phospholipids. These oxidized phospholipids are known to promote calcification in vitro through multiple mechanisms. They induce inflammatory cytokines (7), including TNF-alpha, which promotes calcification in part by enhancing BMP-2 activity through inhibition of its inhibitor, Smad6 (7). Modified phospholipids also stimulate calcification by impaired phagocytosis of apoptotic bodies (38). The elastin layer of the artery wall is often the first site of hydroxyapatite crystal formation, especially the ends of fragments, and this may relate to its association with lipids. Elastin is known to act as a sponge for fatty acids (39) and to have high affinity for lipids, LDL, and calcium (40). Thus, pathogenic factors involved in atherogenesis are also involved in calcific disease of the artery wall.
Lipids are also involved in CAVD. As in atherosclerosis, lipid accumulation progresses with age in aortic valves (25, 41). Neutral lipids accumulate in the fibrosa (36). The lesion area, which is usually at the base of the valve leaflet, shows substantial displacement of elastin and thickening of the fibrosa (42). Calcified valves contain sub-endothelial accumulations of lipoproteins ApoB and Apo(a) (36, 42). The earliest calcium deposits are found adjacent to lipoproteins in the deeper regions of the fibrosa along the aortic annulus (42). Lipoprotein deposits are known to undergo non-enzymatic oxidation, and oxidized lipids activate T lymphocytes leading to expression of the oxidized lipid receptor, LOX-1, and proinflammatory cytokines (43, 44). Consistent with this, activated T lymphocytes are also found in the fibrosa layer of the stenotic valves adjacent to calcium deposits (36, 45–47). Even sphingolipid accumulation is associated with calcific valve disease, as seen in patients with Gaucher's sphingolipid storage disease (48). Broadly speaking, it is generally accepted that the mechanisms underlying CAVD are similar to those of atherosclerosis, but they have distinct features, which remain to be clarified. In bioprosthetic valves, lipids also deposit in the spongiosa layer, and the observed lipids (phospholipids, oleic acid, triglycerides, and unesterified cholesterol) are thought to act as nucleation sites for mineral crystals (49).
Lipoprotein(a) [Lp(a)]
Lp(a) received increased attention in this field in 2013, when Thanassoulis and colleagues reported that a genetic variant in the LPA locus encoding Lp(a) showed genome-wide significance for the presence of aortic valve calcification and stenosis across multiple racial and ethnic groups (50). Lp(a) levels have also been associated with cardiovascular risk in a subgroup of patients statins in a clinical trial (51). Lp(a), a lipoprotein particle similar to LDL, is unique in that the apoB-100 protein spanning it has a covalent, disulfide, bond with a glycoprotein, apolipoprotein(a) [apo(a)].
Epidemiologically, Lp(a) levels have a significant positive relation with serum levels of matrix GLA protein (MGP), a known inhibitor of bone morphogenetic protein-2 (BMP-2) (52). If this association were due to Lp(a) induction of MGP, then one would expect Lp(a) to have an inhibitory effect on BMP-2 and an inverse association with vascular calcification. However, Lp(a) is positively associated with coronary calcification, suggesting that the high levels of MGP associated with Lp(a) are due to a negative feedback loop. Patients with the most progression of coronary calcification detected by electron-beam computed tomography (EBCT) imaging also had the highest levels of Lp(a), and patients with the highest levels (>30 mg/dL) of Lp(a) also had the greatest progression of coronary calcification in hypercholesterolemic patients undergoing statin therapy (53).
Calcified human aortic valves have an abundance of Lp(a), and in vitro studies have shown that exposure to Lp(a) can promote the chondro-osteogenic phenotype in human aortic valve interstitial cells (54). Interestingly, however, the association of Lp(a) with cardiovascular calcification may be explained by its potential role as a delivery vehicle for pro-calcific and pro-inflammatory factors to sites of endothelial injury. One of its components, apo(a), is a homolog of plasminogen, and as such, can bind to exposed fibrin at areas of denuded or injured endothelium. For unknown reasons, Lp(a) is a preferential carrier of oxidized phospholipids (55), and also carries the important enzyme, autotaxin (56), which is discussed in more detail below. Thus, via Lp(a), these compounds may be targeted to sites of endothelial injury, such as occurs on mechanically stressed aortic valve leaflets or atherosclerotic plaque, thereby potentiating the calcification process in these lesions.
Autotaxin And Lysophosphatidic Acid
One potential therapeutic target for cardiovascular calcification, lysophosphatidic acid (LPA), is a derivative of oxidized phospholipids, and a potent proinflammatory factor (57, 58). It is produced from lysophosphatidylcholine (LPC) by the action of autotaxin, a lysophospholipase, and another potential therapeutic target. Lp(a), together with autotaxin activity and LPA, are found in human CAVD where they colocalize with oxidized LDL and calcium deposits (56). Autotaxin is also produced and secreted by VICs, and its expression is associated with inflammatory markers in VICs (56). It is widely thought to be involved in calcific atherosclerosis and valvulopathy, but clinical trials have not been completed.
Autotaxin and LPA are also actively considered therapeutic targets in other forms of chronic inflammation, such as idiopathic pulmonary fibrosis and arthritis, as well as in multiple sclerosis and cancer, where it is upregulated (59–61). Genetic deletion of the autotaxin gene is embryonically lethal due to vascular and neuronal defects (62–64). Coincidentally, autotaxin also functions as a phosphodiesterase, ectonucleotide pyrophosphatase/phosphodiesterase-2 (ENPP2) (65). Autotaxin is structurally similar to ectonucleotide pyrophosphatase/phosphodiesterase-1 (ENPP1), a nucleotide pyrophosphatase that generates pyrophosphate, a potent inhibitor of calcification (66). ENPP1 deficiency underlies disorders of ectopic calcification (66, 67). The main structural difference between the two ENPPs is that autotaxin has a lipid-binding pocket and open tunnel, presumably for the fatty acid chain, whereas ENPP1 does not (66). Autotaxin has a rapid turnover (68), and interestingly, in breast tumors, blocking LPA production with a competitive autotaxin inhibitor decreased expression of Nrf2, multidrug-resistant transporters, and antioxidant genes (69), possibly as a feedback response. Autotaxin is known to be bound and inhibited by bile salts (70), raising interesting questions of whether it has any relation to why the famous “Paigen diet” which features the addition of the bile salt, cholate, to a high-fat diet doubles the severity of atherosclerosis (71). From the standpoint of calcification, it is intriguing that, like its relative, ENPP1, autotaxin has the capacity for producing pyrophosphate, an inhibitor of calcification, raising questions about possible pro- and anti-atherogenic effects of autotaxin inhibition.
The role of autotaxin in disease pathogenesis is supported by evidence of chronic inflammation and enhanced rates of breast cancer in autotaxin transgenic mice (72) as well as by the attenuation of rheumatoid arthritis and pulmonary fibrosis in mice with conditional deletion of autotaxin (73, 74). A large number of autotaxin inhibitors have been tested for possible therapeutic use in inflammatory diseases and cancer (75–81). Katsifa et al. have shown that 80% reduction of LPA levels is well-tolerated in autotaxin-null mice or in mice treated with the autotaxin inhibitor, PF8380 (82).
Statins
Given the substantial links between lipids and cardiovascular calcification, it was anticipated that lipid lowering with statins would be certain to prove to be an effective treatment. Reports from the 1990's and 2000's were supportive of that possibility, although they were less impressive than expected. In mice, lipid lowering by genetic techniques inhibited aortic valve disease (83), but, in humans, the ASTRONOMER trial showed no reduction of aortic stenosis progression by statin therapy in patients with mild to moderate disease (84). Several reasons have been proposed, including the possibility that the disease was already too advanced by the time of treatment, and that a benefit may be seen if treatment were to begin at an earlier stage of disease. It may also indicate that statin treatment has different effects than genetic manipulation. Moreover, statins tend to increase Lp(a) levels (85), which, as noted above, may promote human CAVD. So far, only statins have been tested by a randomized, controlled trial as a possible medical treatment for aortic stenosis.
Compared with mice, larger animal models may provide more accurate measurements of stenosis severity, based on Doppler jet velocity, and may provide physiological conditions more similar to those of humans. For instance, a hamster model of hyperlipidemic–hyperglycemic cardiovascular calcification has been reported (86). In addition, a myocardial infarction-prone strain of Watanabe heritable hyperlipidemic (WHHLMI) rabbits develops more severe aortic valve stenosis (almost 50% reduction) and more evident transvalvular pressure gradients (almost 50% increase) with thickened and degenerated valve leaflets as well as calcified nodules and increased expression of osteogenic factors including Sox9, RANKL, BMP-2, and Runx2 at 30 months of age (86).
In pre-clinical studies, statins were found to reduce progression of calcification in rats with vascular calcification induced by vitamin D and warfarin (87), even though the mechanism of calcification in this pharmacological model is not known to involve lipids. In non-randomized clinical studies, when patients without known coronary artery disease were treated with statins for over a year based on their calcium scores, a decrease in calcification was reported for those who lowered their LDL-cholesterol level below 120 mg/dl (88). In a later study, coronary calcium score progression was significantly reduced, and reversal was seen in patients whose LDL dropped to below 100 mg/dl (89). Later studies found less benefit, including no significant effect of 10 years of lipid-lowering therapy on carotid calcification as measured by MRI (89). Even though prosthetic valve degeneration is independently associated with dyslipidemia in patients (90), and even though direct ethanol-extraction of lipids from bioprosthetic valves reduces their calcification in vivo (91–93), statin treatment failed to reduce bioprosthetic valve calcification in patients (94).
In the last decade, compelling evidence is showing the opposite effect, that statins promote vascular calcification. In a London-based clinical study of almost 400 patients with diabetes, Anand and colleagues found that statin treatment was an independent predictor of progression of coronary calcification with an OR of 2.3, but not a predictor of acute cardiac events (95). Some suggested that this unexpected result was attributable to higher baseline coronary calcium scores or insufficient lowering of LDL in the statin-treated group (96). However, those potential confounders were excluded in a later VA Diabetes Trial, in which Saremi and colleagues, showed the same results: frequent statin use was associated with accelerated cardiovascular calcification compared with infrequent use, but incidence of cardiovascular events with frequent statin use was not significantly different from those with infrequent statin use over a 4–5 year follow-up (96). Consistent with this, Puri and colleagues used coronary intravascular ultrasound to show that statin treatment promotes coronary atherosclerotic calcification (97). Subsequent reports have consistently tied statin treatment to increased coronary calcification. The Rotterdam study of over 1,700 patients showed statin treatment, at any dosage, was associated with greater calcification, which increased with duration of statin use (98). In the PARADIGM study, statin treatment was associated with more rapid progression of coronary calcification (99). In a study, all 147 patients on statins had progression of coronary calcification (53). Statin-induced calcification occurred even in combination with proprotein convertase subtilisin/kexin type 9 (PCSK9) inhibitors (100). However, patients on a combination of statin and PCSK9-inhibitor therapy had a slower rate of coronary calcification progression compared with statin mono-therapy (100). Mechanisms by which statins may promote coronary calcification remain unclear. Some in vitro studies have suggested a direct effect (101), but indirect effects, such as statin-mediated increase in Lp(a) levels (85), are also possible mechanisms.
This has created a dilemma, putting two long established “truths” in conflict: (1) that cardiovascular calcification increases cardiovascular mortality, and (2) that statins reduce cardiovascular mortality. To resolve this, some authors have dismissed or reversed the first. Although the evidence linking vascular calcification and mortality has not changed, some reports assert that the calcification is beneficial, as in “statin use seems to beneficially influence the composition of carotid atherosclerosis” (98) and others have proposed that promotion of calcification is the mechanism for the beneficial effect of statins on mortality (97). Theoretically, the possibility that statins alter biomechanical effects of calcification through changes in specific morphological features cannot be excluded.
This conundrum has translated to clinical care: physicians are advised to tell their patients that coronary calcification is dangerous as the basis for initiating statin therapy, and, later, when the calcification progresses further on the statin therapy, to tell their patients that coronary calcification is protective. Thus, a deeper understanding of the relationships between statin therapy, cardiovascular calcification, and clinical outcomes is necessary.
Lipids And Phospholipids In Bone/Cartilage Calcification
Lipids are also closely associated with bone mineralization. Based on nuclear magnetic resonance (NMR) spectroscopic analysis, vascular calcium deposits, and bone both have fatty-acid lipids entrained in their mineral. These fatty acids may include methyl-branched fatty acids, which would be consistent with lipoprotein particle remnants (102). As noted by Reid et al., “colocalization of mineral and lipid may be coincidental, but it could also reflect an essential mechanistic component of biomineralization” (102). As evidence of their strong association with mineral, phospholipids cannot be totally extracted from calcified tissues, such as bone, until the tissues are decalcified. Among phospholipids, phosphatidylserine is considered the most likely to be involved in initiation of mineralization because of its extremely high binding affinity for Ca2+ ions (103). Specific membrane proteins in lipid rafts also participate in mineralization. Phospholipids are even involved in mineralization of dental plaque, the calcium deposits formed on teeth by bacteria (104). The lipid profiles found in human cardiovascular calcification share many features with those in newly mineralized bone and calcified cartilage, particularly the complex acidic phospholipids (105).
Matrix Vesicles
Both bone and vascular mineralization appear to be initiated by matrix vesicles, ~100 nm membrane-bound extracellular vesicles. Phospholipids form the membrane of matrix vesicles (also known as extracellular vesicles), where calcium hydroxyapatite crystal formation is initiated when calcium and phosphate ions interact with phosphatidylserine to form phospholipid-calcium-phosphate complexes. Matrix vesicles are extruded from the outer plasma membrane of cells, which gives them a composition similar to lipid rafts (106), and they are enriched in certain components, including cholesterol, free fatty acids, and sphingomyelin (107). They may also be released by fusion of multivesicular bodies (MVB) with the plasma membrane. Interestingly, lipoproteins may interfere with analyses of matrix vesicle composition, because LDL particles may themselves produce extracellular vesicles (108), and because LDL particles co-pellet with matrix vesicles in these analyses (109, 110).
Matrix vesicles from bone were described poetically by H.C. Anderson as: “protected and controlled internal microenvironments outside cells in which specific metabolic objectives of the host cell may be pursued vigorously at a distance from the host cell” (111). They were originally discovered in 1967 in bone tissue, where they were identified as nucleation sites for hydroxyapatite crystals. Once in the extracellular space, they often bind to collagen, a process that appears to induce alkaline phosphatase activity and calcification (112). Matrix vesicles were found a decade later in calcific atherosclerotic lesions in specimens of human aortas (113). Now they are known to be present in human calcific vasculopathy and valvulopathy (114), and, most likely, they would be found at any sites of physiological or pathophysiological calcification. Matrix vesicles from vascular smooth muscle cells and bone cells both include calcium- and phospholipid-binding proteins, phosphate transporters, and cytoskeletal and surface proteins (115).
Matrix vesicles also mediate a wide range of functions besides initiation of mineralization, such as cell-cell communication, depending on their distinct contents and properties. These may include different microRNA species (miR-122-5p vs. miR-150-5p), different specific phospholipids, different activities of alkaline phosphatase and phospholipase A2, different membrane stiffness, and different membrane receptors (116). Due to these distinct functions and properties, matrix vesicles are now described by a wide variety of names: microvesicles, extracellular membrane vesicles, ectosomes, extracellular vesicles, microparticles, and exosomes, depending on cells of origin, tissue location, and the investigator's field of science.
A growing consensus is that matrix vesicles are a type of exosome, perhaps the prototypical one, or at least homologous with exosomes (117). Given their tendency to bind to collagen, it has been proposed that they are “anchored exosomes” (118). Exosomes are extracellular, membrane-bound products of the complex endosomal pathway. Their multilamellar membranes (119) may be understood by topological analysis of progressive invagination, fusion, autophagy, and evagination steps of endosomal processing through MVB (Figure 1). Most interesting, given that the multivesicular body may be contiguous at times with the extracellular space, mature matrix vesicles within the multivesicular body may be, nonetheless, topologically exterior to the cell's cytoplasm. As such, they may begin formation of hydroxyapatite crystals while still “deep within” the perimeter of the cell (118). Intracellular vesicles containing calcium phosphate have been demonstrated within osteoblasts—in skeletal bone formation (121), although spontaneous mineral formation within intracellular vesicles may be inhibited where there is an acidic microenvironment.
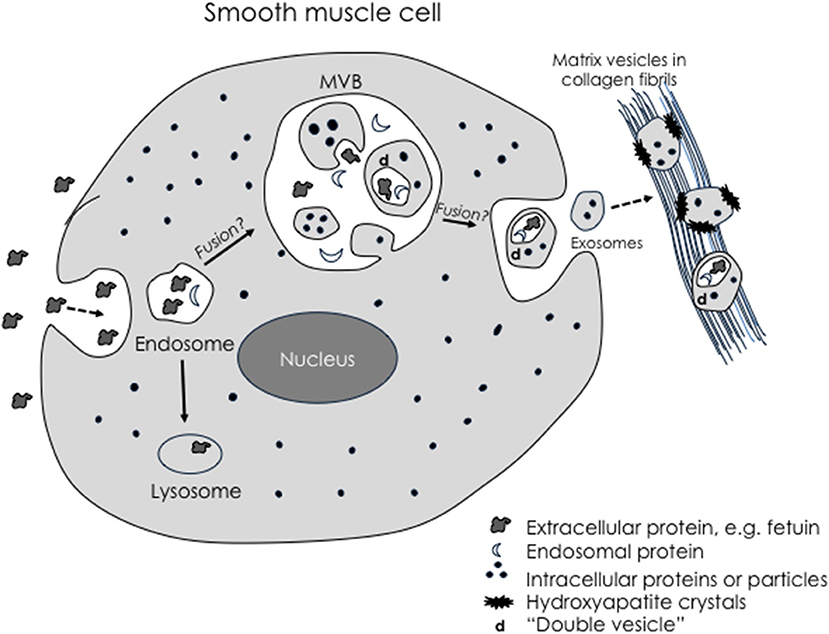
Figure 1. Schematic of possible exosomal biogenesis. Modified from Tintut and Demer (120). Exosomes arise through invagination of the plasma membrane during pinocytosis, which produces endosomes containing extracellular-derived material. Some endosomes may fuse with large multivesicular bodies (MVB). Double membranes are produced when simple microvesicles are formed by a “secondary” evagination of the MVB membrane, which then undergo “tertiary” invagination to engulf extracellular-derived material, creating a double membrane vesicle. When the MVB fuses with the plasma membrane, the microvesicles return to the extracellular space as exosomes. Based on this topological scheme, extracellular particles would be found only in complex exosomes.
Of potential clinical significance, the matrix vesicles produced by vascular smooth muscle cells contain alkaline phosphatase that is responsive to active vitamin D (122). The LDL particles that deposit in the subendothelial space carry inactive vitamin D. The vitamin D activating enzyme (1-alpha-hydroxylase) is produced by smooth muscle cells. Thus, LDL accumulation in atherosclerotic plaque may lead to vitamin-D induced induction of alkaline phosphatase, which promotes calcification and raises questions on the potential cardiovascular effects of vitamin D supplementation (123). Vitamin D has even been used to generate rodent models of vascular calcification (124). While these models do produce robust calcific vasculopathy, questions have been raised about whether the mechanisms reflect those of calcific disease found in human atherosclerosis or chronic kidney disease (CKD).
Potential Targets—Inhibitors Of Lipid-Related Cardiovascular Calcification
Inhibitors of oxidant stress, lipid oxidation, and oxidized lipids have been shown to reduce calcific disease. Omega-3 fatty acids reduce osteogenic gene expression in pharmacologically induced medial calcification (125, 126). Activity of a specific enzymatic inhibitor of lipid oxidation, paraoxonase-1, is lower in patients with CAVD and inversely correlated with the severity of the disease (127). A natural antibody to oxidized phospholipids, E06, attenuates both atherosclerosis, and aortic valve calcification in Ldlr−/− mice on a high-cholesterol diet (128). Pioglitazone, a ligand for peroxisome proliferator-activated receptor-γ, has been shown to inhibit lipid deposition and calcification in aortic valves of hyperlipidemic mice (129). Interestingly, the effect of pioglitazone was unique to the valve, not affecting the aorta. An inhibitor of oxidant stress, fibulin, also reduces osteogenic differentiation of vascular cells (130). A lipid phosphatase, PTEN has been shown to regulate vascular calcification in multiple models (131), and sodium dichloroacetate, a small molecule inhibitor of AKT, induces vascular cell calcification through activation of p38 MAPK, independently of AKT (132). Small molecule inhibitors of autotaxin are considered potential therapeutic agents in cardiovascular calcification, given that PF8380 [6-(3-(piperazin-1-yl)propanoyl)benzo[d]oxazol-2(3H)-one] (a specific inhibitor of autotaxin) reduces LPA levels in small intestine, liver, and plasma (57, 77).
An important consideration in developing a medical therapy for calcific disease of the cardiovascular system is that treatments that reduce calcium mineral deposits may adversely affect skeletal bone. Epidemiological studies show that calcific vasculopathy and osteoporosis are associated in an age-independent manner (34, 133–139). The simultaneous formation of ectopic mineral suggests that calcium and vitamin D intake may not be the limiting factors in bone formation in osteoporosis. It appears that inflammation promotes calcification in soft tissues, such as in artery, lung, breast, and tendon tissues, but it promotes decalcification in skeletal tissues. An active research question is whether widely used osteoporosis treatments, such as bisphosphonates, which inhibit osteoclastic bone resorption, will affect vascular calcification and in what manner they may do so. The one FDA-approved osteoporosis therapy that promotes anabolic bone formation is teriparatide, an active peptide of parathyroid hormone. Its effect on bone depends dramatically on timing of administration. Repeated intermittent administration is anabolic for bone, whereas sustained high levels are catabolic, as seen in the bone loss in clinical hyperparathyroidism and chronic renal disease. A key question is whether intermittent teriparatide is anabolic or catabolic for the mineral formation in the cardiovascular system. Important mouse studies found prevention of atherosclerotic calcification in hyperlipidemic, hyperglycemic mice (140). Sebastian and colleagues went on to show reduction of medial calcification in the context of CKD, where secondary (2°) hyperparathyroidism was blocked by parathyroidectomy (141). However, treatment of aged hyperlipidemic mice with teriparatide also changes the morphology of pre-existing aortic calcium deposits, raising the possibility that it may affect plaque stability (142).
Conclusion
Overall, the regulatory mechanisms underlying calcific vasculopathy and valvulopathy are complex, and phospholipids, lipoproteins, and apolipoproteins are prominent among the mediating factors. While medical therapies for cardiovascular calcification have remained elusive, continued study of lipoprotein-mediated pathways hold promise for identifying effective therapeutic targets.
Author Contributions
All authors listed have made a substantial, direct and intellectual contribution to the work, and approved it for publication.
Funding
This work was supported in part by funding from the National Institutes of Health (HL114709, HL121019, HL007895), the Claude D. Pepper Older American Independence Center (OAIC) at UCLA, and an award from the UCLA Specialty Training and Advanced Research (STAR) Program.
Conflict of Interest Statement
The authors declare that the research was conducted in the absence of any commercial or financial relationships that could be construed as a potential conflict of interest.
References
1. Bostrom K, Watson KE, Horn S, Wortham C, Herman IM, Demer LL. Bone morphogenetic protein expression in human atherosclerotic lesions. J Clin Invest. (1993) 91:1800–9. doi: 10.1172/JCI116391
2. Shanahan CM, Cary NR, Metcalfe JC, Weissberg PL. High expression of genes for calcification-regulating proteins in human atherosclerotic plaques. J Clin Invest. (1994) 93:2393–402. doi: 10.1172/JCI117246
3. Wada T, McKee MD, Steitz S, Giachelli CM. Calcification of vascular smooth muscle cell cultures: inhibition by osteopontin. Circ Res. (1999) 84:166–78. doi: 10.1161/01.RES.84.2.166
4. Tintut Y, Patel J, Parhami F, Demer LL. Tumor necrosis factor-alpha promotes in vitro calcification of vascular cells via the cAMP pathway. Circulation (2000) 102:2636–42. doi: 10.1161/01.CIR.102.21.2636
5. Al-Aly Z, Shao JS, Lai CF, Huang E, Cai J, Behrmann A, et al. Aortic Msx2-Wnt calcification cascade is regulated by TNF-alpha-dependent signals in diabetic Ldlr−/− mice. Arterioscler Thromb Vasc Biol. (2007) 27:2589–96. doi: 10.1161/ATVBAHA.107.153668
6. Lai CF, Shao JS, Behrmann A, Krchma K, Cheng SL, Towler DA. TNFR1-activated reactive oxidative species signals up-regulate osteogenic Msx2 programs in aortic myofibroblasts. Endocrinology (2012) 153:3897–910. doi: 10.1210/en.2012-1216
7. Li X, Lim J, Lu J, Pedego TM, Demer L, Tintut Y. Protective role of Smad6 in inflammation-induced valvular cell calcification. J Cell Biochem. (2015) 116:2354–64. doi: 10.1002/jcb.25186
8. Lim J, Ehsanipour A, Hsu JJ, Lu J, Pedego T, Wu A, et al. Inflammation drives retraction, stiffening, and nodule formation via cytoskeletal machinery in a three-dimensional culture model of aortic stenosis. Am J Pathol. (2016). 186:2378–89. doi: 10.1016/j.ajpath.2016.05.003
9. Tota-Maharaj R, Al-Mallah MH, Nasir K, Qureshi WT, Blumenthal RS, Blaha MJ. Improving the relationship between coronary artery calcium score and coronary plaque burden: addition of regional measures of coronary artery calcium distribution. Atherosclerosis (2015) 238:126–31. doi: 10.1016/j.atherosclerosis.2014.11.008
10. Lin R, Chen S, Liu G, Xue Y, Zhao X. Association between carotid atherosclerotic plaque calcification and intraplaque hemorrhage: a magnetic resonance imaging study. Arterioscler Thromb Vasc Biol. (2017) 37:1228–33. doi: 10.1161/ATVBAHA.116.308360
11. Cowell SJ, Newby DE, Boon NA, Elder AT. Calcific aortic stenosis: same old story? Age Ageing (2004) 33:538–44. doi: 10.1093/ageing/afh175
12. Gondrie MJ, van der Graaf Y, Jacobs PC, Oen AL, Mali WP. The association of incidentally detected heart valve calcification with future cardiovascular events. Eur Radiol. (2011) 21:963–73. doi: 10.1007/s00330-010-1995-0
13. Aikawa E, Nahrendorf M, Figueiredo JL, Swirski FK, Shtatland T, Kohler RH, et al. Osteogenesis associates with inflammation in early-stage atherosclerosis evaluated by molecular imaging in vivo. Circulation (2007) 116:2841–50. doi: 10.1161/CIRCULATIONAHA.107.732867
14. Shanahan CM. Inflammation ushers in calcification: a cycle of damage and protection? Circulation (2007) 116:2782–5. doi: 10.1161/CIRCULATIONAHA.107.749655
15. Scatena M, Liaw L, Giachelli CM. Osteopontin: a multifunctional molecule regulating chronic inflammation and vascular disease. Arterioscler Thromb Vasc Biol. (2007) 27:2302–9. doi: 10.1161/ATVBAHA.107.144824
16. Miller JD, Chu Y, Brooks RM, Richenbacher WE, Pena-Silva R, Heistad DD. Dysregulation of antioxidant mechanisms contributes to increased oxidative stress in calcific aortic valvular stenosis in humans. J Am Coll Cardiol. (2008) 52:843–50. doi: 10.1016/j.jacc.2008.05.043
17. Shao JS, Cheng SL, Sadhu J, Towler DA. Inflammation and the osteogenic regulation of vascular calcification: a review and perspective. Hypertension (2010) 55:579–92. doi: 10.1161/HYPERTENSIONAHA.109.134205
18. Sage AP, Tintut Y, Demer LL. Regulatory mechanisms in vascular calcification. Nat Rev Cardiol. (2010) 7:528–36. doi: 10.1038/nrcardio.2010.115
19. Awan Z, Alrasadi K, Francis GA, Hegele RA, McPherson R, Frohlich J, et al. Vascular calcifications in homozygote familial hypercholesterolemia. Arterioscler Thromb Vasc Biol. (2008) 28:777–85. doi: 10.1161/ATVBAHA.107.160408
20. Alrasadi K, Alwaili K, Awan Z, Valenti D, Couture P, Genest J. Aortic calcifications in familial hypercholesterolemia: potential role of the low-density lipoprotein receptor gene. Am Heart J. (2009) 157:170–6. doi: 10.1016/j.ahj.2008.08.021
21. Hulin A, Hego A, Lancellotti P, Oury C. Advances in pathophysiology of calcific aortic valve disease propose novel molecular therapeutic targets. Front Cardiovasc Med. (2018) 5:21. doi: 10.3389/fcvm.2018.00021
22. Balachandran K, Sucosky P, Jo H, Yoganathan AP. Elevated cyclic stretch induces aortic valve calcification in a bone morphogenic protein-dependent manner. Am J Pathol. (2010) 177:49–57. doi: 10.2353/ajpath.2010.090631
23. Simmons CA, Nikolovski J, Thornton AJ, Matlis S, Mooney DJ. Mechanical stimulation and mitogen-activated protein kinase signaling independently regulate osteogenic differentiation and mineralization by calcifying vascular cells. J Biomech. (2004) 37:1531–41. doi: 10.1016/j.jbiomech.2004.01.006
24. Merryman WD, Schoen FJ. Mechanisms of calcification in aortic valve disease: role of mechanokinetics and mechanodynamics. Curr Cardiol Rep. (2013) 15:355. doi: 10.1007/s11886-013-0355-5
25. Sell S, Scully RE. Aging changes in the aortic and mitral valves. histologic and histochemical studies, with observations on the pathogenesis of calcific aortic stenosis and calcification of the mitral annulus. Am J Pathol. (1965) 46:345–65.
26. Weinberg EJ, Schoen FJ, Mofrad MR. A computational model of aging and calcification in the aortic heart valve. PLoS ONE (2009) 4:e5960. doi: 10.1371/journal.pone.0005960
27. Hoshino T, Chow LA, Hsu JJ, Perlowski AA, Abedin M, Tobis J, et al. Mechanical stress analysis of a rigid inclusion in distensible material: a model of atherosclerotic calcification and plaque vulnerability. Am J Physiol Heart Circ Physiol. (2009) 297:H802–10. doi: 10.1152/ajpheart.00318.2009
28. Roy-Cardinal MH, Destrempes F, Soulez G, Cloutier G. Assessment of carotid artery plaque components with machine learning classification using homodyned-K parametric maps and elastograms. IEEE Trans Ultrason Ferroelectr Freq Control (2018). doi: 10.1109/TUFFC.2018.2851846. [Epub ahead of print].
29. Orakzai SH, Nasir K, Blaha M, Blumenthal RS, Raggi P. Non-HDL cholesterol is strongly associated with coronary artery calcification in asymptomatic individuals. Atherosclerosis (2009) 202:289–95. doi: 10.1016/j.atherosclerosis.2008.03.014
30. Tsao CW, Preis SR, Peloso GM, Hwang SJ, Kathiresan S, Fox CS, et al. Relations of long-term and contemporary lipid levels and lipid genetic risk scores with coronary artery calcium in the framingham heart study. J Am Coll Cardiol. (2012) 60:2364–71. doi: 10.1016/j.jacc.2012.09.007
31. Lardenoye JH, de Vries MR, Lowik CW, Xu Q, Dhore CR, Cleutjens JP, et al. Accelerated atherosclerosis and calcification in vein grafts: a study in APOE*3 Leiden transgenic mice. Circ Res. (2002) 91:577–84. doi: 10.1161/01.RES.0000036901.58329.D7
32. Vorkas PA, Isaac G, Holmgren A, Want EJ, Shockcor JP, Holmes E, et al. Perturbations in fatty acid metabolism and apoptosis are manifested in calcific coronary artery disease: an exploratory lipidomic study. Int J Cardiol. (2015) 197:192–9. doi: 10.1016/j.ijcard.2015.06.048
33. Liu M, Luo M, Sun H, Ni B, Shao Y. Integrated bioinformatics analysis predicts the key genes involved in aortic valve calcification: from hemodynamic changes to extracellular remodeling. Tohoku J Exp Med. (2017) 243:263–73. doi: 10.1620/tjem.243.263
34. Parhami F, Morrow AD, Balucan J, Leitinger N, Watson AD, Tintut Y, et al. Lipid oxidation products have opposite effects on calcifying vascular cell and bone cell differentiation. A possible explanation for the paradox of arterial calcification in osteoporotic patients. Arterioscler Thromb Vasc Biol. (1997) 17:680–7. doi: 10.1161/01.ATV.17.4.680
35. Proudfoot D, Davies JD, Skepper JN, Weissberg PL, Shanahan CM Acetylated low-density lipoprotein stimulates human vascular smooth muscle cell calcification by promoting osteoblastic differentiation and inhibiting phagocytosis. Circulation (2002) 106:3044–50. doi: 10.1161/01.CIR.0000041429.83465.41
36. Olsson M, Thyberg J, Nilsson J. Presence of oxidized low density lipoprotein in nonrheumatic stenotic aortic valves. Arterioscler Thromb Vasc Biol. (1999) 19:1218–22. doi: 10.1161/01.ATV.19.5.1218
37. Berliner JA, Navab M, Fogelman AM, Frank JS, Demer LL, Edwards PA, et al. Atherosclerosis: basic mechanisms. Oxidation, inflammation, and genetics. Circulation (1995) 91:2488–96. doi: 10.1161/01.CIR.91.9.2488
38. Proudfoot D, Skepper JN, Hegyi L, Bennett MR, Shanahan CM, Weissberg PL. Apoptosis regulates human vascular calcification in vitro: evidence for initiation of vascular calcification by apoptotic bodies. Circ Res. (2000) 87:1055–62. doi: 10.1161/01.RES.87.11.1055
39. Chaudiere J, Derouette JC, Mendy F, Jacotot B, Robert L. In vitro preparation of elastin–triglyceride complexes. Fatty acid uptake and modification of the susceptibility to elastase action. Atherosclerosis (1980) 36:183–94. doi: 10.1016/0021-9150(80)90227-0
40. Srinivasan SR, Yost C, Radhakrishnamurthy B, Dalferes ER Jr Berenson GS. Lipoprotein-elastin interactions in human aorta fibrous plaque lesions. Atherosclerosis (1981) 38:137–47. doi: 10.1016/0021-9150(81)90111-8
41. Kim KM, Valigorsky JM, Mergner WJ, Jones RT, Pendergrass RF, Trump BF. Aging changes in the human aortic valve in relation to dystrophic calcification. Hum Pathol. (1976) 7:47–60. doi: 10.1016/S0046-8177(76)80005-6
42. O'Brien KD, Reichenbach DD, Marcovina SM, Kuusisto J, Alpers CE, Otto CM. Apolipoproteins B, (a), and E accumulate in the morphologically early lesion of 'degenerative' valvular aortic stenosis. Arterioscler Thromb Vasc Biol. (1996) 16:523–32. doi: 10.1161/01.ATV.16.4.523
43. Graham LS, Parhami F, Tintut Y, Kitchen CM, Demer LL, Effros RB. Oxidized lipids enhance RANKL production by T lymphocytes: implications for lipid-induced bone loss. Clin Immunol. (2009) 133:265–75. doi: 10.1016/j.clim.2009.07.011
44. Graham LS, Tintut Y, Parhami F, Kitchen CM, Ivanov Y, Tetradis S, et al. Bone density and hyperlipidemia: the T-lymphocyte connection. J Bone Miner Res. (2010) 25:2460–9. doi: 10.1002/jbmr.148
45. Olsson M, Dalsgaard CJ, Haegerstrand A, Rosenqvist M, Ryden L, Nilsson J. Accumulation of T lymphocytes and expression of interleukin-2 receptors in nonrheumatic stenotic aortic valves. J Am Coll Cardiol. (1994) 23:1162–70. doi: 10.1016/0735-1097(94)90606-8
46. Olsson M, Rosenqvist M, Nilsson J. Expression of HLA-DR antigen and smooth muscle cell differentiation markers by valvular fibroblasts in degenerative aortic stenosis. J Am Coll Cardiol. (1994) 24:1664–71. doi: 10.1016/0735-1097(94)90172-4
47. Otto CM, Kuusisto J, Reichenbach DD, Gown AM, O'Brien KD. Characterization of the early lesion of 'degenerative' valvular aortic stenosis. Histological and immunohistochemical studies. Circulation (1994) 90:844–53. doi: 10.1161/01.CIR.90.2.844
48. Abrahamov A, Elstein D, Gross-Tsur V, Farber B, Glaser Y, Hadas-Halpern I, et al. Gaucher's disease variant characterised by progressive calcification of heart valves and unique genotype. Lancet (1995) 346:1000–3. doi: 10.1016/S0140-6736(95)91688-1
49. Dunmore-Buyze J, Boughner DR, Macris N, Vesely I. A comparison of macroscopic lipid content within porcine pulmonary and aortic valves. Implications for bioprosthetic valves. J Thorac Cardiovasc Surg. (1995) 110:1756–61. doi: 10.1016/S0022-5223(95)70039-0
50. Thanassoulis G, Campbell CY, Owens DS, Smith JG, Smith AV, Peloso GM, et al. Genetic associations with valvular calcification and aortic stenosis. N Engl J Med. (2013) 368:503–12. doi: 10.1056/NEJMoa1109034
51. Khera AV, Everett BM, Caulfield MP, Hantash FM, Wohlgemuth J, Ridker PM, et al. Lipoprotein(a) concentrations, rosuvastatin therapy, and residual vascular risk: an analysis from the JUPITER Trial (Justification for the Use of Statins in Prevention: an Intervention Trial Evaluating Rosuvastatin). Circulation (2014) 129:635–42. doi: 10.1161/CIRCULATIONAHA.113.004406
52. Antonopoulos S, Mylonopoulou M, Angelidi AM, Kousoulis AA, Tentolouris N. Association of matrix gamma-carboxyglutamic acid protein levels with insulin resistance and Lp(a) in diabetes: a cross-sectional study. Diabetes Res Clin Pract. (2017) 130:252–7. doi: 10.1016/j.diabres.2017.06.015
53. Ida J, Kotani K, Miyoshi T, Nakamura K, Kohno K, Asonuma H, et al. High baseline lipoprotein(a) level as a risk factor for coronary artery calcification progression: sub-analysis of a prospective multicenter trial. Acta Med Okayama (2018) 72:223–30. doi: 10.18926/AMO/56067
54. Nsaibia MJ, Boulanger MC, Bouchareb R, Mkannez G, Le Quang K, Hadji F, et al. OxLDL-derived lysophosphatidic acid promotes the progression of aortic valve stenosis through a LPAR1-RhoA-NF-kappaB pathway. Cardiovasc Res. (2017) 113:1351–63. doi: 10.1093/cvr/cvx089
55. Bergmark C, Dewan A, Orsoni A, Merki E, Miller ER, Shin MJ, et al. A novel function of lipoprotein [a] as a preferential carrier of oxidized phospholipids in human plasma. J Lipid Res. (2008) 49:2230–9. doi: 10.1194/jlr.M800174-JLR200
56. Bouchareb R, Mahmut A, Nsaibia MJ, Boulanger MC, Dahou A, Lepine JL, et al. Autotaxin derived from lipoprotein(a) and valve interstitial cells promotes inflammation and mineralization of the aortic valve. Circulation (2015) 132:677–90. doi: 10.1161/CIRCULATIONAHA.115.016757
57. Navab M, Chattopadhyay A, Hough G, Meriwether D, Fogelman SI, Wagner AC, et al. Source and role of intestinally derived lysophosphatidic acid in dyslipidemia and atherosclerosis. J Lipid Res. (2015) 56:871–87. doi: 10.1194/jlr.M056614
58. Sun W, Li H, Yu Y, Fan Y, Grabiner BC, Mao R, et al. MEKK3 is required for lysophosphatidic acid-induced NF-kappaB activation. Cell Signal. (2009) 21:1488–94. doi: 10.1016/j.cellsig.2009.05.007
59. Barbayianni E, Kaffe E, Aidinis V, Kokotos G. Autotaxin, a secreted lysophospholipase D, as a promising therapeutic target in chronic inflammation and cancer. Prog Lipid Res. (2015) 58:76–96. doi: 10.1016/j.plipres.2015.02.001
60. Benesch MG, Ko YM, McMullen TP, Brindley DN. Autotaxin in the crosshairs: taking aim at cancer and other inflammatory conditions. FEBS Lett. (2014) 588:2712–27. doi: 10.1016/j.febslet.2014.02.009
61. Sevastou I, Kaffe E, Mouratis MA, Aidinis V. Lysoglycerophospholipids in chronic inflammatory disorders: the PLA(2)/LPC and ATX/LPA axes. Biochim Biophys Acta (2013) 1831:42–60. doi: 10.1016/j.bbalip.2012.07.019
62. Fotopoulou S, Oikonomou N, Grigorieva E, Nikitopoulou I, Paparountas T, Thanassopoulou A, et al. ATX expression and LPA signalling are vital for the development of the nervous system. Dev Biol. (2010) 339:451–64. doi: 10.1016/j.ydbio.2010.01.007
63. Tanaka M, Okudaira S, Kishi Y, Ohkawa R, Iseki S, Ota M, et al. Autotaxin stabilizes blood vessels and is required for embryonic vasculature by producing lysophosphatidic acid. J Biol Chem. (2006) 281:25822–30. doi: 10.1074/jbc.M605142200
64. van Meeteren LA, Ruurs P, Stortelers C, Bouwman P, van Rooijen MA, Pradere JP, et al. Autotaxin, a secreted lysophospholipase D, is essential for blood vessel formation during development. Mol Cell Biol. (2006) 26:5015–22. doi: 10.1128/MCB.02419-05
65. Nakanaga K, Hama K, Aoki J. Autotaxin–an LPA producing enzyme with diverse functions. J Biochem. (2010) 148:13–24. doi: 10.1093/jb/mvq052
66. Jansen S, Perrakis A, Ulens C, Winkler C, Andries M, Joosten RP, et al. Structure of NPP1, an ectonucleotide pyrophosphatase/phosphodiesterase involved in tissue calcification. Structure (2012) 20:1948–59. doi: 10.1016/j.str.2012.09.001
67. Rutsch F, Nitschke Y, Terkeltaub R. Genetics in arterial calcification: pieces of a puzzle and cogs in a wheel. Circ Res. (2011) 109:578–92. doi: 10.1161/CIRCRESAHA.111.247965
68. Jansen S, Andries M, Vekemans K, Vanbilloen H, Verbruggen A, Bollen M. Rapid clearance of the circulating metastatic factor autotaxin by the scavenger receptors of liver sinusoidal endothelial cells. Cancer Lett. (2009) 284:216–21. doi: 10.1016/j.canlet.2009.04.029
69. Venkatraman G, Benesch MG, Tang X, Dewald J, McMullen TP, Brindley DN. Lysophosphatidate signaling stabilizes Nrf2 and increases the expression of genes involved in drug resistance and oxidative stress responses: implications for cancer treatment. FASEB J. (2015) 29:772–85. doi: 10.1096/fj.14-262659
70. Keune WJ, Hausmann J, Bolier R, Tolenaars D, Kremer A, Heidebrecht T, et al. Steroid binding to Autotaxin links bile salts and lysophosphatidic acid signalling. Nat Commun. (2016) 7:11248. doi: 10.1038/ncomms11248
71. Lawrie A, Hameed AG, Chamberlain J, Arnold N, Kennerley A, Hopkinson K, et al. Paigen diet-fed apolipoprotein E knockout mice develop severe pulmonary hypertension in an interleukin-1-dependent manner. Am J Pathol. (2011) 179:1693–705. doi: 10.1016/j.ajpath.2011.06.037
72. Liu S, Umezu-Goto M, Murph M, Lu Y, Liu W, Zhang F, et al. Expression of autotaxin and lysophosphatidic acid receptors increases mammary tumorigenesis, invasion, and metastases. Cancer Cell (2009) 15:539–50. doi: 10.1016/j.ccr.2009.03.027
73. Bourgoin SG, Zhao C. Autotaxin and lysophospholipids in rheumatoid arthritis. Curr Opin Investig Drugs (2010) 11:515–26.
74. Tager AM. Autotaxin emerges as a therapeutic target for idiopathic pulmonary fibrosis: limiting fibrosis by limiting lysophosphatidic acid synthesis. Am J Respir Cell Mol Biol. (2012) 47:563–5. doi: 10.1165/rcmb.2012-0235ED
75. Benesch MG, Tang X, Dewald J, Dong WF, Mackey JR, Hemmings DG, et al. Tumor-induced inflammation in mammary adipose tissue stimulates a vicious cycle of autotaxin expression and breast cancer progression. FASEB J. (2015) 29:3990–4000. doi: 10.1096/fj.15-274480
76. Benesch MG, Tang X, Maeda T, Ohhata A, Zhao YY, Kok BP, et al. Inhibition of autotaxin delays breast tumor growth and lung metastasis in mice. FASEB J. (2014) 28:2655–66. doi: 10.1096/fj.13-248641
77. Gierse J, Thorarensen A, Beltey K, Bradshaw-Pierce E, Cortes-Burgos L, Hall T, et al. A novel autotaxin inhibitor reduces lysophosphatidic acid levels in plasma and the site of inflammation. J Pharmacol Exp Ther. (2010) 334:310–7. doi: 10.1124/jpet.110.165845
78. Leblanc R, Lee SC, David M, Bordet JC, Norman DD, Patil R, et al. Interaction of platelet-derived autotaxin with tumor integrin alphaVbeta3 controls metastasis of breast cancer cells to bone. Blood (2014) 124:3141–50. doi: 10.1182/blood-2014-04-568683
79. Lee SC, Fujiwara Y, Liu J, Yue J, Shimizu Y, Norman DD, et al. Autotaxin and LPA1 and LPA5 receptors exert disparate functions in tumor cells versus the host tissue microenvironment in melanoma invasion and metastasis. Mol Cancer Res. (2015) 13:174–85. doi: 10.1158/1541-7786.MCR-14-0263
80. Oikonomou N, Mouratis MA, Tzouvelekis A, Kaffe E, Valavanis C, Vilaras G, et al. Pulmonary autotaxin expression contributes to the pathogenesis of pulmonary fibrosis. Am J Respir Cell Mol Biol. (2012) 47:566–74. doi: 10.1165/rcmb.2012-0004OC
81. Zhang H, Xu X, Gajewiak J, Tsukahara R, Fujiwara Y, Liu J, et al. Dual activity lysophosphatidic acid receptor pan-antagonist/autotaxin inhibitor reduces breast cancer cell migration in vitro and causes tumor regression in vivo. Cancer Res. (2009) 69:5441–9. doi: 10.1158/0008-5472.CAN-09-0302
82. Katsifa A, Kaffe E, Nikolaidou-Katsaridou N, Economides AN, Newbigging S, McKerlie C, et al. The bulk of autotaxin activity is dispensable for adult mouse life. PLoS ONE (2015) 10:e0143083. doi: 10.1371/journal.pone.0143083
83. Miller JD, Weiss RM, Serrano KM, Brooks RM II, Berry CJ, Zimmerman K, et al. Lowering plasma cholesterol levels halts progression of aortic valve disease in mice. Circulation (2009) 119:2693–701. doi: 10.1161/CIRCULATIONAHA.108.834614
84. Chan KL, Teo K, Dumesnil JG, Ni A, Tam J, Investigators A. Effect of Lipid lowering with rosuvastatin on progression of aortic stenosis: results of the aortic stenosis progression observation: measuring effects of rosuvastatin (ASTRONOMER) trial. Circulation (2010) 121:306–14. doi: 10.1161/CIRCULATIONAHA.109.900027
85. Yeang C, Hung MY, Byun YS, Clopton P, Yang X, Witztum JL, et al. Effect of therapeutic interventions on oxidized phospholipids on apolipoprotein B100 and lipoprotein(a). J Clin Lipidol. (2016) 10:594–603. doi: 10.1016/j.jacl.2016.01.005
86. Sima A, Popov D, Starodub O, Stancu C, Cristea C, Stern D, et al. Pathobiology of the heart in experimental diabetes: immunolocalization of lipoproteins, immunoglobulin G, and advanced glycation endproducts proteins in diabetic and/or hyperlipidemic hamster. Lab Invest. (1997) 77:3–18.
87. Li H, Tao HR, Hu T, Fan YH, Zhang RQ, Jia G, Wang HC. Atorvastatin reduces calcification in rat arteries and vascular smooth muscle cells. Basic Clin Pharmacol Toxicol. (2010) 107:798–802. doi: 10.1111/j.1742-7843.2010.00580.x
88. Callister TQ, Raggi P, Cooil B, Lippolis NJ, Russo DJ. Effect of HMG-CoA reductase inhibitors on coronary artery disease as assessed by electron-beam computed tomography. N Engl J Med. (1998) 339:1972–8. doi: 10.1056/NEJM199812313392703
89. Achenbach S, Ropers D, Pohle K, Leber A, Thilo C, Knez A, et al. Influence of lipid-lowering therapy on the progression of coronary artery calcification: a prospective evaluation. Circulation (2002) 106:1077–82. doi: 10.1161/01.CIR.0000027567.49283.FF
90. Nsaibia MJ, Mahmut A, Mahjoub H, Dahou A, Bouchareb R, Boulanger MC, et al. Association between plasma lipoprotein levels and bioprosthetic valve structural degeneration. Heart (2016) 102:1915–21. doi: 10.1136/heartjnl-2016-309541
91. Vyavahare N, Hirsch D, Lerner E, Baskin JZ, Schoen FJ, Bianco R, et al. Prevention of bioprosthetic heart valve calcification by ethanol preincubation. Efficacy and mechanisms. Circulation (1997) 95:479–88. doi: 10.1161/01.CIR.95.2.479
92. Jorge-Herrero E, Fernandez P, Gutierrez M, Castillo-Olivares JL. Study of the calcification of bovine pericardium: analysis of the implication of lipids and proteoglycans. Biomaterials (1991) 12:683–9. doi: 10.1016/0142-9612(91)90117-S
93. Rossi MA, Braile DM, Teixeira MD, Souza DR, Peres LC. Lipid extraction attenuates the calcific degeneration of bovine pericardium used in cardiac valve bioprostheses. J Exp Pathol (Oxford). (1990) 71:187–96.
94. Gilmanov D, Bevilacqua S, Mazzone A, Glauber M. Do statins slow the process of calcification of aortic tissue valves? Interact Cardiovasc Thorac Surg. (2010) 11:297–301. doi: 10.1510/icvts.2009.230920
95. Anand DV, Lim E, Darko D, Bassett P, Hopkins D, Lipkin D, et al. Determinants of progression of coronary artery calcification in type (2007) 2 diabetes role of glycemic control and inflammatory/vascular calcification markers. J Am Coll Cardiol. 50:2218–25. doi: 10.1016/j.jacc.2007.08.032
96. Saremi A, Bahn G, Reaven PD, Investigators VADT. Progression of vascular calcification is increased with statin use in the Veterans Affairs Diabetes Trial (VADT). Diabetes Care (2012) 35:2390–2. doi: 10.2337/dc12-0464
97. Puri R, Nicholls SJ, Shao M, Kataoka Y, Uno K, Kapadia SR, et al. Impact of statins on serial coronary calcification during atheroma progression and regression. J Am Coll Cardiol. (2015) 65:1273–82. doi: 10.1016/j.jacc.2015.01.036
98. Mujaj B, Bos D, Selwaness M, Leening MJG, Kavousi M, Wentzel JJ, et al. Statin use is associated with carotid plaque composition: the Rotterdam Study. Int J Cardiol. (2018) 260:213–8. doi: 10.1016/j.ijcard.2018.02.111
99. Lee SE, Chang HJ, Sung JM, Park HB, Heo R, Rizvi A, et al. Effects of statins on coronary atherosclerotic plaques: the PARADIGM (Progression of AtheRosclerotic PlAque DetermIned by Computed TomoGraphic Angiography Imaging) study. JACC Cardiovasc Imaging (2018). 11:1475–84. doi: 10.1016/j.jcmg.2018.04.015
100. Ikegami Y, Inoue I, Inoue K, Shinoda Y, Iida S, Goto S, et al. The annual rate of coronary artery calcification with combination therapy with a PCSK9 inhibitor and a statin is lower than that with statin monotherapy. NPJ Aging Mech Dis. (2018) 4:7. doi: 10.1038/s41514-018-0026-2
101. Trion A, Schutte-Bart C, Bax WH, Jukema JW, van der Laarse A. Modulation of calcification of vascular smooth muscle cells in culture by calcium antagonists, statins, and their combination. Mol Cell Biochem. (2008) 308:25–33. doi: 10.1007/s11010-007-9608-1
102. Reid DG, Shanahan CM, Duer MJ, Arroyo LG, Schoppet M, Brooks RA, et al. Lipids in biocalcification: contrasts and similarities between intimal and medial vascular calcification and bone by NMR. J Lipid Res. (2012) 53:1569–75. doi: 10.1194/jlr.M026088
103. Boskey AL, Ullrich W, Spevak L, Gilder H. Persistence of complexed acidic phospholipids in rapidly mineralizing tissues is due to affinity for mineral and resistance to hydrolytic attack: in vitro data. Calcif Tissue Int. (1996) 58:45–51. doi: 10.1007/BF02509545
104. Boyan BD, Boskey AL. Co-isolation of proteolipids and calcium-phospholipid-phosphate complexes. Calcif Tissue Int. (1984) 36:214–8. doi: 10.1007/BF02405320
105. Dmitrovsky E, Boskey AL. Calcium-acidic phospholipid-phosphate complexes in human atherosclerotic aortas. Calcif Tissue Int. (1985) 37:121–5. doi: 10.1007/BF02554830
106. Bottini M, Mebarek S, Anderson KL, Strzelecka-Kiliszek A, Bozycki L, Simao AMS, et al. Matrix vesicles from chondrocytes and osteoblasts: Their biogenesis, properties, functions and biomimetic models. Biochim Biophys Acta (2018) 1862:532–46. doi: 10.1016/j.bbagen.2017.11.005
108. Oorni K, Pentikainen MO, Ala-Korpela M, Kovanen PT. Aggregation, fusion, and vesicle formation of modified low density lipoprotein particles: molecular mechanisms and effects on matrix interactions. J Lipid Res. (2000) 41:1703–14.
109. Sodar BW, Kittel A, Paloczi K, Vukman KV, Osteikoetxea X, Szabo-Taylor K, et al. Low-density lipoprotein mimics blood plasma-derived exosomes and microvesicles during isolation and detection. Sci Rep. (2016) 6:24316. doi: 10.1038/srep24316
110. Zaborowski MP, Balaj L, Breakefield XO, Lai CP. Extracellular vesicles: composition, biological relevance, and methods of study. Bioscience (2015) 65:783–97. doi: 10.1093/biosci/biv084
111. Anderson HC, Mulhall D, Garimella R. Role of extracellular membrane vesicles in the pathogenesis of various diseases, including cancer, renal diseases, atherosclerosis, and arthritis. Lab Invest. (2010) 90:1549–57. doi: 10.1038/labinvest.2010.152
112. Roszkowska M, Strzelecka-Kiliszek A, Bessueille L, Buchet R, Magne D, Pikula S. Collagen promotes matrix vesicle-mediated mineralization by vascular smooth muscle cells. J Inorg Biochem. (2018) 186:1–9. doi: 10.1016/j.jinorgbio.2018.05.007
113. Kim KM Calcification of matrix vesicles in human aortic valve and aortic media. Fed Proc. (1976) 35:156–62.
114. New SE, Goettsch C, Aikawa M, Marchini JF, Shibasaki M, Yabusaki K, et al. Macrophage-derived matrix vesicles: an alternative novel mechanism for microcalcification in atherosclerotic plaques. Circ Res. (2013) 113:72–7. doi: 10.1161/CIRCRESAHA.113.301036
115. Cui L, Houston DA, Farquharson C, MacRae VE. Characterisation of matrix vesicles in skeletal and soft tissue mineralisation. Bone (2016) 87:147–58. doi: 10.1016/j.bone.2016.04.007
116. Lin Z, McClure MJ, Zhao J, Ramey AN, Asmussen N, Hyzy SL, et al. MicroRNA contents in matrix vesicles produced by growth plate chondrocytes are cell maturation dependent. Sci Rep. (2018) 8:3609. doi: 10.1038/s41598-018-21517-4
117. Kapustin AN, Chatrou ML, Drozdov I, Zheng Y, Davidson SM, Soong D, et al. Vascular smooth muscle cell calcification is mediated by regulated exosome secretion. Circ Res. (2015) 116:1312–23. doi: 10.1161/CIRCRESAHA.116.305012
118. Shapiro IM, Landis WJ, Risbud MV. Matrix vesicles: are they anchored exosomes? Bone (2015) 79:29–36. doi: 10.1016/j.bone.2015.05.013
119. Guyton JR, Klemp KF. Ultrastructural discrimination of lipid droplets and vesicles in atherosclerosis: value of osmium-thiocarbohydrazide-osmium and tannic acid-paraphenylenediamine techniques. J Histochem Cytochem. (1988) 36:1319–28. doi: 10.1177/36.10.2458408
120. Tintut Y, Demer LL. Exosomes: nanosized cellular messages. Circ Res. (2015) 116:1281–3. doi: 10.1161/CIRCRESAHA.115.306324
121. Boonrungsiman S, Gentleman E, Carzaniga R, Evans ND, McComb DW, Porter AE, et al. The role of intracellular calcium phosphate in osteoblast-mediated bone apatite formation. Proc Natl Acad Sci USA. (2012) 109:14170–5. doi: 10.1073/pnas.1208916109
122. Boyan BD, Schwartz Z, Bonewald LF, Swain LD. Localization of 1,25-(OH)2D3-responsive alkaline phosphatase in osteoblast-like cells (ROS 17/2.8, MG 63, and MC 3T3) and growth cartilage cells in culture. J Biol Chem. (1989) 264:11879–86.
123. Demer LL, Hsu JJ, Tintut Y. Steroid Hormone vitamin d: implications for cardiovascular disease. Circ Res. (2018) 122:1576–85. doi: 10.1161/CIRCRESAHA.118.311585
124. Hsu JJ, Tintut Y, Demer LL. Vitamin D and osteogenic differentiation in the artery wall. Clin J Am Soc Nephrol. (2008) 3:1542–7. doi: 10.2215/CJN.01220308
125. Kanai S, Uto K, Honda K, Hagiwara N, Oda H. Eicosapentaenoic acid reduces warfarin-induced arterial calcification in rats. Atherosclerosis (2011) 215:43–51. doi: 10.1016/j.atherosclerosis.2010.12.001
126. Abedin M, Lim J, Tang TB, Park D, Demer LL, Tintut Y. N-3 fatty acids inhibit vascular calcification via the p38-mitogen-activated protein kinase and peroxisome proliferator-activated receptor-gamma pathways. Circ Res. (2006) 98:727–9. doi: 10.1161/01.RES.0000216009.68958.e6
127. Cagirci G, Cay S, Karakurt O, Durmaz T, Yazihan N, Canga A, et al. Paraoxonase activity might be predictive of the severity of aortic valve stenosis. J Heart Valve Dis. (2010) 19:453–8.
128. Que X, Hung MY, Yeang C, Gonen A, Prohaska TA, Sun X, et al. Oxidized phospholipids are proinflammatory and proatherogenic in hypercholesterolaemic mice. Nature (2018) 558:301–6. doi: 10.1038/s41586-018-0198-8
129. Chu Y, Lund DD, Weiss RM, Brooks RM, Doshi H, Hajj GP, et al. Pioglitazone attenuates valvular calcification induced by hypercholesterolemia. Arterioscler Thromb Vasc Biol. (2013) 33:523–32. doi: 10.1161/ATVBAHA.112.300794
130. Luong TTD, Schelski N, Boehme B, Makridakis M, Vlahou A, Lang F, et al. Fibulin-3 attenuates phosphate-induced vascular smooth muscle cell calcification by inhibition of oxidative stress. Cell Physiol Biochem. (2018) 46:1305–16. doi: 10.1159/000489144
131. Deng L, Huang L, Sun Y, Heath JM, Wu H, Chen Y. Inhibition of FOXO1/3 promotes vascular calcification. Arterioscler Thromb Vasc Biol. (2015) 35:175–83. doi: 10.1161/ATVBAHA.114.304786
132. Yang Y, Sun Y, Chen J, Bradley WE, Dell'Italia LJ, Wu H, et al. AKT-independent activation of p38 MAP kinase promotes vascular calcification. Redox Biol. (2018) 16:97–103. doi: 10.1016/j.redox.2018.02.009
133. De Schutter TM, Neven E, Persy VP, Behets GJ, Postnov AA, De Clerck NM, et al. Vascular calcification is associated with cortical bone loss in chronic renal failure rats with and without ovariectomy: the calcification paradox. Am J Nephrol. (2011) 34:356–66. doi: 10.1159/000331056
134. Frye MA, Melton LJ III, Bryant SC, Fitzpatrick LA, Wahner HW, Schwartz RS, et al. Osteoporosis and calcification of the aorta. Bone Miner (1992) 19:185–94. doi: 10.1016/0169-6009(92)90925-4
135. Hak AE, Pols HA, van Hemert AM, Hofman A, Witteman JC. Progression of aortic calcification is associated with metacarpal bone loss during menopause: a population-based longitudinal study. Arterioscler Thromb Vasc Biol. (2000) 20:1926–31. doi: 10.1161/01.ATV.20.8.1926
136. Hjortnaes J, Butcher J, Figueiredo JL, Riccio M, Kohler RH, Kozloff KM, et al. Arterial and aortic valve calcification inversely correlates with osteoporotic bone remodelling: a role for inflammation. Eur Heart J. (2010) 31:1975–84. doi: 10.1093/eurheartj/ehq237
137. Kiel DP, Kauppila LI, Cupples LA, Hannan MT, O'Donnell CJ, Wilson PW. Bone loss and the progression of abdominal aortic calcification over a (2001) 25 year period: the Framingham Heart Study. Calcif Tissue Int. 68:271–6. doi: 10.1007/BF02390833
138. Persy V, D'Haese P. Vascular calcification and bone disease: the calcification paradox. Trends Mol Med. (2009) 15:405–16. doi: 10.1016/j.molmed.2009.07.001
139. Schulz E, Arfai K, Liu X, Sayre J, Gilsanz V. Aortic calcification and the risk of osteoporosis and fractures. J Clin Endocrinol Metab. (2004) 89:4246–53. doi: 10.1210/jc.2003-030964
140. Shao JS, Cheng SL, Charlton-Kachigian N, Loewy AP, Towler DA. Teriparatide (human parathyroid hormone (1-34)) inhibits osteogenic vascular calcification in diabetic low density lipoprotein receptor-deficient mice. J Biol Chem. (2003) 278:50195–202. doi: 10.1074/jbc.M308825200
141. Sebastian EM, Suva LJ, Friedman PA. Differential effects of intermittent PTH(1-34) and PTH(7-34) on bone microarchitecture and aortic calcification in experimental renal failure. Bone (2008) 43:1022–30. doi: 10.1016/j.bone.2008.07.250
Keywords: lipoproteins, calcification, Lp(a), autotaxin, osteogenesis
Citation: Tintut Y, Hsu JJ and Demer LL (2018) Lipoproteins in Cardiovascular Calcification: Potential Targets and Challenges. Front. Cardiovasc. Med. 5:172. doi: 10.3389/fcvm.2018.00172
Received: 23 August 2018; Accepted: 08 November 2018;
Published: 23 November 2018.
Edited by:
Dwight A. Towler, University of Texas Southwestern Medical Center, United StatesReviewed by:
Alexander N. Kapustin, AstraZeneca, United KingdomSasha Anna Singh, Harvard Medical School, United States
Copyright © 2018 Tintut, Hsu and Demer. This is an open-access article distributed under the terms of the Creative Commons Attribution License (CC BY). The use, distribution or reproduction in other forums is permitted, provided the original author(s) and the copyright owner(s) are credited and that the original publication in this journal is cited, in accordance with accepted academic practice. No use, distribution or reproduction is permitted which does not comply with these terms.
*Correspondence: Linda L. Demer, TERlbWVyQG1lZG5ldC51Y2xhLmVkdQ==