- 1Jacot Laboratory for Pediatric Regenerative Medicine, Department of Bioengineering, University of Colorado Anschutz Medical Campus, Aurora, CO, United States
- 2Department of Pediatrics, University of Colorado Anschutz Medical Campus, Aurora, CO, United States
Heart disease is the leading cause of death in the United States among both adults and infants. In adults, 5-year survival after a heart attack is <60%, and congenital heart defects are the top killer of liveborn infants. Problematically, the regenerative capacity of the heart is extremely limited, even in newborns. Furthermore, suitable donor hearts for transplant cannot meet the demand and require recipients to use immunosuppressants for life. Tissue engineered myocardium has the potential to replace dead or fibrotic heart tissue in adults and could also be used to permanently repair congenital heart defects in infants. In addition, engineering functional myocardium could facilitate the development of a whole bioartificial heart. Here, we review and compare in vitro and in situ myocardial tissue engineering strategies. In the context of this comparison, we consider three challenges that must be addressed in the engineering of myocardial tissue: recapitulation of myocardial architecture, vascularization of the tissue, and modulation of the immune system. In addition to reviewing and analyzing current progress, we recommend specific strategies for the generation of tissue engineered myocardial patches for heart regeneration and repair.
Introduction
Heart disease is the leading cause of death in the United States (1). Each year, nearly one million Americans suffer myocardial infarctions (MI) that are often fatal and at best lead to necrotic regions of myocardium that cause pathologic remodeling and arrhythmias. Among infants, congenital heart defects (CHD) are the most common cause of death and often require reconstructive surgeries involving the implant of inert patches in the place of myocardium (2, 3). Because the heart has almost no capacity for self-renewal, there is a clinical need for regenerative therapies that can drive myocardial regeneration in MI patients and be used for reconstructive surgeries in CHD patients. Numerous studies of scaffold-free therapies involving the direct delivery of cells, extracellular matrix (ECM), drugs, or biologics for MI patients show promise (4–6). This review, however, will focus predominantly on progress toward a tissue engineered myocardial patch (TEMP) that could be sutured over myocardial infarct regions or full wall-thickness CHD, such as septal defects. To engineer clinically useful tissue for any organ, three principal considerations must be addressed: the architecture of the tissue, vascularization of the engineered tissue, and the host immune response after implant.
The architecture of tissue—the composition and arrangement of its ECM and various cell types—is critically important to the function of all tissue types. As a whole, the heart is designed as an elegant and intricate system of valves, conduits, and pumping chambers that drives blood circulation throughout the body. The muscular heart walls are composed of a thick myocardial layer sandwiched between two single-cell layers called the endocardium (blood-contacting surface) and the epicardium (outer layer of heart). Both the epicardium and endocardium are essential for proper myocardial development, growth, and remodeling (7, 8). In the ventricular myocardium, overlaying layers of cardiomyocytes are oriented in left-handed, circumferential, or right-handed directions, resulting in an efficient torsional “wringing” motion of contraction (9, 10). ECM components include predominantly collagens, but also fibrillins, fibronectin, laminin, and periostin (11). The precise composition, fiber orientation, and crosslinking of the cardiac ECM regulates tissue strength, heart stiffness, cell proliferation, pumping efficiency, and fibroblast behavior (12–18).
In addition to cardiomyocytes, the myocardium is innervated, heavily vascularized, and populated by resident macrophages and cardiac fibroblasts (Figure 1) (19, 20). Vascularization sustains the highly metabolic muscle and allows for muscle hypertrophy during development. A method of nutrient supply is required to generate TEMPs with physiologic cell densities, and rapid anastomosis with host vasculature must be achieved shortly after implant. Within native myocardium, resident macrophages regulate the inflammatory response and are highly active following injuries, such as MI. The host immune response must be considered in TEMPs in avoiding immune rejection and may also be leveraged to improve regenerative therapies. Promoting cardiomyocyte regeneration and connectivity remains a primary hurdle in cardiac repair following malformation or injury. The architecture, vascular supply, and immune response are reviewed here as three primary approaches to coordinate regeneration and integration of engineered cardiac tissue.
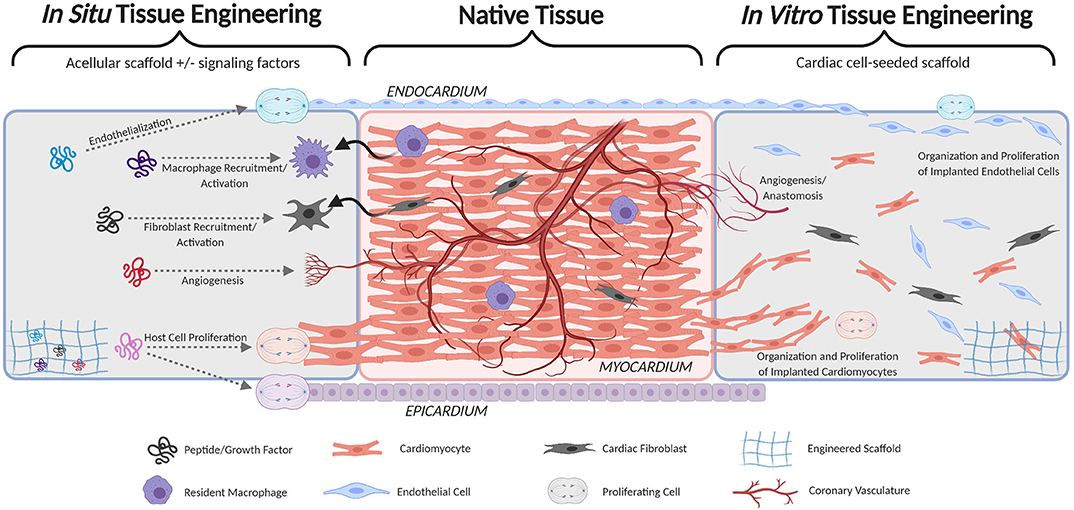
Figure 1. Schematic comparison of cardiac patches implementing in situ cardiac tissue engineering (left) and in vitro cardiac tissue engineering (right). Both seek to fully integrate with native host tissue (middle) by forming continuous endocardium, myocardium, epicardium, and vasculature.
Recapitulating the Architecture of the Heart Wall
In engineering this complex myocardial tissue, the necessary degree of architectural recapitulation is unknown. The in situ tissue engineering approach aims to provide a remodelable scaffold and to harness the natural healing response (inflammation, recellularization, and remodeling) to achieve native tissue architecture and function (Figure 1). Numerous natural (e.g., collagen, fibrin, and native ECM) and synthetic [e.g., poly(glycerol sebacate), polyurethane, and polyethylene glycol] scaffold materials have been tested for myocardial regeneration. These materials enable fine-tuning of various important tissue properties including degradation kinetics, elastic modulus, porosity, and immune response (21–30). In addition, several studies have sought to identify or integrate various signaling factors that can modulate cardiac healing—especially cardiomyocyte proliferation—including growth factors (e.g., NRG-1, FGFs, VEGF, IGF-1), ECM components (e.g., Agrin, Fibronectin, Periostin), and modulators of the Wnt and Hippo pathways (31–36). The in situ approach is attractive because of its simplicity and relative ease of translation (manufacture, storage, handling, etc.), however the regenerative potential of the heart is notoriously limited. Thus, far, no combination of signaling factors and/or ECM components has been shown to drive significant myocardial regeneration. Therefore, a scaffold alone, even if loaded with signaling factors, is unlikely to drive sufficient cardiomyocyte proliferation and migration necessary for total regeneration.
Alternatively, the in vitro tissue engineering approach seeks to create living heart tissue prior to implant by combining a scaffold with cardiomyocytes (Figure 1). Induced pluripotent stem cell-derived cardiomyocytes (iPSC-CM) are the most promising source of cardiomyocytes for tissue engineered myocardium because they can be patient-specific, generated in large quantities, and are relatively proliferative compared to native cardiomyocytes (37, 38). Several versions of engineered myocardium using 3D scaffolds and iPSC-CM have been developed in the past decade (39–51), and these studies unanimously demonstrate that 3D culture of iPSC-CM improves their maturation and connectivity. Combining iPSC-CM with other cardiac cell types in 3D scaffolds has further improved tissue function. In particular, the inclusion of cardiac fibroblasts in tissue engineered myocardium is now considered a near-necessity because of their recently-discovered importance in the viability, organization, durability, and function of engineered and native myocardium (52–55). Coculture with endothelial cells has also been shown to improve iPSC-CM maturity, function, and proliferation and is discussed further in the following section in the context of engineering vasculature (56–58).
Homogenous co-culture of iPSC-CM with other cell types yields clear benefits, but none of these patch designs have sought to recapitulate the trilayer organization (endocardium, myocardium, epicardium) of heart muscle despite the critical signaling roles that these cell layers play on cardiac fibroblast behavior, cardiomyocyte proliferation, and tissue organization. This is likely due to the longstanding difficulty of attaining endocardial and epicardial cells. However, the increasing number of protocols for the differentiation of iPSC-derived endocardial (59) and epicardial (60) cells may open the door for studies investigating their inclusion in tissue engineered myocardium. Recapitulation of this architecture through inclusion of an engineered endocardium and epicardium using iPSC-derived cells and a trilayer scaffold could significantly improve the viability, integration, and growth of implanted TEMPs. Multi-layer scaffolds have been developed and investigated for several soft and hard tissue engineering applications using various fabrication techniques (25, 49, 61–71). Similar approaches could be employed to generate endocardium-myocardium-epicardium tissues in vitro. Such a trilayer tissue would also provide a useful in vitro model for studying aspects of heart development involving cell-layer crosstalk, including myocardial trabeculation and thickening, valve formation, and the epithelial-mesenchymal transition (EMT) of cardiac fibroblasts derived from these two cell layers.
Vascularization of Cardiac Patches in vitro and in situ
Incorporation of vascular cell types into 3D cardiac tissues increases the proliferation and maturation of iPSC-CM and accelerates needed vascularization after implant, resulting in more clinically relevant in vitro tissues. While electrical and mechanical conditioning can also drive iPSC-CM maturation, 3D co-culture with endothelial cells (EC) and stromal cells appears vital in maximizing the functional maturity of iPSC-CM. Early attempts at vascularized cardiac tissues showed that in porous polymer scaffolds, EC combined with embryonic fibroblasts formed networks that did not disrupt the contractility of embryonic stem cell-derived cardiomyocytes. Additionally, the presence of EC increased the proliferation of immature cardiomyocytes and increased the maturation of non-dividing cardiomyocytes (72). Over time, further evidence of the importance of vascular cell types in 3D cardiac tissues has been increasing. EC and smooth muscle cells increased the expression of electrical and mechanical junctions in cardiomyocytes in a fibrin-based hydrogel and helped show that non-myocytes are necessary for the electrical coordination of cardiomyocytes in 3D structures (40). In collagen matrices, addition of EC enhanced the proliferation of cardiomyocytes undergoing stress conditioning (73). Bilayer constructs of cardiomyocytes in a fibrin matrix layered on a microvessel fibrin matrix containing EC and pericytes improved survival, maturation, and twitch force production of the cardiomyocytes compared to constructs without the microvessel layer (43). Furthermore, multiple groups have assessed the drug response of 3D multicell culture systems, unanimously demonstrating that vascularized 3D tissues have significantly different toxicity responses compared to 2D and cardiomyocyte-only systems. These differences have been attributed primarily to more mature contractile properties and calcium handling (74–77). The mechanisms of cellular cross-talk that lead to CM maturation continue to be investigated (78), but it is clear that vascular cell types in 3D culture systems significantly improve CM maturation and function. With evidence of the importance of vascular cell types in cardiac constructs, optimizing the ratio of iPSC-CM to vascular cells has become of interest. Different ratios have been shown to lead to different levels of cardiomyocyte function and maturity (79, 80). However, the optimal ratio may depend on the overall goal and approach to 3D culture (e.g., drug screening vs. therapeutic implant, scaffold-free vs. cell sheet layering) as the degree of vasculature is especially important for in vivo survival.
In addition to improving proliferation and maturation of cardiomyocytes in vitro, vascularization is necessary for creating larger cardiac constructs that survive and engraft in vivo. An in situ approach of releasing angiogenic growth factors from CM patches has shown to increase vessel ingrowth and improve overall outcomes in rodent myocardial infarction models compared to non-angiogenic controls (81, 82). However, the in vitro approach that adds vascular cells to living cardiac constructs may provide quicker and more robust vascular development for MI repair and will be necessary for developing reconstructive tissues for CHD repair. Incorporation of vascular cells into cardiomyocyte constructs led to >10-fold larger cell grafts than cardiomyocyte-only constructs, which became necrotic upon implantation on skeletal muscle (83). Vascular cells in 3D constructs increase the ingrowth of host vasculature and form vessels that can anastomose to the native vasculature and become perfusable (43, 58, 73, 84). The rapid formation of perfusable vasculature in cardiac implants is necessary for survival and function of the implanted iPSC-CM. Recent analysis showed that encapsulated vascular cells that were allowed to self-assemble into networks did not integrate as quickly or to the same degree as vascular cells that were seeded into pre-formed perfusable channels when grafts were implanted onto rat hearts. Consequently, the perfusable vascular grafts showed higher cardiomyocyte and vascular densities within the implant (85). It is important to note that the majority of vascularized cardiac patch research so far has been in rodent models, which have significantly thinner myocardium than larger animals and humans. Scaling up the size of pre-vascularized constructs remains challenging. The number of layered cell sheets of cardiomyocytes and ECs has a limit before necrosis occurs (86). Thus, it appears that engineering scaffolds with perfusable channels or vessels instead of relying on self-assembled vascular networks will be necessary for larger constructs. Potential approaches to robust vasculature in larger tissues have been explored, including decellularizing and then recellularizing pig ventricles connected to a perfusion bioreactor (87), 3D printing vascularized constructs (88), and the AngioChip which can be surgically anastamosed to host vasculature (89).
Harnessing the Immune System in Cardiac Tissue Engineering
The role of the immune system in cardiovascular tissue engineering is becoming increasingly apparent. Research on regenerative therapies and tissue engineering solutions for cardiac tissues has significantly advanced our understanding and ability to modulate both the negative inflammatory response and encourage a tissue repair response through immune pathways. Following myocardial injury, the inflammatory response is critical in helping protect the necrotic infarct area by increasing stiffness and preventing infarct expansion; however, inflammation may also lead to adverse remodeling of the ventricular wall leading to heart failure. Recruitment of inflammatory cells to the site of inflammation occurs through chemokine paracrine signaling, notably via the CCL2 (c-c chemokine ligand 2; MCP-1) and CX3CL1 [chemokine (C-X3-C motif) ligand 1] cytokines and their associated receptors, CCR2 and CX3CR1 (90). Following injury to the myocardium, resident macrophages aid in recruiting CCR2+ macrophages from the bloodstream, which display increased pro-inflammatory (M1) and anti-inflammatory (M2) markers (91). Inhibition of the CCR2 protein resulted in decreased inflammation following MI in mice and decreased adverse remodeling of the ventricular wall, indicating the recruited cells may be responsible for adverse remodeling (92–94).
However, the infiltration of inflammatory cells is not always an adverse response, and in fact may be leveraged to enhance tissue-engineering strategies. The majority of research into this space has been tested using direct injection of cells into infract zones. It was originally believed cell therapy acts via integration of seeded cells, though it is now thought to act through paracrine signaling and recruitment of autologous cells (95). The inflammatory response is critically linked to this process as demonstrated by an observed reduction in heart regeneration when macrophages are depleted pre-injury (96). Recent work into the mechanisms provides greater insight. Mononuclear cells or the immune activator zymosan injected into a mouse heart post-MI was shown to increase recruitment of CCR2+ and CX3CR1+ monocytes, which increased heart function (90). Other approaches have aimed at directly modulating the immune response with cytokines. Pre-treating MSCs with the pro-inflammatory cytokine TNFα prior to injection in an MI rat model led to increased recovery of cardiac function (97). Further work using genetic knockouts found this cardiac protection acts via the TNFR2 receptor, while the TNFR1 receptor does not aid in cardiac protection (98). Other cytokines tested for modulating the immune response include interleukin-10 (IL-10), SDF1a, and RANTES (99–103).
While much of the work in modulating the inflammatory response has focused on injectable approaches, the inflammatory response is equally important in the context of TEMPs. The structure, pore size, material composition, and surface topology of scaffold materials affect the host inflammatory response by modulating cell recruitment, infiltration, and activation (104). The source of seeded cells also influences the inflammatory response. Autologous cell sources are preferred, however time-consuming cell culture is required for reprogramming and differentiation. On the other hand, an off-the-shelf cell source requires HLA type matching. Thus, there is interest in generating HLA-null master iPSCs as a hypo-immunogenic cell source (105). Aside from scaffold and cell considerations, there has been very little work in modulating the immune response with a myocardial patch despite the obvious role it plays in both cardiac injury and healing (106). The MAGNUM clinical trial was an early attempt at a myocardial patch seeded with bone marrow mononuclear cells and demonstrated slight success, likely acting through inflammatory pathways (107). There is great potential in modulating the immune response, both in limiting an adverse response and promoting a healing response, and future work in cardiac tissue engineering should consider leveraging these pathways to enhance outcomes. Specifically, the inclusion of immunomodulatory molecules within a TEMP could be used to positively direct the inflammatory response. For example, since the temporal availability of injected cytokines is short, hydrogels of varying degradation rates have been tested to provide a time-controlled release of Met-CCL5 and CXCL12. These hydrogels led to decreased early neutrophil infiltration, increased neovascularization, and decreased apoptosis in the infarcted myocardium (101). Finally, it should be noted that heart disease patients are not in perfect health, so the pre-existing inflammatory state of the heart must be considered. For example, atherosclerosis, the leading cause of MI, is modulated via inflammatory pathways, specifically CCL2 (108). This leads to chronic inflammation in the surrounding tissues such that following MI, the recruited macrophages are infiltrating a site of chronic inflammation, altering their response and delaying or preventing a regenerative phase (109). Such considerations are often not taken into account for many of the animal models used to test MI therapies, as the injury is often acutely simulated and no pre-existing inflammatory state exists. Cardiac tissue engineering, either via the in situ or in vitro approach, must consider the pre-existing inflamed status of ischemic myocardium as it may alter the regenerative outcome of the implanted biomaterial.
Conclusions
The prevalence of heart disease throughout the human population and the limited supply of donor hearts has created a need for TEMPs that could be used to regenerate myocardial infarct regions or in reconstructive surgeries in CHD patients. We think that an in situ (acellular) tissue engineering strategy would require a suturable, biodegradable scaffold loaded with angiogenic and immunomodulatory signaling factors that encourage both angiogenesis and a healing immune response. However, given the extremely low proliferation rate of cardiomyocytes, we believe that an in vitro (cellularized) tissue engineering approach is the most promising. We think that such a TEMP requires at minimum a biodegradable scaffold seeded with either (1) a low density of proliferative iPSC-CM, cardiac fibroblasts, and EC (that can survive by simple media/blood diffusion prior to the development of a proper vasculature) or (2) a high, more-physiologic density of these cell types in a scaffold that includes engineered vascular channels that can provide perfusion in vitro and rapid anastomosis in vivo. Recapitulation of cardiac innervation in engineered patches could be necessary for complete patch integration in vivo, however action potentials in the myocardium are primarily transmitted through the cardiomyocyte syncytium (110). Therefore, promoting cardiomyocyte regeneration and connectivity is likely to be more important in cardiac tissue engineering than the inclusion of neural networks.
In addition to these minimum requirements of an in vitro TEMP, we postulate that a more complex TEMP that also recapitulates the endocardium and epicardium is the most promising approach for heart regeneration. With any of these strategies, the rate of TEMP integration in the host heart needs to exceed the degradation rate of the scaffold to prevent patch rupture during remodeling. In addition, the scaffold and its degradation products need to be tolerated by the host immune system but should also be considered an immunomodulatory tool that can be leveraged for improved healing and integration. Maintaining the viability of implanted iPSC-cardiomyocytes also remains a challenge that must be addressed prior to clinical translation. The inclusion of an endocardium in the patch, optimizing the maturation level of the cardiomyocytes, and modulating the host immune response are all potential strategies for increasing iPSC-CM viability and electrical integration after implantation.
Tissue engineered myocardium represents an important step toward the cure of heart disease and would also be a significant milestone as the field moves toward the eventual engineering of a whole bioartificial heart. Furthermore, the considerations, challenges, and advancements reviewed herein are translatable to many different tissue engineering endeavors, but especially to other cardiovascular applications including tissue engineered heart valves and large vessels.
Author Contributions
DJ, EV, MV, and JJ contributed equally to conceptualization of the work. DJ was responsible for manuscript organization, figure development, and primary authorship. EV was the lead author on section Vascularization of Cardiac Patches in vitro and in situ. MV was the lead author on section Harnessing the Immune System in Cardiac Tissue Engineering. All authors contributed to the article and approved the submitted version.
Funding
This work was supported by the National Science Foundation grant number DGE-1553798 (awarded to DJ), the National Institute of Health grant numbers 5T32HL072738-16 (awarded to EV) and HL130436 (awarded to JJ), and the American Heart Association grant number 19POST34380541 (awarded to MV).
Conflict of Interest
The authors declare that the research was conducted in the absence of any commercial or financial relationships that could be construed as a potential conflict of interest.
References
1. Benjamin EJ, Muntner P, Alonso A, Bittencourt MS, Callaway CW, Carson AP, et al. Heart disease and stroke statistics-2019 update: a report from the American Heart Association. Circulation. (2019) 139:e56–28. doi: 10.1161/CIR.0000000000000659
2. Miranovic V. The incidence of congenital heart disease: previous findings and perspectives. Srp Arh Celok Lek. (2014) 142:243–8. doi: 10.2298/SARH1404243M
3. Wu W, He J, Shao X. Incidence and mortality trend of congenital heart disease at the global, regional, national level, 1990–2017. Medicine. (2020) 99:e20593. doi: 10.1097/MD.0000000000020593
4. Liu YW, Chen B, Yang X, Fugate JA, Kalucki FA, Futakuchi-Tsuchida A, et al. Human embryonic stem cell-derived cardiomyocytes restore function in infarcted hearts of non-human primates. Nat Biotechnol. (2018) 36:597–605. doi: 10.1038/nbt.4162
5. Seif-Naraghi SB, Singelyn JM, Salvatore MA, Osborn KG, Wang JJ, Sampat U, et al. Safety and efficacy of an injectable extracellular matrix hydrogel for treating myocardial infarction. Sci Transl Med. (2013) 5:173ra25. doi: 10.1126/scitranslmed.3005503
6. Bartunek J, Sherman W, Vanderheyden M, Fernandez-Aviles F, Wijns W, Terzic A. Delivery of biologics in cardiovascular regenerative medicine. Clin Pharmacol Ther. (2009) 85:548–52. doi: 10.1038/clpt.2008.295
7. Jarrell DK, Lennon ML, Jacot JG. Epigenetics and mechanobiology in heart development and congenital heart disease. Diseases. (2019) 7:52. doi: 10.3390/diseases7030052
8. Haack T, Abdelilah-Seyfried S. The force within: endocardial development, mechanotransduction and signalling during cardiac morphogenesis. Development. (2016) 143:373–86. doi: 10.1242/dev.131425
9. Goffinet C, Chenot F, Robert A, Pouleur AC, le Polain de Waroux JB, Vancrayenest D, et al. Assessment of subendocardial vs. subepicardial left ventricular rotation and twist using two-dimensional speckle tracking echocardiography: comparison with tagged cardiac magnetic resonance. Eur Heart J. (2009) 30:608–17. doi: 10.1093/eurheartj/ehn511
10. Nakatani S. Left ventricular rotation and twist: why should we learn? J Cardiovasc Ultrasound. (2011) 19:1–6. doi: 10.4250/jcu.2011.19.1.1
11. Williams C, Quinn KP, Georgakoudi I, Black LD III. Young developmental age cardiac extracellular matrix promotes the expansion of neonatal cardiomyocytes in vitro. Acta Biomater. (2014) 10:194–204. doi: 10.1016/j.actbio.2013.08.037
12. Weng Y, Chen T, Ren J, Lu D, Liu X, Lin S, et al. The association between extracellular matrix metalloproteinase inducer polymorphisms and coronary heart disease: a potential way to predict disease. DNA Cell Biol. (2020) 39:244–54. doi: 10.1089/dna.2019.5015
13. Fan D, Takawale A, Lee J, Kassiri Z. Cardiac fibroblasts, fibrosis and extracellular matrix remodeling in heart disease. Fibrogenesis Tissue Repair. (2012) 5:15. doi: 10.1186/1755-1536-5-15
14. Lockhart M, Wirrig E, Phelps A, Wessels A. Extracellular matrix and heart development. Birth Defects Res A Clin Mol Teratol. (2011) 91:535–50. doi: 10.1002/bdra.20810
15. Frangogiannis NG, Kovacic JC. Extracellular matrix in ischemic heart disease, part 4/4: JACC focus seminar. J Am Coll Cardiol. (2020) 75:2219–35. doi: 10.1016/j.jacc.2020.03.020
16. Imanaka-Yoshida K, Hiroe M, Yoshida T. Interaction between cell and extracellular matrix in heart disease: multiple roles of tenascin-C in tissue remodeling. Histol Histopathol. (2004) 19:517–25. doi: 10.14670/HH-19.517
17. Christensen G, Herum KM, Lunde IG. Sweet, yet underappreciated: proteoglycans and extracellular matrix remodeling in heart disease. Matrix Biol. (2019) 75-76:286–99. doi: 10.1016/j.matbio.2018.01.001
18. Notari M, Ventura-Rubio A, Bedford-Guaus SJ, Jorba I, Mulero L, Navajas D, et al. The local microenvironment limits the regenerative potential of the mouse neonatal heart. Sci Adv. (2018) 4:eaao5553. doi: 10.1126/sciadv.aao5553
19. Gray GA Toor IS Castellan R Crisan M Meloni M. Resident cells of the myocardium: more than spectators in cardiac injury, repair and regeneration. Curr Opin Physiol. (2018) 1:46–51. doi: 10.1016/j.cophys.2017.08.001
20. Ammirati E, Kaski JP. Resident inflammatory cells in the myocardium of children: on the way to set histologic reference standards to differentiate normal myocardium from myocarditis. Int J Cardiol. (2020) 303:64–5. doi: 10.1016/j.ijcard.2019.12.039
21. Williams C, Budina E, Stoppel WL, Sullivan KE, Emani S, Emani SM, et al. Cardiac extracellular matrix-fibrin hybrid scaffolds with tunable properties for cardiovascular tissue engineering. Acta Biomater. (2015) 14:84–95. doi: 10.1016/j.actbio.2014.11.035
22. Muniyandi P, Palaninathan V, Veeranarayanan S, Ukai T, Maekawa T, Hanajiri T, et al. ECM mimetic electrospun porous poly (L-lactic acid) (PLLA) scaffolds as potential substrates for cardiac tissue engineering. Polymers. (2020) 12:451. doi: 10.3390/polym12020451
23. Elamparithi A, Punnoose AM, Kuruvilla S. Electrospun type 1 collagen matrices preserving native ultrastructure using benign binary solvent for cardiac tissue engineering. Artif Cells Nanomed Biotechnol. (2016) 44:1318–25. doi: 10.3109/21691401.2015.1029629
24. Yuan Ye K, Sullivan KE, Black LD. Encapsulation of cardiomyocytes in a fibrin hydrogel for cardiac tissue engineering. J Vis Exp. (2011) 55:3251. doi: 10.3791/3251
25. Tao ZW, Wu S, Cosgriff-Hernandez EM, Jacot JG. Evaluation of a polyurethane-reinforced hydrogel patch in a rat right ventricle wall replacement model. Acta Biomater. (2020) 101:206–18. doi: 10.1016/j.actbio.2019.10.026
26. Castilho M, Feyen D, Flandes-Iparraguirre M, Hochleitner G, Groll J, Doevendans PAF, et al. Melt electrospinning writing of poly-Hydroxymethylglycolide-co-epsilon-caprolactone-based scaffolds for cardiac tissue engineering. Adv Healthc Mater. (2017) 6:311. doi: 10.1002/adhm.201700311
27. Iyer RK, Chiu LL, Radisic M. Microfabricated poly(ethylene glycol) templates enable rapid screening of triculture conditions for cardiac tissue engineering. J Biomed Mater Res A. (2009) 89:616–31. doi: 10.1002/jbm.a.32014
28. Kaiser NJ, Kant RJ, Minor AJ, Coulombe KLK. Optimizing blended collagen-fibrin hydrogels for cardiac tissue engineering with human iPSC-derived cardiomyocytes. ACS Biomater Sci Eng. (2019) 5:887–99. doi: 10.1021/acsbiomaterials.8b01112
29. Vogt L, Rivera LR, Liverani L, Piegat A, El Fray M, Boccaccini AR. Poly(epsilon-caprolactone)/poly(glycerol sebacate) electrospun scaffolds for cardiac tissue engineering using benign solvents. Mater Sci Eng C Mater Biol Appl. (2019) 103:109712. doi: 10.1016/j.msec.2019.04.091
30. Liu Q, Tian S, Zhao C, Chen X, Lei I, Wang Z, et al. Porous nanofibrous poly(L-lactic acid) scaffolds supporting cardiovascular progenitor cells for cardiac tissue engineering. Acta Biomater. (2015) 26:105–14. doi: 10.1016/j.actbio.2015.08.017
31. Zhou QG, Li L, Zhao B, Guan KL. The Hippo pathway in heart development, regeneration, and diseases. Circ Res. (2015) 116:1431–47. doi: 10.1161/CIRCRESAHA.116.303311
32. Bassat E, Mutlak YE, Genzelinakh A, Shadrin IY, Baruch Umansky K, Yifa O, et al. The extracellular matrix protein agrin promotes heart regeneration in mice. Nature. (2017) 547:179–84. doi: 10.1038/nature22978
33. Morikawa Y, Heallen T, Leach J, Xiao Y, Martin JF. Dystrophin-glycoprotein complex sequesters Yap to inhibit cardiomyocyte proliferation. Nature. (2017) 547:227–31. doi: 10.1038/nature22979
34. Mohri Z, Del Rio Hernandez A, Krams R. The emerging role of YAP/TAZ in mechanotransduction. J Thorac Dis. (2017) 9:E507–9. doi: 10.21037/jtd.2017.03.179
35. Zacchigna S, Giacca M. Extra- and intracellular factors regulating cardiomyocyte proliferation in postnatal life. Cardiovasc Res. (2014) 102:312–20. doi: 10.1093/cvr/cvu057
36. Fan Y, Ho BX, Pang JKS, Pek NMQ, Hor JH, Ng SY, et al. Wnt/beta-catenin-mediated signaling re-activates proliferation of matured cardiomyocytes. Stem Cell Res Ther. (2018) 9:338. doi: 10.1186/s13287-018-1086-8
37. Karakikes I, Ameen M, Termglinchan V, Wu JC. Human induced pluripotent stem cell-derived cardiomyocytes: insights into molecular, cellular, functional phenotypes. Circ Res. (2015) 117:80–8. doi: 10.1161/CIRCRESAHA.117.305365
38. Pavlovic BJ, Blake LE, Roux J, Chavarria C, Gilad Y. A comparative assessment of human and chimpanzee iPSC-derived cardiomyocytes with primary heart tissues. Sci Rep. (2018) 8:15312. doi: 10.1038/s41598-018-33478-9
39. Gao L, Gregorich ZR, Zhu W, Mattapally S, Oduk Y, Lou X, et al. Large cardiac muscle patches engineered from human induced-pluripotent stem cell-derived cardiac cells improve recovery from myocardial infarction in swine. Circulation. (2018) 137:1712–30. doi: 10.1161/CIRCULATIONAHA.117.030785
40. Liau B, Christoforou N, Leong KW, Bursac N. Pluripotent stem cell-derived cardiac tissue patch with advanced structure and function. Biomaterials. (2011) 32:9180–7. doi: 10.1016/j.biomaterials.2011.08.050
41. Moreira R, Neusser C, Kruse M, Mulderrig S, Wolf F, Spillner J, et al. Tissue-engineered fibrin-based heart valve with bio-inspired textile reinforcement. Adv Healthc Mater. (2016) 5:2113–21. doi: 10.1002/adhm.201600300
42. Pok S, Benavides OM, Hallal P, Jacot JG. Use of myocardial matrix in a chitosan-based full-thickness heart patch. Tissue Eng Part A. (2014) 20:1877–87. doi: 10.1089/ten.tea.2013.0620
43. Schaefer JA, Guzman PA, Riemenschneider SB, Kamp TJ, Tranquillo RT. A cardiac patch from aligned microvessel and cardiomyocyte patches. J Tissue Eng Regen Med. (2018) 12:546–56. doi: 10.1002/term.2568
44. Scully BB, Fan C, Grigoryan B, Jacot JG, Vick GW III, Kim JJ, et al. Remodeling of ECM patch into functional myocardium in an ovine model: a pilot study. J Biomed Mater Res B Appl Biomater. (2016) 104:1713–20. doi: 10.1002/jbm.b.33484
45. Vunjak Novakovic G, Eschenhagen T, Mummery C. Myocardial tissue engineering: in vitro models. Cold Spring Harb Perspect Med. (2014) 4:a014076. doi: 10.1101/cshperspect.a014076
46. Izadifar M, Chapman D, Babyn P, Chen X, Kelly ME. UV-assisted 3D bioprinting of nanoreinforced hybrid cardiac patch for myocardial tissue engineering. Tissue Eng Part C Methods. (2017) 24:74–88. doi: 10.1089/ten.tec.2017.0346
47. Kerscher P, Turnbull IC, Hodge AJ, Kim J, Seliktar D, Easley CJ, et al. Direct hydrogel encapsulation of pluripotent stem cells enables ontomimetic differentiation and growth of engineered human heart tissues. Biomaterials. (2016) 83:383–95. doi: 10.1016/j.biomaterials.2015.12.011
48. Kraehenbuehl TP, Ferreira LS, Hayward AM, Nahrendorf M, van der Vlies AJ, Vasile E, et al. Human embryonic stem cell-derived microvascular grafts for cardiac tissue preservation after myocardial infarction. Biomaterials. (2011) 32:1102–9. doi: 10.1016/j.biomaterials.2010.10.005
49. Pok S, Stupin IV, Tsao C, Pautler RG, Gao Y, Nieto RM, et al. Full-thickness heart repair with an engineered multilayered myocardial patch in rat model. Adv Healthc Mater. (2017) 6:549. doi: 10.1002/adhm.201600549
50. Shadrin IY, Allen BW, Qian Y, Jackman CP, Carlson AL, Juhas ME, et al. Cardiopatch platform enables maturation and scale-up of human pluripotent stem cell-derived engineered heart tissues. Nat Commun. (2017) 8:1825. doi: 10.1038/s41467-017-01946-x
51. Zhang D, Shadrin IY, Lam J, Xian HQ, Snodgrass HR, Bursac N. Tissue-engineered cardiac patch for advanced functional maturation of human ESC-derived cardiomyocytes. Biomaterials. (2013) 34:5813–20. doi: 10.1016/j.biomaterials.2013.04.026
52. Li Y, Asfour H, Bursac N. Age-dependent functional crosstalk between cardiac fibroblasts and cardiomyocytes in a 3D engineered cardiac tissue. Acta Biomater. (2017) 55:120–30. doi: 10.1016/j.actbio.2017.04.027
53. Liau B, Jackman CP, Li Y, Bursac N. Developmental stage-dependent effects of cardiac fibroblasts on function of stem cell-derived engineered cardiac tissues. Sci Rep. (2017) 7:42290. doi: 10.1038/srep42290
54. Lighthouse JK, Burke RM, Velasquez LS, Dirkx RA Jr, Aiezza A II, Moravec CS, et al. Exercise promotes a cardioprotective gene program in resident cardiac fibroblasts. JCI Insight. (2019) 4:e92098. doi: 10.1172/jci.insight.92098
55. Xiang FL, Fang M, Yutzey KE. Loss of beta-catenin in resident cardiac fibroblasts attenuates fibrosis induced by pressure overload in mice. Nat Commun. (2017) 8:712. doi: 10.1038/s41467-017-00840-w
56. Dunn KK, Reichardt IM, Simmons AD, Jin G, Floy ME, Hoon KM, et al. Coculture of endothelial cells with human pluripotent stem cell-derived cardiac progenitors reveals a differentiation stage-specific enhancement of cardiomyocyte maturation. Biotechnol J. (2019) 14:e1800725. doi: 10.1002/biot.201800725
57. Varzideh F, Mahmoudi E, Pahlavan S. Coculture with noncardiac cells promoted maturation of human stem cell-derived cardiomyocyte microtissues. J Cell Biochem. (2019) 120:16681–91. doi: 10.1002/jcb.28926
58. Sekine H, Shimizu T, Hobo K, Sekiya S, Yang J, Yamato M, et al. Endothelial cell coculture within tissue-engineered cardiomyocyte sheets enhances neovascularization and improves cardiac function of ischemic hearts. Circulation. (2008) 118(14 Suppl.):S145–52. doi: 10.1161/CIRCULATIONAHA.107.757286
59. Neri T, Hiriart E, van Vliet PP, Faure E, Norris RA, Farhat B, et al. Human pre-valvular endocardial cells derived from pluripotent stem cells recapitulate cardiac pathophysiological valvulogenesis. Nat Commun. (2019) 10:1929. doi: 10.1038/s41467-019-09459-5
60. Zhao J, Cao H, Tian L, Huo W, Zhai K, Wang P, et al. Efficient differentiation of TBX18(+)/WT1(+) epicardial-like cells from human pluripotent stem cells using small molecular compounds. Stem Cells Dev. (2017) 26:528–540. doi: 10.1089/scd.2016.0208
61. Ali IH, Khalil IA, El-Sherbiny IM. Phenytoin/sildenafil loaded poly(lactic acid) bilayer nanofibrous scaffolds for efficient orthopedics regeneration. Int J Biol Macromol. (2019) 136:154–64. doi: 10.1016/j.ijbiomac.2019.06.048
62. Arasteh S, Kazemnejad S, Khanjani S, Heidari-Vala H, Akhondi MM, Mobini S. Fabrication and characterization of nano-fibrous bilayer composite for skin regeneration application. Methods. (2016) 99:3–12. doi: 10.1016/j.ymeth.2015.08.017
63. Dos Santos VI, Merlini C, Aragones A, Cesca K, Fredel MC. In vitro evaluation of bilayer membranes of PLGA/hydroxyapatite/beta-tricalcium phosphate for guided bone regeneration. Mater Sci Eng C Mater Biol Appl. (2020) 112:110849. doi: 10.1016/j.msec.2020.110849
64. Figueira DR, Miguel SP, de Sa KD, Correia IJ. Production and characterization of polycaprolactone- hyaluronic acid/chitosan- zein electrospun bilayer nanofibrous membrane for tissue regeneration. Int J Biol Macromol. (2016) 93(Pt A):1100–10. doi: 10.1016/j.ijbiomac.2016.09.080
65. Franco RA, Min YK, Yang HM, Lee BT. Fabrication and biocompatibility of novel bilayer scaffold for skin tissue engineering applications. J Biomater Appl. (2013) 27:605–15. doi: 10.1177/0885328211416527
66. Li X, Huang L, Li L, Tang Y, Liu Q, Xie H, et al. Biomimetic dual-oriented/bilayered electrospun scaffold for vascular tissue engineering. J Biomater Sci Polym Ed. (2020) 31:439–55. doi: 10.1080/09205063.2019.1697171
67. Liu J, Zou Q, Cai B, Wei J, Yuan C, Li Y. Heparin conjugated PCL/Gel - PCL/Gel/n-HA bilayer fibrous membrane for potential regeneration of soft and hard tissues. J Biomater Sci Polym Ed. (2020) 31:1421–36. doi: 10.1080/09205063.2020.1760700
68. Ma J, Wang H, He B, Chen J. A preliminary in vitro study on the fabrication and tissue engineering applications of a novel chitosan bilayer material as a scaffold of human neofetal dermal fibroblasts. Biomaterials. (2001) 22:331–6. doi: 10.1016/S0142-9612(00)00188-5
69. Re'em T, Witte F, Willbold E, Ruvinov E, Cohen S. Simultaneous regeneration of articular cartilage and subchondral bone induced by spatially presented TGF-beta and BMP-4 in a bilayer affinity binding system. Acta Biomater. (2012) 8:3283–93. doi: 10.1016/j.actbio.2012.05.014
70. Zhao Y, He Y, Guo JH, Wu JS, Zhou Z, Zhang M, et al. Time-dependent bladder tissue regeneration using bilayer bladder acellular matrix graft-silk fibroin scaffolds in a rat bladder augmentation model. Acta Biomater. (2015) 23:91–102. doi: 10.1016/j.actbio.2015.05.032
71. Pok S, Myers JD, Madihally SV, Jacot JG. A multilayered scaffold of a chitosan and gelatin hydrogel supported by a PCL core for cardiac tissue engineering. Acta Biomater. (2013) 9:5630–42. doi: 10.1016/j.actbio.2012.10.032
72. Caspi O, Lesman A, Basevitch Y, Gepstein A, Arbel G, Habib IH, et al. Tissue engineering of vascularized cardiac muscle from human embryonic stem cells. Circ Res. (2007) 100:263–72. doi: 10.1161/01.RES.0000257776.05673.ff
73. Tulloch NL, Muskheli V, Razumova MV, Korte FS, Regnier M, Hauch KD, et al. Growth of engineered human myocardium with mechanical loading and vascular coculture. Circ Res. (2011) 109:47–59. doi: 10.1161/CIRCRESAHA.110.237206
74. Amano Y, Nishiguchi A, Matsusaki M, Iseoka H, Miyagawa S, Sawa Y, et al. Development of vascularized iPSC derived 3D-cardiomyocyte tissues by filtration Layer-by-Layer technique and their application for pharmaceutical assays. Acta Biomater. (2016) 33:110–21. doi: 10.1016/j.actbio.2016.01.033
75. Ravenscroft SM, Pointon A, Williams AW, Cross MJ, Sidaway JE. Cardiac non-myocyte cells show enhanced pharmacological function suggestive of contractile maturity in stem cell derived cardiomyocyte microtissues. Toxicol Sci. (2016) 152:99–112. doi: 10.1093/toxsci/kfw069
76. Giacomelli E, Bellin M, Sala L, Van Meer BJ, Tertoolen LGJ, Orlova VV, et al. Three-dimensional cardiac microtissues composed of cardiomyocytes and endothelial cells co-differentiated from human pluripotent stem cells. Development. (2017) 144:1008–17. doi: 10.1242/dev.143438
77. Pointon A, Pilling J, Dorval T, Wang Y, Archer C, Pollard C. From the cover: high-throughput imaging of cardiac microtissues for the assessment of cardiac contraction during drug discovery. Toxicol Sci. (2017) 155:444–57. doi: 10.1093/toxsci/kfw227
78. Giacomelli E, Meraviglia V, Campostrini G, Cochrane A, Cao X, van Helden RWJ, et al. Human-iPSC-derived cardiac stromal cells enhance maturation in 3D cardiac microtissues and reveal non-cardiomyocyte contributions to heart disease. Cell Stem Cell. (2020) 26:862–79.e11. doi: 10.1016/j.stem.2020.05.004
79. Iseoka H, Miyagawa S, Fukushima S, Saito A, Masuda S, Yajima S, et al. Pivotal role of non-cardiomyocytes in electromechanical and therapeutic potential of induced pluripotent stem cell-derived engineered cardiac tissue. Tissue Eng Part A. (2018) 24:287–300. doi: 10.1089/ten.tea.2016.0535
80. Maiullari F, Costantini M, Milan M, Pace V, Chirivi M, Maiullari S, et al. A multi-cellular 3D bioprinting approach for vascularized heart tissue engineering based on HUVECs and iPSC-derived cardiomyocytes. Sci Rep. (2018) 8:13532. doi: 10.1038/s41598-018-31848-x
81. Lakshmanan R, Kumaraswamy P, Krishnan UM, Sethuraman S. Engineering a growth factor embedded nanofiber matrix niche to promote vascularization for functional cardiac regeneration. Biomaterials. (2016) 97:176–95. doi: 10.1016/j.biomaterials.2016.02.033
82. Munarin F, Kant RJ, Rupert CE, Khoo A, Coulombe KLK. Engineered human myocardium with local release of angiogenic proteins improves vascularization and cardiac function in injured rat hearts. Biomaterials. (2020) 251:120033. doi: 10.1016/j.biomaterials.2020.120033
83. Stevens KR, Kreutziger KL, Dupras SK, Korte FS, Regnier M, Muskheli V, et al. Physiological function and transplantation of scaffold-free and vascularized human cardiac muscle tissue. Proc Natl Acad Sci USA. (2009) 106:16568–73. doi: 10.1073/pnas.0908381106
84. Riemenschneider SB, Mattia DJ, Wendel JS, Schaefer JA, Ye L, Guzman PA, et al. Inosculation and perfusion of pre-vascularized tissue patches containing aligned human microvessels after myocardial infarction. Biomaterials. (2016) 97:51–61. doi: 10.1016/j.biomaterials.2016.04.031
85. Redd MA, Zeinstra N, Qin W, Wei W, Martinson A, Wang Y, et al. Patterned human microvascular grafts enable rapid vascularization and increase perfusion in infarcted rat hearts. Nat Commun. (2019) 10:584. doi: 10.1038/s41467-019-08388-7
86. Sakaguchi K, Shimizu T, Okano T. Construction of three-dimensional vascularized cardiac tissue with cell sheet engineering. J Control Release. (2015) 205:83–8. doi: 10.1016/j.jconrel.2014.12.016
87. Sarig U, Nguyen EB, Wang Y, Ting S, Bronshtein T, Sarig H, et al. Pushing the envelope in tissue engineering: ex vivo production of thick vascularized cardiac extracellular matrix constructs. Tissue Eng Part A. (2015) 21:1507–19. doi: 10.1089/ten.tea.2014.0477
88. Kolesky DB, Homan KA, Skylar-Scott MA, Lewis JA. Three-dimensional bioprinting of thick vascularized tissues. Proc Natl Acad Sci USA. (2016) 113:3179–84. doi: 10.1073/pnas.1521342113
89. Zhang B, Montgomery M, Chamberlain MD, Ogawa S, Korolj A, Pahnke A, et al. Biodegradable scaffold with built-in vasculature for organ-on-a-chip engineering and direct surgical anastomosis. Nat Mater. (2016) 15:669–78. doi: 10.1038/nmat4570
90. Vagnozzi RJ, Maillet M, Sargent MA, Khalil H, Johansen AKZ, Schwanekamp JA, et al. An acute immune response underlies the benefit of cardiac stem cell therapy. Nature. (2020) 577:405–9. doi: 10.1038/s41586-019-1802-2
91. Bajpai G, Bredemeyer A, Li W, Zaitsev K, Koenig AL, Lokshina I, et al. Tissue resident CCR2- and CCR2+ cardiac macrophages differentially orchestrate monocyte recruitment and fate specification following myocardial injury. Circ Res. (2019) 124:263–78. doi: 10.1161/CIRCRESAHA.118.314028
92. Dewald O, Zymek P, Winkelmann K, Koerting A, Ren G, Abou-Khamis T, et al. CCL2/Monocyte Chemoattractant Protein-1 regulates inflammatory responses critical to healing myocardial infarcts. Circ Res. (2005) 96:881–9. doi: 10.1161/01.RES.0000163017.13772.3a
93. Kaikita K, Hayasaki T, Okuma T, Kuziel WA, Ogawa H, Takeya M. Targeted deletion of CC chemokine receptor 2 attenuates left ventricular remodeling after experimental myocardial infarction. Am J Pathol. (2004) 165:439–47. doi: 10.1016/S0002-9440(10)63309-3
94. Majmudar MD, Keliher EJ, Heidt T, Leuschner F, Truelove J, Sena BF, et al. Monocyte-directed RNAi targeting CCR2 improves infarct healing in atherosclerosis-prone mice. Circulation. (2013) 127:2038–46. doi: 10.1161/CIRCULATIONAHA.112.000116
95. Zwetsloot PP Vegh AM Jansen of Lorkeers SJ van Hout GP Currie GL Sena ES . Cardiac stem cell treatment in myocardial infarction: a systematic review and meta-analysis of preclinical studies. Circ Res. (2016) 118:1223–32. doi: 10.1161/CIRCRESAHA.115.307676
96. Ben-Mordechai T, Holbova R, Landa-Rouben N, Harel-Adar T, Feinberg MS, Abd Elrahman I, et al. Macrophage subpopulations are essential for infarct repair with and without stem cell therapy. J Am Coll Cardiol. (2013) 62:1890–901. doi: 10.1016/j.jacc.2013.07.057
97. Kim YS, Park HJ, Hong MH, Kang PM, Morgan JP, Jeong MH, et al. TNF-alpha enhances engraftment of mesenchymal stem cells into infarcted myocardium. Front Biosci. (2009) 14:2845–56. doi: 10.2741/3417
98. Tan J, Weil BR, Abarbanell AM, Wang Y, Herrmann JL, Dake ML, et al. Ablation of TNF-alpha receptors influences mesenchymal stem cell-mediated cardiac protection against ischemia. Shock. (2010) 34:236–42. doi: 10.1097/SHK.0b013e3181d75ae3
99. Chen WC, Lee BG, Park DW, Kim K, Chu H, Kim K, et al. Controlled dual delivery of fibroblast growth factor-2 and Interleukin-10 by heparin-based coacervate synergistically enhances ischemic heart repair. Biomaterials. (2015) 72:138–51. doi: 10.1016/j.biomaterials.2015.08.050
100. Alvarez MM, Liu JC, Trujillo-de Santiago G, Cha BH, Vishwakarma A, Ghaemmaghami AM, et al. Delivery strategies to control inflammatory response: Modulating M1-M2 polarization in tissue engineering applications. J Control Release. (2016) 240:349–63. doi: 10.1016/j.jconrel.2016.01.026
101. Projahn D, Simsekyilmaz S, Singh S, Kanzler I, Kramp BK, Langer M, et al. Controlled intramyocardial release of engineered chemokines by biodegradable hydrogels as a treatment approach of myocardial infarction. J Cell Mol Med. (2014) 18:790–800. doi: 10.1111/jcmm.12225
102. Garash R, Bajpai A, Marcinkiewicz BM, Spiller KL. Drug delivery strategies to control macrophages for tissue repair and regeneration. Exp Biol Med. (2016) 241:1054–63. doi: 10.1177/1535370216649444
103. Julier Z, Park AJ, Briquez PS, Martino MM. Promoting tissue regeneration by modulating the immune system. Acta Biomater. (2017) 53:13–28. doi: 10.1016/j.actbio.2017.01.056
104. Badylak SF, Valentin JE, Ravindra AK, McCabe GP, Stewart-Akers AM. Macrophage phenotype as a determinant of biologic scaffold remodeling. Tissue Eng Part A. (2008) 14:1835–42. doi: 10.1089/ten.tea.2007.0264
105. Riolobos L, Hirata RK, Turtle CJ, Wang PR, Gornalusse GG, Zavajlevski M, et al. HLA engineering of human pluripotent stem cells. Mol Ther. (2013) 21:1232–41. doi: 10.1038/mt.2013.59
106. Freytes DO, Santambrogio L, Vunjak-Novakovic G. Optimizing dynamic interactions between a cardiac patch and inflammatory host cells. Cells Tissues Organs. (2012) 195:171–82. doi: 10.1159/000331392
107. Chachques JC, Trainini JC, Lago N, Cortes-Morichetti M, Schussler O, Carpentier A. Myocardial assistance by grafting a new bioartificial upgraded myocardium (MAGNUM trial): clinical feasibility study. Ann Thorac Surg. (2008) 85:901–8. doi: 10.1016/j.athoracsur.2007.10.052
108. Deshmane SL, Kremlev S, Amini S, Sawaya BE. Monocyte chemoattractant protein-1 (MCP-1): an overview. J Interferon Cytokine Res. (2009) 29:313–26. doi: 10.1089/jir.2008.0027
109. Frantz S, Nahrendorf M. Cardiac macrophages and their role in ischaemic heart disease. Cardiovasc Res. (2014) 102:240–8. doi: 10.1093/cvr/cvu025
Keywords: tissue engineering, heart disease, cardiac patch, vascularization, immune system, tissue architecture, heart defects, pluripotent stem cells
Citation: Jarrell DK, Vanderslice EJ, VeDepo MC and Jacot JG (2020) Engineering Myocardium for Heart Regeneration—Advancements, Considerations, and Future Directions. Front. Cardiovasc. Med. 7:586261. doi: 10.3389/fcvm.2020.586261
Received: 22 July 2020; Accepted: 31 August 2020;
Published: 15 October 2020.
Edited by:
Vahid Serpooshan, Emory University, United StatesReviewed by:
Carolina Galvez-Monton, Germans Trias i Pujol Health Science Research Institute (IGTP), SpainRajneesh Jha, Independent Researcher, Seattle, United States
Copyright © 2020 Jarrell, Vanderslice, VeDepo and Jacot. This is an open-access article distributed under the terms of the Creative Commons Attribution License (CC BY). The use, distribution or reproduction in other forums is permitted, provided the original author(s) and the copyright owner(s) are credited and that the original publication in this journal is cited, in accordance with accepted academic practice. No use, distribution or reproduction is permitted which does not comply with these terms.
*Correspondence: Jeffrey G. Jacot, amVmZnJleS5qYWNvdEBjdWFuc2NodXR6LmVkdQ==