Translational Models of Arrhythmia Mechanisms and Susceptibility: Success and Challenges of Modeling Human Disease
- Department of Emergency Medicine, Emergency Care Research Institute and Heart and Vascular Research Center, MetroHealth Campus of Case Western Reserve University, Cleveland, OH, United States
We discuss large animal translational models of arrhythmia susceptibility and sudden cardiac death, focusing on important considerations when interpreting the data derived before applying them to human trials. The utility of large animal models of arrhythmia and the pros and cons of specific translational large animals used will be discussed, including the necessary tradeoffs between models designed to derive mechanisms vs. those to test therapies. Recent technical advancements which can be applied to large animal models of arrhythmias to better elucidate mechanistic insights will be introduced. Finally, some specific examples of past successes and challenges in translating the results of large animal models of arrhythmias to clinical trials and practice will be examined, and common themes regarding the success and failure of translating studies to therapy in man will be discussed.
Introduction
Improved approaches to prevent and treat arrhythmia are of major importance, as sudden cardiac death (SCD) is a major cause of mortality (1, 2). In 2014 in the United States, over 600,000 deaths were caused by heart disease, with half of those being sudden. Ventricular fibrillation/tachycardia (VF/VT) secondary to acute myocardial ischemia or infarction (MI) is devastating, with many patients not reaching the hospital and most of those who do suffering poor outcomes (3). Therefore, improved understanding of both mechanism and novel therapies for arrhythmias derived from translational models, which can be readily applied to humans, is of utmost importance.
There are multiple large animal models used to assess arrhythmia susceptibility, mechanisms of arrhythmogenesis, and therapies for arrhythmia and SCD. In this review we will focus on large animal models designed to derive mechanisms for arrhythmias and SCD as well to test therapies in translational models to provide evidence of efficacy and the bases for clinical trials. We will discuss the pros and cons of animal models using the primary large animal platforms in arrhythmia and SCD research: dog, pig, goat, and sheep. Although smaller animal models of arrhythmia susceptibility such as rabbit models of heart failure (HF) (4) and murine models of ischemia/reperfusion (5) have contributed significantly to our knowledge of arrhythmia mechanisms, this review will focus on large vertebrate models most readily translatable to human therapies.
Why Use Large Animal Models and What Is Their Role?
Large animal models are often used to determine the relevance of previously derived mechanisms in ex vivo or in small animal models. This is an important step in understanding how molecular and cellular mechanisms contribute to arrhythmias in man and oftentimes crucial, as the relevance of mechanisms derived ex vivo or in smaller animal models possessing cardiac electrophysiology and anatomy that has significant differences from man is uncertain. More typically, translational models are performed to justify therapies prior to clinical trials evaluating treatments, i.e., device or drug. Large animal models lend themselves to drug development questions, such as delivery, pharmacodynamics and pharmacokinetics studies and assessment of immune response and toxicity. However, even this remains controversial (6). Rarely are they used to guide therapy in man, with notable exceptions (epinephrine for cardiac arrest was tested in dogs and translated directly to man) (7) or in some instances of rare diseases when clinical trials are unrealistic (typically in toxicology) (8). Although no large animal model encompasses all the anatomical and electrophysiological features of man, many similarities exist. However, important differences need to be understood when attempting to translate findings in large animals to humans (9) (see Figure 1).
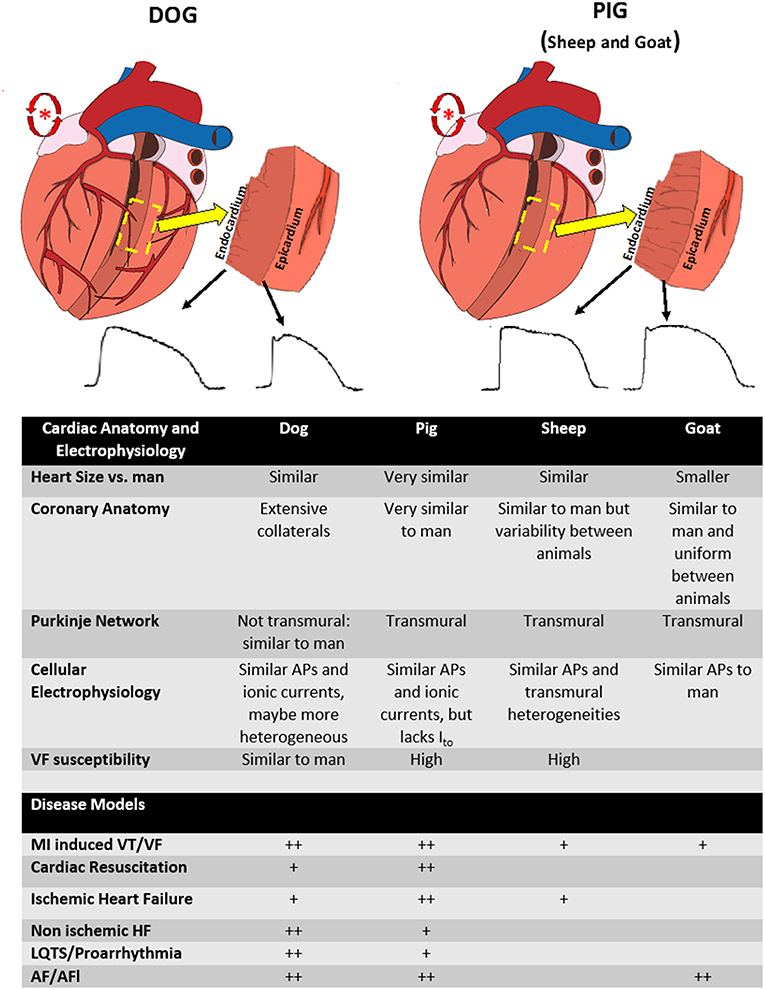
Figure 1. Differences between anatomy and electrophysiology between species. (Top left) Figure of the anatomy of the dog heart. Note the extensive coronary artery collaterals (red vessels), endocardially distributed purkinje network (gray lines on transmural section), and relative transmural heterogeneity in action potentials. (Top right) Figure of the anatomy of the pig heart. Note the lack of coronary collaterals, transmural purkinje system (gray lines on transmural section), and decreased transmural heterogeneity. Both species can be used to model atrial fibrillation (red atrial trigger/reentry schematic). (Lower Table) Summary of the anatomical and electrophysiological differences between animal species as well as the relative common diseases modeled in each species (+, modeled, ++, preferred model).
Testing Arrhythmia Mechanisms and Developing Interventions Using Interventional Electrophysiology and Imaging Techniques
A specific benefit in using large animal models to study mechanisms of arrhythmia and sudden death is that they are very well-suited for assessments of arrhythmia substrates and mechanisms which can also be done in man. Given the size of the adult animal and heart, dogs, pigs, goats, and sheep all can be instrumented with standard human electrophysiology interventions, as well as testing novel catheter based therapies using standard and investigational interventional electrophysiological techniques (9–11). Many of these methods are used clinically in everyday use, or can be adapted to study mechanism. These include 3-D anatomical mapping, contact and non-contact electroanatomical mapping and ablation techniques (10, 12, 13). Imaging modalities (MRI, CT, Echocardiography, etc.) have all been used to develop methods of high resolution combined anatomic and electrical mapping and produce functional measurements of ventricular remodeling (ex. myocardial strain measurements) to determine mechansims of electrophysiological remodeling in disease (14–17).
Which Diseases Have Been Modeled to Determine Arrhythmia Mechanisms and Susceptibility?
Translational models of arrhythmias have naturally focused on modeling diseases which are highly associated with SCD. Models of acute ischemia/reperfusion and MI (3) have been adapted to more chronic models of ischemic cardiomyopathy, with and without acute ischemia (18, 19). Important arrhythmogenic conditions closely related to MI include models of resuscitation from SCD secondary to ischemia and exercise induced VT/VF (20–22). As many as 50% of patients with HF die of arrhythmogenic SCD, so significant effort has been made to model human HF and determine arrhythmia mechanisms in other structural heart diseases, such as isolated hypertrophy (23). Models used to determine arrhythmia specific arrhythmogenic diseases predisposing to SCD have also been well-established. For example, there are specific large animal models of arrhythmogenic right ventricular cardiomyopathy (ARVC) and Duchenne muscular dystrophy (DMD) (24, 25). Large animal models of channelopathies and the drug-induced long QT syndrome (LQTS) have been developed (23, 26, 27). Finally, although not models of SCD, there are multiple, well-characterized large animal models of atrial fibrillation and flutter (AF/AFl), the most common arrhythmia in man (28, 29). Table 1 reviews some of the important specific model platforms used to derive mechanism and test therapies in selected arrhythmogenic diseases.
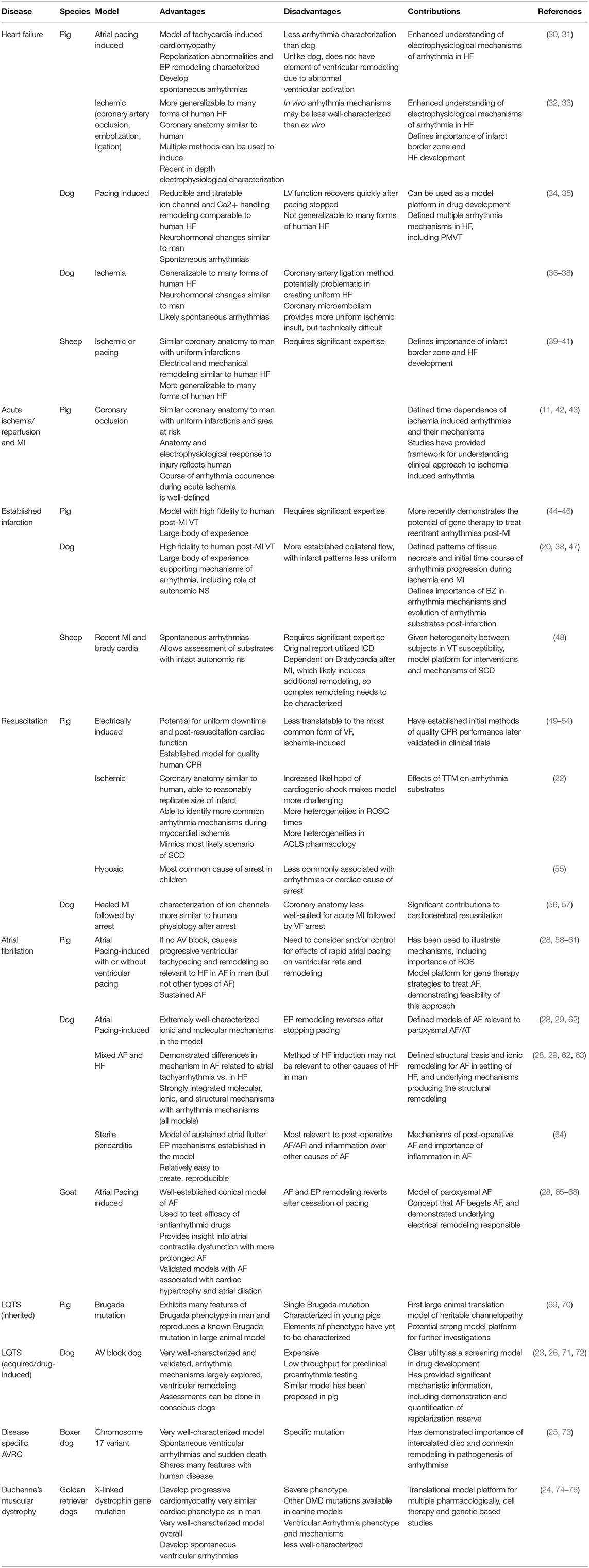
Table 1. Examples of specific models platforms used to derive mechanism and test therapies in selected arrhythmogenic diseases.
Models of Specific Arrhythmogenic Disease
What Are the Pros and Cons of Specific Species in Modeling Human Arrhythmias?
Pig
Given their anatomic and physiological (heart size, coronary anatomy, inflammatory response) and electrophysiological similarities to man, pig models are arguably the preferred species for translational work and have been used to model most arrhythmogenic disease (9). There are some specific electrophysiological differences (most notably a transmural Purkinje system not present in canine or man and diminished transient outward potassium current) which need to be considered, particularly regarding models of acute ischemia (81). In addition, pigs are more susceptible to developing VF than man, which is a consideration when translating outcomes of sudden death to humans (11). Despite this, pig models have been a benchmark for acute MI, acute ischemia and reperfusion and resuscitation studies. This is likely because pigs have more anatomically similar coronary vascular anatomy to man and do not have the extensive collaterals as occurs in canine, making a transmural infarct more similar to man (11, 82, 83). There are established models of ischemic and non-ischemic HF in pigs (Figure 1). A specific consideration in pigs is that although there are established models of atrial tachyarrhythmias, with similarities to the Allessie goat model of atrial fibrillation (65) (discussed below), pigs will develop a tachycardia-induced HF due to the intrinsically fast AV conduction, which needs to be taken into account when designing models of AF (84). Advantages include cost and availability of pigs for research, ability to induce coronary artery disease (85), and that they are amenable to long term outcome studies. Because of their anatomic similarities, well-validated ability to perform cardiopulmonary resuscitation, including CPR, pigs have become the standard translational model for most aspects of human resuscitation, including studies of CPR methodology, arrhythmia mechanisms, and pharmacological treatment (51, 52, 55, 86). Most commonly, VF is induced by electrical current, either on the epicardial surface in an open chest model, or through an intracardiac catheter in a closed chest model. Large animal models also allow for translationally relevant models of myocardial ischemia induced VF and cardiac arrest, due to the ability to perform a transmural infarct (55). As the etiologies of cardiac arrest are also multifaceted, several resuscitation models have been developed, providing important insights (see Table 1).
Canine
Historically, canine models have been used to evaluate cardiac arrhythmia and remain and important model platform. They have similar cellular electrophysiology to man, with similar cellular ionic currents governing depolarization and repolarization and therefore similar action potential profiles. Although controversial, the transmural action potential gradients, producing transmural dispersion of repolarization may be more similar to man (87–89). This makes them perhaps more amenable to studies of acquired or drug-induced long QT syndrome, although this is controversial (23, 27). Similar to man, they do not have a transmural Purkinje system (9, 90). There are other specific advantages to canine models, including better understood autonomic control, which may be more pronounced (slower baseline HR, larger range of HR) (91). They also may be more appropriate for evaluation of autonomic modulation of arrhythmias given their social nature and that they can be trained for conscious experiments which may be particularly important when confounding factor of anesthesia is critical, such as in neural modulation of arrhythmias (20). As in man, dogs can develop valvular disease with aging (92). Much of what we know about mechanisms of arrhythmia in ischemia/reperfusion have also been derived from dog models. There are also a variety of canine HF models (19, 35). A number of AF models in dog have been developed, but AF is not sustained in most dog models (28).
Sheep
Advantages to sheep models include structural heart anatomy that is very similar to man, including similar coronary anatomy and ventricular remodeling after MI (9, 93). However, there has been reported to be significant variability in coronary anatomy between subjects, although uniform infarcts can be achieved (94, 95). Sheep have similar AP profiles to man, including transmural dispersion of repolarization, but a transmural Purkinje system is present, different than man (93, 96–98). There are well-established models of acute ischemia and MI, as well as chronic MI with ventricular remodeling and HF (39–41). Importantly, these models do develop spontaneous VT/VF (48, 99).
Goat
The goat as an AF model is well-established. This model is produced by burst pacing of the atria, and is therefore analogous to the clinical correlate of paroxysmal atrial fibrillation, where the mechanism of AF is related to triggered ectopy originating from the pulmonary veins (29). This model has no underlying structural changes in the atria during short term pacing, but its electrical remodeling is well-defined. However, some structural remodeling (myolysis without fibrosis) occurs with longer term pacing, as does sustained AF (100). It has also been used to evaluate the effectiveness and proarrhythmic effects of antiarrhythmic drugs (101). Given its relatively uniform coronary anatomy between subjects, goat can be used in studies of MI (102).
What Are the Specific Arrhythmogenic Conditions Studied Using Translational Large Animal Models?
Ischemia/Reperfusion and MI
Although canine models were developed first (103), and have provided significant mechanistic information including the paradigm of Phase 1 and 2 arrhythmias (104), ischemic models of cardiac arrest and MI in pig are now the more preferred model platform given anatomic similarities to man regarding coronary anatomy. As cellular studies require simulated ischemia, isolated heart and large animal models have not only historical significance in providing the earliest mechanistic data for studies directly looking at coronary occlusion and MI, but with the exceptions of some advantages of isolated whole heart preparations, are the only models which can faithfully model the complexity of hypoxia, acidosis, and autonomic changes associated with development of ischemia-induced VF (5, 105). Models of established MI without HF and acute ischemia in ischemic cardiomyopathy are created using various methods of coronary artery occlusion in pig, dog, and sheep (Table 1). Coronary occlusion by open ligation, intracoronary microembolism, or reversible balloon occlusion are all options depending on the question being asked.
Cardiopulmonary Resuscitation
Resuscitation from cardiac arrest is complex, with hemodynamic, autonomic, and electrophysiologic factors all playing a role. Furthermore, there are multiple etiologies of cardiac arrest, which can be difficult to initially determine clinically, impeding initiation of specific therapeutic interventions. Even when acute ischemia is the most likely cause, a mix of focal ischemia and global ischemia may promote different and varying arrhythmia substrates not accounted for by advanced cardiac life support (ACLS). The complexity of resuscitation from cardiac arrest in man can best be modeled in large animal models, where realistic resuscitation protocols can be reproduced. For example, the patient undergoing resuscitation from ischemia-induced VF initially undergoes regional ischemia (myocardial infarction), then global ischemia during VF, global reperfusion during resuscitation, and subsequent reperfusion of the culprit lesion. These phases have distinct electrophysiological properties that are not accounted for by current ACLS protocols, and the interplay of arrhythmia substrates and susceptibilities during each phase is unknown. All these phases can be incorporated into a large animal porcine model (22). In vivo porcine models have provided important results on resuscitation approaches and outcomes, although mechanistic information on arrhythmia formation and maintenance is less well-understood (49, 50, 53, 54). We recently reported that in a porcine translational model of ischemia-induced SCA that arrhythmia substrates were in fact dynamic during different phases of resuscitation that they were modified by therapeutic hypothermia. In addition, cardiac alternans was an important mechanism of recurrent VF during resuscitation (22). Regarding species specific models of cardiopulmonary resuscitation, the advantages of transmural and regional ion currents, more human like conduction system and lower susceptibility to VF in dog vs. pig is a consideration when evaluating outcomes or therapies (11, 14). However, given additional factors, particularly similar coronary anatomy, ability to perform chest compressions during cardiopulmonary resuscitation, and cost and housing considerations, and importantly successful translation of interventions to man, the pig has emerged as the primary model (9, 51, 52, 106, 107).
Heart Failure
Multiple HF models in pig, dog, and sheep exist, each with specific advantages and disadvantages. Pacing-induced HF in dog is a well-established model of dilated cardiomyopathy. Its mechanical, ion channel and electrical remodeling has been thoroughly investigated (35). It is titratable and relatively easy to induce reproducible degrees of HF. However, despites its fidelity to many aspects of clinical HF in man, its ready reversibility and mechanism of induction using tachypacing is relevant to a small population with tachycardia induced HF, making it potentially less attractive HF model (35, 108). This tachypacing model has also been used in pig and other large animals (30). Models of ischemic HF after MI from coronary occlusion or microembolism are well-established and validated (19, 32). Pig MI models are more reproducible, given their more uniform coronary anatomy and less prominent coronary collateral circulation vs. dog (11). As ischemic cardiomyopathy is highly clinically prevalent, these models have major translational importance. When microembolism techniques are used to induce ischemia, more uniform and controlled ischemic insults can be produced. This produces more controlled HF and is perhaps the preferred method in dog models of ischemic cardiomyopathy (19, 33).
Channelopathies
Much of our knowledge of regarding animal models of inherited channelopathies, such as the LQTS, comes from murine and small animal models. Ex vivo large animal models, typically recapitulating a channelopathy using pharmacology, have also contributed significantly to understanding mechanism (27). Acquired channelopathies can be related to disease (i.e., HF and hypertrophy), and/or drug induced. An important specific translational model of acquired long QT syndrome is the AV block dog which recapitulates acquired long QT syndrome in dogs which develop hypertrophy. This model is characterized by spontaneous TdP, with significant characterization of its ion channel remodeling, repolarization reserve and abnormal calcium cycling. It has been used as a preclinical test for drug induced TdP and to evaluate TdP mechanisms (23).
Other Arrhythmogenic Diseases
One example of specific model based on a genetic mutation is that of arrhythmogenic right ventricular cardiomyopathy in Boxer dogs. It is associated with a chromosome 17 variant, and the phenotype shares features consistent with human disease, including structural/pathological changes in the ventricle, significant electrophysiological remodeling, spontaneous ventricular arrhythmias, and SCD (25, 73). In addition, this model is characterized by VT of suspected right ventricle origin and structural right ventricular abnormalities. It is extremely well-characterized in terms pathology, structural, and electrophysiological remodeling, as well the arrhythmogenic phenotype.
AF
Importantly, there are multiple AF models, many providing important complimentary mechanistic insight into human AF, which have formed the basis for preclinical development of multiple pharmacologic and other surgical/ablation interventions (28, 29) (see Table 1). Models have been developed in dog, goat, pig and sheep, incorporating pacing-induced remodeling, HF, sterile pericarditis, mitral valve disease, and autonomic modulation, amongst others. Most have been very well-characterized by multiple groups, including underlying structural and electrical remodeling and determination of arrhythmia substrates and triggers. One of the most influential is Allessie's model of AF in the goat, where rapid atrial pacing promoted atrial electrical remodeling and eventually sustained AF (65). There are multiple AF models incorporating different aspects of human disease. These include burst rapid pacing of atria (as in paroxysmal AF) with or without ventricular pacing to produce combined AF with HF and atrial structural remodeling (a common but distinct clinical scenario) and a sterile pericarditis model (which mimics post-operative atrial flutter). This is produced by using talc to irritate the pericardium after mediansternomotomy. All these models have given insight into the multifaceted mechanisms in AF using different, complimentary approaches (28, 29).
In summary, several large animal species have been used to study disease states. Although pigs, dogs, goats, and sheep possess many similarities to cellular and whole heart electrophysiology to man, there are clear differences between species and the right model will always be a compromise (Figure 1). Well-established models of common arrhythmogenic diseases have been developed in these species, with each species having advantages and disadvantages for modeling these diseases (see Table 1 for details). Studies evaluating treatments and interventions for arrhythmogenic disease need to take into account the relative and sometimes complex advantages of each species and disease model.
Lessons Learned: Successes and Pitfalls in Translation
It should be clear when discussing successes vs. failures in electrophysiological research that the majority of translational animal studies do not result in positive clinical trials as 60–80% of the translational studies which are ultimately tested in clinical trials fail (109, 110). It is also important to acknowledge that much large animal translational work suffers from methodological pitfalls that are well-recognized and common to all animal studies and certainly inherent in studies of arrhythmia mechanism. Sample size calculations and power analyses are infrequently reported or adhered to in translational studies, making it difficult to precisely estimate effect of studied interventions (110). Standard approaches used in clinical trials, like blinding and randomization, are inconsistently done, and those studies which do not report blinding or randomization strategies are more likely to be positive (110, 111). Taken together, this would be expected to overestimate positive results provided by subpar methodology, and along with publication bias, more likely to form an inaccurate basis for a clinical trial (110, 112). As young, healthy animals are often used in translational studies, this also makes the extension of results problematic, however, in many of the models discussed above regarding arrhythmia inducibility in disease, this is somewhat mitigated by replicating arrhythmia substrates in chronic animal models.
Challenges in Large Animal Models
By their nature, large animal studies tend to be less mechanistic than those in ex vivo or smaller animal studies (such as transgenic mice). Given the complexity of large animal models, validating specific mechanisms represents a significant challenge and sometimes falls short, or more often is not attempted at all (110). Even when significant efforts are made to create a translational model with high fidelity to human disease and to fully characterize it, challenges remain to directly relate documented cellular mechanisms to whole heart arrhythmia mechanisms given the complexity inherent in any large animal model. In vivo large animal studies are costly, labor-intensive and ethically problematic (6, 113). Anesthesia considerations are particularly important as they can alter sympathetic modulation of arrhythmias, arrhythmia inducibility, ischemic insults, and anesthesia itself can be proarrhythmic (20, 114, 115). Cost is certainly a consideration when performing large animal translational experiments, particularly if purpose bread or genetically modified animals are being studied (69). Societal acceptance for using large animal models can be highly variable, but is generally low, and strict regulatory concerns must be addressed. Unfortunately, cost and ethical considerations can make it difficult to justify and perform large studies in these species, and many large animal studies suffer low power and resulting inaccurate point estimates of the outcome variables (110–112). All these considerations of design and implementation and potential compromises made must be considered when interpreting and applying results of large animal models to clinical trials.
Challenges Specific to Determining Arrhythmia and SCD Mechanism and Testing Therapies in Translational Models
An important feature of all translational model platforms is that they either faithfully reproduce the relevant arrhythmia mechanisms in the disease in question if testing therapy, or are designed to determine new mechanisms in a disease. However, there are important considerations when evaluating the effect of an antiarrhythmic therapy in a large animal model. How well does the model link the antiarrhythmic effect observed to an established arrhythmia mechanism in the disease of interest? How well does the study characterize the arrhythmias and what is their mechanistic basis in the model? Are mechanisms being established or tested in vivo assessments or ex vivo using tissue/cells (32, 116). This can be particularly challenging when mechanisms can by dynamic and time dependent (such as in ischemia/reperfusion or autonomic modulation of arrhythmias), there are multiple mechanisms at play (as in HF, for example), and these mechanisms may be interdependent (117). Modeling both arrhythmia triggers as well as substrates are central to understanding development of reentrant excitation in man, as both are typically required. Models in which dispersion of repolarization can be quantified and directly related to development of conduction block have provided significant information, but mapping these arrhythmias can be challenging (118). A necessary trade off when testing therapies is that determination of specific electrophysiological substrates and the mapping of arrhythmia initiation oftentimes requires extensive invasive monitoring, and although may provide mechanistic information, this can be at the expense of compromising the model. For example, large animal models of cardiac alternans, an important mechanism of reentrant arrhythmia in ischemic heart disease and HF, have been used to evaluate efficacy of antiarrhythmic drugs to suppress alternans and arrhythmias (119, 120). These studies have provided valuable information on potential therapeutics, but not necessarily direct mechanistic insights. Triggered activity is an additional important arrhythmia mechanism which has been reproduced in translational models. However, directly relating triggered activity to its underlying mechanisms (calcium dysregulation in HF or altered repolarization) is more difficult in the whole heart than in simpler model platforms (121), sometimes limiting mechanistic insight when testing potential therapies. Additional conical arrhythmia mechanisms, such as increased automaticity, are also important to consider, but difficult to study in vivo. Finally, neural modulation of arrhythmia substrates, triggers, and automaticity is an important consideration and can be directly examined in experimental models (20, 78).
A particular problem with developing models to determine mechanisms and test therapies is that the occurrence of spontaneous arrhythmia in man is spontaneous. This is difficult to replicate in animal models. Although we know much about mechanisms of arrhythmia triggers and initiation, the “holy grail” is to be determined: why any individual would have a particular event at a particular time? Modeling of spontaneous arrhythmias and their suppression is highly important but rarely achieved as it requires specific monitoring, which is not a trivial task. Moreover, while arrhythmia inducibility using programmed electrical stimulation protocols or drugs is oftentimes used, its translational relevance is questionable. Many models utilized different protocols to induce arrhythmias, but it is critically important to understand the mechanism of the arrhythmia being studied as well as the protocol used to induce the arrhythmia to insure that findings match. Using standard programmed stimulation protocols during bradycardia to induce torsades des pointes (TdP) in models of LQTS (27) provides insight into mechanism, but performing rapid pacing to induce VT in that same model would not be as insightful. Rapid pacing protocols alone, without attempts to determine the etiology of the arrhythmia induced (alternans, triggered activity, for example), are routinely performed but lack mechanistic information.
In summary, large animal models of arrhythmia help us understand the relevance of previously defined in vitro arrhythmia mechanisms within the whole animal. However, performing translational studies in large animal models can be time and resource intensive while becoming less mechanistic in the process. Models of specific arrhythmia disease states have been developed, but reproducing human arrhythmia substrates in these models has been challenging. Spontaneous arrhythmias in these models are not uniform, and protocols to stimulate arrhythmias may be less translational. However, large animals models remain an important translational link between individual arrhythmia mechanisms and arrhythmia formation and management in the whole organism.
Success Stories in Translation
Cardiac Arrest and Resuscitation
As cardiac arrest induces global multi-organ dysfunction, large animal models are particularly well-suited to study the complex interactions between ischemia-reperfusion in multiple organ systems and develop evidence based therapies. The first large animal model of cardiac resuscitation was in 1957, which described closed chest compression (122). The pig has emerged as the best model of resuscitation due to its physiologic parameters being similar to human, the ease of instrumentation, the ability for post-resuscitation neurologic testing, and the ability to perform CPR (51, 52, 55). Although the pig model suffers from all the challenges of large animal models described above, it is a model success due to its ability to provide mechanistic knowledge of resuscitation and its ability to test interventions in a challenging clinical setting. Many lessons learned from animal models have been incorporated into cardiopulmonary resuscitation protocols, tested in humans, and incorporated into guidelines, improving outcomes (123). Optimal delivery of CPR, including chest compression ratios and avoiding pauses in compressions, as well as compression only CPR where initially tested in pig models (51, 52). Years of work in cerebral resuscitation and protection by therapeutic hypothermia was tested in multiple animal models, until eventually being brought to the clinic (124).
Determining Mechanism of Arrhythmias in Ischemia/Reperfusion
Years of work in multiple translational models have firmly established basic mechanisms of arrhythmias in ischemia/reperfusion. Initial development of the paradigm of phases of arrhythmias was first determined in dogs, and this paradigm was reproduced in other models, notably a pig model of ischemia, showing different phases of ischemia and arrhythmia, consistent with human arrhythmia development during ischemia (42, 43). Particular underlying mechanisms of the arrhythmias observed were then further determined using other model platforms. For example, using the established paradigm, the etiology of decreased cell to cell coupling, conduction slowing and reentry arrhythmias demonstrated in type 1b arrhythmias in pig was later demonstrated to be in part attributable to connexin 43 remodeling in rat model of ischemia, with changes in Cx43 localization, expression and phosphorylation (125). This then formed the basis for additional large animal translational studies on gap junction modulation to suppress ventricular arrhythmia (42, 125). This is only one example of defining a mechanisms of ischemia/reperfusion arrhythmias using large animal models, amongst a wealth of others (5, 43).
Determining Mechanisms and Therapies of Arrhythmias in AF
Our basic understanding of mechanisms of AF initiation, maintenance, and variability in substrates and triggers in different diseases has also been firmly established using translational large animal models. Although not uniformly demonstrating spontaneous AF, multiple pacing-induced AF models in dog, goat, pig, initially based on Allessie's model of rapid pacing of atria in the goat, have provided significant insight into mechanism later allowing testing of therapies (65). The rapid atrial pacing model adapted to HF in AF model and a sterile pericarditis model, mimicking post-operative AF, amongst others, all have given insight into the multifaceted mechanisms in AF using different, complimentary models (64). These complimentary models were adapted to investigate different features of human disease, such as lone AF, paroxysmal, or AF in HF with structural remodeling. Differences in these models regarding remodeling of repolarization, heterogeneities of conduction, and structural remodeling, including fibrosis, have demonstrated the importance of different substrates in different clinical subtypes of AF and identified common themes providing insight into mechanisms and therapies for AF in man (28, 65). The careful characterization of arrhythmia mechanism in these models allowed development and preclinical evaluation of therapy based on established mechanisms (29, 126).
Establishing Treatment in HF With Mechanical Dyssynchrony
Translational studies investigating mechanisms underlying the clinical observation of worsening mechanical function in patients undergoing ventricular pacing and left bundle branch block were central to the development of cardiac resynchronization therapy for HF patients (127). Studies in large animal models evaluated both adverse and favorable electrical and mechanical remodeling using pacing strategies in heart failure (77) were subsequently tested in man, and bi-ventricular pacing is now standard therapy for subgroups of HF patients with low ejection fraction and conduction delay (128). A large body of evidence looking at effects of asynchronous ventricular activation on mechanical strain, causing adverse mechanical and electrical remodeling, also suggested potential for proarrhythmia using epicardial pacing (15, 129, 130). However, it is more likely that effects of reverse mechanical and electrical remodeling, improvement of HF and mitigation of ischemia is ultimately antiarrhythmic, and clearly improves HF related morbidity and mortality. Clinical studies show that HF patients treated with CRT-ICDs have less arrhythmia events and ICD shocks (131, 132).
Challenges in Translation
When examining large animal translational studies which ultimately did not lead to clinical therapies or treatment, multiple themes arise. As with current clinical therapies for arrhythmias (ablation, antiarrhythmic drugs), translating interventions developed in large animals to long-term efficacy is highly challenging and must be considered. If an intervention is expected to induce reverse remodeling (as in biventricular pacing in HF), it can be expected to potentially mitigate long term arrhythmia substrates and triggers, but a one-time intervention (as with gene transfer interventions for specific reentrant arrhythmias) may only provide more short term improvements, particularly if underlying arrhythmia substrates continue to progress (133). Specific themes and examples of difficulties with translating findings in large animal models of arrhythmia and SCD are reviewed below:
Heterogeneities of Human Disease Not Adequately Modeled
Heterogeneities in human disease are not typically manifest in any specific animal models, and mechanisms identified or therapies tried can't be extended to all cases. HF is the clearest example where there are multiple different clinical phenotypes (ex. diastolic, systolic, HF with preserved ejection fraction, mixed) and etiologies (ex. ischemic, hypertensive, toxin-mediated, and other dilated cardiomyopathies), and each etiology and subtype is itself extremely complex. Addressing complexity with different, complimentary models, as has been done in AF is a clear example of developing mechanistic understanding by the different models. However, although there are models reflecting the heterogeneities in human disease, it is of course unrealistic to expect a specific therapy can be necessarily used in all patients, and when testing, very difficult to perform a trial with only specific subtypes of a disease are being identified and included, particularly with funding often through industry who may stress more global applications. So although large animal models have clearly informed our mechanistic understanding of the multifaceted mechanisms of arrhythmias in human HF, it remains difficult to extend testing of translation therapies in any one model to a heterogeneous population.
Translating Human Standard of Care to Animal Models
In clinical trials, patient care is optimized. For example, in HF studies patients receive close monitoring and better HF care than they might otherwise, so it is difficult to detect differences in an intervention. Gene therapy for improvement of left ventricular function and arrhythmias using SERCA2A overexpression has been tested in both small animal and larger translational models of HF and ischemia/reperfusion and has robust arrhythmic effects, based on well-defined mechanisms, and importantly did not promote proarrhythmia due to triggered activity (79, 134, 135). However, when in a careful well-executed clinical trial designed to determine effect of SERCA2a overexpression on heart failure, essentially the effect on HF was neutral, while arrhythmia susceptibility was not an outcome measure (136). In this trial, patients were well-matched with multiple underlying etiologies of HF and received state of the art medical care. Although absolutely necessary when designing a clinical trial, demonstrating efficacy in a population which is optimally managed requires a significant treatment effect, also more difficult to determine in a heterogeneous treatment population. Similar difficulties in establishing efficacy were observed in a clinical trial testing a novel peptide based therapy to maintain gap junction coupling to limit ischemia/reperfusion injury in ST elevation MI (80). A strategy of maintaining gap junction coupling during ischemia and reperfusion to suppress arrhythmias was tested in multiple large animal models, where both clear signals of limitation of infarct size and suppression of ventricular arrhythmias was shown (137–139). However, no improvement in myocardial preservation was observed in this carefully performed clinical trial. It was performed in a system where remarkably quick coronary reperfusion was achieved (door to balloon time approximately 30 min and symptom onset to first medical contact less than an hour), limiting ischemia time and exposure to treatment prior to reperfusion (80). With such exemplary clinical care, it would be very difficult to detect differences in myocardial preservation, as the majority of patients would not be expected to have significant long term myocardial damage.
Incompletely Elucidating Mechanisms
It is important to understand the arrhythmia mechanisms being studied by an intervention to insure its relevance when translating to human trials. Much of what we know about mechanisms of antiarrhythmic medications for resuscitation was tested and developed in other settings. There is ample preclinical data on mechanisms of lidocaine and amiodarone, but not during resuscitation. This may be in part why their utility was initially demonstrated and drugs where incorporated into wide clinical use, only later to be called into question, except in particular subgroups (140). A potential criticism of the strategy of pharmacologically maintaining gap junction coupling to prevent arrhythmias in ischemia/reperfusion in man, discussed above, was that despite the strong treatment effect demonstrated in translational animal models, the mechanism of action of the peptide tested remains incompletely understood. This is despite some mechanistic studies and significant biologically plausibility of its antiarrhythmic action and conflicting, but largely positive data regarding effects on myocardial preservation by maintaining gap junction coupling (141). In contrast, although also not completely elucidated, multiple translational studies supporting biventricular pacing were used to derive the mechanisms underlying mechanical and electrical reverse remodeling, supporting its clinical implementation (130, 142).
Translating Outcome Variables From Animal to Human
Oftentimes in performing translational studies, multiple outcome variables are examined to maximize knowledge gained in very time consuming, expensive work. However, when translating to clinical trials, only the most impactful or marketable outcomes are pursued. It is important to align primary outcomes from translational large animal studies to human trials. This is particularly important when outcomes evaluated in the animal trials may have been secondary outcomes, and the preclinical study which may not have been designed to specifically demonstrate differences between a variable of interest that was later was tested in a clinical trial. Sometimes there is a mismatch between the disease or specific target tested in animal models and primary outcome to which it is applied in a clinical trial (wrong target, wrong disease). This is oftentimes due to necessary compromises made in clinical studies, or that a target may not be able to be practically pursued, and if benefit in more than one outcome has been demonstrated, choices need to be made. Spinal cord stimulation has been used for years to treat chronic pain and as a therapy decrease refractory angina in man. A wealth of data demonstrated the efficacy of spinal cord stimulation in translational models to suppresses ischemia induced ventricular arrhythmias where the initial antiarrhythmic signal tested during acute ischemia with and without establishment of HF (18, 143). Improvement in indexes of ventricular remodeling was an outcome in these preclinical studies. These findings were robust and reproduced in multiple studies and models, providing strong evidence of both the antiarrhythmic and reverse ventricular remodeling efficacy of spinal cord stimulation and had significant mechanistic basis. Primary and secondary outcomes of the clinical trial were related to LV function, and although arrhythmias were examined in the majority of the patients and were not different between treated and untreated patients, the design of the study was not necessarily to asses those outcomes. Similarly, in preclinical studies of peptide strategies to maintaining gap junction coupling during ischemia and reperfusion a strong signal for antiarrhythmic effects was demonstrated in multiple large animals, from multiple groups of ischemia reperfusion, which was not the primary outcome when tested in a clinical trial (80).
Translation of the Intervention
Because of practical concerns of cost, judicious use of animal resources, and time constraints, large animal models testing therapies are optimized to demonstrate effectiveness. However, this occasionally results in interventions that can't be delivered as well-clinically and additionally, compliance of patients with tested therapies is a challenge. An example is use of spinal cord stimulation in man, which has significant translational promise as an antiarrhythmic and HF therapy, but its clinical implementation is not trivial. This is despite extensive preclinical dosing development in translational experiments (144). Investigators could not deliver the same duration or type of stimulation as was done in animals, and subjects couldn't be adequately blinded as patients could often tell and were bothered by paresthesias when the stimulator was on. Enrollment was stopped due to futility (145). Of note, a non-controlled study using a different stimulation protocol did show a signal of benefit, suggesting the negative results may have in part been explained by the stimulation protocol used (146). Another example of difficulty in translating therapy in man is gene delivery, where there has been exciting data in translational models demonstrating great therapeutic potential, but has proven difficult to implement successful in clinical trials. In man, gene delivery is challenging and expensive, particularly if not focused to a specific anatomical region. The large doses needed is a limitation. More targeted delivery to a scar and MI border zone or atrial epicardium may be more practical and likely to succeed in the near future (147). Resuscitation studies have also suffered from difficulty in delivery of interventions. Drug delivery occurs quicker in animal models of cardiac arrest, which likely improve outcomes compared to clinical studies (148). Furthermore, animal models were used in device testing for mechanical CPR, leading to a large clinical trial of prehospital mechanical CPR which did not show an improvement of survival (149, 150).
In summary, large animal models of arrhythmia have been both successful and challenging to translate into medical practice. Large animal studies often suffer from decreased scientific rigor of their human counterparts and focus on outcomes that might not be as pertinent in human studies. However, large animal models have been highly influential, with characterization of arrhythmia mechanisms, establishing novel therapies, and translation of diseases with low survival being the most successful. Common challenges of large animal studies tend to revolve around common themes: outcome variables not directly translatable to humans, incompletely elucidated mechanisms, and clinical standard of care. Some of these pitfalls may be avoided with careful study design, and understanding of outcomes that would likely be tested in human studies. A summary of Translational successes and pitfalls are reviewed in Table 2.
Recent Advancements
Although large animal models are oftentimes limited mechanistically due to inherent limitations in invasive arrhythmia mapping and establishing direct mechanistic links in a highly complex system, there have been significant strides made recently to improve methodology and provide more mechanistic insight.
Genetic Modification in Large Animal Models of Arrhythmia
A porcine model of Brugada syndrome was produced by Park et al. in which they engineered an early truncation mutation in SCN5a (E555X) to produce SCN5a haploinsufficiency (70). Although this model did not have in vivo arrhythmias, in Langendorf experiments, temperature-dependent changes in conduction and arrhythmias induced by rapid pacing were demonstrated and consistent with Brugada syndrome. A criticism of the model was that the pigs did not exhibit classic changes observed in the Brugada ECG. Due to cost and housing limitations, investigators were unable to allow the pigs to mature into adulthood so perhaps the phenotype was not fully realized, and pigs minimally express the transient outward potassium current, which has been demonstrated to be mechanistically related to arrhythmia susceptibility in Brugada patients (69). There are also multiple mutations which create a Brugada phenotype. However, this model represents a major advance in studying Brugada syndrome. Given that multiple transgenic pig models have been produced, it is likely it will be used more as a model platform for additional arrhythmogenic disease in the future (9, 151). Transgenic goat model of cardiac- specific overexpression of TGFB1 with atrial fibrosis and increased susceptibility to atrial fibrillation has also been developed (152).
Genetically modified large animals serving as model platforms to understand mechanism or test therapies have clear advantages over rodent models, where although signaling pathways and underlying mechanisms can be better delineated, its relevance to the human electrophysiological phenotype is oftentimes questionable. However, establishing large animal models are expensive and difficult to perform. By their nature, they are targeting a specific disease or mutation, so not necessarily relevant to the more common complex arrhythmogenic diseases, such as HF (151). Importantly, the phenotypes produced do not tend to fully represent the human disease, which is not necessarily surprising given that even in arrhythmogenic disease thought to be monogenetic, and most potentially amenable to be reproduced in large animal models, multiple modifying factor (genetic as well as environmental) are at play, complicating phenotypical penetrance in man. This would presumably be only more problematic in large animal models (153).
Targeted Gene Delivery
Gene transfer technologies have been applied to translational large animal models for some time and continue to provide significant mechanistic insight into arrhythmias and have potential as therapies. Multiple targets have been tested in large animal models, primarily targeting reentrant mechanism by disrupting reentrant circuits locally by prolonging repolarization (154) or enhancing conduction in both ventricle and atria (44, 58) and have generally found to be antiarrhythmic. An additional strategy is normalizing calcium cycling using gene overexpression of SERCA2a during ischemia/reperfusion (135) or HF, in which the antiarrhythmic mechanisms of normalizing calcium cycling have been demonstrated in smaller animals (134), but yet to be fully elucidated large animal translational models. In a pig model of complete heart block, a “biological pacemaker” was created using an adenoviral vector delivery of the of transcription factor T-box 18 to convert ventricular myocytes into pacemaker cells. The investigators demonstrated improved heart rate response to exercise, and although the duration of effect was only on the order of 2 weeks, they proposed this could represent a temporizing therapy for patient with lead infections requiring explanation, antibiotics and time prior to reimplantation (155). Although targeted gene delivery has potentially significant therapeutic potential, this has yet to be realized, and has significant limitations which need to be overcome (133).
In vivo Optical Mapping
A particularly exciting recent advancement is the potential use of optical mapping in vivo in large animal models. Optical mapping utilizes voltage sensitive fluorescent dyes to measure cardiac action potentials with high spatial and temporal resolution (156). It has provided significant insight into mechanisms of arrhythmias in both normal and disease models. It has traditionally required ex vivo preparations due to limitations of using voltage sensitive dyes in blood perfused preparations and requirements for mechanical or pharmacological motion control of the preparations; however, in vivo optical mapping in small animals, also incorporating calcium indicators has been done (157) and was initially reported in canine using a fiber optic probe and intracoronary injection of dye. This allowed action potentials to be recorded from a 4.5 vs. 4.5 cm area of the LV epicardial surface and study in situ mechanisms of VF and response to defibrillation (158). Recently, a new method for in vivo optical mapping of pigs has been reported (159). Using two near-infrared voltage sensitive dyes, the authors were able to demonstrate that, in vivo, ratiometric signals could reduce motion artifacts, allowing for mapping of activation (at faster CLs) and VF dynamics. The addition of a fiberoptic system allowed measurements of repolarization and action potential maps with high fidelity from a more limited area of the heart. Clearly, this can become a very valuable tool for studying arrhythmia mechanisms in large translational models, and it is highly likely that further refinement of this system, such as incorporating multidetector panoramic or intrachamber imaging could allow for even greater mechanistic evaluations (160).
Conclusions
Large animal models of arrhythmia have been instrumental to the translation of specific ex vivo arrhythmia mechanisms into whole organism systems to both determine relevance of indentified arrhythmia mechanisms and approaches to their treatment in humans. Large animal models have led to changes in established therapy, novel approaches to treatment, and continue to be a necessary vessel for introduction and testing of novel techniques and therapies. Several large animal species are available with multiple specific disease models. Understanding the pros and cons of these different species and disease models is paramount when interpreting data derived from translational models and before testing novel therapies in man. By understanding past successes and challenges, large animal models of arrhythmias can be further developed and refined to better translate novel ideas into therapy. Studies in large animal models of arrhythmia will continue to be an essential tool to further our understanding of arrhythmias and design therapies to improve outcomes in patients suffering from cardiac arrhythmias.
Author Contributions
Both authors contributed equally to the conception, design, and writing of the review.
Funding
This work was funded by Departmental MetroHealth Foundation Grant (JP and LW) and National Institute of Health Grant 1R56HL142754 (LW and JP).
Conflict of Interest Statement
JP received grant from ZOLL Foundation. JP and LW received a gift of investigational compounds from Zealand Pharma.
References
1. Zipes DP, Wellens HJ. Sudden cardiac death. Circulation. (1998) 98:2334–51. doi: 10.1161/01.CIR.98.21.2334
2. Solomon SD, Zelenkofske S, McMurray JJ, Finn PV, Velazquez E, Ertl G, et al. Sudden death in patients with myocardial infarction and left ventricular dysfunction, heart failure, or both. N Engl J Med. (2005) 352:2581–8. doi: 10.1056/NEJMoa043938
3. Benjamin EJ, Blaha MJ, Chiuve SE, Cushman M, Das SR, Deo R, et al. Heart disease and stroke statistics-2017 update: a report from the American Heart Association. Circulation. (2017) 135:e146–603. doi: 10.1161/CIR.0000000000000485
4. Pogwizd SM, Schlotthauer K, Li L, Yuan W, Bers DM. Arrhythmogenesis and contractile dysfunction in heart failure: Roles of sodium-calcium exchange, inward rectifier potassium current, and residual beta-adrenergic responsiveness. Circ Res. (2001) 88:1159–67. doi: 10.1161/hh1101.091193
5. Di Diego JM, Antzelevitch C. Ischemic ventricular arrhythmias: experimental models and their clinical relevance. Heart Rhythm. (2011) 8:1963–8. doi: 10.1016/j.hrthm.2011.06.036
6. Shanks N, Greek R, Greek J. Are animal models predictive for humans? Philos Ethics Hum Med. (2009) 4:2. doi: 10.1186/1747-5341-4-2
7. Callaway CW. Epinephrine for cardiac arrest. Curr Opin Cardiol. (2013) 28:36–42. doi: 10.1097/HCO.0b013e32835b0979
8. Shepherd G, Klein-Schwartz W. High-dose insulin therapy for calcium-channel blocker overdose. Ann Pharmacother. (2005) 39:923–30. doi: 10.1345/aph.1E436
9. Clauss S, Bleyer C, Schuttler D, Tomsits P, Renner S, Klymiuk N, et al. Animal models of arrhythmia: classic electrophysiology to genetically modified large animals. Nat Rev Cardiol. (2019) 16:457–75. doi: 10.1038/s41569-019-0179-0
10. Suzuki Y, Yeung AC, Ikeno F. The pre-clinical animal model in the translational research of interventional cardiology. JACC Cardiovasc Intervent. (2009) 2:373–83. doi: 10.1016/j.jcin.2009.03.004
11. Lukacs E, Magyari B, Toth L, Petrasi Z, Repa I, Koller A, et al. Overview of large animal myocardial infarction models (review). Acta Physiol Hung. (2012) 99:365–81. doi: 10.1556/APhysiol.99.2012.4.1
12. Lehmann HI, Graeff C, Simoniello P, Constantinescu A, Takami M, Lugenbiel P, et al. Feasibility study on cardiac arrhythmia ablation using high-energy heavy ion beams. Sci Rep. (2016) 6:38895. doi: 10.1038/srep38895
13. Nordbeck P, Hiller KH, Fidler F, Warmuth M, Burkard N, Nahrendorf M, et al. Feasibility of contrast-enhanced and nonenhanced MRI for intraprocedural and postprocedural lesion visualization in interventional electrophysiology: animal studies and early delineation of isthmus ablation lesions in patients with typical atrial flutter. Circ Cardiovasc Imaging. (2011) 4:282–94. doi: 10.1161/CIRCIMAGING.110.957670
14. Tschabrunn CM, Roujol S, Nezafat R, Faulkner-Jones B, Buxton AE, Josephson ME, et al. A swine model of infarct-related reentrant ventricular tachycardia: electroanatomic, magnetic resonance, and histopathological characterization. Heart Rhythm. (2016) 13:262–73. doi: 10.1016/j.hrthm.2015.07.030
15. Jeyaraj D, Wilson LD, Zhong J, Flask C, Saffitz JE, Deschenes I, et al. Mechanoelectrical feedback as novel mechanism of cardiac electrical remodeling. Circulation. (2007) 115:3145–55. doi: 10.1161/CIRCULATIONAHA.107.688317
16. Prinzen FW, Hunter WC, Wyman BT, McVeigh ER. Mapping of regional myocardial strain and work during ventricular pacing: experimental study using magnetic resonance imaging tagging. J Am Coll Cardiol. (1999) 33:1735–42. doi: 10.1016/S0735-1097(99)00068-6
17. Spragg DD, Akar FG, Helm RH, Tunin RS, Tomaselli GF, Kass DA. Abnormal conduction and repolarization in late-activated myocardium of dyssynchronously contracting hearts. Cardiovasc Res. (2005) 67:77–86. doi: 10.1016/j.cardiores.2005.03.008
18. Issa ZF, Zhou X, Ujhelyi MR, Rosenberger J, Bhakta D, Groh WJ, et al. Thoracic spinal cord stimulation reduces the risk of ischemic ventricular arrhythmias in a postinfarction heart failure canine model. Circulation. (2005) 111:3217–20. doi: 10.1161/CIRCULATIONAHA.104.507897
19. Adamson PB, Vanoli E. Early autonomic and repolarization abnormalities contribute to lethal arrhythmias in chronic ischemic heart failure: characteristics of a novel heart failure model in dogs with postmyocardial infarction left ventricular dysfunction. J Am Coll Cardiol. (2001) 37:1741–8. doi: 10.1016/S0735-1097(01)01185-8
20. Billman GE. A comprehensive review and analysis of 25 years of data from an in vivo canine model of sudden cardiac death: implications for future anti-arrhythmic drug development. Pharmacol Ther. (2006) 111:808–35. doi: 10.1016/j.pharmthera.2006.01.002
21. Niemann JT, Rosborough JP, Youngquist S, Thomas J, Lewis RJ. Is all ventricular fibrillation the same? A comparison of ischemically induced with electrically induced ventricular fibrillation in a porcine cardiac arrest and resuscitation model. Critic Care Med. (2007) 35:1356–61. doi: 10.1097/01.CCM.0000261882.47616.7D
22. Piktel JS, Cheng A, McCauley M, Dale Z, Nassal M, Maleski D, et al. Hypothermia modulates arrhythmia substrates during different phases of resuscitation from ischemic cardiac arrest. J Am Heart Assoc. (2017) 6:e006472. doi: 10.1161/JAHA.117.006472
23. Vos MA, de Groot SH, Verduyn SC, van der Zande J, Leunissen HD, Cleutjens JP, et al. Enhanced susceptibility for acquired torsade de pointes arrhythmias in the dog with chronic, complete AV block is related to cardiac hypertrophy and electrical remodeling. Circulation. (1998) 98:1125–35. doi: 10.1161/01.CIR.98.11.1125
24. Kornegay JN, Bogan JR, Bogan DJ, Childers MK, Li J, Nghiem P, et al. Canine models of Duchenne muscular dystrophy and their use in therapeutic strategies. Mammal Genome. (2012) 23(1–2):85–108. doi: 10.1007/s00335-011-9382-y
25. Basso C, Fox PR, Meurs KM, Towbin JA, Spier AW, Calabrese F, et al. Arrhythmogenic right ventricular cardiomyopathy causing sudden cardiac death in boxer dogs: a new animal model of human disease. Circulation. (2004) 109:1180–5. doi: 10.1161/01.CIR.0000118494.07530.65
26. Oros A, Beekman JD, Vos MA. The canine model with chronic, complete atrio-ventricular block. Pharmacol Ther. (2008) 119:168–78. doi: 10.1016/j.pharmthera.2008.03.006
27. Akar FG, Yan GX, Antzelevitch C, Rosenbaum DS. Unique topographical distribution of M cells underlies reentrant mechanism of torsade de pointes in the long-QT syndrome. Circulation. (2002) 105:1247–53. doi: 10.1161/hc1002.105231
28. Finet JE, Rosenbaum DS, Donahue JK. Information learned from animal models of atrial fibrillation. Cardiol Clin. (2009) 27:45–54, viii. doi: 10.1016/j.ccl.2008.09.005
29. Nishida K, Michael G, Dobrev D, Nattel S. Animal models for atrial fibrillation: clinical insights and scientific opportunities. Europace. (2010) 12:160–72. doi: 10.1093/europace/eup328
30. Spinale FG, Hendrick DA, Crawford FA, Smith AC, Hamada Y, Carabello BA. Chronic supraventricular tachycardia causes ventricular dysfunction and subendocardial injury in swine. Am J Physiol. (1990) 259(1 Pt 2):H218–29. doi: 10.1152/ajpheart.1990.259.1.H218
31. Lacroix D, Gluais P, Marquie C, D'Hoinne C, Adamantidis M, Bastide M. Repolarization abnormalities and their arrhythmogenic consequences in porcine tachycardia-induced cardiomyopathy. Cardiovasc Res. (2002) 54:42–50. doi: 10.1016/S0008-6363(02)00236-5
32. Hegyi B, Bossuyt J, Griffiths LG, Shimkunas R, Coulibaly Z, Jian Z, et al. Complex electrophysiological remodeling in postinfarction ischemic heart failure. Proc Natl Acad Sci USA. (2018) 115:E3036–44. doi: 10.1073/pnas.1718211115
33. Dixon JA, Spinale FG. Large animal models of heart failure: a critical link in the translation of basic science to clinical practice. Circ Heart Fail. (2009) 2:262–71. doi: 10.1161/CIRCHEARTFAILURE.108.814459
34. Akar FG, Rosenbaum DS. Transmural electrophysiological heterogeneities underlying arrhythmogenesis in heart failure. Circ Res. (2003) 93:638–45. doi: 10.1161/01.RES.0000092248.59479.AE
35. Pak PH, Nuss HB, Tunin RS, Kaab S, Tomaselli GF, Marban E, et al. Repolarization abnormalities, arrhythmia and sudden death in canine tachycardia-induced cardiomyopathy. J Am Coll Cardiol. (1997) 30:576–84. doi: 10.1016/S0735-1097(97)00193-9
36. Sabbah HN, Stein PD, Kono T, Gheorghiade M, Levine TB, Jafri S, et al. A canine model of chronic heart failure produced by multiple sequential coronary microembolizations. Am J Physiol. (1991) 260(4 Pt 2):H1379–84. doi: 10.1152/ajpheart.1991.260.4.H1379
37. Sabbah HN, Shimoyama H, Kono T, Gupta RC, Sharov VG, Scicli G, et al. Effects of long-term monotherapy with enalapril, metoprolol, and digoxin on the progression of left ventricular dysfunction and dilation in dogs with reduced ejection fraction. Circulation. (1994) 89:2852–9. doi: 10.1161/01.CIR.89.6.2852
38. Ursell PC, Gardner PI, Albala A, Fenoglio JJ Jr, Wit AL. Structural and electrophysiological changes in the epicardial border zone of canine myocardial infarcts during infarct healing. Circ Res. (1985) 56:436–51. doi: 10.1161/01.RES.56.3.436
39. Jackson BM, Gorman JH, Moainie SL, Guy TS, Narula N, Narula J, et al. Extension of borderzone myocardium in postinfarction dilated cardiomyopathy. J Am Coll Cardiol. (2002) 40:1160–7; discussion 8–71. doi: 10.1016/S0735-1097(02)02121-6
40. Zaitsev AV, Berenfeld O, Mironov SF, Jalife J, Pertsov AM. Distribution of excitation frequencies on the epicardial and endocardial surfaces of fibrillating ventricular wall of the sheep heart. Circ Res. (2000) 86:408–17. doi: 10.1161/01.RES.86.4.408
41. Kelley ST, Malekan R, Gorman JH III, Jackson BM, Gorman RC, Suzuki Y, et al. Restraining infarct expansion preserves left ventricular geometry and function after acute anteroapical infarction. Circulation. (1999) 99:135–42. doi: 10.1161/01.CIR.99.1.135
42. Smith WTt, Fleet WF, Johnson TA, Engle CL, Cascio WE. The Ib phase of ventricular arrhythmias in ischemic in situ porcine heart is related to changes in cell-to-cell electrical coupling. Experimental Cardiology Group, University of North Carolina. Circulation. (1995) 92:3051–60. doi: 10.1161/01.CIR.92.10.3051
43. Carmeliet E. Cardiac ionic currents and acute ischemia: from channels to arrhythmias. Physiol Rev. (1999) 79:917–1017. doi: 10.1152/physrev.1999.79.3.917
44. Greener ID, Sasano T, Wan X, Igarashi T, Strom M, Rosenbaum DS, et al. Connexin43 gene transfer reduces ventricular tachycardia susceptibility after myocardial infarction. J Am Coll Cardiol. (2012) 60:1103–10. doi: 10.1016/j.jacc.2012.04.042
45. Eldar M, Fitzpatrick AP, Ohad D, Smith MF, Hsu S, Whayne JG, et al. Percutaneous multielectrode endocardial mapping during ventricular tachycardia in the swine model. Circulation. (1996) 94:1125–30. doi: 10.1161/01.CIR.94.5.1125
46. Sasano T, Kelemen K, Greener ID, Donahue JK. Ventricular tachycardia from the healed myocardial infarction scar: validation of an animal model and utility of gene therapy. Heart Rhythm. (2009) 6(Suppl. 8):S91–7. doi: 10.1016/j.hrthm.2009.03.048
47. Reimer KA, Lowe JE, Rasmussen MM, Jennings RB. The wavefront phenomenon of ischemic cell death. 1. Myocardial infarct size vs duration of coronary occlusion in dogs. Circulation. (1977) 56:786–94. doi: 10.1161/01.CIR.56.5.786
48. Killingsworth CR, Walcott GP, Gamblin TL, Girouard SD, Smith WM, Ideker RE. Chronic myocardial infarction is a substrate for bradycardia-induced spontaneous tachyarrhythmias and sudden death in conscious animals. J Cardiovasc Electrophysiol. (2006) 17:189–97. doi: 10.1111/j.1540-8167.2005.00336.x
49. Boddicker KA, Zhang Y, Zimmerman MB, Davies LR, Kerber RE. Hypothermia improves defibrillation success and resuscitation outcomes from ventricular fibrillation. Circulation. (2005) 111:3195–201. doi: 10.1161/CIRCULATIONAHA.104.492108
50. Gotberg M, van der Pals J, Olivecrona GK, Gotberg M, Koul S, Erlinge D. Mild hypothermia reduces acute mortality and improves hemodynamic outcome in a cardiogenic shock pig model. Resuscitation. (2010) 81:1190–6. doi: 10.1016/j.resuscitation.2010.04.033
51. Kern KB, Hilwig RW, Berg RA, Sanders AB, Ewy GA. Importance of continuous chest compressions during cardiopulmonary resuscitation: improved outcome during a simulated single lay-rescuer scenario. Circulation. (2002) 105:645–9. doi: 10.1161/hc0502.102963
52. Berg RA, Kern KB, Hilwig RW, Berg MD, Sanders AB, Otto CW, et al. Assisted ventilation does not improve outcome in a porcine model of single-rescuer bystander cardiopulmonary resuscitation. Circulation. (1997) 95:1635–41. doi: 10.1161/01.CIR.95.6.1635
53. Grueskin J, Tanen DA, Harvey P, Dos Santos F, Richardson WH III, Riffenburgh RH. A pilot study of mechanical stimulation and cardiac dysrhythmias in a porcine model of induced hypothermia. Wilderness Environ Med. (2007) 18:133–7. doi: 10.1580/06-WEME-BR-034R1.1
54. Menegazzi JJ, Rittenberger JC, Suffoletto BP, Logue ES, Salcido DD, Reynolds JC, et al. Effects of pre-arrest and intra-arrest hypothermia on ventricular fibrillation and resuscitation. Resuscitation. (2009) 80:126–32. doi: 10.1016/j.resuscitation.2008.09.002
55. Cherry BH, Nguyen AQ, Hollrah RA, Olivencia-Yurvati AH, Mallet RT. Modeling cardiac arrest and resuscitation in the domestic pig. World J Critic Care Med. (2015) 4:1–12. doi: 10.5492/wjccm.v4.i1.1
56. Sridhar A, Nishijima Y, Terentyev D, Terentyeva R, Uelmen R, Kukielka M, et al. Repolarization abnormalities and afterdepolarizations in a canine model of sudden cardiac death. Am J Physiol Regulat Integr Comp Physiol. (2008) 295:R1463–72. doi: 10.1152/ajpregu.90583.2008
57. Nozari A, Safar P, Stezoski SW, Wu X, Henchir J, Radovsky A, et al. Mild hypothermia during prolonged cardiopulmonary cerebral resuscitation increases conscious survival in dogs. Critic Care Med. (2004) 32:2110–6. doi: 10.1097/01.CCM.0000142700.19377.AE
58. Igarashi T, Finet JE, Takeuchi A, Fujino Y, Strom M, Greener ID, et al. Connexin gene transfer preserves conduction velocity and prevents atrial fibrillation. Circulation. (2012) 125:216–25. doi: 10.1161/CIRCULATIONAHA.111.053272
59. Liu Z, Donahue JK. The Use of gene therapy for ablation of atrial fibrillation. Arrhythm Electrophysiol Rev. (2014) 3:139–44. doi: 10.15420/aer.2014.3.3.139
60. Bikou O, Thomas D, Trappe K, Lugenbiel P, Kelemen K, Koch M, et al. Connexin 43 gene therapy prevents persistent atrial fibrillation in a porcine model. Cardiovasc Res. (2011) 92:218–25. doi: 10.1093/cvr/cvr209
61. Dudley SC Jr, Hoch NE, McCann LA, Honeycutt C, Diamandopoulos L, Fukai T, et al. Atrial fibrillation increases production of superoxide by the left atrium and left atrial appendage: role of the NADPH and xanthine oxidases. Circulation. (2005) 112:1266–73. doi: 10.1161/CIRCULATIONAHA.105.538108
62. Yue L, Melnyk P, Gaspo R, Wang Z, Nattel S. Molecular mechanisms underlying ionic remodeling in a dog model of atrial fibrillation. Circ Res. (1999) 84:776–84. doi: 10.1161/01.RES.84.7.776
63. Li D, Fareh S, Leung TK, Nattel S. Promotion of atrial fibrillation by heart failure in dogs: atrial remodeling of a different sort. Circulation. (1999) 100:87–95. doi: 10.1161/01.CIR.100.1.87
64. Page PL, Plumb VJ, Okumura K, Waldo AL. A new animal model of atrial flutter. J Am Coll Cardiol. (1986) 8:872–9. doi: 10.1016/S0735-1097(86)80429-6
65. Wijffels MC, Kirchhof CJ, Dorland R, Allessie MA. Atrial fibrillation begets atrial fibrillation. A study in awake chronically instrumented goats. Circulation. (1995) 92:1954–68. doi: 10.1161/01.CIR.92.7.1954
66. Greiser M, Neuberger HR, Harks E, El-Armouche A, Boknik P, de Haan S, et al. Distinct contractile and molecular differences between two goat models of atrial dysfunction: AV block-induced atrial dilatation and atrial fibrillation. J Mol Cell Cardiol. (2009) 46:385–94. doi: 10.1016/j.yjmcc.2008.11.012
67. Neuberger HR, Schotten U, Verheule S, Eijsbouts S, Blaauw Y, van Hunnik A, et al. Development of a substrate of atrial fibrillation during chronic atrioventricular block in the goat. Circulation. (2005) 111:30–7. doi: 10.1161/01.CIR.0000151517.43137.97
68. Remes J, van Brakel TJ, Bolotin G, Garber C, de Jong MM, van der Veen FH, et al. Persistent atrial fibrillation in a goat model of chronic left atrial overload. J Thor Cardiovasc Surg. (2008) 136:1005–11. doi: 10.1016/j.jtcvs.2008.05.015
69. Wilde AA, Postema PG. Bringing home the bacon? The next step in cardiac sodium channelopathies. J Clin Invest. (2015) 125:99–101. doi: 10.1172/JCI80014
70. Park DS, Cerrone M, Morley G, Vasquez C, Fowler S, Liu N, et al. Genetically engineered SCN5A mutant pig hearts exhibit conduction defects and arrhythmias. J Clin Invest. (2015) 125:403–12. doi: 10.1172/JCI76919
71. Sprenkeler DJ, Bossu A, Beekman JDM, Schoenmakers M, Vos MA. An augmented negative force-frequency relationship and slowed mechanical restitution are associated with increased susceptibility to drug-induced torsade de pointes arrhythmias in the chronic atrioventricular block dog. Front Physiol. (2018) 9:1086. doi: 10.3389/fphys.2018.01086
72. Sugiyama A, Nakamura Y, Akie Y, Saito H, Izumi Y, Yamazaki H, et al. Microminipig, a non-rodent experimental animal optimized for life science research: in vivo proarrhythmia models of drug-induced long QT syndrome: development of chronic atrioventricular block model of microminipig. J Pharmacol Sci. (2011) 115:122–6. doi: 10.1254/jphs.10R21FM
73. Oxford EM, Everitt M, Coombs W, Fox PR, Kraus M, Gelzer AR, et al. Molecular composition of the intercalated disc in a spontaneous canine animal model of arrhythmogenic right ventricular dysplasia/cardiomyopathy. Heart Rhythm. (2007) 4:1196–205. doi: 10.1016/j.hrthm.2007.05.025
74. Valentine BA, Winand NJ, Pradhan D, Moise NS, de Lahunta A, Kornegay JN, et al. Canine X-linked muscular dystrophy as an animal model of Duchenne muscular dystrophy: a review. Am J Med Genet. (1992) 42:352–6. doi: 10.1002/ajmg.1320420320
75. Valentine BA, Cummings JF, Cooper BJ. Development of Duchenne-type cardiomyopathy. Morphologic studies in a canine model. Am J Pathol. (1989) 135:671–8.
76. Chetboul V, Escriou C, Tessier D, Richard V, Pouchelon JL, Thibault H, et al. Tissue Doppler imaging detects early asymptomatic myocardial abnormalities in a dog model of Duchenne's cardiomyopathy. Eur Heart J. (2004) 25:1934–9. doi: 10.1016/j.ehj.2004.09.007
77. Leclercq C, Faris O, Tunin R, Johnson J, Kato R, Evans F, et al. Systolic improvement and mechanical resynchronization does not require electrical synchrony in the dilated failing heart with left bundle-branch block. Circulation. (2002) 106:1760–3. doi: 10.1161/01.CIR.0000035037.11968.5C
78. Lopshire JC, Zhou X, Dusa C, Ueyama T, Rosenberger J, Courtney N, et al. Spinal cord stimulation improves ventricular function and reduces ventricular arrhythmias in a canine postinfarction heart failure model. Circulation. (2009) 120:286–94. doi: 10.1161/CIRCULATIONAHA.108.812412
79. Kawase Y, Ly HQ, Prunier F, Lebeche D, Shi Y, Jin H, et al. Reversal of cardiac dysfunction after long-term expression of SERCA2a by gene transfer in a pre-clinical model of heart failure. J Am Coll Cardiol. (2008) 51:1112–9. doi: 10.1016/j.jacc.2007.12.014
80. Engstrom T, Nepper-Christensen L, Helqvist S, Klovgaard L, Holmvang L, Jorgensen E, et al. Danegaptide for primary percutaneous coronary intervention in acute myocardial infarction patients: a phase 2 randomised clinical trial. Heart. (2018) 104:1593–9. doi: 10.1136/heartjnl-2017-312774
81. Li GR, Du XL, Siow YL, O K, Tse HF, Lau CP. Calcium-activated transient outward chloride current and phase 1 repolarization of swine ventricular action potential. Cardiovasc Res. (2003) 58:89–98. doi: 10.1016/S0008-6363(02)00859-3
82. Weaver ME, Pantely GA, Bristow JD, Ladley HD. A quantitative study of the anatomy and distribution of coronary arteries in swine in comparison with other animals and man. Cardiovasc Res. (1986) 20:907–17. doi: 10.1093/cvr/20.12.907
83. Hughes GC, Post MJ, Simons M, Annex BH. Translational physiology: porcine models of human coronary artery disease: implications for preclinical trials of therapeutic angiogenesis. J Appl Physiol. (2003) 94:1689–701. doi: 10.1152/japplphysiol.00465.2002
84. Bauer A, McDonald AD, Donahue JK. Pathophysiological findings in a model of persistent atrial fibrillation and severe congestive heart failure. Cardiovasc Res. (2004) 61:764–70. doi: 10.1016/j.cardiores.2003.12.013
85. Vilahur G, Padro T, Badimon L. Atherosclerosis and thrombosis: insights from large animal models. J Biomed Biotechnol. (2011) 2011:907575. doi: 10.1155/2011/907575
86. Wang J, Weil MH, Tang W, Chang YT, Huang L. A comparison of electrically induced cardiac arrest with cardiac arrest produced by coronary occlusion. Resuscitation. (2007) 72:477–83. doi: 10.1016/j.resuscitation.2006.06.041
87. Glukhov AV, Fedorov VV, Lou Q, Ravikumar VK, Kalish PW, Schuessler RB, et al. Transmural dispersion of repolarization in failing and nonfailing human ventricle. Circ Res. (2010) 106:981–91. doi: 10.1161/CIRCRESAHA.109.204891
88. Wilson LD, Jennings MM, Rosenbaum DS. Point: M cells are present in the ventricular myocardium. Heart Rhythm. (2011) 8:930–3. doi: 10.1016/j.hrthm.2011.01.026
89. Janse MJ, Coronel R, Opthof T. Counterpoint: M cells do not have a functional role in the ventricular myocardium of the intact heart. Heart Rhythm. (2011) 8:934–7. doi: 10.1016/j.hrthm.2010.10.048
90. Friedman PL, Stewart JR, Wit AL. Spontaneous and induced cardiac arrhythmias in subendocardial Purkinje fibers surviving extensive myocardial infarction in dogs. Circ Res. (1973) 33:612–26. doi: 10.1161/01.RES.33.5.612
91. Fossa AA. The impact of varying autonomic states on the dynamic beat-to-beat QT-RR and QT-TQ interval relationships. Br J Pharmacol. (2008) 154:1508–15. doi: 10.1038/bjp.2008.123
92. Guglielmini C. Cardiovascular diseases in the ageing dog: diagnostic and therapeutic problems. Vet Res Commun. (2003) 27(Suppl. 1):555–60. doi: 10.1023/B:VERC.0000014216.73396.f6
93. Gorman JH III, Gorman RC, Plappert T, Jackson BM, Hiramatsu Y, St John-Sutton MG, et al. Infarct size and location determine development of mitral regurgitation in the sheep model. J Thor Cardiovasc Surg. (1998) 115:615–22. doi: 10.1016/S0022-5223(98)70326-5
94. Shofti R, Zaretzki A, Cohen E, Engel A, Bar-El Y. The sheep as a model for coronary artery bypass surgery. Lab Anim. (2004) 38:149–57. doi: 10.1258/002367704322968821
95. Locatelli P, Olea FD, Mendiz O, Salmo F, Fazzi L, Hnatiuk A, et al. An ovine model of postinfarction dilated cardiomyopathy in animals with highly variable coronary anatomy. ILAR J. (2011) 52:E16–21. doi: 10.1093/ilar.52.1.E16
96. Ryu S, Yamamoto S, Andersen CR, Nakazawa K, Miyake F, James TN. Intramural Purkinje cell network of sheep ventricles as the terminal pathway of conduction system. Anatom Rec. (2009) 292:12–22. doi: 10.1002/ar.20827
97. Ansari A, Ho SY, Anderson RH. Distribution of the Purkinje fibres in the sheep heart. Anatom Rec. (1999) 254:92–7. doi: 10.1002/(SICI)1097-0185(19990101)254:1<92::AID-AR12>3.0.CO;2-3
98. Walton RD, Martinez ME, Bishop MJ, Hocini M, Haissaguerre M, Plank G, et al. Influence of the Purkinje-muscle junction on transmural repolarization heterogeneity. Cardiovasc Res. (2014) 103:629–40. doi: 10.1093/cvr/cvu165
99. Dardenne A, Fernandez C, Wagner A, Milewski K, Ordanes DR, Mount PA, et al. Benefits of standardizing the treatment of arrhythmias in the sheep (Ovis aries) model of chronic heart failure after myocardial infarction. J Am Assoc Lab Anim Sci. (2013) 52:290–4.
100. Ausma J, Wijffels M, Thone F, Wouters L, Allessie M, Borgers M. Structural changes of atrial myocardium due to sustained atrial fibrillation in the goat. Circulation. (1997) 96:3157–63. doi: 10.1161/01.CIR.96.9.3157
101. Duytschaever M, Blaauw Y, Allessie M. Consequences of atrial electrical remodeling for the anti-arrhythmic action of class IC and class III drugs. Cardiovasc Res. (2005) 67:69–76. doi: 10.1016/j.cardiores.2005.02.019
102. Lipovetsky G, Fenoglio JJ, Gieger M, Srinivasan MR, Dobelle WH. Coronary artery anatomy of the goat. Artif Organs. (1983) 7:238–45. doi: 10.1111/j.1525-1594.1983.tb04192.x
103. Harris AS. Ventricular fibrillation and standstill in coronary occlusion, anoxia and hemorrhage. Feder Proc. (1946) 5(1 Pt 2):41.
104. Kaplinsky E, Ogawa S, Balke CW, Dreifus LS. Two periods of early ventricular arrhythmia in the canine acute myocardial infarction model. Circulation. (1979) 60:397–403. doi: 10.1161/01.CIR.60.2.397
105. Curtis MJ. Characterisation, utilisation and clinical relevance of isolated perfused heart models of ischaemia-induced ventricular fibrillation. Cardiovasc Res. (1998) 39:194–215. doi: 10.1016/S0008-6363(98)00083-2
106. Halperin HR, Paradis N, Ornato JP, Zviman M, Lacorte J, Lardo A, et al. Cardiopulmonary resuscitation with a novel chest compression device in a porcine model of cardiac arrest: improved hemodynamics and mechanisms. J Am Coll Cardiol. (2004) 44:2214–20. doi: 10.1016/j.jacc.2004.08.061
107. Wollborn J, Ruetten E, Schlueter B, Haberstroh J, Goebel U, Schick MA. Standardized model of porcine resuscitation using a custom-made resuscitation board results in optimal hemodynamic management. Am J Emerg Med. (2018) 36:1738–44. doi: 10.1016/j.ajem.2018.01.059
108. Nuss HB, Kaab S, Kass DA, Tomaselli GF, Marban E. Cellular basis of ventricular arrhythmias and abnormal automaticity in heart failure. Am J Physiol. (1999) 277:H80–91. doi: 10.1152/ajpheart.1999.277.1.H80
109. Perrin S. Preclinical research: make mouse studies work. Nature. (2014) 507:423–5. doi: 10.1038/507423a
110. Hackam DG, Redelmeier DA. Translation of research evidence from animals to humans. JAMA. (2006) 296:1731–2. doi: 10.1001/jama.296.14.1731
111. Bebarta V, Luyten D, Heard K. Emergency medicine animal research: does use of randomization and blinding affect the results? Acad Emerg Med. (2003) 10:684–7. doi: 10.1111/j.1553-2712.2003.tb00056.x
112. van der Worp HB, Howells DW, Sena ES, Porritt MJ, Rewell S, O'Collins V, et al. Can animal models of disease reliably inform human studies? PLoS Med. (2010) 7:e1000245. doi: 10.1371/journal.pmed.1000245
113. van der Helm MW, van der Meer AD, Eijkel JC, van den Berg A, Segerink LI. Microfluidic organ-on-chip technology for blood-brain barrier research. Tissue Barriers. (2016) 4:e1142493. doi: 10.1080/21688370.2016.1142493
114. Dunnink A, Sharif S, Oosterhoff P, Winckels S, Montagne D, Beekman J, et al. Anesthesia and arrhythmogenesis in the chronic atrioventricular block dog model. J Cardiovasc Pharmacol. (2010) 55:601–8. doi: 10.1097/FJC.0b013e3181da7768
115. Savage RM, Guth B, White FC, Hagan AD, Bloor CM. Correlation of regional myocardial blood flow and function with myocardial infarct size during acute myocardial ischemia in the conscious pig. Circulation. (1981) 64:699–707. doi: 10.1161/01.CIR.64.4.699
116. Wilson LD, Jeyaraj D, Wan X, Hoeker GS, Said TH, Gittinger M, et al. Heart failure enhances susceptibility to arrhythmogenic cardiac alternans. Heart Rhythm. (2009) 6:251–9. doi: 10.1016/j.hrthm.2008.11.008
117. Sayadi O, Puppala D, Ishaque N, Doddamani R, Merchant FM, Barrett C, et al. A novel method to capture the onset of dynamic electrocardiographic ischemic changes and its implications to arrhythmia susceptibility. J Am Heart Assoc. (2014) 3:e001055. doi: 10.1161/JAHA.114.001055
118. el-Sherif N, Caref EB, Yin H, Restivo M. The electrophysiological mechanism of ventricular arrhythmias in the long QT syndrome. Tridimensional mapping of activation and recovery patterns. Circ Res. (1996) 79:474–92. doi: 10.1161/01.RES.79.3.474
119. Bacic D, Carneiro JS, Bento AA, Nearing BD, Rajamani S, Belardinelli L, et al. Eleclazine, an inhibitor of the cardiac late sodium current, is superior to flecainide in suppressing catecholamine-induced ventricular tachycardia and T-wave alternans in an intact porcine model. Heart Rhythm. (2017) 14:448–54. doi: 10.1016/j.hrthm.2016.10.021
120. Alves Bento AS, Bacic D, Saran Carneiro J, Nearing BD, Fuller H, Justo FA, et al. Selective late INa inhibition by GS-458967 exerts parallel suppression of catecholamine-induced hemodynamically significant ventricular tachycardia and T-wave alternans in an intact porcine model. Heart Rhythm. (2015) 12:2508–14. doi: 10.1016/j.hrthm.2015.07.025
121. Vandersickel N, Bossu A, De Neve J, Dunnink A, Meijborg VMF, van der Heyden MAG, et al. Short-lasting episodes of torsade de pointes in the chronic atrioventricular block dog model have a focal mechanism, while longer-lasting episodes are maintained by re-entry. JACC Clin Electrophysiol. (2017) 3:1565–76. doi: 10.1016/j.jacep.2017.06.016
122. Kouwenhoven WB, Milnor WR, Knickerbocker GG, Chesnut WR. Closed chest defibrillation of the heart. Surgery. (1957) 42:550–61.
123. Dorph E, Wik L, Stromme TA, Eriksen M, Steen PA. Oxygen delivery and return of spontaneous circulation with ventilation:compression ratio 2:30 versus chest compressions only CPR in pigs. Resuscitation. (2004) 60:309–18. doi: 10.1016/j.resuscitation.2003.12.001
124. Safar P, Behringer W, Bottiger BW, Sterz F. Cerebral resuscitation potentials for cardiac arrest. Critic Care Med. (2002) 30(Suppl. 4):S140–4. doi: 10.1097/00003246-200204001-00004
125. Beardslee MA, Lerner DL, Tadros PN, Laing JG, Beyer EC, Yamada KA, et al. Dephosphorylation and intracellular redistribution of ventricular connexin43 during electrical uncoupling induced by ischemia. Circ Res. (2000) 87:656–62. doi: 10.1161/01.RES.87.8.656
126. Dobrev D, Carlsson L, Nattel S. Novel molecular targets for atrial fibrillation therapy. Nat Rev Drug Disc. (2012) 11:275–91. doi: 10.1038/nrd3682
127. Sweeney MO, Prinzen FW. Ventricular pump function and pacing: physiological and clinical integration. Circ Arrhythm Electrophysiol. (2008) 1:127–39. doi: 10.1161/CIRCEP.108.777904
128. Normand C, Linde C, Singh J, Dickstein K. Indications for cardiac resynchronization therapy: a comparison of the major international guidelines. JACC Heart Fail. (2018) 6:308–16. doi: 10.1016/j.jchf.2018.01.022
129. Lin CY, Chen SL, He YL. [Effect of cardiac resynchronization therapy on cardiac function post A-V and V-V optimization]. Zhonghua Xin Xue Guan Bing Za Zhi. (2007) 35:1105–7. doi: 10.3760/j.issn:0253-3758.2007.12.005
130. Aiba T, Tomaselli GF. Electrical remodeling in the failing heart. Curr Opin Cardiol. (2010) 25:29–36. doi: 10.1097/HCO.0b013e328333d3d6
131. Carson P, Anand I, O'Connor C, Jaski B, Steinberg J, Lwin A, et al. Mode of death in advanced heart failure: the Comparison of Medical, Pacing, and Defibrillation Therapies in Heart Failure (COMPANION) trial. J Am Coll Cardiol. (2005) 46:2329–34. doi: 10.1016/j.jacc.2005.09.016
132. Ermis C, Seutter R, Zhu AX, Benditt LC, VanHeel L, Sakaguchi S, et al. Impact of upgrade to cardiac resynchronization therapy on ventricular arrhythmia frequency in patients with implantable cardioverter-defibrillators. J Am Coll Cardiol. (2005) 46:2258–63. doi: 10.1016/j.jacc.2005.04.067
133. Donahue JK. Current state of the art for cardiac arrhythmia gene therapy. Pharmacol Ther. (2017) 176:60–5. doi: 10.1016/j.pharmthera.2017.06.005
134. Cutler MJ, Wan X, Plummer BN, Liu H, Deschenes I, Laurita KR, et al. Targeted sarcoplasmic reticulum Ca2+ ATPase 2a gene delivery to restore electrical stability in the failing heart. Circulation. (2012) 126:2095–104. doi: 10.1161/CIRCULATIONAHA.111.071480
135. Prunier F, Kawase Y, Gianni D, Scapin C, Danik SB, Ellinor PT, et al. Prevention of ventricular arrhythmias with sarcoplasmic reticulum Ca2+ ATPase pump overexpression in a porcine model of ischemia reperfusion. Circulation. (2008) 118:614–24. doi: 10.1161/CIRCULATIONAHA.108.770883
136. Greenberg B, Butler J, Felker GM, Ponikowski P, Voors AA, Desai AS, et al. Calcium upregulation by percutaneous administration of gene therapy in patients with cardiac disease (CUPID 2): a randomised, multinational, double-blind, placebo-controlled, phase 2b trial. Lancet. (2016) 387:1178–86. doi: 10.1016/S0140-6736(16)00082-9
137. Pedersen CM, Venkatasubramanian S, Vase H, Hyldebrandt JA, Contractor H, Schmidt MR, et al. Rotigaptide protects the myocardium and arterial vasculature from ischaemia reperfusion injury. Br J Clin Pharmacol. (2016) 81:1037–45. doi: 10.1111/bcp.12882
138. Hennan JK, Swillo RE, Morgan GA, Keith JC Jr, Schaub RG, Smith RP, et al. Rotigaptide (ZP123) prevents spontaneous ventricular arrhythmias and reduces infarct size during myocardial ischemia/reperfusion injury in open-chest dogs. J Pharmacol Exp Ther. (2006) 317:236–43. doi: 10.1124/jpet.105.096933
139. Xing D, Kjolbye AL, Nielsen MS, Petersen JS, Harlow KW, Holstein-Rathlou NH, et al. ZP123 increases gap junctional conductance and prevents reentrant ventricular tachycardia during myocardial ischemia in open chest dogs. J Cardiovasc Electrophysiol. (2003) 14:510–20. doi: 10.1046/j.1540-8167.2003.02329.x
140. Kudenchuk PJ, Daya M, Dorian P, Resuscitation outcomes consortium I. Amiodarone, lidocaine, or placebo in out-of-hospital cardiac arrest. N Engl J Med. (2016) 375:802–3. doi: 10.1056/NEJMoa1514204
141. De Vuyst E, Boengler K, Antoons G, Sipido KR, Schulz R, Leybaert L. Pharmacological modulation of connexin-formed channels in cardiac pathophysiology. Br J Pharmacol. (2011) 163:469–83. doi: 10.1111/j.1476-5381.2011.01244.x
142. Jeyaraj D, Wan X, Ficker E, Stelzer JE, Deschenes I, Liu H, et al. Ionic bases for electrical remodeling of the canine cardiac ventricle. Am J Physiol Heart Circ Physiol. (2013) 305:H410–9. doi: 10.1152/ajpheart.00213.2013
143. Odenstedt J, Linderoth B, Bergfeldt L, Ekre O, Grip L, Mannheimer C, et al. Spinal cord stimulation effects on myocardial ischemia, infarct size, ventricular arrhythmia, and noninvasive electrophysiology in a porcine ischemia-reperfusion model. Heart Rhythm. (2011) 8:892–8. doi: 10.1016/j.hrthm.2011.01.029
144. Lopshire JC, Zipes DP. Spinal cord stimulation for heart failure: preclinical studies to determine optimal stimulation parameters for clinical efficacy. J Cardiovasc Transl Res. (2014) 7:321–9. doi: 10.1007/s12265-014-9547-7
145. Zipes DP, Neuzil P, Theres H, Caraway D, Mann DL, Mannheimer C, et al. Determining the feasibility of spinal cord neuromodulation for the treatment of chronic systolic heart failure: the DEFEAT-HF study. JACC Heart Fail. (2016) 4:129–36. doi: 10.1016/j.jchf.2015.10.006
146. Tse HF, Turner S, Sanders P, Okuyama Y, Fujiu K, Cheung CW, et al. Thoracic Spinal Cord Stimulation for Heart Failure as a Restorative Treatment (SCS HEART study): first-in-man experience. Heart Rhythm. (2015) 12:588–95. doi: 10.1016/j.hrthm.2014.12.014
147. Wolfram JA, Donahue JK. Gene therapy to treat cardiovascular disease. J Am Heart Assoc. (2013) 2:e000119. doi: 10.1161/JAHA.113.000119
148. Reynolds JC, Rittenberger JC, Menegazzi JJ. Drug administration in animal studies of cardiac arrest does not reflect human clinical experience. Resuscitation. (2007) 74:13–26. doi: 10.1016/j.resuscitation.2006.10.032
149. Gates S, Smith JL, Ong GJ, Brace SJ, Perkins GD. Effectiveness of the LUCAS device for mechanical chest compression after cardiac arrest: systematic review of experimental, observational and animal studies. Heart. (2012) 98:908–13. doi: 10.1136/heartjnl-2011-301571
150. Perkins GD, Lall R, Quinn T, Deakin CD, Cooke MW, Horton J, et al. Mechanical versus manual chest compression for out-of-hospital cardiac arrest (PARAMEDIC): a pragmatic, cluster randomised controlled trial. Lancet. (2015) 385:947–55. doi: 10.1016/S0140-6736(14)61886-9
151. Aigner B KB, Klymiuk N, Kuome M, Renner S, Wunsch A, Wolf E. Genetically tailored pig models for translational biomedical research. In: Michael Conn P, editor. Animal Models for Translational Biomedical Research. 2nd ed. Amsterdam: Elsevier (2017). p. 671–701. doi: 10.1016/B978-0-12-809468-6.00026-7
152. Polejaeva IA, Ranjan R, Davies CJ, Regouski M, Hall J, Olsen AL, et al. Increased susceptibility to atrial fibrillation secondary to atrial fibrosis in transgenic goats expressing transforming growth factor-beta1. J Cardiovasc Electrophysiol. (2016) 27:1220–9. doi: 10.1111/jce.13049
153. Chai S, Wan X, Ramirez-Navarro A, Tesar PJ, Kaufman ES, Ficker E, et al. Physiological genomics identifies genetic modifiers of long QT syndrome type 2 severity. J Clin Invest. (2018) 128:1043–56. doi: 10.1172/JCI94996
154. Sasano T, McDonald AD, Kikuchi K, Donahue JK. Molecular ablation of ventricular tachycardia after myocardial infarction. Nat Med. (2006) 12:1256–8. doi: 10.1038/nm1503
155. Hu YF, Dawkins JF, Cho HC, Marban E, Cingolani E. Biological pacemaker created by minimally invasive somatic reprogramming in pigs with complete heart block. Sci Transl Med. (2014) 6:245ra94. doi: 10.1126/scitranslmed.3008681
156. Girouard SD, Laurita KR, Rosenbaum DS. Unique properties of cardiac action potentials recorded with voltage-sensitive dyes. J Cardiovasc Electrophysiol. (1996) 7:1024–38. doi: 10.1111/j.1540-8167.1996.tb00478.x
157. Lee P, Taghavi F, Yan P, Ewart P, Ashley EA, Loew LM, et al. In situ optical mapping of voltage and calcium in the heart. PLoS ONE. (2012) 7:e42562. doi: 10.1371/journal.pone.0042562
158. Dillon SM, Kerner TE, Hoffman J, Menz V, Li KS, Michele JJ. A system for in-vivo cardiac optical mapping. IEEE Eng Med Biol Magaz. (1998) 17:95–108. doi: 10.1109/51.646226
159. Lee P, Quintanilla JG, Alfonso-Almazan JM, Galan-Arriola C, Yan P, Sanchez-Gonzalez J, et al. In-vivo ratiometric optical mapping enables high-resolution cardiac electrophysiology in pig models. Cardiovasc Res. (2019) 15:1659–71. doi: 10.1093/cvr/cvz039
Keywords: animal models, translational models, sudden cardiac death, resuscitation, arrhythmias
Citation: Piktel JS and Wilson LD (2019) Translational Models of Arrhythmia Mechanisms and Susceptibility: Success and Challenges of Modeling Human Disease. Front. Cardiovasc. Med. 6:135. doi: 10.3389/fcvm.2019.00135
Received: 28 May 2019; Accepted: 27 August 2019;
Published: 10 September 2019.
Edited by:
Sebastian Clauss, Ludwig Maximilian University of Munich, GermanyReviewed by:
Bernhard Maisch, Philipps-Universität Marburg, GermanyKatharina Scherschel, University Medical Center Hamburg-Eppendorf, Germany
Copyright © 2019 Piktel and Wilson. This is an open-access article distributed under the terms of the Creative Commons Attribution License (CC BY). The use, distribution or reproduction in other forums is permitted, provided the original author(s) and the copyright owner(s) are credited and that the original publication in this journal is cited, in accordance with accepted academic practice. No use, distribution or reproduction is permitted which does not comply with these terms.
*Correspondence: Lance D. Wilson, lwilson@metrohealth.org