Toward Human Models of Cardiorenal Syndrome in vitro
- 1Department of Anatomy and Embryology, Leiden University Medical Center, Leiden, Netherlands
- 2Department of Applied Stem Cell Technologies, University of Twente, Enschede, Netherlands
- 3Department of Internal Medicine-Nephrology, Leiden University Medical Center, Leiden, Netherlands
- 4Einthoven Laboratory of Vascular and Regenerative Medicine, Leiden University Medical Center, Leiden, Netherlands
- 5Department of Biology, University of Padua, Padua, Italy
- 6Veneto Institute of Molecular Medicine, Padua, Italy
Heart and kidney diseases cause high morbidity and mortality. Heart and kidneys have vital functions in the human body and, interestingly, reciprocally influence each other’s behavior: pathological changes in one organ can damage the other. Cardiorenal syndrome (CRS) is a group of disorders in which there is combined dysfunction of both heart and kidney, but its underlying biological mechanisms are not fully understood. This is because complex, multifactorial, and dynamic mechanisms are likely involved. Effective treatments are currently unavailable, but this may be resolved if more was known about how the disease develops and progresses. To date, CRS has actually only been modeled in mice and rats in vivo. Even though these models can capture cardiorenal interaction, they are difficult to manipulate and control. Moreover, interspecies differences may limit extrapolation to patients. The questions we address here are what would it take to model CRS in vitro and how far are we? There are already multiple independent in vitro (human) models of heart and kidney, but none have so far captured their dynamic organ-organ crosstalk. Advanced in vitro human models can provide an insight in disease mechanisms and offer a platform for therapy development. CRS represents an exemplary disease illustrating the need to develop more complex models to study organ-organ interaction in-a-dish. Human induced pluripotent stem cells in combination with microfluidic chips are one powerful tool with potential to recapitulate the characteristics of CRS in vitro. In this review, we provide an overview of the existing in vivo and in vitro models to study CRS, their limitations and new perspectives on how heart-kidney physiological and pathological interaction could be investigated in vitro for future applications.
Introduction
Heart and kidney are highly interdependent both in health and disease. They are essential for regulating cardiovascular (CV) homeostasis, maintaining hemodynamic stability and controlling fluid and nutrient perfusion of organs in the whole body (1). Heart and kidney communicate reciprocally through a variety of pathways and paracrine signaling (2). Neurohormonal activity of the atrial natriuretic peptides (ANP), renin-angiotensin-aldosterone system (RAAS), and sympathetic nervous system (SNS) finely tune their dialogue. Dysfunction or disease of the heart may initiate disease of the kidney and vice versa (3).
“Cardiorenal syndrome” (CRS) is an umbrella term used to describe the dysfunction between the two organs (2, 4) and it includes disorders where acute or chronic dysfunction in one organ may induce acute or chronic dysfunction of the other (5). A five-part classification system for CRS was introduced in 2008 and is summarized in Table 1 (2). CRS subtypes can be distinguished depending on the pathological conditions, time frame and nature of the concomitant cardiac and renal dysfunction.
However, the practicality and clinical applicability of this classification system has been recently questioned, as pathological cascades involving heart, kidney, and neurohormonal systems are already initiated by the time clinical manifestations are detected. Hence, it is almost impossible to define which factor was the initiator and which was its consequence. Another classification system was proposed where CRS is categorized on the response to various treatment modalities (6). Although this classification system may be of more practical value in clinical settings, this review is structured around the five-part classification system because in vitro one can control and investigate the role of the initiator and its consequence.
Cardiorenal syndrome causes high morbidity and mortality, yet the underlying biological mechanisms are not fully understood because complex, multifactorial, and dynamic mechanisms are involved. So far, the disease has only been modeled in vivo. Although animal models can capture the interaction between the organs, they remain difficult to manipulate and control. Moreover, interspecies differences in genetics, anatomy, vascular neural conduction may limit extrapolation to the human condition. Heart and kidney have each been modeled separately in vitro but models so far have had insufficient complexity to capture the dynamic interactions between the organs. For this reason, CRS is clearly a condition that would benefit from increased complexity of in vitro models that reflect organ-organ interaction “in-a-dish.”
In this review, we describe the state of the art regarding in vivo and in vitro models available to recapitulate CRS and, more generally, models of either of the two organs that demonstrate hallmarks of CRS such as oxidative stress, inflammation, and fibrosis. Importantly, we discuss new perspectives on how CRS can be investigated in vitro using these state-of-the-art organ models.
Mechanisms and Hallmarks of Cardiorenal Syndrome
Cardiorenal syndrome develops through hemodynamic and non-hemodynamic mechanisms. Hemodynamic mechanisms are defined by the interactions of blood pressure, renal function, extracellular fluid volumes, and cardiac contractility. Non-hemodynamic mechanisms include cardiorenal connectors such as RAAS, SNS, nitric oxide (NO), and reactive oxygen species (ROS) (7). While hemodynamic mechanisms may explain the adverse relationship between heart and kidney in acute failure, the interpretation of the complex physiological, biochemical, and hormonal mechanisms involved in chronic CRS remains poorly understood. RAAS, SNS activation, oxidative stress and inflammation, fibrosis and tissue remodeling represent the most important mechanisms that, upon dysregulation, lead to cardiorenal damage (Figure 1).
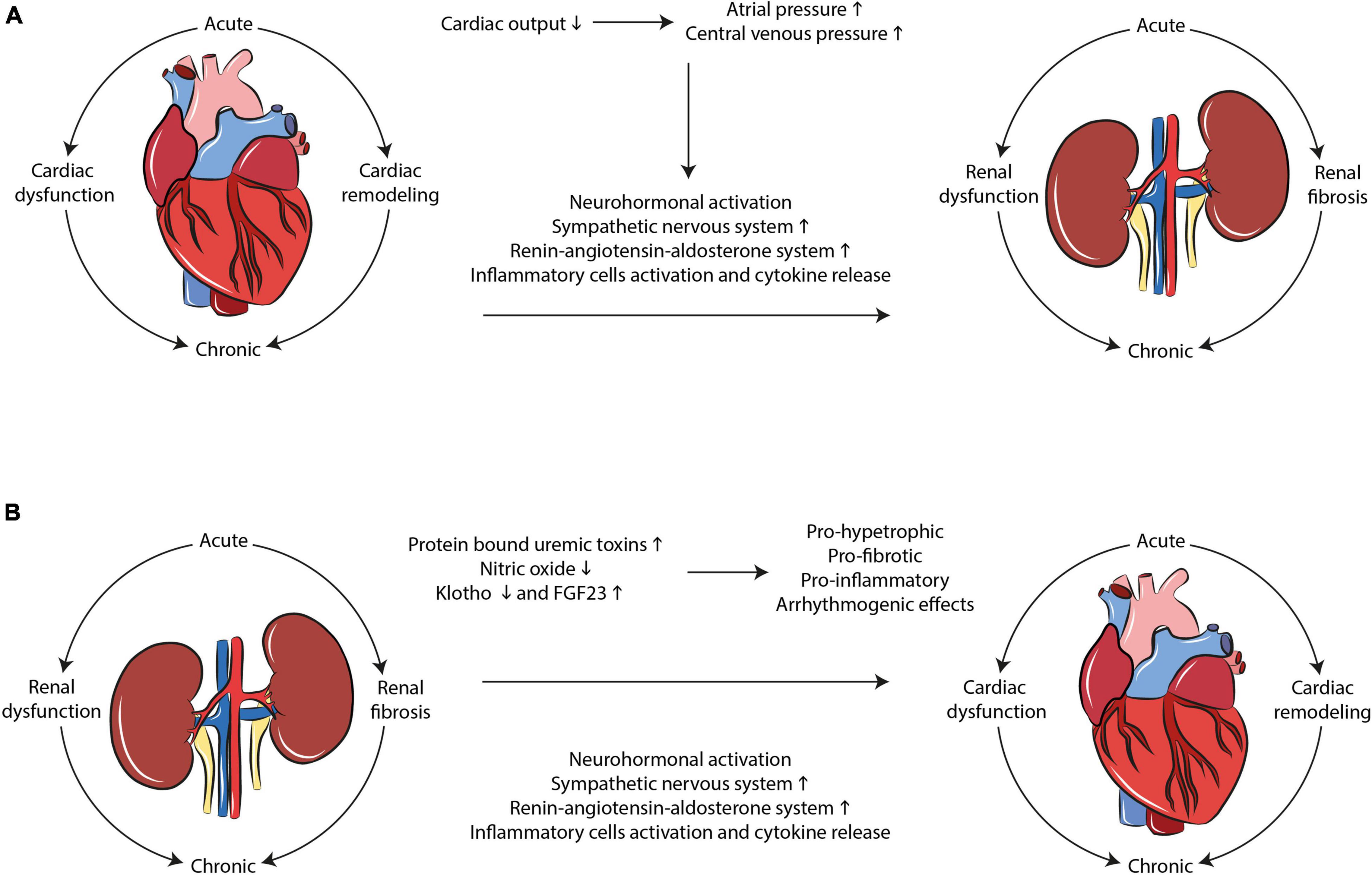
Figure 1. Mechanisms interplaying in CRS development. Multiple complex and dynamic mechanisms play a role in the occurrence of CRS. SNS/RAAS system activation, oxidative stress, and inflammation as well as tissue remodeling and fibrosis are the main hallmarks of the disease in both (A) cardio-renal and (B) reno-cardiac types of CRS.
Sympathetic Nervous System and Renin-Angiotensin-Aldosterone System Activation
Cardiovascular homeostasis is maintained by a set of complex and finely tuned interactions between heart and kidney, where SNS and RAAS are crucial effectors. Imbalance in RAAS and SNS is tightly coupled and, collectively, they play an important role in the development of CRS (8). Kidneys of heart failure (HF) patients release large amounts of renin with consequent increased angiotensin II production, which results in efferent arteriolar constriction and increase in oncotic pressure of peritubular capillaries. Deterioration in the kidney function was associated with elevated central renal pressure, arterial underfilling, and renal venous congestion (9). High venous pressure worsens glomerular filtration rate (GFR), suggesting that persistent RAAS and SNS activation contribute to chronic kidney disease (CKD) progression (10, 11). In acute kidney injury (AKI) patients, interactions between kidney and heart include RAAS and SNS hyperactivity. Myocardial activity is impaired by the hyperactivity of SNS with abnormal norepinephrine secretion from adrenal glands, which reduces Ca2+ metabolism, increases myocardial oxygen demand and myocardial cell β1-adrenergic mediated apoptosis and stimulates α1 receptors (10). RAAS activation was detected in the plasma of acute HF patients as increased plasma renin-activity and aldosterone levels. These increases were associated with greater renal failure (12). Clinical studies have also shown that SNS overactivity affects kidney function through adrenergic receptors (13). Overall, RAAS and SNS are key cardiorenal connectors, as they are induced by the bidirectional failure of heart and kidney (7).
Oxidative Stress and Inflammation
Other cardiorenal mediators are activated in conjunction with RAAS and SNS. In patients affected by CRS, angiotensin II-induced ROS production by nicotinamide adenine dinucleotide phosphate (NADPH) oxidase causes inflammation. Chronic inflammation is a hallmark of both heart and kidney failure. Sera from CRS patients were characterized by high levels of proinflammatory cytokines and proapoptotic factors (14).
The balance between NO and ROS and inflammation is complex. NO and ROS are both involved, in opposing ways, in renal sodium handling, systemic and renal hemodynamics (7). They are also essential for the regulation of cardiac function (15). Heart and renal failure are associated with decreased NO bioavailability and oxidative stress. Oxidative stress results from the body’s ability to metabolize ROS, or when antioxidant defense mechanisms are depleted. The inactivation of the relaxing, endothelium-derived factor NO is an important effect of ROS. Anion O2– reacts with NO and inactivates its beneficial effect by forming peroxynitrite, which oxidizes lipids, DNA and proteins (16).
Oxidative stress is a hallmark of CRS, and patients displayed increased circulating ROS and reactive nitrogen species, together with augmented expression of interleukin-6 (IL-6). Patients had increased levels of NADPH oxidase and myeloperoxidase—an enzyme catalyzing the formation of ROS—with upregulation of proinflammatory mediators (17). In AKI, circulating levels of inflammatory cytokines like TNF-α, IL-1, and IL-6 were increased after renal ischemia and, together with other cytokines and interferon-α, have direct cardio-depressant effects (18). The decreased mitochondrial oxidative metabolism in the heart is compensated by an increase in glucose uptake and glycolysis, with consequent decrease in myocardial adenosine triphosphate (ATP). Cytokines and the transforming growth factor-β (TGF-β) characterize the pro-inflammatory and pro-fibrotic state and are activated by ROS. In the kidneys, TGF-β1-induced cellular ROS is caused by NADPH oxidases and mitochondrial metabolism. The oxidative-dependent activation of transcription factors such as NF-κB and c-Jun leads to the upregulation of renal genes contributing to interstitial fibrosis and inflammation, like phospholipase A2, MCP-1, CSF-1, and COX-2 (19).
Fibrosis and Tissue Remodeling
Fibrosis is a typical tissue-remodeling process following stress and injury. It is the result of several phenomena including the epithelial-to-mesenchymal transition (EMT), fibroblast activation to produce extracellular matrix (ECM), and the recruitment of inflammatory cells with cellular regeneration at the site of damage. EMT in particular is one of the major causes of renal fibrosis: tubular epithelial cells lose epithelial properties and acquire myofibroblast characteristics by producing excessive deposition of ECM (20). Increased levels of aldosterone may cause TGF-β upregulation and increased secretion of fibronectin, causing glomerular fibrosis (21). Yet, studies suggested that renal epithelial cells undergo a partial EMT, contributing in a paracrine manner to fibrogenesis and inflammation (22).
In AKI patients, cardiac injury is characterized by myocyte apoptosis and neutrophil infiltration. The upregulation of beta-galactoside-binding lectin galectin-3 (Gal-3) expression in renal ischemia links AKI to cardiac fibrosis (10). Gal-3 is secreted by activated macrophages and is a marker of hypertrophic hearts developing HF. As in patients with chronic ischemic heart and hypertension, CKD patients develop cardiac fibrosis in the endocardium and epicardium. Uremic toxins like indoxyl sulfate and p-cresol contribute to cardiac fibrosis (10). When compared to a control population, CKD patients had a 300-fold higher concentration of indoxyl sulfate, correlated with cardiac fibrosis, TGF-β synthesis, tissue inhibitor of metalloproteinase-1 (TIMP-1) and α-1 collagen (10, 23). Upregulation of Gal-3 was also shown. In fact, Gal-3 interacts with ECM proteins like laminin, synexin, and integrins and it can bind to cardiac fibroblasts leading to increased collagen production in the myocardium (23). Cardiac remodeling and fibrosis are also associated with elevated levels of B-type natriuretic peptide (BNP) and related N-terminal pro-BNP in CKD patients when compared to cohorts with preserved renal function (24).
Cardiac fibrosis and left ventricular hypertrophy (LVH) are common features of cardiomyopathies contributing to mortality in CKD patients. Excessive cardiac fibrosis and enhanced accumulation of collagen fibrils occurs as the result of increased ECM protein synthesis, in parallel with ECM degradation regulated by matrix metalloproteinases (MMPs) and TIMPs (25). In the myocardial tissue of CKD patients, the accumulation of fibrillar collagens I and III was increased and correlated to dialysis vintage, α-Klotho deficiency and enhanced cardiac angiotensinogen expression (26). α-Klotho (hereinafter called Klotho) is a membrane protein highly expressed in kidney which functions as a co-receptor of fibroblast growth factor (FGF) receptors, activating the FGF23 signaling pathway. A decreased level of soluble Klotho in serum and urine, followed by an increase in FGF23 serves as an early marker for kidney dysfunction and predictor of CV disease. Deficiency indicates CKD progression and CV disease. Preventing Klotho decline, endogenous activation of its production or exogenous supplementation was shown to attenuate renal fibrosis and CKD progression, enhance mineral metabolism, ameliorate cardiomyopathy, and prevent vascular calcification (27).
Cardiac hypertrophy and fibrosis were linked to increased serum levels of FGF23 in CKD patients (28). FGF23 is a member of the FGF ligand family, implicated in the regulation, growth, and differentiation of cardiac myocytes. In kidney, it has paracrine functions blocking vitamin D3 synthesis. In CKD development, phosphate accumulation leads to increased FGF23 secretion, promoting LVH and cardiac remodeling (29). Enhanced myocardial expression of FGF23 and Klotho deficiency were also observed in CKD patients, which strongly correlated with LVH (30, 31). Cardiac levels of FGF23 were associated with up-regulation of FGFR24 and activation of the calcineurin NFAT signaling pathway, a mediator of cardiac remodeling and LVH (30). Moreover, changes in circulating levels and activities of phosphate regulators FGF23 and Klotho led to the development of uremic cardiomyopathy in CKD patients (32).
Modeling Cardiorenal Syndrome Mechanisms and Hallmarks In Vivo
Animal models have a pivotal role in the identification of molecules related to cardiac and renal injury, helping in the elucidation of the mechanisms underlying heart-kidney interactions. Because of their availability and possibility to introduce targeted genetic mutations (e.g., knock-out, knock-in, or transgenic), the large majority of preclinical studies has been performed in either rats or mice. However, the predictive value of murine models is limited, mainly because of species-specific differences; as a result, translation of results to patients has also been limited (32). In the following section, an overview of the animal models used to study the heart and kidney interaction in disease is given and summarized in Table 2.
Animal Models Where Heart Dysfunction Affects the Kidney
To model acute cardiac dysfunction, intravenous injection of potassium chloride in mice has been widely used to induce cardiac arrest followed by cardiopulmonary resuscitation. The acute response resulted in AKI, characterized by reduced GFR and increased serum creatinine levels (33). Cardiac arrest models have provided increasing evidence of the role of an inflammatory response in acute cardiac dysfunction.
Experimental myocardial infarction (MI) is used to induce regional injury and chronic HF though the sudden occlusion of the left anterior descending coronary artery or one of its branches (34). In a mouse model of MI, the development of chronic HF was followed by left ventricular (LV) dilation and thinning of the anterior wall; reduced GFR, elevated serum creatinine associated with mild renal fibrosis and swelling of glomeruli and tubules (35). Systemic depletion of immunocompetent cells (monocytes and macrophages) attenuated renal fibrosis, suggesting their role in its development after MI. The TGF-β/Smad/NF-κB pathway might be mediating renal inflammation and fibrosis (36). A commonly shared pathogenic pathway is among the primary causes of CKD and, as fibrosis increases, the nephron gradually loses its regenerative capacity and apoptosis occurs. Hence, inflammatory processes activated upon MI may result in renal fibrosis, affecting renal function (32).
In murine models of CRS type 1, circulating levels of TNF-α and monocyte chemoattractant protein-1 (MCP-1) decreased upon treatment with exogenous apela, a secretory hormone playing an important role in embryonic CV system development. Apela inhibited apoptosis, DNA damage, inflammation, and fibrosis in renal cells of AKI mice and also inhibited the expression of adhesion molecules in renal tissue of CRS mice (37, 38). In the pericardial sac, Gal-3 leads to inflammation, fibrosis and cardiac dysfunction and its effects are possibly mediated by TGF-β/Smad signaling pathways. In Gal-3-treated rats, it enhanced macrophage and mast cell infiltration, and increased cardiac interstitial and perivascular fibrosis. Gal-3 also increased TGF-β expression and Smad3 phosphorylation (39).
Transverse aortic constriction was used to examine renal response to chronic cardiac pressure overload. A stable pressure gradient across the thoracic aorta of the mouse resulted in ventricle hypertrophy and renal inflammation. Elevated serum creatinine and tubulointerstitial fibrosis characterized the resultant CKD. This model can be considered as clinically relevant for CRS type 2, as it showed the development of CKD in a non-ischemic, hypertrophic chronic HF model (40). Renal response to chronic cardiac volume overload was instead investigated through an aortic valve regurgitation model (41), where treated rats had reduced LV ejection fraction, LV enlargement, and hypertrophy. Moreover, aortic regurgitation also caused albuminuria due to glomerular podocyte injury. The inappropriate activation of the RAAS/SNS system is possibly the mechanism responsible for the onset of albuminuria under cardiac volume overload, suggesting that HF patients may be at increased risk of renal dysfunction due to sympathetic hyperactivity (42).
Animal Models Where Kidney Dysfunction Affects the Heart
Warm ischemia-reperfusion is characterized by a sudden decline in renal function with severe injury in the straight segment of the proximal tubules (43) and it is currently the most used AKI model. Renal ischemia can be induced by clamping the renal pedicle causing damage by hypoxia; it is often associated with compromised intrarenal circulation and oxygenation (44). The resulting renal impairment causes LV fractional shortening and dilation, cardiac hypertrophy, and apoptosis. Renal ischemia-reperfusion-induced cardiac hypertrophy in mice, showed that Toll-like receptors 2 and 4 regulate the release of histones from dying renal cells and contribute to AKI-stimulated cardiac inflammation by selectively regulating the systemic inflammatory profile and NF-κB activation (45).
Toll-like receptor 4 mutant mice and T-cell deficient mice elucidated the role of inflammation in AKI development. CD4+ T-cells mediated post-ischemic renal injury, which was associated with increased inflammation. While both Toll-like receptor 4 mutant mice and T-cell deficient mice were resistant to AKI, expression of proinflammatory genes such as IL-6 was increased in the kidneys of mice when subjected to cardiac arrest (46, 47).
Following ischemic renal injury, inflammatory cells may activate systemic cytokines TNF-α, IL-1, and intercellular adhesion molecule 1 (ICAM-1), that can impair cardiac function by decreasing contractility and increasing myocyte apoptosis. Mitochondrial fragmentation could lead to cardiomyocyte apoptosis and cardiac dysfunction (48, 49).
In a model of CRS type 4, morphological and functional changes in myocardial mitochondria of CKD mice were observed, particularly a decrease in oxidative phosphorylation and fatty acid metabolism. High phosphate contributed to myocardial energy metabolism dysfunction by the downregulation of peroxisome proliferator-activated receptor gamma coactivator 1α (PGC1α). Alterations were attenuated in vivo and in vitro through restoration of PGC1α expression or genetic knockdown of interferon regulatory factor 1 (50).
Metabolomic analysis following ischemic cardiac injury showed inadequate oxidative phosphorylation and oxidative stress. A shift of energy depletion and oxidative stress, reflected systemically in the plasma, was detected in the metabolomic analysis of the kidney (51).
Heart remodeling in CKD is characterized by interstitial fibrosis and capillary loss. The potential pleiotropic effects on heart remodeling of erythropoietin, used to correct renal anemia, was investigated in rats. In combination with enalapril, erythropoietin caused reduced cardiac fibrosis and microvessel disease subtotal nephrectomy (STNx) rats, presumably by decreasing myocardial oxidative stress (52).
Uremic cardiomyopathy and atrial fibrillation in CKD may be caused by inadequate renal clearance and the subsequent accumulation of protein-bound uremic toxins (53). Indoxyl sulfate and p-cresyl sulfate are the most studied protein-bound uremic toxins and they were shown to have pro-hypertrophic and pro-fibrotic effects on cardiomyocytes and cardiac fibroblasts (54–57).
Other major uremic toxins contributing to CV disease are asymmetric dimethylarginine (ADMA), advanced glycation end products (AGE), and trimethyl amine N-oxide (TMAO) that induce both heart and kidney damage (58, 59). Indeed, intrarenal administration of ADMA attenuated renal fibrosis in mouse unilateral ureteral obstruction (UUO) model, whilst knockdown of Ddah1 and Ddah2 increased the amount of ADMA in the kidneys (60).
The development of uremic cardiomyopathy is characterized by declined renal function linked to changes in circulating levels and activities of physiological phosphate regulators FGF23 and Klotho (61). Administration of renal toxin adenine through diet induces severe CKD and vascular calcification (62, 63). Soluble Klotho protects the myocardium from pathological stress stimuli (64). Klotho-deficient mice exhibited cardiac impairment and hypertrophy before 12 weeks of age followed by fibrosis. The extent of cardiac hypertrophy and fibrosis correlated tightly with plasma phosphate concentration and inversely with plasma Klotho concentration (65). Intramyocardial and intravenous injection of FGF23 induced cardiac hypertrophy in wild type mice, while FGF receptor blockage was shown to attenuate it in STNx rats (66).
Impaired endothelial and renal NO production causes systemic NO reduction in CKD. In rats, chronic NO depletion by the administration of an NO synthase inhibitor induced hypertension, proteinuria, glomerulosclerosis, tubulointerstitial fibrosis, systolic dysfunction, and cardiac hypertrophy. Bilateral renal denervation ameliorated these changes and this was associated with decreased RAAS activation (67). Cardiac dysfunction was ameliorated by NO supplementation (68).
Despite extensive investigation, the pathogenesis of fibrotic diseases is complex and unclear. Myofibroblasts were identified by numerous studies as the cells responsible for the progression of fibrosis. The role of endothelial-to-mesenchymal transition (EndoMT) in the pathogenesis of fibrotic disorders was reviewed some years ago (69) and an earlier review considered experimental evidence of EndoMT leading to fibrotic development in animal models of kidney fibrosis (70). EndoMT characterized experimentally induced cardiac fibrosis in mice subjected to aortic banding and rats administered with isoprotenol (71, 72). A model of renal injury followed by heart failure was established by the subcutaneous administration of isoprenaline in rats. Spironolactone showed to inhibit EndoMT via the A2A receptor, ameliorating cardiorenal fibrosis (73). The signals triggering fibrosis in the kidney were also reviewed (22). Myofibroblasts in animal models of renal fibrosis express α-smooth muscle actin (α-SMA) and are mainly located in the renal interstitium/glomeruli. Their loss in peritubular capillaries combined with renal interstitial fibrosis decreases the oxygen diffusion rate and evoke interstitial hypoxia during advanced stages of CKD (74).
Animal Models of the Combined Dysfunction of Heart and Kidney
The investigation of experimental models of dual insults combining elements of chronic cardiac and renal dysfunction is key to mimicking the complex disease processes that occur in individuals with comorbid conditions. To understand the pathophysiology of concomitant organ dysfunction, a rat model of MI, followed by STNx was used. ANP, TGF-β1 and collagen type I were increased. STNx induced a significant decrease in GFR, while MI accelerated STNx-resulting renal cortical tubulointerstitial fibrosis. Altogether, decreased cardiac function as well as increasing cardiac remodeling and renal tubulointerstitial fibrosis was observed in MI/STNx rats, nicely recapitulating the features of the clinical disease manifestations (75). Another model combining chronic HF and CKD was created by performing STNx in rats with doxorubicin-induced dilated cardiomyopathy (76).
Myocardial infarction accelerated renal fibrosis in STNx rats and increased the expression of kidney injury molecule 1 (77). STNx increased cardiac hypertrophy and fibrosis, resulting in impaired diastolic function. Increased expression of hypertrophic and profibrotic marker genes as well as activated MAPK expression in the LV were associated with accelerated organ deterioration (78). MI was performed in renin and angiotensinogen double-transgenic mice to elucidate the RAAS activity in the post-MI prognosis of CKD (79). Renal impairment post-MI cardiac remodeling was mediated by excessive RAAS activation. The effect of RAAS inhibitors has also been investigated (80, 81). In particular, the use of angiotensin II type 1 receptor blockers improved LV ejection fraction and cardiac remodeling associated with macrophage infiltration, inhibition of cardiac oxidative and inflammatory pathways. Although renal function did not improve, the increase in renal fibrosis was attenuated (80).
Emerging Models of Cardiorenal Syndrome Mechanisms and Hallmarks In Vitro
Because of the common principles of animal development and organ physiology, in vivo models have led to detailed mechanistic understanding of many human diseases. However, biological processes that are specific to humans cannot be modeled in other animals. Current models of heart and kidney have been studied separately in vitro but are presently often too simplistic to capture complex organ crosstalk. The following section focuses on in vitro models used to study heart and kidney, which are summarized in Table 3. Some limitations of in vivo disease modeling in animals can be potentially overcome through human in vitro (3D) cell culture approaches using stem cells from different organs (82). The characterization of human pluripotent stem cells (hPSCs) and the more recent organoid technology provided the opportunity to build in vitro models of human specialized tissue cells, including cardiac and kidney, and to study human disease in controllable settings. These technologies already revealed novel disease mechanisms and more advanced techniques are being developed to capture more complex phenotypes and better mimic in vivo physiology.
Cardiorenal Syndrome Mechanisms and Hallmarks Recapitulated by in vitro Heart Models
As discussed, RAAS, SNS, and inflammation are the most important mechanisms whose dysregulation may lead to cardiorenal damage. RAAS stimulation induces NAPDH oxidase activation, which in turn increases ROS formation as observed in neonatal rat cardiomyocytes (83) and human myocardial tissue homogenates (83–86). Moreover, SNS hyperactivity activates RAAS, leading to angiotensin II release which has a direct effect on cellular hypertrophy and apoptosis in adult rat ventricular myocytes (87). FGF23 activated local RAAS by increasing expression of Agt, Ren, Ace, Ngal in neonatal rat cardiomyocytes and cardiac fibroblasts in vitro. In human cardiomyocytes isolated from CKD patients, FGF23 stimulated angiotensinogen expression (28). RAAS components angiotensin II and aldosterone induced FGF23 expression in cardiac myocytes. FGF23 enhanced collagen remodeling, expression of pro-inflammatory, pro-survival, and pro-hypertrophic genes. In cultured human cardiac fibroblasts, FGF23 also stimulated cell proliferation, migration, expression of pro-fibrotic TGF-β receptor/Smad complexes and collagen synthesis (26). The effect of protein membrane Klotho was also studied in neonatal rat ventricular cardiomyocytes and cardiac fibroblasts in vitro. It was observed that Klotho inhibited fibrosis induced by TGF-β1, angiotensin II, or high phosphate fibrosis, while it abolished TGF-β1 or angiotensin II-induced hypertrophy (65).
The role of Wnt signaling in heart and kidney injury was investigated in parallel in vivo and in vitro models of CRS type 2. In vitro, Wnt3a induced multiple components of the RAAS in both primary neonatal rat ventricular cardiomyocytes and cardiac fibroblasts. Serum from transverse aortic constriction mice activated β-catenin and triggered cell injury; moreover, TNF-α inhibited Klotho, induced β-catenin activation, and cell injury. Altogether, these results identified Wnt/β-catenin signaling as a common pathogenic mediator for heart and kidney in CRS type 2 (88).
Uremic toxin accumulation plays a major role in CRS and high serum levels of uremic toxin p-cresol are associated with CV diseases. A study performed on cultured neonatal rat cardiomyocytes showed that p-cresol induced disassembly of gap junctions, increased Ca2+ levels and Ca2+-dependent protein kinase Cα activation (89).
Fibrosis was previously described as a key feature in the pathogenesis of HF. A major impediment in the study of cardiac fibrosis is the lack of reliable and high throughput in vitro models. In TGF-β-treated human primary cardiac fibroblasts, an increase in labeling of detectable glycosaminoglycan chain epitopes with extracellular structures associated with collagen was detected. Newly synthesized collagen fibrils are associated with glycosaminoglycans like dermatan sulfate (90). In arrhythmogenic cardiomyopathy (ACM), the myocardial tissue is replaced with fibrotic or fibro-fatty tissue and inflammatory infiltrates in the heart. ACM patient-derived tissue exhibited higher fibrotic markers and TGF-β levels, thus suggesting that TGF-β stimulation drives pro-fibrotic differentiation of cardiac stromal cells in ACM (91). In a human cardiac fibrosis-on-a-chip model, co-cultured human cardiac fibroblasts, and human induced pluripotent stem cell (hiPSC)-derived cardiomyocytes displayed a 20% increase in α-SMA-positive cell intensity relative to control upon TGF-β exposure; the cardiac fibrosis model displayed increased collagen deposition, higher tissue stiffness, loss of contractile function, and induced BNP secretion (92).
Cardiorenal Syndrome Mechanisms and Hallmarks Recapitulated by in vitro Kidney Models
In the kidneys, ROS generation increases in response to specific stimuli (e.g., angiotensin II and aldosterone) and influences several physiologic processes. The primary source of ROS in the kidney cortex and medulla are the NADPH oxidase (NOX) enzymes. Upon angiotensin II and aldosterone stimulation, ROS production increases. The function of NOX-derived ROS in the kidneys can be classified into regulation of renal blood flow, alteration of cell fate and regulation of gene expression. NOX-derived ROS can alter renal cell fate by enhancing EMT by (i) MAP kinase activation, (ii) mesangial cell apoptosis, and (iii) ERK1/2 activation promoting cellular hypertrophy; this was shown in renal tubular epithelial cells (93, 94). ROS activates pro-inflammatory and fibrotic mechanisms via cytokines and TGF-β signaling, which in turn induces EMT, one of the major causes of renal fibrosis. In cultured rat kidney tubular epithelial cells, TGF-β1 signaling was sufficient to induce EMT (95, 96).
As mentioned above, the effect of apela on renal function and anti-inflammatory effects in CRS type 1 mice have been investigated. Moreover, this study used human renal glomerular endothelial cells to evaluate the adhesion of monocytes in vitro. In vitro, apela inhibited the expression of TNF-α, MCP-1, and intracellular adhesion molecule-1 in renal glomerular endothelial cells induced by angiotensin II. Apela also inhibited the promotion of angiotensin II on the adhesion of THP-1 cells (37).
In a study examining the validity of in vitro models in nephrology, kidney-derived human cells were exposed to TGF-β and/or hypoxic conditions. The expression levels of genes related to these two signaling pathways (TGFβ1, SMAD3, SMAD7, COL1A1, HIF1α, EGLN1, EGLN3, HIF1AN, SIAH2) were quantified. In all in vitro experimental groups (hypoxia, normoxia, hypoxia + TGF-β, normoxia + TGF-β), the expression of the genes was noisy with no consistent pattern. However, in the UUO animal model counterpart, TGF-β pathway-related genes were overexpressed in the ureteral obstruction group compared with the sham controls. Results suggest in vitro findings should be interpreted cautiously (97). Integrins play a major role in CKD tubulointerstitial fibrosis, but the underlying mechanisms are not fully understood. The hypothesis that integrins are pro-fibrotic via regulation of functional interactions between human renal proximal tubular epithelial cells and renal fibroblasts was tested on an in vitro system consisting of a contact co-culture of the two cell types. Integrin-mediated pathways can facilitate the spontaneous accumulation of ECM during fibroblast-epithelial cell interactions (98).
Myofibroblasts are crucial mediators in kidney fibrosis through production of ECM. They prime for apoptosis, expressing higher levels of molecules such as BCL-2 family member BIM, but evade apoptosis by activating pro-survival signals such as TGF-β1 (99). A TGF-β1/Smad oligodeoxynucleotide (ODN), a synthetic short DNA containing complementary sequence for Smad transcription factor and TGF-β1 mRNA, was designed to study the role of TGF-β1/Smad signaling pathways in EMT and EndoMT. The study investigated the anti-fibrotic effect of synthetic TGF-β1/Smad ODN in parallel in a UUO-induced kidney fibrosis model in vivo and TGF-β1-induced in vitro model using renal tubular epithelial cell line NRK-52E. TGF-β1/Smad ODN treatment suppressed inflammatory response and ECM deposition. Through the suppression of TGF-β1/Smad-dependent and independent signaling pathways, the activation of myofibroblasts was inhibited. Moreover, it was demonstrated that synthetic ODN attenuated TGF-β1-induced epithelial dedifferentiation and EndoMT program via blocking TGF-β1/Smad signaling (100).
The Next Step: Developing Cardiorenal Syndrome in vitro Models
While in vitro human cell culture models allow study of human specific biology, many limitations restrict their application, especially for complex, multi-organ diseases such as CRS. For example, human cardiac arrhythmia due to mutations in cardiac ion channels and their treatment can be accurately studied in monotypic cultures of 2D hiPSC-derived cardiomyocytes (101), but they can clearly not recapitulate organ-organ interaction or systemic responses. To this end, cell culture models are continuously being improved by co-culture of multiple cell types, which may or may not be derived from patients. However, human physiology is not only defined by cell types involved but also their environment. We and others have demonstrated that cardiomyocytes cultured in 3D and in combination with their natural “cellular neighbors” behave more like adult cardiomyocytes in vivo compared to cardiomyocytes cultured in a monolayer (102). Not only cell-cell interaction and/or cell-secreted factors are important here but also ECM and their physical environment. For example, while normal cell culture plastic used in vitro would present an extreme supraphysiological load to the cardiomyocytes, 3D co-culture provides a more authentic cellular niche, which mimics the physiological load of cardiomyocytes in vivo more closely. In engineered heart tissues this mimicry can even recapitulate the dynamic pre- and after-load that the heart continuously experiences (103).
The importance of a physiological environment and neighbors is also true for the renal counterpart. hiPSC-derived kidney organoids develop essential nephron subunits consisting of glomeruli and tubular structures. Several protocols show the simultaneous generation of multiple cell types in a single differentiation or by combining multiple progenitor populations first (104–106). While this results in different configuration of cell types in kidney organoids, they are all characterized by a higher degree of organization with segmentation, specialization and maturation compared to their 2D equivalents. The crosstalk between all cell types in the developing kidney organoid is essential. Apart from the cellular composition, kidney organoids should ideally be functionally vascularized to reach enhanced structural maturation through flow in vivo or in vitro (107–109).
Improved engineered tissues have the potential to become more precise platforms for both disease modeling and the development of new therapeutic approaches. Cells from various sources can be collected and cultured in 2D or 3D to study disease in great detail and can be manipulated very precisely (104, 110). In addition, the environment can be engineered to support the cellular components in microphysiological systems or Organ-on-Chip (OoC) microfluidic approaches. It is such combinations of control and ability to manipulate the environment and cells that is required to increase our ability to study and understand the mechanisms underlying the multiple types of CRS.
Control and Manipulation of the Cellular Component
In vitro disease models can be based on multiple cell types of different sources, depending on the study of interest. The development of methods for the generation of 3D organoids has opened the way for modeling human heart and kidney development and disease in vitro. Since organs typically consist of multiple specialized cell types (e.g., cardiomyocytes for the heart and podocytes in Bowman’s capsule for the kidney) in combination with supporting cells (such as endothelial cells and fibroblasts, which often are also organ-specific) and cells of the immune system, the cellular components of complex in vitro models should include all cell types involved in the disease response. In self-organizing organoid cultures, relevant cell types are derived during organoid development or differentiation, often with some spatial arrangement reminiscent of the native organ. Such is the case for kidney organoids and several protocols for the generation of human (pluripotent and adult) stem cell-derived kidney organoids were described (104, 105, 111). The question remains how comparable these structures are to true kidney morphology. The importance of generating progenitor populations (ureteric bud and metanephric mesenchyme) separately and assembling them at a later stage resulted in “higher order” kidney organoids that showed branching morphogenesis, recapitulating the kidney development process (106). Most recently, the addition of a third progenitor population, the organotypic stromal component, demonstrates that the protocol of kidney organoid generation can be further advanced (112). The maturation of the structures in vitro is still challenging. While tubular cells in organoids adequately mature to uptake fluorescent dextrans from the lumen of tubules (113, 114), full maturation to adult-like tubular structures is not achieved. Advances have been made studying glomerular functionality from human hPSCs-derived organoids, with podocytes connecting with endothelial cells in glomerular structures and also showing basic filtration capacity when transplanted in vivo (107, 115, 116).
The level of self-organization reached by kidney organoids is still in development for cardiac 3D models. Most of cardiac tissue models require pre-differentiated cell types of interest to be mixed, in presence or absence of an extracellular or hydrogel component. Self-organization of hiPSCs into cardioids have also been demonstrated, but this technology is still relatively new (117). We recently developed a multicellular model using (pre-differentiated) hiPSC-derived cardiomyocytes, cardiac fibroblasts, and endothelial cells to create cardiac microtissues (118). Similarly, other microtissue models were built using different cell types (119, 120). Microtissues can be considered as small “pieces” of contractile myocardium in which, since they are made with predefined cell types, the cellular ratios and components can be fully controlled and their reciprocal influence and contribution to functional properties examined. For example, ACM is a genetic disease presenting with ventricular arrhythmias and sudden cardiac death. While it seems logical that the cell types responsible for the electrical and contractile activity (i.e., cardiomyocytes) cause this disease, we recently exploited the cardiac microtissue model by replacing healthy fibroblasts with cardiac fibroblasts derived from an ACM patient. This was sufficient for an arrhythmogenic phenotype to appear, therefore confirming recent concepts where cardiac fibroblasts may play an active role in disease onset and progression, and identifying cardiac fibroblasts among the cellular “culprits” underlying the disease phenotype (102, 121). This shows that control of the cellular components is crucial in dissecting and understanding disease mechanisms. For a more accurate and physiologically complex model, the possibility to include additional cell types such as smooth muscle cells, neurons as well as an immune component can be considered.
Controlling the Environment
Advances in technology, such as microfluidics and hydrogels, enable the generation of “instructive” environments that can drive tissue topology and formation of organ-specific structures. For kidney disease modeling, microfluidics may help mimicking the fluid environment that supports tubular cell growth and provides a porous membrane support for cell polarity (122, 123). A multi-layered microfluidic system separated by a membrane was built to stimulate renal filtration in mouse kidney medullary collecting duct cells. The same device was later used to culture human primary renal epithelial cells (124, 125). A method to induce hiPSC-derived podocytes to form human glomerular chips, which mimicked the structure and function of the glomerular capillary wall, has also been described (126). A reusable microfluidic chip was employed in human proximal tubules and glomeruli which permitted renal epithelial cells to grow under different conditions (127). A stable tubule culture system was also designed, which allowed for extended expansion and human kidney tissue analysis (128). Vascularized kidney organoids obtained from hPSCs cultured under flow on millifluidic chips showed improved maturation when compared to the static controls (109). Until now, most enhanced maturation is reached when kidney organoids are functionally vascularized upon transplantation in mice and on the chorioallantonic membrane in chickens (107, 108). The latter study showed that the soft in vivo niche improved growth, differentiation and even vascularization of kidney organoids. By replacing this environment with compliant hydrogels, growth and differentiation were indeed improved.
Similarly, controlled environmental cues have already benefited the generation of different cardiac models, ranging from approaches similar to the above mentioned microtissues but with addition of hydrogels, to fully engineered tissue designs and chips to support bundles with different stiffness. Techniques like photolithography, bioprinting, whole-heart decellularization, micropatterning, biowires, or casting molds scaffolds can be used to build different formats of hPSC-derived engineered heart tissues (129–136). Mechanical support, pillars and elastic strips providing mechanical load and physiologically similar conditioning are used to grow engineered heart tissues; these were shown to induce hiPSC-cardiomyocyte maturation and reveal pathological features (103, 137, 138). In addition, either external or embedded electrodes can be used to electrically stimulate the cardiac tissue and thereby control the beating frequency of the otherwise spontaneously active cells. Control of the environment, as with the cellular component, is therefore essential. For example, in the previously mentioned ACM microtissue model, the arrhythmogenic phenotype was only found at relatively high stimulation frequencies (i.e., above 2 Hz). This correlates with the clinical phenotype since in patients, the trigger for arrhythmic events is usually strenuous exercise or more generally, catecholaminergic stress. The evolution of microfluidics enabled the in vitro bionic study of cardiac tissue. 3D printing technology allowed micro-organ tissue chip production, permitting the integration of myocardial and vascular systems (139, 140). Interestingly, a heart-on-chip device using high-speed impedance detection was used to assess cardiac drug efficacy (141). The physiological and mechanical environment of cardiomyocytes in a heart organ platform was successfully mimicked (142). Moreover, methods to design convenient and efficient chips to generate hiPSC-derived heart tissues in controlled environments are also available (143).
Outlook: Organ-Organ Interaction and Integration of Readouts
Complex models can provide an insight in disease mechanisms and offer a platform for therapy development. Nonetheless, they may not completely recapitulate and predict the effects of a disease in the entire organism, as cells and microorganisms are isolated from their natural environment. Importantly, these models should be as simple as possible in order not to overcomplicate experimental setups, but be complex enough to capture the (disease) mechanism of interest. Microfluidic devices and microfabrication may represent the technological implementations needed to achieve organ vascularization and organ-organ interaction, which are crucial in complex disorders involving multiple organs/tissues as CRS. Multi-OoC could redefine the way human health research is done (144) and CRS is a perfect example of the need for more precise in vitro human models to study organ-organ interaction. Such models connect separate organ chambers together, thus resembling the interactions between different organs (145). Moreover, they could serve as pharmacokinetic and pharmacodynamic models for monitoring the response of multiple organs to pharmaceutical compounds (146, 147).
The ability to measure physiologically relevant parameters is crucial in the development of OoCs in general. When looking at studying CRS, it will be essential to be able to detect changes in both the cardiac and renal functions as necessary, in their respective compartments. Within the cardiac field, chips already exist that integrate electrodes (for measuring the electrical field) and impedance (which is an indication of contraction). In addition, most of the chips are transparent, allowing optical analysis for example to measure calcium transients. For the kidney structures on-chip, measuring nephron functionality will be challenging. Differential clearance of albumin should ideally be achieved as well removal of uremic solutes, when combined with endothelial cells (126). Alternatively, uptake assays, analysis of genetic, proteomic, and metabolic signatures need to be evaluated, as well as structural analysis of nephron segments using (electron) microscopy. For all of these assays, it is important that sampling of the medium—ideally at different time points and even locations within the chip—is possible, as well as that the chip can be opened (or is open-top) and the tissue collected for further processing.
Figure 2 gives a general overview of how coupling heart and kidney organoids into a microfluidic system could support the study of CRS in vitro, capturing the complex interaction of the organs, allowing for the measurement of critical readouts and recapitulating the hallmarks of the disease.
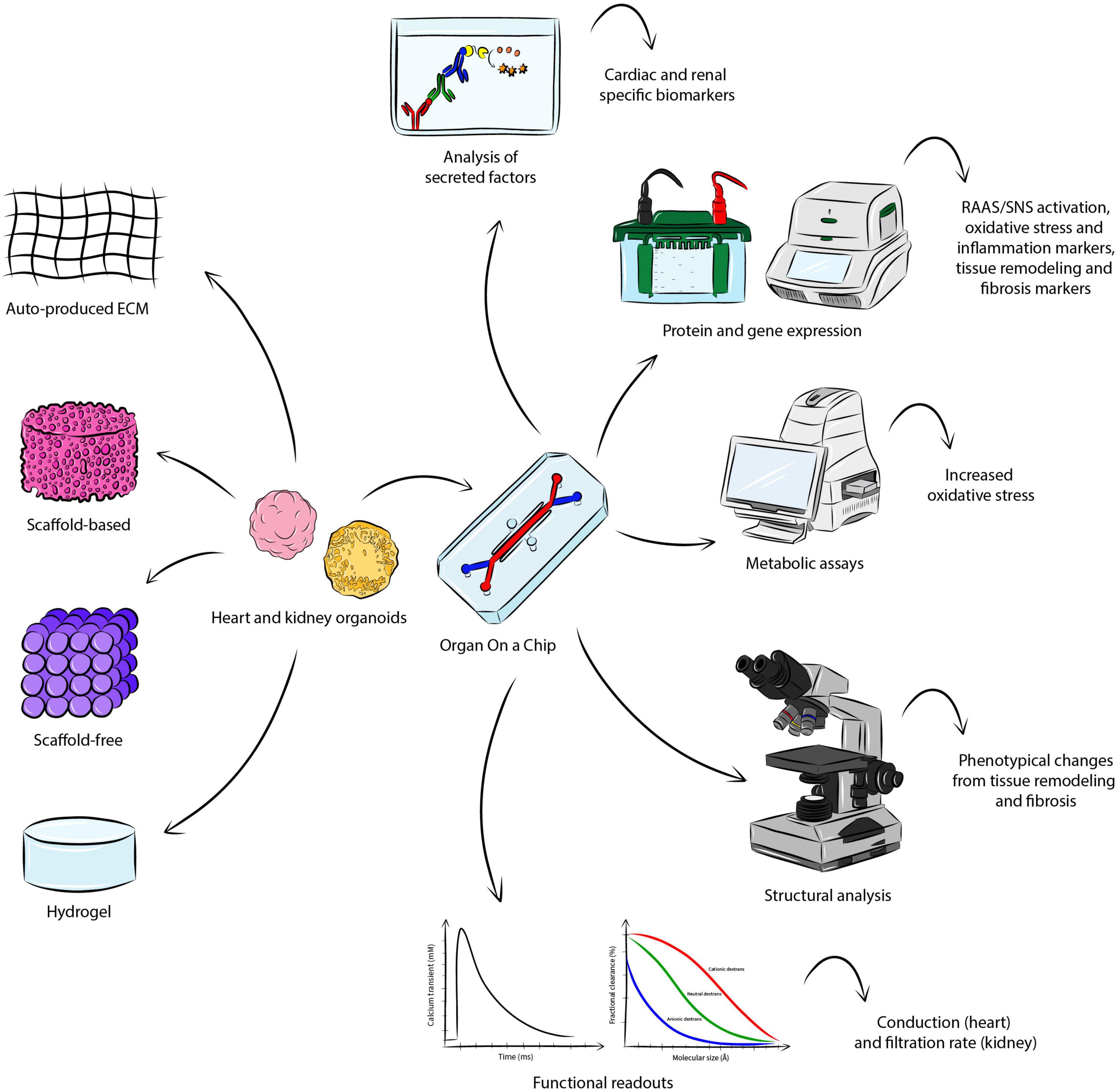
Figure 2. Modeling CRS in vitro. There are several approaches for the fabrication of heart and kidney organoids, where aggregation is either based on endogenously-produced extracellular matrix, or addition of scaffold material, or hydrogel. Coupling the engineered organoid constructs into a OoC microfluidic system may allow the establishment of CRS in vitro, supporting the study of the organ-organ interaction and the measurement of important parameters identifying the hallmarks of the disease. The analysis of secreted factors could detect cardio-renal biomarkers, while protein and gene expression may be investigated though Western blot and quantitative polymerase chain reaction. Metabolic assays would serve to detect a significant change in increased oxidative stress. Structural analysis would allow the visualization of phenotypical changes in the organoids. Functional analyses specific to the organoid of interest (e.g. conduction for the heart and filtration rate for the kidney) may also be conducted.
Conclusion
Cardiorenal syndrome is a broad term used to define the combined dysfunction of heart and kidney. It has recently gained attention through the realization that there is considerable reciprocal influence between the two organs that may help explaining the pathology of the disease and its variants. Although the biological mechanisms underlying the disease are complex and dynamic, new advances in modeling diseases in vitro may help unraveling what is still unknown about primary and secondary events.
Whilst animal studies have clearly been (and still are) valuable tools in understanding (patho)physiological signaling pathways underlying heart and kidney communication, we have argued here that innovative in vitro models will soon contribute to a deeper understanding and modeling of CRS in humans. For this, the convergence of human stem cell biology with engineering technologies will enable control of environmental cues, the development of tailored assays and the design of relevant read-outs that reflect what occurs in patients. Cardiorenal OoC technology has the potential to be the next “best-in-class” platform to precisely model human CRS, allowing drug screening and development of novel treatments.
Author Contributions
All authors outlined, drafted, wrote, and approved the final version of the manuscript.
Funding
BG was supported by the LUMC-Ph.D. grant for the project UNIONS (Unified organoid system for modeling kidney and heart interaction in chronic disease and its treatment). This work was supported by the European Research Council (ERC-CoG Mini-HEART no. 101001746), the Netherlands Organisation for Health Research and Development ZonMW (MKMD project no. 114022504), and the Netherlands Organ-on-Chip Initiative, an NWO Gravitation project (024.003.001) funded by the Ministry of Education, Culture, and Science of the government of the Netherlands.
Conflict of Interest
The authors declare that the research was conducted in the absence of any commercial or financial relationships that could be construed as a potential conflict of interest.
Publisher’s Note
All claims expressed in this article are solely those of the authors and do not necessarily represent those of their affiliated organizations, or those of the publisher, the editors and the reviewers. Any product that may be evaluated in this article, or claim that may be made by its manufacturer, is not guaranteed or endorsed by the publisher.
Abbreviations
ACM, arrhythmogenic cardiomyopathy; LV, left ventricular; ADMA, asymmetric dimethylarginine; LVH, left ventricular hypertrophy; AGE, advanced glycation endproduct; MCP-1, monocyte chemoattractant protein-1; AKI, acute kidney injury; MI, myocardial infarction; ANP, atrial natriuretic peptides; MMP, matrix metalloproteinase; ATP, adenosine triphosphate; NADPH, nicotinamide adenine dinucleotide phosphate; BNP, B-type natriuretic peptide; NO, nitric oxide; CKD, chronic kidney disease; NOX, NAPDH oxidase; CRS, cardiorenal syndrome; ODN, oligodeoxynucleotide; CV, cardiovascular; OoC, organ-on-a-chip; ECM, extracellular matrix; PGC1 α, proliferator-activated receptor gamma coactivator 1 α; EndoMT, endothelial-to-mesenchymal transition; RAAS, renin-angiotensin-aldosterone system; EMT, epithelial-to-mesenchymal transition; ROS, reactive oxygen species; FGF, fibroblast growth factor; α -SMA, α -Smooth muscle actin; Gal-3, Galectin-3; SNS, sympathetic nervous system; GFR, Glomerular filtration rate; STNx, 5/6 subtotal nephrectomy; HF, heart failure; TGF- β, transforming growth factor- β; hPSC, human pluripotent stem cell; TIMP, tissue inhibitor of metalloproteinase; hiPSC, human induced pluripotent stem cell; TMAO, trimethyl amine N-oxide; ICAM, intercellular adhesion molecule; TNF- α, tumor necrosis factor- α; IL, interleukin; UUO, unilateral ureteral obstruction.
References
1. Schefold JC, Filippatos G, Hasenfuss G, Anker SD, von Haehling S. Heart failure and kidney dysfunction: epidemiology, mechanisms and management. Nat Rev Nephrol. (2016) 12:610–23. doi: 10.1038/nrneph.2016.113
2. Ronco C, Haapio M, House AA, Anavekar N, Bellomo R. Cardiorenal syndrome. J Am Coll Cardiol. (2008) 52:1527–39.
3. Boudoulas KD, Triposkiadis F, Parissis J, Butler J, Boudoulas H. The Cardio-Renal Interrelationship. Prog Cardiovasc Dis. (2017) 59:636–48.
5. Ronco C, McCullough P, Anker SD, Anand I, Aspromonte N, Bagshaw SM, et al. Cardio-renal syndromes: report from the consensus conference of the acute dialysis quality initiative. Eur Heart J. (2010) 31:703–11. doi: 10.1093/eurheartj/ehp507
6. Hatamizadeh P, Fonarow GC, Budoff MJ, Darabian S, Kovesdy CP, Kalantar-Zadeh K. Cardiorenal syndrome: pathophysiology and potential targets for clinical management. Nat Rev Nephrol. (2013) 9:99–111. doi: 10.1038/nrneph.2012.279
7. Braam B, Joles JA, Danishwar AH, Gaillard CA. Cardiorenal syndrome–current understanding and future perspectives. Nat Rev Nephrol. (2014) 10:48–55. doi: 10.1038/nrneph.2013.250
8. Petra E, Zoidakis J, Vlahou A. Protein biomarkers for cardiorenal syndrome. Expert Rev Proteomics. (2019) 16:325–36. doi: 10.1080/14789450.2019.1592682
9. Viswanathan G, Gilbert S. The cardiorenal syndrome: making the connection. Int J Nephrol. (2010) 2011:283137. doi: 10.4061/2011/283137
10. Di Lullo L, Bellasi A, Barbera V, Russo D, Russo L, Di Iorio B, et al. Pathophysiology of the cardio-renal syndromes types 1-5: an uptodate. Indian Heart J. (2017) 69:255–65. doi: 10.1016/j.ihj.2017.01.005
11. Merrill AJ, Morrison JL, Branno ES. Concentration of renin in renal venous blood in patients with chronic heart failure. Am J Med. (1946) 1:468. doi: 10.1016/0002-9343(46)90067-8
12. Mentz RJ, Stevens SR, DeVore AD, Lala A, Vader JM, AbouEzzeddine OF, et al. Decongestion strategies and renin-angiotensin-aldosterone system activation in acute heart failure. JACC Heart Fail. (2015) 3:97–107. doi: 10.1016/j.jchf.2014.09.003
13. Noh MR, Jang H-S, Kim J, Padanilam BJ. Renal sympathetic nerve-derived signaling in acute and chronic kidney diseases. Int J Mol Sci. (2020) 21:1647. doi: 10.3390/ijms21051647
14. Virzì GM, Torregrossa R, Cruz DN, Chionh CY, de Cal M, Soni SS, et al. Cardiorenal syndrome type 1 may be immunologically mediated: a pilot evaluation of monocyte apoptosis. Cardiorenal Med. (2012) 2:33–42. doi: 10.1159/000335499
15. Rosenbaugh EG, Savalia KK, Manickam DS, Zimmerman MC. Antioxidant-based therapies for angiotensin II-associated cardiovascular diseases. Am J Physiol Regul Integr Comp Physiol. (2013) 304:R917–28. doi: 10.1152/ajpregu.00395.2012
16. Modlinger PS, Wilcox CS, Aslam S. Nitric oxide, oxidative stress, and progression of chronic renal failure. Semin Nephrol. (2004) 24:354–65. doi: 10.1016/j.semnephrol.2004.04.007
17. Virzì GM, Clementi A, de Cal M, Brocca A, Day S, Pastori S, et al. Oxidative stress: dual pathway induction in cardiorenal syndrome type 1 pathogenesis. Oxid Med Cell Longev. (2015) 2015:391790. doi: 10.1155/2015/391790
18. Blake P, Hasegawa Y, Khosla MC, Fouad-Tarazi F, Sakura N, Paganini EP. Isolation of “myocardial depressant factor(s)” from the ultrafiltrate of heart failure patients with acute renal failure. ASAIO J. (1996) 42:M911. doi: 10.1097/00002480-199609000-00127
19. Rubattu S, Mennuni S, Testa M, Mennuni M, Pierelli G, Pagliaro B, et al. Pathogenesis of chronic cardiorenal syndrome: is there a role for oxidative stress? Int J Mol Sci. (2013) 14:23011–32. doi: 10.3390/ijms141123011
20. Yang J, Liu Y. Dissection of key events in tubular epithelial to myofibroblast transition and its implications in renal interstitial fibrosis. Am J Pathol. (2001) 159:1465–75. doi: 10.1016/S0002-9440(10)62533-3
21. Remuzzi G, Cattaneo D, Perico N. The aggravating mechanisms of aldosterone on kidney fibrosis. J Am Soc Nephrol. (2008) 19:1459–62. doi: 10.1681/ASN.2007101079
22. Nieto MA, Huang RY-J, Jackson RA, Thiery JP. EMT: 2016. Cell. (2016) 166:21–45. doi: 10.1016/j.cell.2016.06.028
23. Lok DJ, Lok SI, Bruggink-André de la Porte PW, Badings E, Lipsic E, van Wijngaarden J, et al. Galectin-3 is an independent marker for ventricular remodeling and mortality in patients with chronic heart failure. Clin Res Cardiol. (2013) 102:103–10. doi: 10.1007/s00392-012-0500-y
24. Maisel AS, Katz N, Hillege HL, Shaw A, Zanco P, Bellomo R, et al. Biomarkers in kidney and heart disease. Nephrol Dial Transplant. (2011) 26:62–74. doi: 10.1093/ndt/gfq647
25. Manabe I, Shindo T, Nagai R. Gene expression in fibroblasts and fibrosis. Circ Res. (2002) 91:1103–13. doi: 10.1161/01.res.0000046452.67724.b8
26. Leifheit-Nestler M, Kirchhoff F, Nespor J, Richter B, Soetje B, Klintschar M, et al. Fibroblast growth factor 23 is induced by an activated renin-angiotensin-aldosterone system in cardiac myocytes and promotes the pro-fibrotic crosstalk between cardiac myocytes and fibroblasts. Nephrol Dial Transplant. (2018) 33:1722–34. doi: 10.1093/ndt/gfy006
27. Lu X, Hu MC. Klotho/FGF23 axis in chronic kidney disease and cardiovascular disease. Kidney Dis. (2017) 3:15–23. doi: 10.1159/000452880
28. Böckmann I, Lischka J, Richter B, Deppe J, Rahn A, Fischer D-C, et al. FGF23-mediated activation of local RAAS promotes cardiac hypertrophy and fibrosis. Int J Mol Sci. (2019) 20:4634. doi: 10.3390/ijms20184634
29. Jovanovich A, Ix JH, Gottdiener J, McFann K, Katz R, Kestenbaum B, et al. Fibroblast growth factor 23, left ventricular mass, and left ventricular hypertrophy in community-dwelling older adults. Atherosclerosis. (2013) 231:114–9. doi: 10.1016/j.atherosclerosis.2013.09.002
30. Leifheit-Nestler M, Große Siemer R, Flasbart K, Richter B, Kirchhoff F, Ziegler WH, et al. Induction of cardiac FGF23/FGFR4 expression is associated with left ventricular hypertrophy in patients with chronic kidney disease. Nephrol Dial Transplant. (2016) 31:1088–99. doi: 10.1093/ndt/gfv421
31. Gutiérrez OM, Januzzi JL, Isakova T, Laliberte K, Smith K, Collerone G, et al. Fibroblast growth factor 23 and left ventricular hypertrophy in chronic kidney disease. Circulation. (2009) 119:2545–52.
32. Liu S. Heart-kidney interactions: mechanistic insights from animal models. Am J Physiol Renal Physiol. (2019) 316:F974–85. doi: 10.1152/ajprenal.00624.2017
33. Ikeda M, Wakasaki R, Schenning KJ, Swide T, Lee JH, Miller MB, et al. Determination of renal function and injury using near-infrared fluorimetry in experimental cardiorenal syndrome. Am J Physiol Renal Physiol. (2017) 312:F629–39. doi: 10.1152/ajprenal.00573.2016
34. Dragneva G, Korpisalo P, Ylä-Herttuala S. Promoting blood vessel growth in ischemic diseases: challenges in translating preclinical potential into clinical success. Dis Model Mech. (2013) 6:312–22. doi: 10.1242/dmm.010413
35. Lu J, Wang X, Wang W, Muniyappa H, Deshmukh A, Hu C, et al. Abrogation of lectin-like oxidized LDL receptor-1 attenuates acute myocardial ischemia-induced renal dysfunction by modulating systemic and local inflammation. Kidney Int. (2012) 82:436–44. doi: 10.1038/ki.2012.186
36. Lekawanvijit S, Krum H. Cardiorenal syndrome: acute kidney injury secondary to cardiovascular disease and role of protein-bound uraemic toxins. J Physiol. (2014) 592:3969–83. doi: 10.1113/jphysiol.2014.273078
37. Jin L, Li Q, Li J, Pan Y, Zou J, Wu X, et al. Apela inhibits systemic and renal inflammatory reactions in mice with type I cardiorenal syndrome. FASEB J. (2021) 35:e21907. doi: 10.1096/fj.202101030R
38. Chen H, Wang L, Wang W, Cheng C, Zhang Y, Zhou Y, et al. ELABELA and an ELABELA Fragment Protect against AKI. J Am Soc Nephrol. (2017) 28:2694–707. doi: 10.1681/ASN.2016111210
39. Liu Y-H, D’Ambrosio M, Liao T-D, Peng H, Rhaleb N-E, Sharma U, et al. N-acetyl-seryl-aspartyl-lysyl-proline prevents cardiac remodeling and dysfunction induced by galectin-3, a mammalian adhesion/growth-regulatory lectin. Am J Physiol Heart Circ Physiol. (2009) 296:H404–12. doi: 10.1152/ajpheart.00747.2008
40. Kamal FA, Travers JG, Schafer AE, Ma Q, Devarajan P, Blaxall BCG. Protein-coupled receptor-G-protein βγ-subunit signaling mediates renal dysfunction and fibrosis in heart failure. J Am Soc Nephrol. (2017) 28:197–208. doi: 10.1681/ASN.2015080852
41. Noma T, Nishiyama A, Mizushige K, Murakami K, Tsuji T, Kohno M, et al. Possible role of uncoupling protein in regulation of myocardial energy metabolism in aortic regurgitation model rats. FASEB J. (2001) 15:1206–8. doi: 10.1096/fj.000569fje
42. Rafiq K, Noma T, Fujisawa Y, Ishihara Y, Arai Y, Nabi AHMN, et al. Renal sympathetic denervation suppresses de novo podocyte injury and albuminuria in rats with aortic regurgitation. Circulation. (2012) 125:1402–13. doi: 10.1161/CIRCULATIONAHA.111.064097
43. Rosenberger C, Rosen S, Heyman SN. Renal parenchymal oxygenation and hypoxia adaptation in acute kidney injury. Clin Exp Pharmacol Physiol. (2006) 33:980–8. doi: 10.1111/j.1440-1681.2006.04472.x
44. Heyman SN, Rosen S, Rosenberger C. Animal models of renal dysfunction: acute kidney injury. Expert Opin Drug Discov. (2009) 4:629–41. doi: 10.1517/17460440902946389
45. Trentin-Sonoda M, da Silva RC, Kmit FV, Abrahão MV, Monnerat Cahli G, Brasil GV, et al. Knockout of Toll-Like Receptors 2 and 4 Prevents Renal Ischemia-Reperfusion-Induced Cardiac Hypertrophy in Mice. PLoS One. (2015) 10:e0139350. doi: 10.1371/journal.pone.0139350
46. Burne-Taney MJ, Kofler J, Yokota N, Weisfeldt M, Traystman RJ, Rabb H. Acute renal failure after whole body ischemia is characterized by inflammation and T cell-mediated injury. Am J Physiol Renal Physiol. (2003) 285:F87–94. doi: 10.1152/ajprenal.00026.2003
47. Burne MJ, Daniels F, El Ghandour A, Mauiyyedi S, Colvin RB, O’Donnell MP, et al. Identification of the CD4(+) T cell as a major pathogenic factor in ischemic acute renal failure. J Clin Invest. (2001) 108:1283–90. doi: 10.1172/JCI12080
48. Kelly KJ. Distant effects of experimental renal ischemia/reperfusion injury. J Am Soc Nephrol. (2003) 14:1549–58. doi: 10.1097/01.asn.0000064946.94590.46
49. Edmunds NJ, Lal H, Woodward B. Effects of tumour necrosis factor-alpha on left ventricular function in the rat isolated perfused heart: possible mechanisms for a decline in cardiac function. Br J Pharmacol. (1999) 126:189–96. doi: 10.1038/sj.bjp.0702294
50. Huang Y, Wang S, Zhou J, Liu Y, Du C, Yang K, et al. IRF1-mediated downregulation of PGC1α contributes to cardiorenal syndrome type 4. Nat Commun. (2020) 11:4664.
51. Fox BM, Gil H-W, Kirkbride-Romeo L, Bagchi RA, Wennersten SA, Haefner KR, et al. Metabolomics assessment reveals oxidative stress and altered energy production in the heart after ischemic acute kidney injury in mice. Kidney Int. (2019) 95:590–610. doi: 10.1016/j.kint.2018.10.020
52. Gut N, Piecha G, Aldebssi F, Schaefer S, Bekeredjian R, Schirmacher P, et al. Erythropoietin combined with ACE inhibitor prevents heart remodeling in 5/6 nephrectomized rats independently of blood pressure and kidney function. Am J Nephrol. (2013) 38:124–35. doi: 10.1159/000353106
53. Gao H, Liu S. Role of uremic toxin indoxyl sulfate in the progression of cardiovascular disease. Life Sci. (2017) 185:23–9. doi: 10.1016/j.lfs.2017.07.027
54. Lekawanvijit S, Adrahtas A, Kelly DJ, Kompa AR, Wang BH, Krum H. Does indoxyl sulfate, a uraemic toxin, have direct effects on cardiac fibroblasts and myocytes? Eur Heart J. (2010) 31:1771–9. doi: 10.1093/eurheartj/ehp574
55. Ito S, Higuchi Y, Yagi Y, Nishijima F, Yamato H, Ishii H, et al. Reduction of indoxyl sulfate by AST-120 attenuates monocyte inflammation related to chronic kidney disease. J Leukoc Biol. (2013) 93:837–45. doi: 10.1189/jlb.0112023
56. Lekawanvijit S, Kompa AR, Manabe M, Wang BH, Langham RG, Nishijima F, et al. Chronic kidney disease-induced cardiac fibrosis is ameliorated by reducing circulating levels of a non-dialysable uremic toxin, indoxyl sulfate. PLoS One. (2012) 7:e41281. doi: 10.1371/journal.pone.0041281
57. Han H, Zhu J, Zhu Z, Ni J, Du R, Dai Y, et al. p-Cresyl sulfate aggravates cardiac dysfunction associated with chronic kidney disease by enhancing apoptosis of cardiomyocytes. J Am Heart Assoc. (2015) 4:e001852. doi: 10.1161/JAHA.115.001852
58. Taguchi K, Elias BC, Brooks CR, Ueda S, Fukami K. Uremic Toxin-Targeting as a Therapeutic Strategy for Preventing Cardiorenal Syndrome. Circ J. (2019) 84:2–8. doi: 10.1253/circj.CJ-19-0872
59. Matsumoto Y, Ueda S, Yamagishi S-I, Matsuguma K, Shibata R, Fukami K, et al. Dimethylarginine dimethylaminohydrolase prevents progression of renal dysfunction by inhibiting loss of peritubular capillaries and tubulointerstitial fibrosis in a rat model of chronic kidney disease. J Am Soc Nephrol. (2007) 18:1525–33. doi: 10.1681/ASN.2006070696
60. Wu M, Yuan M, Wang Y, Tan B, Huang D, Wang C, et al. Renal asymmetric dimethylarginine inhibits fibrosis. FEBS Open Bio. (2020) 10:2003–9. doi: 10.1002/2211-5463.12949
61. Husain-Syed F, McCullough PA, Birk H-W, Renker M, Brocca A, Seeger W, et al. Cardio-pulmonary-renal interactions: a multidisciplinary approach. J Am Coll Cardiol. (2015) 65:2433–48. doi: 10.1016/j.jacc.2015.04.024
62. Ferrari GO, Ferreira JC, Cavallari RT, Neves KR, dos Reis LM, Dominguez WV, et al. Mineral bone disorder in chronic kidney disease: head-to-head comparison of the 5/6 nephrectomy and adenine models. BMC Nephrol. (2014) 15:69. doi: 10.1186/1471-2369-15-69
63. De Schutter TM, Neven E, Persy VP, Behets GJ, Postnov AA, De Clerck NM, et al. Vascular calcification is associated with cortical bone loss in chronic renal failure rats with and without ovariectomy: the calcification paradox. Am J Nephrol. (2011) 34:356–66. doi: 10.1159/000331056
64. Xie J, Yoon J, An S-W, Kuro-o M, Huang C-L. Soluble Klotho Protects against Uremic Cardiomyopathy Independently of Fibroblast Growth Factor 23 and Phosphate. J Am Soc Nephrol. (2015) 26:1150–60. doi: 10.1681/ASN.2014040325
65. Hu MC, Shi M, Cho HJ, Adams-Huet B, Paek J, Hill K, et al. Klotho and phosphate are modulators of pathologic uremic cardiac remodeling. J Am Soc Nephrol. (2015) 26:1290–302. doi: 10.1681/ASN.2014050465
66. Faul C, Amaral AP, Oskouei B, Hu M-C, Sloan A, Isakova T, et al. FGF23 induces left ventricular hypertrophy. J Clin Invest. (2011) 121:4393–408.
67. Eriguchi M, Tsuruya K, Haruyama N, Yamada S, Tanaka S, Suehiro T, et al. Renal denervation has blood pressure-independent protective effects on kidney and heart in a rat model of chronic kidney disease. Kidney Int. (2015) 87:116–27. doi: 10.1038/ki.2014.220
68. Bongartz LG, Braam B, Verhaar MC, Cramer MJM, Goldschmeding R, Gaillard CA, et al. The nitric oxide donor molsidomine rescues cardiac function in rats with chronic kidney disease and cardiac dysfunction. Am J Physiol Heart Circ Physiol. (2010) 299:H2037–45. doi: 10.1152/ajpheart.00400.2010
69. Piera-Velazquez S, Mendoza FA, Jimenez SA. Endothelial to mesenchymal transition (EndoMT) in the pathogenesis of human fibrotic diseases. J Clin Med Res. (2016) 5:45. doi: 10.3390/jcm5040045
70. Li J, Bertram JF. Review: endothelial-myofibroblast transition, a new player in diabetic renal fibrosis. Nephrology. (2010) 15:507–12. doi: 10.1111/j.1440-1797.2010.01319.x
71. Zeisberg EM, Tarnavski O, Zeisberg M, Dorfman AL, McMullen JR, Gustafsson E, et al. Endothelial-to-mesenchymal transition contributes to cardiac fibrosis. Nat Med. (2007) 13:952–61.
72. Zhou X, Chen X, Cai JJ, Chen LZ, Gong YS, Wang LX, et al. Relaxin inhibits cardiac fibrosis and endothelial-mesenchymal transition via the Notch pathway. Drug Des Devel Ther. (2015) 9:4599–611.
73. Chen X, Ge W, Dong T, Hu J, Chen L, Fan X, et al. Spironolactone inhibits endothelial-mesenchymal transition via the adenosine A2A receptor to reduce cardiorenal fibrosis in rats. Life Sci. (2019) 224:177–86.
74. Sun YBY, Qu X, Caruana G, Li J. The origin of renal fibroblasts/myofibroblasts and the signals that trigger fibrosis. Differentiation. (2016) 92:102–7. doi: 10.1016/j.diff.2016.05.008
75. Liu S, Kompa AR, Kumfu S, Nishijima F, Kelly DJ, Krum H, et al. Subtotal nephrectomy accelerates pathological cardiac remodeling post-myocardial infarction: implications for cardiorenal syndrome. Int J Cardiol. (2013) 168:1866–80. doi: 10.1016/j.ijcard.2012.12.065
76. Chua S, Lee F-Y, Chiang H-J, Chen K-H, Lu H-I, Chen Y-T, et al. The cardioprotective effect of melatonin and exendin-4 treatment in a rat model of cardiorenal syndrome. J Pineal Res. (2016) 61:438–56. doi: 10.1111/jpi.12357
77. Lekawanvijit S, Kompa AR, Zhang Y, Wang BH, Kelly DJ, Krum H. Myocardial infarction impairs renal function, induces renal interstitial fibrosis, and increases renal KIM-1 expression: implications for cardiorenal syndrome. Am J Physiol Heart Circ Physiol. (2012) 302:H1884–93. doi: 10.1152/ajpheart.00967.2011
78. Liu S, Wang BH, Kelly DJ, Krum H, Kompa AR. Chronic kidney disease with comorbid cardiac dysfunction exacerbates cardiac and renal damage. J Cell Mol Med. (2018) 22:628–45. doi: 10.1111/jcmm.13349
79. Ogawa M, Suzuki J-I, Takayama K, Senbonmatsu T, Hirata Y, Nagai R, et al. Impaired post-infarction cardiac remodeling in chronic kidney disease is due to excessive renin release. Lab Invest. (2012) 92:1766–76. doi: 10.1038/labinvest.2012.136
80. Watanabe R, Suzuki J-I, Wakayama K, Kumagai H, Ikeda Y, Akazawa H, et al. Angiotensin II receptor blocker irbesartan attenuates cardiac dysfunction induced by myocardial infarction in the presence of renal failure. Hypertens Res. (2016) 39:237–44. doi: 10.1038/hr.2015.141
81. Windt WAKM, Henning RH, Kluppel ACA, Xu Y, de Zeeuw D, van Dokkum RPE. Myocardial infarction does not further impair renal damage in 5/6 nephrectomized rats. Nephrol Dial Transplant. (2008) 23:3103–10. doi: 10.1093/ndt/gfn233
82. Kim J, Koo B-K, Knoblich JA. Human organoids: model systems for human biology and medicine. Nat Rev Mol Cell Biol. (2020) 21:571–84. doi: 10.1038/s41580-020-0259-3
83. Nakagami H, Takemoto M, Liao JK. NADPH oxidase-derived superoxide anion mediates angiotensin II-induced cardiac hypertrophy. J Mol Cell Cardiol. (2003) 35:851–9. doi: 10.1016/s0022-2828(03)00145-7
84. Ushio-Fukai M, Maziar Zafari A, Fukui T, Ishizaka N, Griendling KK. p22phox is a critical component of the superoxide-generating NADH/NADPH oxidase system and regulates angiotensin II-induced hypertrophy in vascular smooth muscle cells. J Biol Chem. (1996) 271:23317–21. doi: 10.1074/jbc.271.38.23317
85. Chabrashvili T, Kitiyakara C, Blau J, Karber A, Aslam S, Welch WJ, et al. Effects of ANG II type 1 and 2 receptors on oxidative stress, renal NADPH oxidase, and SOD expression. Am J Physiol Regul Integr Comp Physiol. (2003) 285:R117–24. doi: 10.1152/ajpregu.00476.2002
86. Heymes C, Bendall JK, Ratajczak P, Cave AC, Samuel J-L, Hasenfuss G, et al. Increased myocardial NADPH oxidase activity in human heart failure. J Am Coll Cardiol. (2003) 41:2164–71. doi: 10.1016/s0735-1097(03)00471-6
87. Kajstura J, Cigola E, Malhotra A, Li P, Cheng W, Meggs LG, et al. Angiotensin II induces apoptosis of adult ventricular myocytes in vitro. J Mol Cell Cardiol. (1997) 29:859–70. doi: 10.1006/jmcc.1996.0333
88. Zhao Y, Wang C, Hong X, Miao J, Liao Y, Hou FF, et al. Wnt/β-catenin signaling mediates both heart and kidney injury in type 2 cardiorenal syndrome. Kidney Int. (2019) 95:815–29. doi: 10.1016/j.kint.2018.11.021
89. Peng Y-S, Ding H-C, Lin Y-T, Syu J-P, Chen Y, Wang S-M. Uremic toxin p-cresol induces disassembly of gap junctions of cardiomyocytes. Toxicology. (2012) 302:11–7. doi: 10.1016/j.tox.2012.07.004
90. Palano G, Jansson M, Backmark A, Martinsson S, Sabirsh A, Hultenby K, et al. A high-content, in vitro cardiac fibrosis assay for high-throughput, phenotypic identification of compounds with anti-fibrotic activity. J Mol Cell Cardiol. (2020) 142:105–17. doi: 10.1016/j.yjmcc.2020.04.002
91. Maione AS, Stadiotti I, Pilato CA, Perrucci GL, Saverio V, Catto V, et al. Excess TGF-β1 Drives Cardiac Mesenchymal Stromal Cells to a Pro-Fibrotic Commitment in Arrhythmogenic Cardiomyopathy. Int J Mol Sci. (2021) 22:2673. doi: 10.3390/ijms22052673
92. Mastikhina O, Moon B-U, Williams K, Hatkar R, Gustafson D, Mourad O, et al. Human cardiac fibrosis-on-a-chip model recapitulates disease hallmarks and can serve as a platform for drug testing. Biomaterials. (2020) 233:119741. doi: 10.1016/j.biomaterials.2019.119741
93. Rhyu DY, Yang Y, Ha H, Lee GT, Song JS, Uh S-T, et al. Role of reactive oxygen species in TGF-beta1-induced mitogen-activated protein kinase activation and epithelial-mesenchymal transition in renal tubular epithelial cells. J Am Soc Nephrol. (2005) 16:667–75. doi: 10.1681/ASN.2004050425
94. Gorin Y, Ricono JM, Wagner B, Kim N-H, Bhandari B, Choudhury GG, et al. Angiotensin II-induced ERK1/ERK2 activation and protein synthesis are redox-dependent in glomerular mesangial cells. Biochem J. (2004) 381:231–9. doi: 10.1042/BJ20031614
95. Böttinger EP, Bitzer M. TGF-beta signaling in renal disease. J Am Soc Nephrol. (2002) 13:2600–10.
96. Fan JM, Ng YY, Hill PA, Nikolic-Paterson DJ, Mu W, Atkins RC, et al. Transforming growth factor-beta regulates tubular epithelial-myofibroblast transdifferentiation in vitro. Kidney Int. (1999) 56:1455–67. doi: 10.1046/j.1523-1755.1999.00656.x
97. Moein S, Moradzadeh K, Javanmard SH, Nasiri SM, Gheisari Y. In vitro versus in vivo models of kidney fibrosis: time-course experimental design is crucial to avoid misinterpretations of gene expression data. J Res Med Sci. (2020) 25:84. doi: 10.4103/jrms.JRMS_906_19
98. Bon H, Hales P, Lumb S, Holdsworth G, Johnson T, Qureshi O, et al. Spontaneous extracellular matrix accumulation in a human in vitro model of renal fibrosis is mediated by αV integrins. Nephron. (2019) 142:328–50. doi: 10.1159/000499506
99. Fan D, Kassiri Z. Modulation of Cardiac Fibrosis in and Beyond Cells. Front Mol Biosci. (2021) 8:750626. doi: 10.3389/fmolb.2021.750626
100. Gwon M-G, An H-J, Kim J-Y, Kim W-H, Gu H, Kim H-J, et al. Anti-fibrotic effects of synthetic TGF-β1 and Smad oligodeoxynucleotide on kidney fibrosis in vivo and in vitro through inhibition of both epithelial dedifferentiation and endothelial-mesenchymal transitions. FASEB J. (2020) 34:333–49. doi: 10.1096/fj.201901307RR
101. Zhang M, D’Aniello C, Verkerk AO, Wrobel E, Frank S, Ward-van Oostwaard D, et al. Recessive cardiac phenotypes in induced pluripotent stem cell models of Jervell and Lange-Nielsen syndrome: disease mechanisms and pharmacological rescue. Proc Natl Acad Sci USA. (2014) 111:E5383–92. doi: 10.1073/pnas.1419553111
102. Giacomelli E, Meraviglia V, Campostrini G, Cochrane A, Cao X, van Helden RWJ, et al. Human-iPSC-derived cardiac stromal cells enhance maturation in 3D cardiac microtissues and reveal non-cardiomyocyte contributions to heart disease. Cell Stem Cell. (2020) 26:862–79.e11. doi: 10.1016/j.stem.2020.05.004
103. Bliley JM, Vermeer MCSC, Duffy RM, Batalov I, Kramer D, Tashman JW, et al. Dynamic loading of human engineered heart tissue enhances contractile function and drives a desmosome-linked disease phenotype. Sci Transl Med. (2021) 13:eabd1817. doi: 10.1126/scitranslmed.abd1817
104. Romero-Guevara R, Ioannides A, Xinaris C. Kidney organoids as disease models: strengths, weaknesses and perspectives. Front Physiol. (2020) 11:563981. doi: 10.3389/fphys.2020.563981
105. Koning M, van den Berg CW, Rabelink TJ. Stem cell-derived kidney organoids: engineering the vasculature. Cell Mol Life Sci. (2020) 77:2257–73. doi: 10.1007/s00018-019-03401-0
106. Taguchi A, Nishinakamura R. Higher-order kidney organogenesis from pluripotent stem cells. Cell Stem Cell. (2017) 21:730–46.e6. doi: 10.1016/j.stem.2017.10.011
107. van den Berg CW, Ritsma L, Avramut MC, Wiersma LE, van den Berg BM, Leuning DG, et al. Renal subcapsular transplantation of PSC-derived kidney organoids induces neo-vasculogenesis and significant glomerular and tubular maturation in vivo. Stem Cell Rep. (2018) 10:751–65. doi: 10.1016/j.stemcr.2018.01.041
108. Garreta E, Prado P, Tarantino C, Oria R, Fanlo L, Martí E, et al. Fine tuning the extracellular environment accelerates the derivation of kidney organoids from human pluripotent stem cells. Nat Mater. (2019) 18:397–405. doi: 10.1038/s41563-019-0287-6
109. Homan KA, Gupta N, Kroll KT, Kolesky DB, Skylar-Scott M, Miyoshi T, et al. Flow-enhanced vascularization and maturation of kidney organoids in vitro. Nat Methods. (2019) 16:255–62. doi: 10.1038/s41592-019-0325-y
110. Hoes MF, Bomer N, van der Meer P. Concise review: the current state of human in vitro cardiac disease modeling: a focus on gene editing and tissue engineering. Stem Cells Transl Med. (2019) 8:66–74. doi: 10.1002/sctm.18-0052
111. Takasato M, Er PX, Chiu HS, Little MH. Generation of kidney organoids from human pluripotent stem cells. Nat Protoc. (2016) 11:1681–92.
112. Tanigawa S, Tanaka E, Miike K, Ohmori T, Inoue D, Cai C-L, et al. Generation of the organotypic kidney structure by integrating pluripotent stem cell-derived renal stroma. Nat Commun. (2022) 13:611. doi: 10.1038/s41467-022-28226-7
113. Freedman BS, Brooks CR, Lam AQ, Fu H, Morizane R, Agrawal V, et al. Modelling kidney disease with CRISPR-mutant kidney organoids derived from human pluripotent epiblast spheroids. Nat Commun. (2015) 6:8715. doi: 10.1038/ncomms9715
114. Takasato M, Er PX, Chiu HS, Maier B, Baillie GJ, Ferguson C, et al. Kidney organoids from human iPS cells contain multiple lineages and model human nephrogenesis. Nature. (2015) 526:564–8.
115. van den Berg CW, Koudijs A, Ritsma L, Rabelink TJ. In vivo assessment of size-selective glomerular sieving in transplanted human induced pluripotent stem cell-derived kidney organoids. J Am Soc Nephrol. (2020) 31:921–9. doi: 10.1681/ASN.2019060573
116. Bantounas I, Ranjzad P, Tengku F, Silajdžić E, Forster D, Asselin M-C, et al. Generation of functioning nephrons by implanting human pluripotent stem cell-derived kidney progenitors. Stem Cell Rep. (2018) 10:766–79. doi: 10.1016/j.stemcr.2018.01.008
117. Hofbauer P, Jahnel SM, Papai N, Giesshammer M, Deyett A, Schmidt C, et al. Cardioids reveal self-organizing principles of human cardiogenesis. Cell. (2021) 184:3299–317.e22. doi: 10.1016/j.cell.2021.04.034
118. Campostrini G, Meraviglia V, Giacomelli E, van Helden RWJ, Yiangou L, Davis RP, et al. Generation, functional analysis and applications of isogenic three-dimensional self-aggregating cardiac microtissues from human pluripotent stem cells. Nat Protoc. (2021) 16:2213–56. doi: 10.1038/s41596-021-00497-2
119. Mills RJ, Titmarsh DM, Koenig X, Parker BL, Ryall JG, Quaife-Ryan GA, et al. Functional screening in human cardiac organoids reveals a metabolic mechanism for cardiomyocyte cell cycle arrest. Proc Natl Acad Sci USA. (2017) 114:E8372–81. doi: 10.1073/pnas.1707316114
120. Polonchuk L, Chabria M, Badi L, Hoflack J-C, Figtree G, Davies MJ, et al. Cardiac spheroids as promising in vitro models to study the human heart microenvironment. Sci Rep. (2017) 7:7005. doi: 10.1038/s41598-017-06385-8
121. Sommariva E, Brambilla S, Carbucicchio C, Gambini E, Meraviglia V, Dello Russo A, et al. Cardiac mesenchymal stromal cells are a source of adipocytes in arrhythmogenic cardiomyopathy. Eur Heart J. (2016) 37:1835–46. doi: 10.1093/eurheartj/ehv579
122. Wu Q, Liu J, Wang X, Feng L, Wu J, Zhu X, et al. Organ-on-a-chip: recent breakthroughs and future prospects. Biomed Eng Online. (2020) 19:9. doi: 10.1186/s12938-020-0752-0
124. Jang K-J, Suh K-Y. A multi-layer microfluidic device for efficient culture and analysis of renal tubular cells. Lab Chip. (2010) 10:36–42. doi: 10.1039/b907515a
125. Jang K-J, Mehr AP, Hamilton GA, McPartlin LA, Chung S, Suh K-Y, et al. Human kidney proximal tubule-on-a-chip for drug transport and nephrotoxicity assessment. Integr Biol. (2013) 5:1119–29. doi: 10.1039/c3ib40049b
126. Musah S, Dimitrakakis N, Camacho DM, Church GM, Ingber DE. Directed differentiation of human induced pluripotent stem cells into mature kidney podocytes and establishment of a Glomerulus Chip. Nat Protoc. (2018) 13:1662–85. doi: 10.1038/s41596-018-0007-8
127. Sakolish CM, Philip B, Mahler GJ. A human proximal tubule-on-a-chip to study renal disease and toxicity. Biomicrofluidics. (2019) 13:014107. doi: 10.1063/1.5083138
128. Schutgens F, Rookmaaker MB, Margaritis T, Rios A, Ammerlaan C, Jansen J, et al. Tubuloids derived from human adult kidney and urine for personalized disease modeling. Nat Biotechnol. (2019) 37:303–13. doi: 10.1038/s41587-019-0048-8
129. Nunes SS, Miklas JW, Liu J, Aschar-Sobbi R, Xiao Y, Zhang B, et al. Biowire: a platform for maturation of human pluripotent stem cell-derived cardiomyocytes. Nat Methods. (2013) 10:781–7.
130. Gao L, Kupfer ME, Jung JP, Yang L, Zhang P, Da Sie Y, et al. Myocardial tissue engineering with cells derived from human-induced pluripotent stem cells and a native-like, high-resolution, 3-dimensionally printed scaffold. Circ Res. (2017) 120:1318–25. doi: 10.1161/CIRCRESAHA.116.310277
131. Ong CS, Fukunishi T, Zhang H, Huang CY, Nashed A, Blazeski A, et al. Biomaterial-free three-dimensional bioprinting of cardiac tissue using human induced pluripotent stem cell derived cardiomyocytes. Sci Rep. (2017) 7:4566. doi: 10.1038/s41598-017-05018-4
132. Goldfracht I, Efraim Y, Shinnawi R, Kovalev E, Huber I, Gepstein A, et al. Engineered heart tissue models from hiPSC-derived cardiomyocytes and cardiac ECM for disease modeling and drug testing applications. Acta Biomater. (2019) 92:145–59. doi: 10.1016/j.actbio.2019.05.016
133. Wanjare M, Kawamura M, Hu C, Alcazar C, Wang H, Woo YJ, et al. Vascularization of Engineered Spatially Patterned Myocardial Tissue Derived From Human Pluripotent Stem Cells in vivo. Front Bioeng Biotechnol. (2019) 7:208. doi: 10.3389/fbioe.2019.00208
134. Zhao Y, Rafatian N, Feric NT, Cox BJ, Aschar-Sobbi R, Wang EY, et al. A platform for generation of chamber-specific cardiac tissues and disease modeling. Cell. (2019) 176:913–27.e18. doi: 10.1016/j.cell.2018.11.042
135. Dostanić M, Windt LM, Stein JM, van Meer BJ, Bellin M, Orlova V, et al. A miniaturized EHT platform for accurate measurements of tissue contractile properties. J Microelectromech Syst. (2020) 29:881–7.
136. Breckwoldt K, Letuffe-Brenière D, Mannhardt I, Schulze T, Ulmer B, Werner T, et al. Differentiation of cardiomyocytes and generation of human engineered heart tissue. Nat Protoc. (2017) 12:1177–97.
137. Mannhardt I, Breckwoldt K, Letuffe-Brenière D, Schaaf S, Schulz H, Neuber C, et al. Human engineered heart tissue: analysis of contractile force. Stem Cell Rep. (2016) 7:29–42. doi: 10.1016/j.stemcr.2016.04.011
138. Tiburcy M, Hudson JE, Balfanz P, Schlick S, Meyer T, Chang Liao M-L, et al. Defined engineered human myocardium with advanced maturation for applications in heart failure modeling and repair. Circulation. (2017) 135:1832–47. doi: 10.1161/CIRCULATIONAHA.116.024145
139. Zhang D, Shadrin IY, Lam J, Xian H-Q, Snodgrass HR, Bursac N. Tissue-engineered cardiac patch for advanced functional maturation of human ESC-derived cardiomyocytes. Biomaterials. (2013) 34:5813–20. doi: 10.1016/j.biomaterials.2013.04.026
140. Zhang YS, Arneri A, Bersini S, Shin S-R, Zhu K, Goli-Malekabadi Z, et al. Bioprinting 3D microfibrous scaffolds for engineering endothelialized myocardium and heart-on-a-chip. Biomaterials. (2016) 110:45–59. doi: 10.1016/j.biomaterials.2016.09.003
141. Zhang X, Wang T, Wang P, Hu N. High-throughput assessment of drug cardiac safety using a high-speed impedance detection technology-based heart-on-a-chip. Micromachines. (2016) 7:122. doi: 10.3390/mi7070122
142. Marsano A, Conficconi C, Lemme M, Occhetta P, Gaudiello E, Votta E, et al. Beating heart on a chip: a novel microfluidic platform to generate functional 3D cardiac microtissues. Lab Chip. (2016) 16:599–610. doi: 10.1039/c5lc01356a
143. Schneider O, Zeifang L, Fuchs S, Sailer C, Loskill P. User-Friendly and Parallelized Generation of Human Induced Pluripotent Stem Cell-Derived Microtissues in a Centrifugal Heart-on-a-Chip. Tissue Eng Part A. (2019) 25:786–98. doi: 10.1089/ten.TEA.2019.0002
144. Picollet-D’hahan N, Zuchowska A, Lemeunier I, Le Gac S. Multiorgan-on-a-chip: a systemic approach to model and decipher inter-organ communication. Trends Biotechnol. (2021) 39:788–810. doi: 10.1016/j.tibtech.2020.11.014
145. Zhao Y, Kankala RK, Wang S-B, Chen A-Z. Multi-organs-on-chips: towards long-term biomedical investigations. Molecules. (2019) 24:675. doi: 10.3390/molecules24040675
146. Herland A, Maoz BM, Das D, Somayaji MR, Prantil-Baun R, Novak R, et al. Quantitative prediction of human pharmacokinetic responses to drugs via fluidically coupled vascularized organ chips. Nat Biomed Eng. (2020) 4:421–36. doi: 10.1038/s41551-019-0498-9
Keywords: cardiorenal syndrome, disease modeling, hiPSCs, organ-on-chip, tissue-specific organoids
Citation: Gabbin B, Meraviglia V, Mummery CL, Rabelink TJ, van Meer BJ, van den Berg CW and Bellin M (2022) Toward Human Models of Cardiorenal Syndrome in vitro. Front. Cardiovasc. Med. 9:889553. doi: 10.3389/fcvm.2022.889553
Received: 04 March 2022; Accepted: 09 May 2022;
Published: 26 May 2022.
Edited by:
Ernesto Martinez-Martinez, Universidad Complutense de Madrid, SpainReviewed by:
Parta Hatamizadeh, University of Florida, United StatesHao Zhou, First Affiliated Hospital of Wenzhou Medical University, China
Copyright © 2022 Gabbin, Meraviglia, Mummery, Rabelink, van Meer, van den Berg and Bellin. This is an open-access article distributed under the terms of the Creative Commons Attribution License (CC BY). The use, distribution or reproduction in other forums is permitted, provided the original author(s) and the copyright owner(s) are credited and that the original publication in this journal is cited, in accordance with accepted academic practice. No use, distribution or reproduction is permitted which does not comply with these terms.
*Correspondence: Milena Bellin, m.bellin@lumc.nl, milena.bellin@unipd.it