- 1Department of Clinical Medicine, Dalian Medical University, Dalian, China
- 2State Key Laboratory of Organ Failure Research, Department of Cardiology, Nanfang Hospital, Southern Medical University, Guangzhou, China
- 3Department of Clinical Pharmacology, College of Pharmacy, Dalian Medical University, Dalian, China
Coronary chronic total occlusion (CTO) contributes to the progression of heart failure in patients with ischemic cardiomyopathy. Randomized controlled trials demonstrated that percutaneous coronary intervention (PCI) for CTO significantly improves angina symptoms and quality of life but fails to reduce clinical events compared with optimal medical therapy. Even so, intervening physicians strongly support CTO-PCI. Cardiac regeneration therapy after CTO-PCI should be a promising approach to improving the prognosis of ischemic cardiomyopathy. However, the relationship between CTO revascularization and cardiac regeneration has rarely been studied, and experimental studies on cardiac regeneration usually employ rodent models with permanent ligation of the coronary artery rather than reopening of the occlusive artery. Limited early-stage clinical trials demonstrated that cell therapy for cardiac regeneration in ischemic cardiomyopathy reduces scar size, reverses cardiac remodeling, and promotes angiogenesis. This review focuses on the status quo of CTO-PCI in ischemic cardiomyopathy and the clinical prospect of cardiac regeneration in this setting.
Introduction
Due to the limited proliferation potential of cardiomyocytes, injured mammalian hearts do not regenerate adequately but instead develop fibrosis and scarring, leading to heart failure, arrhythmia, and even death. Ischemic cardiomyopathy (ICM) with coronary artery chronic total occlusion (CTO) accelerates the progression of heart failure, which is the leading cause of death worldwide. Despite the development of optimal medical therapy (OMT) and interventional and surgical strategies, the morbidity and mortality of patients with ICM remain relatively high. CTO is associated with a negative impact on long-term prognosis (1), and CTO lesions in a non-infarct-related artery are a high-risk factor for mortality after acute myocardial infarction (AMI) (2). Under such circumstances, it is reasonable to consider that revascularization of the occluded coronary artery would improve the prognosis of patients with CTO. However, several randomized controlled trials (RCT) demonstrated that percutaneous coronary intervention (PCI) for CTO significantly improves angina symptoms and quality of life but fails to reduce clinical events such as mortality, myocardial infarction (MI), stroke, and repeat revascularization rates compared with OMT (3–7). Nevertheless, support for CTO-PCI remains high in clinical practice worldwide. In addition to improving quality of life, we speculate that revascularization should be a premise for further regenerative therapy to improve the prognosis of ICM.
In ICM with CTO, the presence of myocardial hibernation is a primary reason for considering revascularization therapy (8). It is believed that restoring blood flow in the infarcted or ischemic area is important for the repair of myocardial injury. Otherwise, cardiomyocytes are lost quickly or gradually, causing myocardial fibrosis and arrhythmia and leading to heart failure. Accordingly, there has been great support for CTO-PCI or coronary artery bypass grafts (CABGs) over the past two decades. Because fibrotic scar formation often occurs in patients with CTO, restoration of blood flow alone is not able to replace fibrotic scars with cardiomyocytes. In addition to heart transplantation, we believe that effective regenerative therapy combined with the opening of the CTO and OMT would be an optimal approach for curing ICM with CTO.
Cardiac regeneration is a research hotspot that has developed rapidly, with an annual increase of more than 1000 publications in recent years (9). Although substantial progress has been made in experimental studies and various strategies have been developed to induce cardiac regeneration, these interventions still lack adequate success for use in the clinic. In addition to the low efficiency of current regenerative therapy, one contributing factor may be that many efforts have focused primarily on generating cardiomyocytes, with less attention to simultaneous angiogenesis. To maintain the survival and growth of regenerated cardiomyocytes, blood supply to the cells is necessary for oxygen transfer, nutrient absorption and removal of metabolic waste.
Angiogenesis in the heart is formed from preexisting coronary vessels (10). Effective vascular regeneration is critical for enabling the survival of transplanted or regenerated cells. The absence of clinically applicable means of (re)generating vessels is one of the main obstacles in cell replacement therapy (11). The vasculature could also provide important cues for stem cell-derived tissues, which remain immature in vitro and require an in vivo environment for maturation. Therefore, the role of an appropriate vasculature goes beyond integration with the host system and blood perfusion, and implementing effective vascularization strategies is critical for the success of regenerative medicine. The effects of most cell therapies are mediated by paracrine signaling rather than replacement of lost cardiomyocytes, mainly through the induction of angiogenesis and immunomodulation (9). Thus far, cell-based therapies have delivered unsatisfactory results, prompting the search for cell-free alternatives that can induce the heart to repair itself through cardiomyocyte proliferation and angiogenesis. It seems reasonable to open the occluded arteries as preexisting vessels for angiogenesis and nutrient delivery to the regenerated cardiomyocytes.
Poor prognosis of coronary chronic total occlusion
Coronary CTO, which is defined as a complete luminal obstruction of a native coronary artery for ≥ 3 months, has been diagnosed in nearly 20% of patients with coronary artery disease (12, 13). In contrast to patients with non-occlusive coronary artery disease, patients with CTO usually have severe comorbidities, such as diabetes mellitus, hypertension, peripheral vascular disease and prior MI (14). CTO can be considered the final stage of obstructive coronary artery disease and is associated with a negative impact on long-term prognosis (1). An undiagnosed or untreated acute thrombotic event is regularly the origin of CTO development, which is supported by electrocardiographic evidence of pathological Q-waves corresponding to the myocardial territory subtended by an occluded artery in one-quarter of patients (12). However, the majority of patients with a CTO have not experienced previous MI (12). In those patients, the occlusion seems to be the result of long-term gradual luminal narrowing allowing for recruitment of collaterals to the occluded vessel. The recruitment of collaterals has a protective role by supplying myocardial blood flow to the CTO territory and thereby preventing acute myocardial ischemia (15).
The myocardial territory supplied by a CTO is a proarrhythmogenic milieu due to the heterogeneity in repolarization and is characterized by scar tissue, hibernating myocardium, and residual ischemia even in the presence of collateral circulation (16). The presence of concurrent CTO is a strong predictor for both short-term and long-term mortality. Patients with a CTO and an implantable cardioverter defibrillator for prevention of sudden cardiac death have a higher incidence of shocks than patients with ICM without a CTO (17). CTOs in a non-infarct-related artery (non-IRA) are present in 10% of patients with ST elevation MI (STEMI) and 23.5% of patients with MI and multivessel disease complicated by cardiac shock (12, 18). The presence of a concomitant CTO in those patients with STEMI is responsible for a higher 30-day event rate and poor long-term prognosis (19). The prognosis especially deteriorates when the occluded vessel receives collateral flow from the IRA (20). In the HORIZONS-AMI trial reported by Claessen et al., patients with a non-IRA CTO were significantly less likely to achieve satisfactory postprocedural reperfusion flow and less frequently achieved complete ST-segment resolution than patients without a CTO (21). Analyses from three clinical trials (HORIZONS-AMI, CULPRIT-SHOCK and TAPAS) demonstrated that multivessel disease with CTO in a non-IRA increases the risk of death for 1 month to 3 years by approximately twofold (HR: 1.63–2.88) (18, 21, 22). CTO has also been reported to worsen the prognosis of patients with type 2 diabetes. Compared to patients without CTO, patients with diabetes and CTO had higher myocardial jeopardy scores and higher 5-year mortality rates than non-CTO patients (23).
The poor prognosis of a concurrent CTO in ICM patients suggests that revascularization therapy of occluded arteries should be highly effective, which is one of the reasons that intervening physicians actively perform CTO-PCI.
Limited clinical benefits of percutaneous coronary intervention for chronic total occlusion
Ischemic cardiomyopathy is one of the most common causes of congestive heart failure. Accumulating evidence indicates that hibernating myocardium is present in the blood supply region of an occluded artery. Evaluation of viable myocardium can be fundamental for planning myocardial revascularization. Even if excellent collateral circulation develops, symptomatic patients with a CTO usually have a persistent ischemic zone, evidenced by lower fractional flow reserve of the myocardium supplied by a CTO (24). Cardiac magnetic resonance (CMR) can be used to identify inducible myocardial ischemia and viability in the perfusion territory of the artery with CTO; thus, it is believed that CMR is helpful for selecting patients likely to benefit from revascularization (25). In a prospective study of 50 consecutive CTO patients undergoing CMR, Bucciarelli-Ducci et al. reported that CTO recanalization reduces ischemic burden, favors reverse remodeling, and improves quality of life for patients, showing CMR evidence of significant myocardial inducible perfusion defects and viability (26). Similar findings were also found in STEMI patients with CTO (EXPLORE trial) (27).
Percutaneous coronary intervention for CTO has been extensively performed worldwide in the last 2 decades. In the Web of Science database, approximately 4000 papers on CTO-PCI could be found up to April 2022. Of them, only 114 articles were related to clinical trials, and the publication numbers peaked in 2018 (Figure 1). A recent meta-analysis reported by Khan et al. compared the clinical effects of CTO-PCI versus OMT from 2006 to 2019 (3). The authors included a total of 16 studies with 11,314 patients. Observational studies showed that CTO-PCI was associated with lower mortality (OR: 0.45) and cardiac deaths (OR: 0.58) than medical therapy alone, but in RCTs, no significant differences in major adverse cardiac events (MACEs) (OR: 0.71, P = 0.54), myocardial reinfarction (OR: 0.71, P = 0.54), stroke (OR: 0.61, P = 0.14), or repeat PCI (OR: 1.28, P = 0.16) were observed (3). The possible explanations for the inconsistency of the above results are as follows: First, all the RCTs (Euro CTO, REVASC trials, EXPLORE, DECISION-CTO) included in this analysis were underpowered due to slow enrollment rates and a high crossover rate introducing significant selection bias. Second, majority of these trials involved enrollment of a minimally symptomatic population with relatively lower angina scores, and a better comorbidity index. Although not statistically significant, an average 30–40% risk reduction for MACEs, reinfarction and stroke by CTO-PCI was very impressive. It is reasonable to expect that CTO-PCI would be superior to OMT alone if a sufficiently large sample size and adjunctive regenerative therapy were guaranteed. To date, only 4 RCT comparing clinical prognosis between CTO-PCI and optimal or routine medical therapy alone have been published (Table 1). The DECISION-CTO, EXPLORE, EUROCTO, and IMPACTOR-CTO trials included 417, 150, 259, and 39 PCI patients with procedure failure rates of 9.6, 27, 13.4, and 17%, respectively (4–7). The comparisons among PCI and OMT studies for CTO in RCTs and observational studies were list in Table 2.
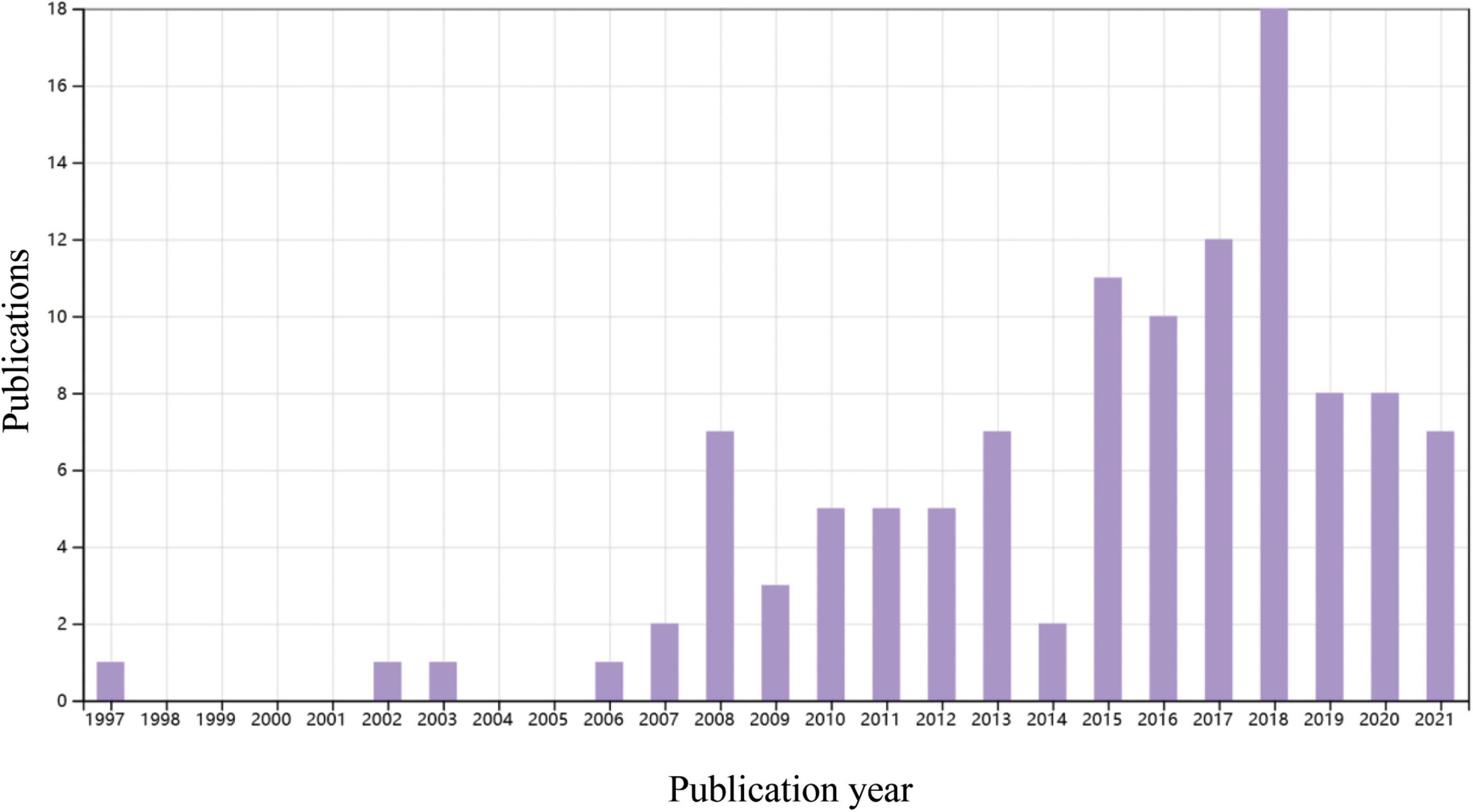
Figure 1. A time course of publications concerning clinical trials of percutaneous coronary intervention (PCI) or coronary artery bypass graft in patients with coronary chronic total occlusion (CTO). Data source: Web of Science, searched with the topics (CTO or “chronic total occlusion”) AND [(PCI or “percutaneous coronary intervention”) OR CABG or “coronary artery bypass graft”] and then refined by document types “Clinical Trial” and “Articles.”
In patients with diabetes and concurrent CTO, Khan et al. analyzed the results of early revascularization in 1196 cases and OMT in 1252 cases and demonstrated that OMT was associated with higher all-cause mortality [HR: 1.70, P = 0.11] and cardiac mortality (HR: 1.68, P = 0.07) and a higher risk of repeat revascularization (HR: 1.62, P < 0.00001). Subgroup analysis of OMT vs. PCI demonstrated higher all-cause (HR: 1.98, P = 0.0003) and cardiac mortality (HR: 1.87, P = 0.06) in the OMT group (28). Similarly, Damluji et al. compared the clinical outcome between 482 diabetic patients with prompt revascularization and 490 patients with intensive medical therapy alone. They found that CTO of coronary arteries is associated with increased mortality in patients treated medically but not in patients treated with revascularization (23).
It is generally believed that CTO-PCI can improve the quality of life of patients even if there is no significant reduction in MACEs. The effects of adjunctive regenerative therapy, as well as those of OMT, in patients who undergo CTO-PCI merit further study.
Effect of opening CTO on cardiac regeneration
Cardiac regenerative medicine focusing on preclinical studies and early-stage clinical trials is rapidly evolving with novel approaches involving cell-based, cell-free and tissue engineering therapies (29, 30). Several thousand review papers have been published on cardiac regeneration, but few have paid attention to cardiac regeneration in the setting of CTO-PCI.
The main conclusions of the clinical trials of cell-based therapy over the last 2 decades are that the outcomes of cell therapy were neutral or marginally positive regarding clinically relevant end points (31). By reviewing the clinical studies on ICM, Nair et al. concluded that a combined approach of simultaneous revascularization and stem cell therapy appears to produce the maximum benefit in ICM (32). In addition to cell therapy, the activation of cardiomyocyte proliferation in situ is a promising approach for replacing lost cardiomyocytes. Although potential interest is switching from an exogenous to an endogenous strategy in basic research, there is no clinical trial on endogenous regenerative therapy for the time being.
In the research field of cardiac regenerative therapy, it is common for clinical trials to recruit patients with patent coronary arteries, while experimental studies utilize animal models without coronary revascularization. There are three routes of cell or regeneration-promoting agent delivery: intracoronary, intravenous and intramyocardial (transendocardial) injection. In the setting of CTO without collateral supply, neither intracoronary nor intravenous routes can work for regenerative therapy before the occluded artery is vascularized. Intramyocardial injection is the preferred delivery route for cell therapy in most clinical trials on ICM (33), while intravenously delivered mesenchymal stem cells could improve left ventricular dysfunction through systemic anti-inflammatory effects in ICM (30, 34). Choudhry et al. used a combination of growth factors and bone marrow cells to treat heart failure in ICM patients who had no further treatment options after receiving OMT and undergoing revascularization. They noted that intramyocardial delivery was more effective in improving left ventricular ejection fraction (LVEF) at 1 year than the intracoronary approach (35). However, the outcome of the intracoronary approach for cell therapy in the majority of clinical trials on ICM was positive (32).
In some early clinical studies without CTO-PCI, intracoronary infusion of stem cells from the patent coronary artery to the distribution areas of the occluded artery by collateral flow was performed. Even in that case, a lower incidence of angina symptoms or an increase in LVEF by stem cell therapy was observed (36, 37). As early as 2005, Erbs et al. performed the first RCT to examine whether intracoronary infusion of circulating progenitor cells exerts beneficial effects in patients after recanalization of CTO (38). The authors noted that intracoronary cell therapy after recanalization of CTO results in an improvement in macro- and microvascular function, evidenced by decreases in the infarct size and number of hibernating segments in the target region, an increase in LVEF by 14%, and a reduction in the amount of myocardium with a perfusion-metabolism mismatch in the treatment group (38, 39). Although the sample size was small (26 patients), their results were encouraging.
Over the last 20 years, 35 articles on clinical trials of regenerative therapy in ICM were published, with peak publication in 2017 and peak citations in 2018 (Figure 2). The average number of citations for each paper was 102, suggesting that regenerative therapy in ICM is a hot topic. Nair et al. summarized 24 completed clinical trials of stem cell therapy in ischemic heart disease, and positive outcomes (improvement in LVEF and reduction in infarct size) were obtained in 13 trials (32), suggesting that regenerative therapy would be a promising approach for resolving heart failure in ICM.
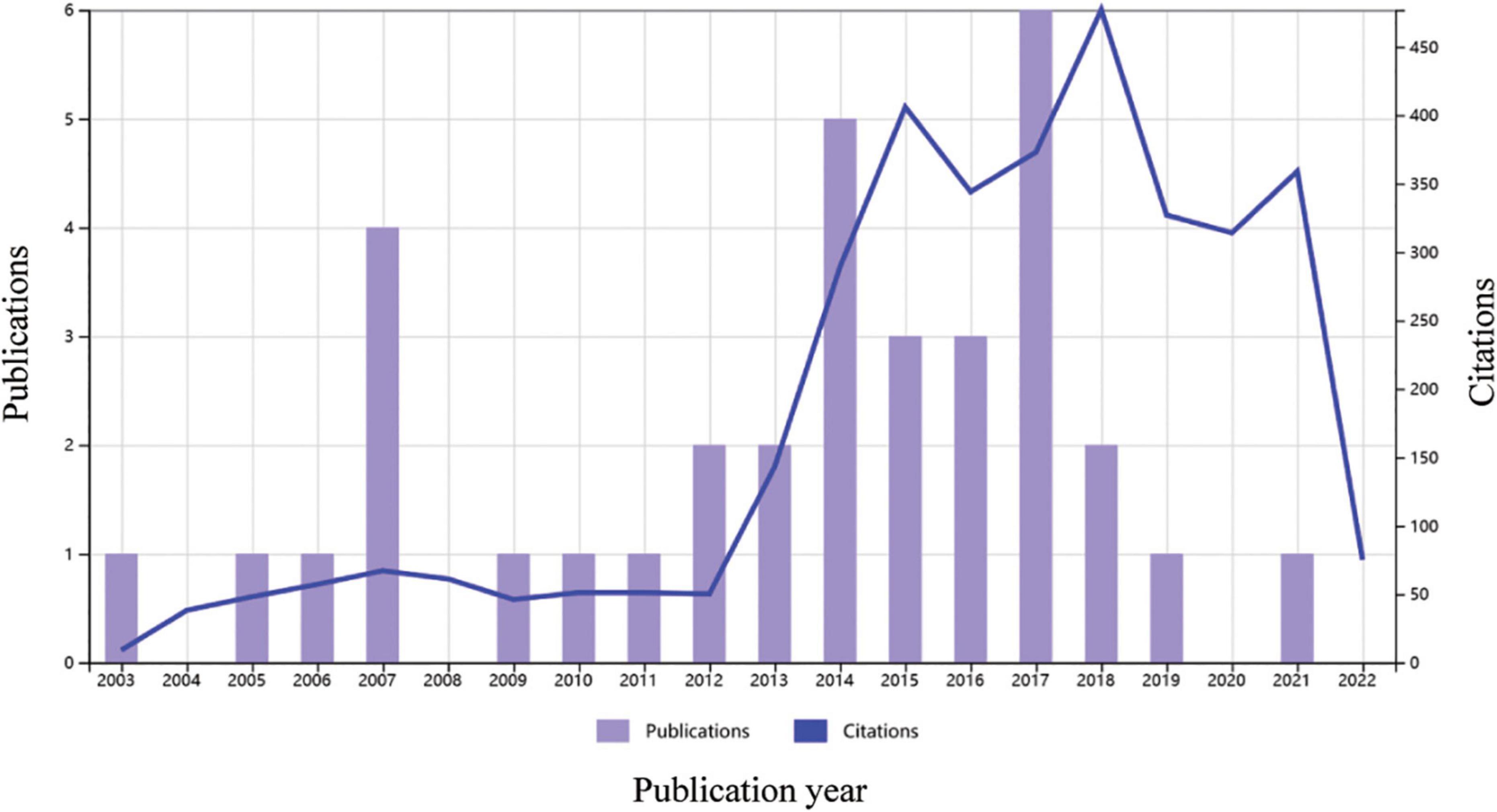
Figure 2. Times cited and number of publications over time related to cardiac regenerative therapy in patients with ischemic heart disease. Data source: Web of Science, searched with the topics “Ischemic cardiomyopathy” AND “Regenerat” and then refined by document types “Articles” and “Clinical Trial,” MeSH headings of “Humans” and “Treatment Outcome,” and excluding document types “Retracted Publications” and “Publication with Expression of Concern.”
Simultaneous regeneration of both myocytes and vessels
A water supply is a necessary prerequisite for greening a desert. MI induced by permanent ligation of the left coronary artery in mice usually leads to large ventricular aneurysm (40), which is similar to a desert. It seems incredible to carry out cardiomyocyte regeneration in an aneurysm in the absence of reperfusion. Clinical trials of cardiac regeneration after MI are usually performed in patients with reopening of the infarct-related coronary artery. In contrast, most of the animal studies on cardiac regeneration employed rodent MI models with permanent occlusion of the coronary artery. By searching the Web of Science database, we found more than 2500 original research papers focusing on MI-related heart regeneration in experiments using rodents, while only 28 papers adopted an ischemia/reperfusion model to study cardiac regeneration (Figure 3). Although many encouraging results on cardiomyocyte regeneration have been reported in MI animal models, it is still questionable how the regenerated cells survive without an adequate blood supply. Revascularization or surgical reshaping of the excessively dilated left ventricle would facilitate regenerative therapy (38, 39, 41).
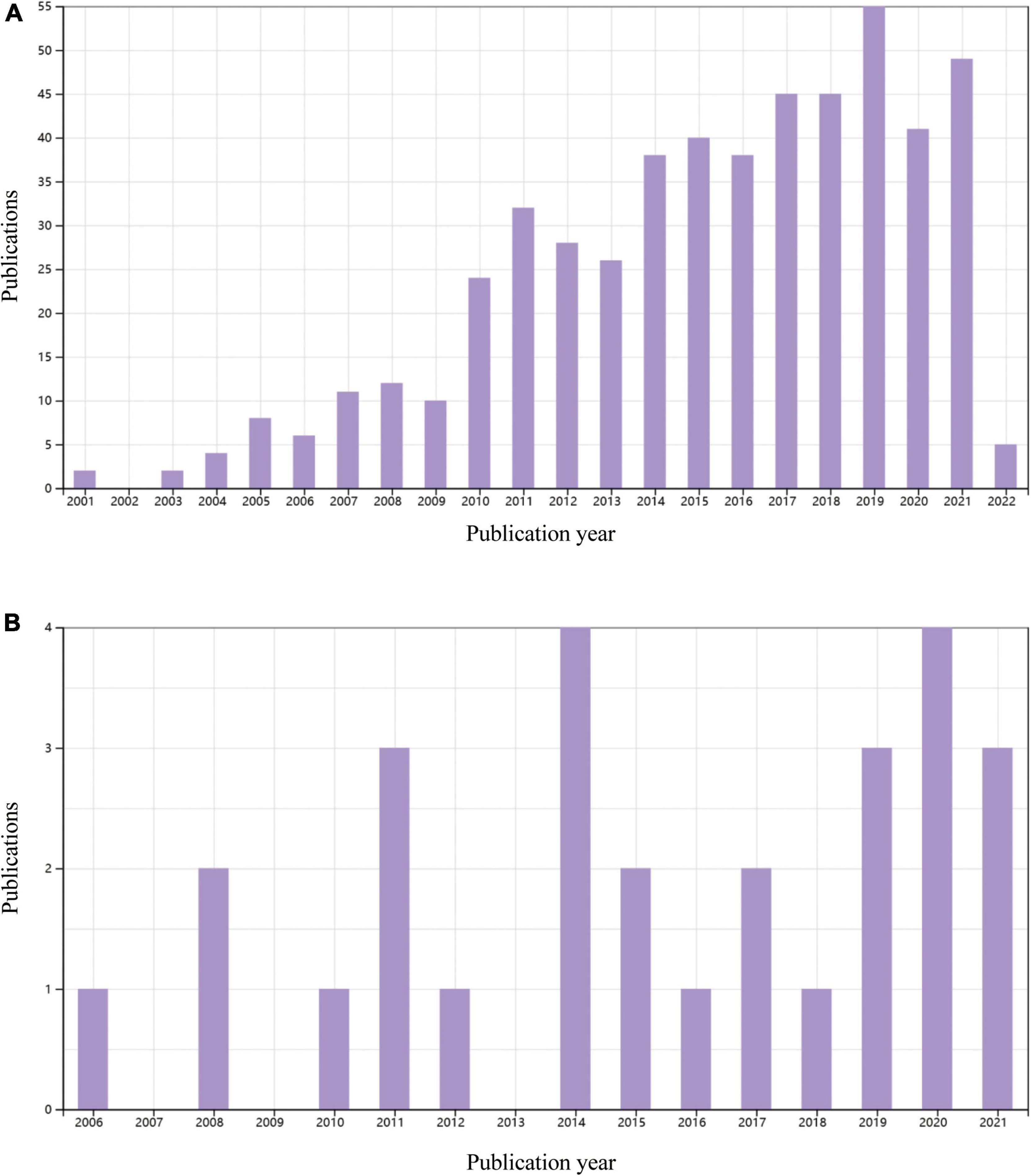
Figure 3. Original publications over time related to cardiac regenerative research in rodents with permanent myocardial infarction of myocardial ischemia/reperfusion in the last 20 years. (A) 521 papers on a permanent myocardial infarction model. Data source: Web of Science, searched with the topics “myocardial infarction” AND (”cardiac regeneration” OR “heart regeneration”) and then refined by document type “Articles” and MeSH headings of “Animals.” (B) 28 articles using a myocardial ischemia/reperfusion model. Data source: Web of Science, searched with the topic “ischemic/reperfusion” AND (”cardiac regeneration” OR “heart regeneration”) and then refined by document type “Articles” and MeSH headings of “Animals.” Reviews, meeting papers, and editorial materials were excluded from both (A,B).
Adult mammalian cardiomyocytes have poor proliferative and consequently regenerative potential following injury. The inability to replace lost cardiomyocytes after MI is paralleled by scarring at the injured area. Timely revascularization is an effective treatment to curb cardiac deterioration. Although it is largely unknown the effects and mechanisms of CTO-PCI on cardiac regeneration in patients, the key mechanisms of cardiac repair and regeneration after MI or ischemia/reperfusion clarified in animal studies likely work in patients with CTO-PCI. As summarized in Figure 4, cardiac regeneration may be achieved by way of: (1) alterations in the cardiac microenvironment, (2) angiogenesis/vascularization, (3) stem cell therapy, (4) proliferation and cell cycle molecular regulation. The adult heart consists of cardiac myocytes, endothelial cells (majority representing vascular endothelial cells), fibroblasts, and immune cells. Under physiological conditions, non-cardiomyocytes act on cardiomyocytes through a paracrine mechanism. In the CTO-PCI heart, due to changes in the cardiac microenvironment caused by the restoration of coronary artery blood flow, various cells in the heart act on cardiomyocytes through various mechanisms, promoting myocardial regeneration or reducing cardiomyocyte death, and ultimately improving cardiac function.
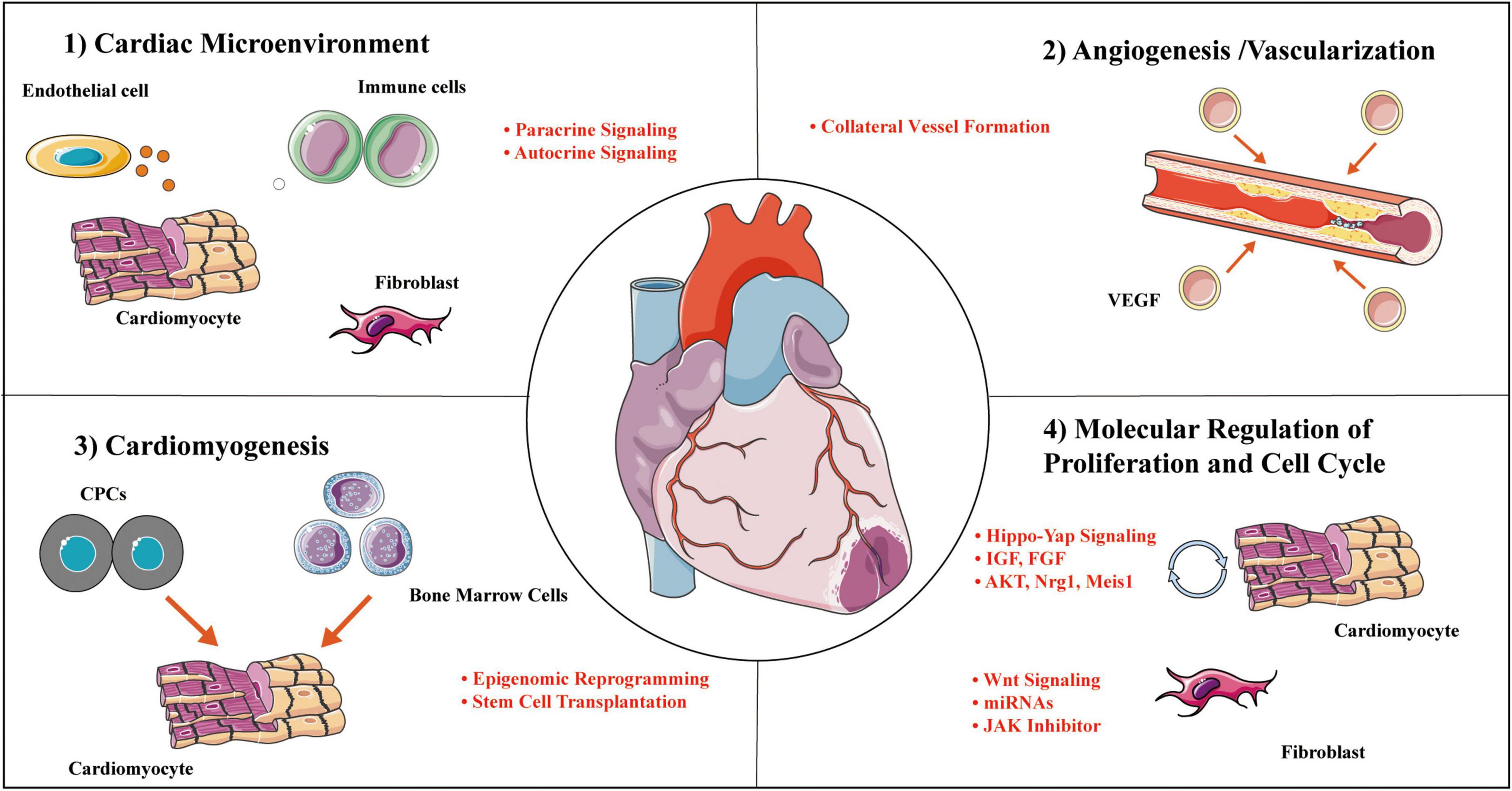
Figure 4. Cardial regeneration involves multiple mechanisms. Representative categories and selected examples of processes to enhance cardial regeneration covered in this review. Mechanisms work independently on a molecular level to collectively mediate concurrent cellular actions of regenerative responses. CPC, cardiac progenitor cell; IGF, insulin-like growth factor; JAK, janus kinase; Meis-1, Meis homobox 1; Mps-1, monopolar spindle 1; VEGF, vascular endothelial growth factor.
Angiogenesis is essential for the repair and regeneration of cardiac tissue after MI. The formation of new capillaries may be of clinical importance in facilitating regeneration in fibrotic cardiac tissue after MI. Vascular endothelial growth factor (VEGF) is a cornerstone cytokine involved in promoting the formation of new blood vessels, and thus has been a focus in the treatment of heart disease (42, 43). VEGF expression is increased in the epicardium and subepicardium cells of the aortic root, and these molecules are thought to regulate endothelial cell penetration into the aorta (44). In addition, direct intravenous injection of VEGF into endothelial cells induces an angiogenic phenotype similar to that found in coronary vessels (45). The delivery of VEGF-A in combination with various stent combinations has also been successful in stimulating angiogenesis and restoring cardiac function (46, 47). Members of the VEGF family are key regulators of the development of blood vessel and lymphatic vessels. Similar to systemic lymphatics, cardiac lymphatics require Vegfr3-Vegfc signaling to develop in genetic models such as Vegfr3–/– and Vegfc± zebrafish (48, 49). It is known that an adult zebrafish can regenerate its injured heart with an early response of coronary revascularization, while disruption of this process by blocking VEGFc signaling leads to impaired cardiomyocyte repopulation (50). VEGFc is secreted by the epicardium and pro-inflammatory macrophages after MI in mice, which drives lymphangiogenesis and extensive remodeling of the cardiac lymphatic network (51). This endogenous response of cardiac lymphatics attempts to maintain the optimal immune cell load necessary for effective tissue repair (52). Thus, disruption of Vedfr3-Vegfc pathway blocks lymphatic response to freeze injury, which leads to inefficient immune cell clearance and increased scar formation. Hence, coronary revascularization holds great therapeutic potential for myocyte regeneration.
At the same time, stem cell therapy is one of the most commonly used treatments for improving cardiac function in clinical studies of ICM after revascularization (Tables 3, 4). Possible mechanisms for its improved cardiac function include myocardial regeneration, angiogenesis, and paracrine activities of the cells. Even in a permanent MI mouse model without revascularization, angiogenesis is usually accompanied by successful myocyte proliferation in response to intramyocardial injection of exosomes secreted by human diosphere-derived cells or embryonic stem cells. (53, 54). Intramyocardial injection at the border zone of MI is not clinically appealing due to its invasive nature. Vandergriff et al. utilized an ischemia/reperfusion rat model to examine the effect of intravenously infused exosomes on cellular proliferation and angiogenesis (55). They noted that cardiac-homing peptide-derived exosomes significantly improved the outcomes of myocyte proliferation and angiogenesis (55). Similarly, systemic injection of regeneration-associated cells in a rat model of ischemia/reperfusion improved cardiac function and enhanced capillary density (56). These findings suggest that the reopening of the IRA is important for targeting exosomes to the infarcted heart. Numerous preclinical studies have shown that exosomes are protective in ischemic heart disease by alleviating myocardial ischemia–reperfusion injury, promoting angiogenesis, inhibiting fibrosis, and facilitating cardiac regeneration (57), further supporting the importance of simultaneously promoting myocyte proliferation and angiogenesis.
Genetic triggers for cell cycle reactivation to drive mitosis in adult cardiac myocytes have been advanced as potential therapeutic targets for cardiac regeneration. For example, Hipo-YAP signaling is critical for the intracellular regulation of cardiomyocyte proliferation. Hippo deficient mouse embryos developed cardiac hypertrophy, high proliferation of cardiomyocytes, and enhanced classical Wnt (wingless-type mouse mammary tumor virus)/β-catenin signaling (58). Yap-conditional knockout neonatal hearts failed to regenerate after MI at postnatal day 2, displayed extensive fibrotic infarct scar and deleterious loss of healthy myocardium, while constitutive Yap activation in adult heart significantly enhanced cardiac regeneration, improved cardiac function (59). The regeneration activity of Yap is partly related to the stimulation of the IGF/Akt/GSK-3β/β-catenin pathway. Another candidate for regulating cardiomyocyte proliferation is MEIS-1 (Meis homobox 1), a homeodomain transcription factor essential for normal cardiogenesis and embryonic hematopoiesis. Loss of MEIS-1 in the adult heart increases the number of cardiomyocytes that enter the cell cycle and increases cytokinesis (60). Moreover, fibroblasts play an important role in cardiac regeneration through myofibroblast transdifferentiation via the WNT signaling pathway. Using genetic engineering, fibroblasts can be induced to differentiate into cardiomyocytes or cardiac pluripotent stem cells with selected miRNA or JAK (Janus kinase) inhibitors (61, 62).
In addition to the above-mentioned cells in the heart, the cardiac rhythm cells have an irreplaceable role in maintaining the normal operation of the heart. Lerchenmüller et al. reported that exercise can induce cardiac regeneration and pathways related to circadian rhythm in mice (63). Although the evidence is limited on relations between myocardial regeneration and cardiac rhythmic cells after revascularization, it can be expected that circadian rhythm plays a crucial role in cardiac regeneration.
The feasibility of cardiac regeneration after chronic total occlusion revascularization in animals
It is unclear whether endogenous regenerative therapy would be more effective for the prognosis of patients with CTO revascularization. Basic research on CTO is largely limited due to the difficulty in establishing an experimental animal model of CTO that can accept manipulation of CTO revascularization. Animal experiments on myocardial ischemia are mostly performed in young and healthy animals that lack the risk factors and comorbidities that are characteristic of patients suffering from acute or chronic myocardial ischemia. Although there is no animal model that can fully mimic both CTO and CTO revascularization in humans, attempts toward creating an animal model of coronary recanalization would be helpful for seeking and confirming new therapeutic targets as well as clarifying the underlying mechanisms.
A major limitation of atherosclerotic animal models is that atherosclerotic plaques usually occur in the aorta and proximal great arteries rather than in the coronary arteries. It is workable to generate a CTO model by adding environmental stress to gene-targeted mice (64), but it is difficult to perform CTO revascularization in those animals. In 2019, Marino et al. reported a mouse model with atherosclerosis capable of recapitulating coronary plaque disruption, thrombosis, and MI (65). They demonstrated that exposure of the heart of ApoE knockout mice to high pressure could induce myocardial events due to coronary plaque thrombosis and occlusion in 74% of the mice. This model is strikingly similar to patients with coronary artery disease and hypertension, and some of those animals could experience coronary occlusion similar to human CTO. As early as 2002, Braun et al. reported that mice with double knockout of the high-density lipoprotein receptor SR-BI and ApoE exhibit coronary artery occlusion, spontaneous MI and cardiac dysfunction with similarities to those seen in human coronary artery disease, but all of those mice died at 8 weeks of age (66). In addition, ApoE–/–:Ins2+/Akita male mice fed a Western diet (hyperglycemic and hyperlipidemic mice) also have coronary atherosclerosis, MI and a significant reduction in lifespan (67), while chronic intermittent mental stress promotes plaque instability and MI in ApoE(–/–)fibrillin-1 (C1039G±) mice (68).
At present, there is no CTO model in large animals that simulates the developmental process of CTO in humans for the following reasons: (1) CTO in coronary arteries cannot be directly induced by surgical methods; (2) it is difficult for the coronary arteries of large animals to form atherosclerotic changes similar to those in humans, especially calcification; (3) the process of CTO also includes the occurrence of inflammatory reactions, which is not easy to achieve in animal models; and (4) although conventional interventional treatments such as balloon dilation and stent placement can cause damage to the coronary endothelium and the formation of neointima in animals, the probability of complete occlusion of the blood vessel is very small (69, 70). For the above reasons, many of the reported CTO models use the peripheral blood vessels of animals, which are not feasible for the study of cardiac regeneration.
The model animals in the basic research on interventional cardiology include mice, rats, rabbits, dogs, and pigs, among which the pig and rabbit models are the most commonly used because the response to injury of porcine coronary artery or rabbit femoral artery is closer to that of human coronary arteries, and the choice of surgical approach is more convenient (71). For the CTO model, more damage is needed to cause vascular occlusion. Compared with the miniature pig coronary artery model, the rabbit femoral artery model is relatively simple to establish and costs less. It was reported that the degree of injury in the rabbit femoral artery after balloon strain was very similar to that of the human coronary artery, both of which exhibited tearing of the vascular medium membrane and plaque rupture (72), and this model could simulate many features of human coronary CTO, including early thrombosis, an acute inflammatory response, and vascular remodeling (73, 74).
Coronary occlusion in large animals can be achieved by direct ligation or placement of an artery constrictor (75, 76), but few investigators have tried to reopen the vessels. Suzuki et al. from Japan used bone meal and an absorbable gelatin sponge to establish a coronary artery CTO lesion model in miniature pigs that could simulate the calcification process in human CTO and induce pathological processes such as inflammatory cell infiltration and the formation of bridge collaterals. More importantly, this type of coronary CTO could be reopened by interventional therapy (77). This model should be an ideal animal model that largely simulates human CTO, but there are obstacles to performing experimental studies on post-CTO regeneration of cardiomyocytes in the porcine heart due to the difficulty of gene manipulation and the high cost.
More accurate small-animal models that represent human CTO and heart failure are needed to perform early efficacy testing of novel regenerative therapies. A rodent model of CTO and CTO revascularization would be essential for connecting the basic and clinical research on post-CTO regeneration of cardiomyocytes. However, except for the acute ischemia/acute reperfusion model, no rodent model of CTO/reperfusion is available. It seems reasonable to use an absorbable suture to ligate a coronary artery to partially simulate CTO revascularization in mice or rats. Using a 2-week absorbable suture to constrict the mouse aortic arch, Lao et al. demonstrated that this procedure could cause significant myocardial hypertrophy at 2 weeks and that myocardial hypertrophy almost completely regressed to baseline at 4 weeks after surgery. (78). It is imaginable that absorbable suture ligation can induce complete coronary occlusion in the early stage and allow the coronary artery to reopen after the ligating suture has been absorbed in the late stage. One concern that should be noted is that permanent coronary ligation of the left coronary artery in mice would induce large ventricular aneurysm (40); in that situation, what is the value of reopening the ligated coronary artery? Surgical ventricular restoration to reshape the markedly dilated LV and collapse the large aneurysm would facilitate regenerative therapy (41). In fact, surgical ventricular restoration has repeatedly been suggested as a viable alternative in managing heart failure in select patients with a large LV and refractory heart failure, as it is believed that surgically returning the ventricle to its original dimensions is possible and is associated with favorable outcomes (79). It may be feasible to generate a murine model with a smaller infarct size using absorbable suture ligation, which would facilitate regenerative studies after CTO recanalization.
Clinical prospects
Ischemic cardiomyopathy is a major contributor to refractory heart failure, which has a poor prognosis. OMT and different coronary revascularization strategies are the mainstays in the management of ICM. Although the role of medications and mechanical circulatory support is ever increasing, cardiac transplantation remains the last hope for treating advanced heart failure. Limited by the small number of available and suitable donor hearts, efficient cardiac regeneration would be an ideal replacement for cardiac transplantation in alleviating heart failure.
Randomized controlled trials including the Decision CTO and the Euro CTO studies did not yield positive results, showing that CTO patients were not able to obtain hard end point improvement (reduction in MACEs) from PCI. However, this should not be misinterpreted to mean that CTO recanalization was an invalid measure (4). Based on clinical practice and relevant guidelines (80), the current indications for interventional treatment of CTO lesions include the following: (1) CTO with symptoms of myocardial ischemia, and CTO with poor angina control that is still present after OMT; (2) non-invasive examination confirmation of the presence of massive myocardial ischemia in the area dominated by the diseased vessels; and (3) coronary angiography showing that the occlusion is suitable for interventional therapy. Application of viability testing is helpful in predicting whether revascularization is able to prevent further damage by protecting the residual viable myocardium from subsequent acute coronary events (81). The J-CTO scoring system summarized based on the success rate of surgery can reflect the difficulty of CTO surgery to a large extent and predict the success rate of surgery (82).
As early as 2002–2004, three studies initiated cell-based therapy by intracoronary injections to treat patients with acute MI after percutaneous transluminal coronary angioplasty (83–85). In 2005, Erbs et al. first reported an intracoronary cell-therapy RCT in patients with coronary CTO but without heart failure after PCI (38). Early-stage clinical trials suggest that cardiac regeneration induced by exogenous cell therapy is effective in improving cardiac function in patients with ICM (32), but there are several shortcomings, such as low efficiency, ventricular arrhythmias, and immune rejection (86). Clinical translation of endogenous regenerative therapy would provide new hope for alleviating heart failure. Revascularization and surgical ventricular reshaping may be beneficial for improving the regenerative environment. Recanalization of CTO would enhance the delivery efficiency of endogenous regenerative factors such as extracellular vesicles and autologous mitochondria (87, 88), promote angiogenesis and deliver nutrients to the proliferated cardiomyocytes.
Chronic total occlusion revascularization, surgery to reshape the excessively enlarged left ventricle and the development of high-efficiency regenerative therapy may hold promise in the future for providing permanent solutions for refractory heart failure in patients with ICM.
Author contributions
HL and HS: concept design, data interpretation, manuscript writing and revising. RL: data collection, analysis, interpretation, and manuscript writing. ZL and QW: data analysis and interpretation. All authors contributed to the article and approved the submitted version.
Funding
This work was supported by grants from the National Natural Science Foundation of China (82073851 to HS and 82100407 to HL) and the China Postdoctoral Science Foundation (2021M690074 to HL).
Conflict of interest
The authors declare that the research was conducted in the absence of any commercial or financial relationships that could be construed as a potential conflict of interest.
Publisher’s note
All claims expressed in this article are solely those of the authors and do not necessarily represent those of their affiliated organizations, or those of the publisher, the editors and the reviewers. Any product that may be evaluated in this article, or claim that may be made by its manufacturer, is not guaranteed or endorsed by the publisher.
References
1. Farooq V, Serruys PW, Garcia-Garcia HM, Zhang Y, Bourantas CV, Holmes DR, et al. The negative impact of incomplete angiographic revascularization on clinical outcomes and its association with total occlusions: the SYNTAX (Synergy Between Percutaneous Coronary Intervention with Taxus and Cardiac Surgery) trial. J Am Coll Cardiol. (2013) 61:282–94. doi: 10.1016/j.jacc.2012.10.017
2. Lee JH, Park HS, Ryu HM, Lee H, Bae MH, Lee JH, et al. Impact of multivessel coronary disease with chronic total occlusion on one-year mortality in patients with acute myocardial infarction. Korean Circ J. (2012) 42:95–9. doi: 10.4070/kcj.2012.42.2.95
3. Khan AA, Khalid MF, Ayub MT, Murtaza G, Sardar R, White CJ, et al. Outcomes of percutaneous coronary intervention versus optimal medical treatment for chronic total occlusion: a comprehensive meta-analysis. Curr Prob Cardiol. (2021) 46:100695. doi: 10.1016/j.cpcardiol.2020.100695
4. Lee SW, Lee PH, Ahn JM, Park DW, Yun SC, Han S, et al. Randomized trial evaluating percutaneous coronary intervention for the treatment of chronic total occlusion. Circulation. (2019) 139:1674–83.
5. Henriques JP, Hoebers LP, Ramunddal T, Laanmets P, Eriksen E, Bax M, et al. Percutaneous intervention for concurrent chronic total occlusions in patients with STEMI: the EXPLORE Trial. J Am Coll Cardiol. (2016) 68:1622–32.
6. Werner GS, Martin-Yuste V, Hildick-Smith D, Boudou N, Sianos G, Gelev V, et al. A randomized multicentre trial to compare revascularization with optimal medical therapy for the treatment of chronic total coronary occlusions. Eur Heart J. (2018) 39:2484–93. doi: 10.1093/eurheartj/ehy220
7. Obedinskiy AA, Kretov EI, Boukhris M, Kurbatov VP, Osiev AG, Ibn Elhadj Z, et al. The IMPACTOR-CTO Trial. JACC Cardiovasc Interv. (2018) 11:1309–11. doi: 10.1016/j.jcin.2018.04.017
8. Wang L, Lu MJ, Feng L, Wang J, Fang W, He ZX, et al. Relationship of myocardial hibernation, scar, and angiographic collateral flow in ischemic cardiomyopathy with coronary chronic total occlusion. J Nucl Cardiol. (2019) 26:1720–30. doi: 10.1007/s12350-018-1241-8
9. Sedlakova V, Ahumada M, Suuronen EJ, Alarcon EI. Building new cardiac vasculature and myocardium: where are we at? Curr Opin Cardiol. (2021) 36:728–34.
10. Lu P, Wang Y, Liu Y, Wang Y, Wu B, Zheng D, et al. Perinatal angiogenesis from pre-existing coronary vessels via DLL4-NOTCH1 signalling. Nature Cell Biol. (2021) 23:967–77. doi: 10.1038/s41556-021-00747-1
11. Aghazadeh Y, Khan ST, Nkennor B, Nunes SS. Cell-based therapies for vascular regeneration: past, present and future. Pharmacol Therap. (2021) 231:107976.
12. Fefer P, Knudtson ML, Cheema AN, Galbraith PD, Osherov AB, Yalonetsky S, et al. Current perspectives on coronary chronic total occlusions: the Canadian multicenter chronic total occlusions registry. J Am Coll Cardiol. (2012) 59:991–7. doi: 10.1016/j.jacc.2011.12.007
13. Ramunddal T, Hoebers LP, Henriques JP, Dworeck C, Angeras O, Odenstedt J, et al. Chronic total occlusions in Sweden–a report from the Swedish coronary angiography and angioplasty registry (SCAAR). PLoS One. (2014) 9:e103850. doi: 10.1371/journal.pone.0103850
14. Schumacher SP, Stuijfzand WJ, Opolski MP, van Rossum AC, Nap A, Knaapen P. Percutaneous coronary intervention of chronic total occlusions: when and how to treat. Cardiovasc Revasc Med. (2019) 20:513–22.
15. Habib GB, Heibig J, Forman SA, Brown BG, Roberts R, Terrin ML, et al. Influence of coronary collateral vessels on myocardial infarct size in humans. Results of phase I thrombolysis in myocardial infarction (TIMI) trial. The TIMI investigators. Circulation. (1991) 83:739–46. doi: 10.1161/01.cir.83.3.739
16. Assaf A, Diletti R, Hoogendijk MG, van der Graaf M, Zijlstra F, Szili-Torok T, et al. Vulnerability for ventricular arrhythmias in patients with chronic coronary total occlusion. Expert Rev Cardiovasc Ther. (2020) 18:487–94.
17. Di Marco A, Anguera I, Teruel L, Muntane G, Campbell NG, Fox DJ, et al. Chronic total occlusion in an infarct-related coronary artery and the risk of appropriate ICD therapies. J Cardiovasc Electrophysiol. (2017) 28:1169–78. doi: 10.1111/jce.13290
18. Braik N, Guedeney P, Behnes M, Desch S, Barthelemy O, Sandri M, et al. Impact of chronic total occlusion and revascularization strategy in patients with infarct-related cardiogenic shock: a subanalysis of the culprit-shock trial. Am Heart J. (2021) 232:185–93. doi: 10.1016/j.ahj.2020.11.009
19. Watanabe H, Morimoto T, Shiomi H, Furukawa Y, Nakagawa Y, Ando K, et al. Chronic total occlusion in a non-infarct-related artery is closely associated with increased five-year mortality in patients with ST-segment elevation acute myocardial infarction undergoing primary percutaneous coronary intervention (from the CREDO-Kyoto AMI registry). EuroIntervention. (2017) 12:e1874–82. doi: 10.4244/EIJ-D-15-00421
20. Fujii T, Sakai K, Nakano M, Ohno Y, Nakazawa G, Shinozaki N, et al. Impact of the origin of the collateral feeding donor artery on short-term mortality in ST-elevation myocardial infarction with comorbid chronic total occlusion. Int J Cardiol. (2016) 218:158–63. doi: 10.1016/j.ijcard.2016.05.023
21. Claessen BE, Dangas GD, Weisz G, Witzenbichler B, Guagliumi G, Mockel M, et al. Prognostic impact of a chronic total occlusion in a non-infarct-related artery in patients with ST-segment elevation myocardial infarction: 3-year results from the HORIZONS-AMI trial. Eur Heart J. (2012) 33:768–75. doi: 10.1093/eurheartj/ehr471
22. Lexis CP, van der Horst IC, Rahel BM, Lexis MA, Kampinga MA, Gu YL, et al. Impact of chronic total occlusions on markers of reperfusion, infarct size, and long-term mortality: a substudy from the TAPAS-trial. Catheter Cardiovasc Interv. (2011) 77:484–91. doi: 10.1002/ccd.22664
23. Damluji AA, Pomenti SF, Ramireddy A, Al-Damluji MS, Alfonso CE, Schob AH, et al. Influence of total coronary occlusion on clinical outcomes (from the Bypass Angioplasty Revascularization Investigation 2 DiabetesTrial). Am J Cardiol. (2016) 117:1031–8. doi: 10.1016/j.amjcard.2015.12.047
24. Sachdeva R, Agrawal M, Flynn SE, Werner GS, Uretsky BF. The myocardium supplied by a chronic total occlusion is a persistently ischemic zone. Catheter Cardiovasc Interv. (2014) 83:9–16. doi: 10.1002/ccd.25001
25. Muscogiuri G, Ricci F, Scafuri S, Guglielmo M, Baggiano A, De Stasio V, et al. Cardiac magnetic resonance tissue characterization in ischemic cardiomyopathy. J Thorac Imaging. (2022) 37:2–16.
26. Bucciarelli-Ducci C, Auger D, Di Mario C, Locca D, Petryka J, O’Hanlon R, et al. Guidance for recanalization of coronary chronic total occlusion. JACC Cardiovasc Imaging. (2016) 9:547–56.
27. Elias J, van Dongen IM, Hoebers LP, Ouweneel DM, Claessen B, Ramunddal T, et al. Improved recovery of regional left ventricular function after PCI of chronic total occlusion in STEMI patients: a cardiovascular magnetic resonance study of the randomized controlled EXPLORE trial. J Cardiovasc Magn Reson. (2017) 19:53.
28. Khan MS, Sami F, Singh H, Ullah W, Al-Dabbas M, Changal KH, et al. Medical therapy vs early revascularization in diabetics with chronic total occlusions: a meta-analysis and systematic review. World J Cardiol. (2020) 12:559–70. doi: 10.4330/wjc.v12.i11.559
29. Kir D, Patel MJ, Munagala MR. What is the status of regenerative therapy in heart failure? Curr Cardiol Rep. (2021) 23:146.
30. Zhang J, Bolli R, Garry DJ, Marban E, Menasche P, Zimmermann WH, et al. Basic and translational research in cardiac repair and regeneration: JACC state-of-the-art review. J Am Coll Cardiol. (2021) 78:2092–105. doi: 10.1016/j.jacc.2021.09.019
31. Bolli R, Solankhi M, Tang XL, Kahlon A. Cell therapy in patients with heart failure: a comprehensive review and emerging concepts. Cardiovasc Res. (2021) 118:951–76. doi: 10.1093/cvr/cvab135
32. Nair N, Gongora E. Stem cell therapy in heart failure: where do we stand today? Biochim Biophys Acta Mol Basis Dis. (2020) 1866:165489. doi: 10.1016/j.bbadis.2019.06.003
33. Razeghian-Jahromi I, Matta AG, Canitrot R, Zibaeenezhad MJ, Razmkhah M, Safari A, et al. Surfing the clinical trials of mesenchymal stem cell therapy in ischemic cardiomyopathy. Stem Cell Res Ther. (2021) 12:361. doi: 10.1186/s13287-021-02443-1
34. Luger D, Lipinski MJ, Westman PC, Glover DK, Dimastromatteo J, Frias JC, et al. Intravenously delivered mesenchymal stem cells: systemic anti-inflammatory effects improve left ventricular dysfunction in acute myocardial infarction and ischemic cardiomyopathy. Circ Res. (2017) 120:1598–613. doi: 10.1161/CIRCRESAHA.117.310599
35. Choudhury T, Mozid A, Hamshere S, Yeo C, Pellaton C, Arnous S, et al. An exploratory randomized control study of combination cytokine and adult autologous bone marrow progenitor cell administration in patients with ischaemic cardiomyopathy: the REGENERATE-IHD clinical trial. Eur J Heart Fail. (2017) 19:138–47. doi: 10.1002/ejhf.676
36. Adler DS, Lazarus H, Nair R, Goldberg JL, Greco NJ, Lassar T, et al. Safety and efficacy of bone marrow-derived autologous CD133+ stem cell therapy. Front Biosci. (2011) 3:506–14. doi: 10.2741/e265
37. Li X, Hu YD, Guo Y, Chen Y, Guo DX, Zhou HL, et al. Safety and efficacy of intracoronary human umbilical cord-derived mesenchymal stem cell treatment for very old patients with coronary chronic total occlusion. Curr Pharm Des. (2015) 21:1426–32.
38. Erbs S, Linke A, Adams V, Lenk K, Thiele H, Diederich KW, et al. Transplantation of blood-derived progenitor cells after recanalization of chronic coronary artery occlusion: first randomized and placebo-controlled study. Circ Res. (2005) 97:756–62. doi: 10.1161/01.RES.0000185811.71306.8b
39. Kendziorra K, Barthel H, Erbs S, Emmrich F, Hambrecht R, Schuler G, et al. Effect of progenitor cells on myocardial perfusion and metabolism in patients after recanalization of a chronically occluded coronary artery. J Nucl Med. (2008) 49:557–63. doi: 10.2967/jnumed.107.046706
40. Zhu Y, Wang Q, Lin H, Chen K, Zheng C, Chen L, et al. Characterizing a long-term chronic heart failure model by transcriptomic alterations and monitoring of cardiac remodeling. Aging. (2021) 13:13585–614. doi: 10.18632/aging.202879
41. Ma S, Yan J, Yang D, Liao W, Bin J, Lin H, et al. A modified surgical ventricular reconstruction in post-infarction mice persistently alleviates heart failure and improves cardiac regeneration. Front Cardiovasc Med. (2021) 8:789493. doi: 10.3389/fcvm.2021.789493
42. Crottogini A, Meckert PC, Vera Janavel G, Lascano E, Negroni J, Del Valle H, et al. Arteriogenesis induced by intramyocardial vascular endothelial growth factor 165 gene transfer in chronically ischemic pigs. Hum Gene Ther. (2003) 14:1307–18. doi: 10.1089/104303403322319390
43. Locatelli P, Olea FD, Hnatiuk A, De Lorenzi A, Cerda M, Gimenez CS, et al. Mesenchymal stromal cells overexpressing vascular endothelial growth factor in ovine myocardial infarction. Gene Ther. (2015) 22:449–57.
44. Ramai D, Lai J, Monzidelis C, Reddy S. Coronary artery development: origin. malformations, and translational vascular reparative therapy. J Cardiovasc Pharmacol Ther. (2018) 23:292–300. doi: 10.1177/1074248418769633
45. Weis SM, Lindquist JN, Barnes LA, Lutu-Fuga KM, Cui J, Wood MR, et al. Cooperation between VEGF and beta3 integrin during cardiac vascular development. Blood. (2007) 109:1962–70. doi: 10.1182/blood-2005-10-038893
46. Wu J, Zeng F, Huang XP, Chung JC, Konecny F, Weisel RD, et al. Infarct stabilization and cardiac repair with a VEGF-conjugated, injectable hydrogel. Biomaterials. (2011) 32:579–86. doi: 10.1016/j.biomaterials.2010.08.098
47. Formiga FR, Pelacho B, Garbayo E, Abizanda G, Gavira JJ, Simon-Yarza T, et al. Sustained release of VEGF through PLGA microparticles improves vasculogenesis and tissue remodeling in an acute myocardial ischemia-reperfusion model. J Control Release. (2010) 147:30–7. doi: 10.1016/j.jconrel.2010.07.097
48. Vivien CJ, Pichol-Thievend C, Sim CB, Smith JB, Bower NI, Hogan BM, et al. Vegfc/d-dependent regulation of the lymphatic vasculature during cardiac regeneration is influenced by injury context. NPJ Regen Med. (2019) 4:18. doi: 10.1038/s41536-019-0079-2
49. Harrison MR, Feng X, Mo G, Aguayo A, Villafuerte J, Yoshida T, et al. Late developing cardiac lymphatic vasculature supports adult zebrafish heart function and regeneration. Elife. (2019) 8:e42762. doi: 10.7554/eLife.42762
50. El-Sammak H, Yang B, Guenther S, Chen W, Marin-Juez R, Stainier DYR. A Vegfc-Emilin2a-Cxcl8a signaling axis required for zebrafish cardiac regeneration. Circ Res. (2022) 130:1014–29. doi: 10.1161/CIRCRESAHA.121.319929
51. Henri O, Pouehe C, Houssari M, Galas L, Nicol L, Edwards-Levy F, et al. Selective stimulation of cardiac lymphangiogenesis reduces myocardial edema and fibrosis leading to improved cardiac function following myocardial infarction. Circulation. (2016) 133:1484–97.
52. Vieira JM, Norman S, Villa Del Campo C, Cahill TJ, Barnette DN, Gunadasa-Rohling M, et al. The cardiac lymphatic system stimulates resolution of inflammation following myocardial infarction. J Clin Invest. (2018) 128:3402–12. doi: 10.1172/JCI97192
53. Ibrahim AG, Cheng K, Marban E. Exosomes as critical agents of cardiac regeneration triggered by cell therapy. Stem Cell Rep. (2014) 2:606–19. doi: 10.1016/j.stemcr.2014.04.006
54. Khan M, Nickoloff E, Abramova T, Johnson J, Verma SK, Krishnamurthy P, et al. Embryonic stem cell-derived exosomes promote endogenous repair mechanisms and enhance cardiac function following myocardial infarction. Circ Res. (2015) 117:52–64. doi: 10.1161/CIRCRESAHA.117.305990
55. Vandergriff A, Huang K, Shen D, Hu S, Hensley MT, Caranasos TG, et al. Targeting regenerative exosomes to myocardial infarction using cardiac homing peptide. Theranostics. (2018) 8:1869–78. doi: 10.7150/thno.20524
56. Salybekov AA, Salybekova A, Sheng Y, Shinozaki Y, Yokoyama K, Kobayashi S, et al. Extracellular vesicles derived from regeneration associated cells preserve heart function after ischemia-induced injury. Front Cardiovasc Med. (2021) 8:754254. doi: 10.3389/fcvm.2021.78949
57. Chen GH, Xu J, Yang YJ. Exosomes: promising sacks for treating ischemic heart disease? Am J Physiol Heart Circ Physiol. (2017) 313:H508–23. doi: 10.1152/ajpheart.00213.2017
58. Heallen T, Zhang M, Wang J, Bonilla-Claudio M, Klysik E, Johnson RL, et al. Hippo pathway inhibits Wnt signaling to restrain cardiomyocyte proliferation and heart size. Science. (2011) 332:458–61. doi: 10.1126/science.1199010
59. Xin M, Kim Y, Sutherland LB, Murakami M, Qi X, McAnally J, et al. Hippo pathway effector Yap promotes cardiac regeneration. Proc Natl Acad Sci U.S.A. (2013) 110:13839–44.
60. Mahmoud AI, Kocabas F, Muralidhar SA, Kimura W, Koura AS, Thet S, et al. Meis1 regulates postnatal cardiomyocyte cell cycle arrest. Nature. (2013) 497:249–53.
61. Ieda M, Fu JD, Delgado-Olguin P, Vedantham V, Hayashi Y, Bruneau BG, et al. Direct reprogramming of fibroblasts into functional cardiomyocytes by defined factors. Cell. (2010) 142:375–86.
62. Broughton KM, Wang BJ, Firouzi F, Khalafalla F, Dimmeler S, Fernandez-Aviles F, et al. Mechanisms of cardiac repair and regeneration. Circ Res. (2018) 122:1151–63.
63. Lerchenmuller C, Vujic A, Mittag S, Wang A, Rabolli CP, Hess C, et al. Restoration of cardiomyogenesis in aged mouse hearts by voluntary exercise. Circulation. (2022) 146:412–26. doi: 10.1161/CIRCULATIONAHA.121.057276
64. Golforoush P, Yellon DM, Davidson SM. Mouse models of atherosclerosis and their suitability for the study of myocardial infarction. Basic Res Cardiol. (2020) 115:73. doi: 10.1007/s00395-020-00829-5
65. Marino A, Zhang Y, Rubinelli L, Riemma MA, Ip JE, Di Lorenzo A. Pressure overload leads to coronary plaque formation, progression, and myocardial events in ApoE(-)(/-) mice. JCI Insight. (2019) 4:e128220. doi: 10.1172/jci.insight.128220
66. Braun A, Trigatti BL, Post MJ, Sato K, Simons M, Edelberg JM, et al. Loss of SR-BI expression leads to the early onset of occlusive atherosclerotic coronary artery disease, spontaneous myocardial infarctions, severe cardiac dysfunction, and premature death in apolipoprotein E-deficient mice. Circ Res. (2002) 90:270–6. doi: 10.1161/hh0302.104462
67. Venegas-Pino DE, Lagrotteria A, Wang PW, Morphet J, Clapdorp C, Shi Y, et al. Evidence of extensive atherosclerosis, coronary artery disease and myocardial infarction in the ApoE(-/-):Ins2(+/Akita) mouse fed a western diet. Atherosclerosis. (2018) 275:88–96. doi: 10.1016/j.atherosclerosis.2018.05.044
68. Roth L, Rombouts M, Schrijvers DM, Lemmens K, De Keulenaer GW, Martinet W, et al. Chronic intermittent mental stress promotes atherosclerotic plaque vulnerability, myocardial infarction and sudden death in mice. Atherosclerosis. (2015) 242:288–94. doi: 10.1016/j.atherosclerosis.2015.07.025
69. Katsuragawa M, Fujiwara H, Miyamae M, Sasayama S. Histologic studies in percutaneous transluminal coronary angioplasty for chronic total occlusion: comparison of tapering and abrupt types of occlusion and short and long occluded segments. J Am Coll Cardiol. (1993) 21:604–11. doi: 10.1016/0735-1097(93)90091-e
70. Srivatsa SS, Edwards WD, Boos CM, Grill DE, Sangiorgi GM, Garratt KN, et al. Histologic correlates of angiographic chronic total coronary artery occlusions: influence of occlusion duration on neovascular channel patterns and intimal plaque composition. J Am Coll Cardiol. (1997) 29:955–63. doi: 10.1016/s0735-1097(97)00035-1
71. Schwartz RS, Edelman ER, Carter A, Chronos NA, Rogers C, Robinson KA, et al. Preclinical evaluation of drug-eluting stents for peripheral applications: recommendations from an expert consensus group. Circulation. (2004) 110:2498–505. doi: 10.1161/01.CIR.0000145164.85178.2E
72. Schwartz RS, Edelman E, Virmani R, Carter A, Granada JF, Kaluza GL, et al. Drug-eluting stents in preclinical studies: updated consensus recommendations for preclinical evaluation. Circ Cardiovasc Interv. (2008) 1:143–53. doi: 10.1161/CIRCINTERVENTIONS.108.789974
73. Strauss BH, Goldman L, Qiang B, Nili N, Segev A, Butany J, et al. Collagenase plaque digestion for facilitating guide wire crossing in chronic total occlusions. Circulation. (2003) 108:1259–62. doi: 10.1161/01.CIR.0000086320.24172.A1
74. Jaffe R, Leung G, Munce NR, Thind AS, Leong-Poi H, Anderson KJ, et al. Natural history of experimental arterial chronic total occlusions. J Am Coll Cardiol. (2009) 53:1148–58.
75. Elzinga WE. Ameroid constrictor: uniform closure rates and a calibration procedure. J Appl Physiol. (1969) 27:419–21. doi: 10.1152/jappl.1969.27.3.419
76. Bredee JJ, Blickman JR, Holman van der Heide JN, Kootstra GJ, Zeelenberg HJ, Zijlstra WG. Standardized induction of myocardial ischaemia in the dog. Eur Surg Res. (1975) 7:269–86.
77. Suzuki K, Saito N, Zhang G, Conditt G, McGregor J, Flynn AM, et al. Development of a novel calcified total occlusion model in porcine coronary arteries. J Invasive Cardiol. (2008) 20:296–301.
78. Lao Y, Zheng C, Zhu H, Lin H, Huang X, Liao Y. Operating transverse aortic constriction with absorbable suture to obtain transient myocardial hypertrophy. J Vis Exp. (2020) 163. doi: 10.3791/61686
79. Fatehi Hassanabad A, Wiebe K, Ali IS. Clinical and hemodynamic outcomes of the Dor procedure in adults with ischemic cardiomyopathy. J Cardiac Surg. (2021) 36:4345–66.
80. Brilakis ES, Mashayekhi K, Tsuchikane E, Abi Rafeh N, Alaswad K, Araya M, et al. Guiding principles for chronic total occlusion percutaneous coronary intervention. Circulation. (2019) 140:420–33.
81. Panza JA, Chrzanowski L, Bonow RO. Myocardial viability assessment before surgical revascularization in ischemic cardiomyopathy: JACC review topic of the week. J Am Coll Cardiol. (2021) 78:1068–77. doi: 10.1016/j.jacc.2021.07.004
82. Morino Y, Abe M, Morimoto T, Kimura T, Hayashi Y, Muramatsu T, et al. Predicting successful guidewire crossing through chronic total occlusion of native coronary lesions within 30 minutes: the J-CTO (Multicenter CTO Registry in Japan) score as a difficulty grading and time assessment tool. JACC Cardiovasc Interv. (2011) 4:213–21. doi: 10.1016/j.jcin.2010.09.024
83. Strauer BE, Brehm M, Zeus T, Kostering M, Hernandez A, Sorg RV, et al. Repair of infarcted myocardium by autologous intracoronary mononuclear bone marrow cell transplantation in humans. Circulation. (2002) 106:1913–8.
84. Assmus B, Schachinger V, Teupe C, Britten M, Lehmann R, Dobert N, et al. Transplantation of progenitor cells and regeneration enhancement in acute myocardial infarction (TOPCARE-AMI). Circulation. (2002) 106:3009–17.
85. Wollert KC, Meyer GP, Lotz J, Ringes-Lichtenberg S, Lippolt P, Breidenbach C, et al. Intracoronary autologous bone-marrow cell transfer after myocardial infarction: the BOOST randomised controlled clinical trial. Lancet. (2004) 364:141–8.
86. Garbern JC, Lee RT. Heart regeneration: 20 years of progress and renewed optimism. Dev Cell. (2022) 57:424–39. doi: 10.1016/j.devcel.2022.01.012
87. Ju C, Shen Y, Ma G, Liu Y, Cai J, Kim IM, et al. Transplantation of cardiac mesenchymal stem cell-derived exosomes promotes repair in ischemic myocardium. J Cardiovasc Transl Res. (2018) 11:420–8.
88. Blitzer D, Guariento A, Doulamis IP, Shin B, Moskowitzova K, Barbieri GR, et al. Delayed transplantation of autologous mitochondria for cardioprotection in a porcine model. Ann Thorac Surg. (2020) 109:711–9. doi: 10.1016/j.athoracsur.2019.06.075
89. Arslan U, Balcioglu AS, Timurkaynak T, Cengel A. The clinical outcomes of percutaneous coronary intervention in chronic total coronary occlusion. Int Heart J. (2006) 47:811–9.
90. Valenti R, Marrani M, Cantini G, Migliorini A, Carrabba N, Vergara R, et al. Impact of chronic total occlusion revascularization in patients with acute myocardial infarction treated by primary percutaneous coronary intervention. Am J Cardiol. (2014) 114:1794–800.
91. Ladwiniec A, Allgar V, Thackray S, Alamgir F, Hoye A. Medical therapy, percutaneous coronary intervention and prognosis in patients with chronic total occlusions. Heart. (2015) 101:1907–14.
92. Jang WJ, Yang JH, Choi SH, Song YB, Hahn JY, Choi JH, et al. Long-term survival benefit of revascularization compared with medical therapy in patients with coronary chronic total occlusion and well-developed collateral circulation. JACC Cardiovasc Interv. (2015) 8:271–9. doi: 10.1016/j.jcin.2014.10.010
93. Tomasello SD, Boukhris M, Giubilato S, Marza F, Garbo R, Contegiacomo G, et al. Management strategies in patients affected by chronic total occlusions: results from the Italian registry of chronic total occlusions. Eur Heart J. (2015) 36:3189–98. doi: 10.1093/eurheartj/ehv450
94. Hwang JW, Yang JH, Choi SH, Hwang JK, Jang WJ, Hahn JY, et al. Optimal medical therapy may be a better initial strategy in patients with chronic total occlusion of a single coronary artery. Int J Cardiol. (2016) 210:56–62. doi: 10.1016/j.ijcard.2016.02.084
95. Yang JH, Kim BS, Jang WJ, Ahn J, Park TK, Song YB, et al. Optimal medical therapy vs. percutaneous coronary intervention for patients with coronary chronic total occlusion - a propensity-matched analysis. Circ J. (2016) 80:211–7. doi: 10.1253/circj.CJ-15-0673
96. Shuvy M, Qiu F, Chee ATA, Graham JJ, Abuzeid W, Buller C, et al. Management of chronic total coronary occlusion in stable ischemic heart disease by percutaneous coronary intervention versus coronary artery bypass grafting versus medical therapy. Am J Cardiol. (2017) 120:759–64.
97. Choi SY, Choi BG, Rha SW, Baek MJ, Ryu YG, Park Y, et al. Percutaneous coronary intervention versus optimal medical therapy for chronic total coronary occlusion with well-developed collaterals. J Am Heart Assoc. (2017) 6:e006357.
98. Guo L, Zhong L, Chen K, Wu J, Huang RC. Long-term clinical outcomes of optimal medical therapy vs. successful percutaneous coronary intervention for patients with coronary chronic total occlusions. Hellenic J Cardiol. (2018) 59:281–7.
99. Choo EH, Koh YS, Seo SM, Lee JM, Kim HY, Park HJ, et al. Comparison of successful percutaneous coronary intervention versus optimal medical therapy in patients with coronary chronic total occlusion. J Cardiol. (2019) 73:156–62.
100. Rha SW, Choi BG, Baek MJ, Ryu YG, Li H, Choi SY, et al. Five-Year outcomes of successful percutaneous coronary intervention with drug-eluting stents versus medical therapy for chronic total occlusions. Yonsei Med J. (2018) 59:602–10. doi: 10.3349/ymj.2018.59.5.602
101. Choi JY, Rha SW, Choi BG, Choi SY, Byun JK, Jang WY, et al. Percutaneous coronary intervention for chronic total occlusion in single coronary arteries. Tex Heart Inst J. (2021) 48:e197023.
102. Juricic SA, Tesic MB, Galassi AR, Petrovic ON, Dobric MR, Orlic DN, et al. Randomized controlled comparison of optimal medical therapy with percutaneous recanalization of chronic total occlusion (COMET-CTO). Int Heart J. (2021) 62:16–22. doi: 10.1536/ihj.20-427
103. Schachinger V, Erbs S, Elsasser A, Haberbosch W, Hambrecht R, Holschermann H, et al. Intracoronary bone marrow-derived progenitor cells in acute myocardial infarction. N Engl J Med. (2006) 355:1210–21.
104. Lunde K, Solheim S, Aakhus S, Arnesen H, Abdelnoor M, Egeland T, et al. Intracoronary injection of mononuclear bone marrow cells in acute myocardial infarction. N Engl J Med. (2006) 355:1199–209.
105. Huikuri HV, Kervinen K, Niemela M, Ylitalo K, Saily M, Koistinen P, et al. Effects of intracoronary injection of mononuclear bone marrow cells on left ventricular function, arrhythmia risk profile, and restenosis after thrombolytic therapy of acute myocardial infarction. Eur Heart J. (2008) 29:2723–32. doi: 10.1093/eurheartj/ehn436
106. Zhao Q, Sun Y, Xia L, Chen A, Wang Z. Randomized study of mononuclear bone marrow cell transplantation in patients with coronary surgery. Ann Thorac Surg. (2008) 86:1833–40.
107. Ang KL, Chin D, Leyva F, Foley P, Kubal C, Chalil S, et al. Randomized, controlled trial of intramuscular or intracoronary injection of autologous bone marrow cells into scarred myocardium during CABG versus CABG alone. Nat Clin Pract Cardiovasc Med. (2008) 5:663–70.
108. Hirsch A, Nijveldt R, van der Vleuten PA, Tijssen JG, van der Giessen WJ, Tio RA, et al. Intracoronary infusion of mononuclear cells from bone marrow or peripheral blood compared with standard therapy in patients after acute myocardial infarction treated by primary percutaneous coronary intervention: results of the randomized controlled HEBE trial. Eur Heart J. (2011) 32:1736–47.
109. Traverse JH, Henry TD, Ellis SG, Pepine CJ, Willerson JT, Zhao DX, et al. Effect of intracoronary delivery of autologous bone marrow mononuclear cells 2 to 3 weeks following acute myocardial infarction on left ventricular function: the LateTIME randomized trial. JAMA. (2011) 306:2110–9.
110. Hu S, Liu S, Zheng Z, Yuan X, Li L, Lu M, et al. Isolated coronary artery bypass graft combined with bone marrow mononuclear cells delivered through a graft vessel for patients with previous myocardial infarction and chronic heart failure: a single-center, randomized, double-blind, placebo-controlled clinical trial. J Am Coll Cardiol. (2011) 57:2409–15. doi: 10.1016/j.jacc.2011.01.037
111. Patila T, Lehtinen M, Vento A, Schildt J, Sinisalo J, Laine M, et al. Autologous bone marrow mononuclear cell transplantation in ischemic heart failure: a prospective, controlled, randomized, double-blind study of cell transplantation combined with coronary bypass. J Heart Lung Transplant. (2014) 33:567–74.
112. Can A, Ulus AT, Cinar O, Topal Celikkan F, Simsek E, Akyol M, et al. Human umbilical cord mesenchymal stromal cell transplantation in myocardial ischemia (HUC-HEART Trial). A study protocol of a phase 1/2, controlled and randomized trial in combination with coronary artery bypass grafting. Stem Cell Rev Rep. (2015) 11:752–60. doi: 10.1007/s12015-015-9601-0
113. Nicolau JC, Furtado RHM, Silva SA, Rochitte CE, Rassi A Jr, Moraes J Jr, et al. Stem-cell therapy in ST-segment elevation myocardial infarction with reduced ejection fraction: a multicenter, double-blind randomized trial. Clin Cardiol. (2018) 41:392–9.
114. Lim SY, Kim YS, Ahn Y, Jeong MH, Hong MH, Joo SY, et al. The effects of mesenchymal stem cells transduced with Akt in a porcine myocardial infarction model. Cardiovasc Res. (2006) 70:530–42. doi: 10.1016/j.cardiores.2006.02.016
115. Moelker AD, Baks T, van den Bos EJ, van Geuns RJ, de Feyter PJ, Duncker DJ, et al. Reduction in infarct size, but no functional improvement after bone marrow cell administration in a porcine model of reperfused myocardial infarction. Eur Heart J. (2006) 27:3057–64. doi: 10.1093/eurheartj/ehl401
116. Price MJ, Chou CC, Frantzen M, Miyamoto T, Kar S, Lee S, et al. Intravenous mesenchymal stem cell therapy early after reperfused acute myocardial infarction improves left ventricular function and alters electrophysiologic properties. Int J Cardiol. (2006) 111:231–9. doi: 10.1016/j.ijcard.2005.07.036
117. Makela J, Ylitalo K, Lehtonen S, Dahlbacka S, Niemela E, Kiviluoma K, et al. Bone marrow-derived mononuclear cell transplantation improves myocardial recovery by enhancing cellular recruitment and differentiation at the infarction site. J Thorac Cardiovasc Surg. (2007) 134:565–73. doi: 10.1016/j.jtcvs.2007.05.004
118. Moelker AD, Baks T, Wever KM, Spitskovsky D, Wielopolski PA, van Beusekom HM, et al. Intracoronary delivery of umbilical cord blood derived unrestricted somatic stem cells is not suitable to improve LV function after myocardial infarction in swine. J Mol Cell Cardiol. (2007) 42:735–45. doi: 10.1016/j.yjmcc.2007.01.005
119. Qian H, Yang Y, Huang J, Gao R, Dou K, Yang G, et al. Intracoronary delivery of autologous bone marrow mononuclear cells radiolabeled by 18F-fluoro-deoxy-glucose: tissue distribution and impact on post-infarct swine hearts. J Cell Biochem. (2007) 102:64–74. doi: 10.1002/jcb.21277
120. Valina C, Pinkernell K, Song YH, Bai X, Sadat S, Campeau RJ, et al. Intracoronary administration of autologous adipose tissue-derived stem cells improves left ventricular function, perfusion, and remodelling after acute myocardial infarction. Eur Heart J. (2007) 28:2667–77.
121. Yang ZJ, Ma DC, Wang W, Xu SL, Zhang YQ, Chen B, et al. Neovascularization and cardiomyocytes regeneration in acute myocardial infarction after bone marrow stromal cell transplantation: comparison of infarct-relative and noninfarct-relative arterial approaches in swine. Clin Chim Acta. (2007) 381:114–8. doi: 10.1016/j.cca.2007.02.035
122. de Silva R, Raval AN, Hadi M, Gildea KM, Bonifacino AC, Yu ZX, et al. Intracoronary infusion of autologous mononuclear cells from bone marrow or granulocyte colony-stimulating factor-mobilized apheresis product may not improve remodelling, contractile function, perfusion, or infarct size in a swine model of large myocardial infarction. Eur Heart J. (2008) 29:1772–82.
123. Doyle B, Sorajja P, Hynes B, Kumar AH, Araoz PA, Stalboerger PG, et al. Progenitor cell therapy in a porcine acute myocardial infarction model induces cardiac hypertrophy, mediated by paracrine secretion of cardiotrophic factors including TGFbeta1. Stem Cells Dev. (2008) 17:941–51. doi: 10.1089/scd.2007.0214
124. Gyongyosi M, Blanco J, Marian T, Tron L, Petnehazy O, Petrasi Z, et al. Serial noninvasive in vivo positron emission tomographic tracking of percutaneously intramyocardially injected autologous porcine mesenchymal stem cells modified for transgene reporter gene expression. Circ Cardiovasc Imaging. (2008) 1:94–103. doi: 10.1161/CIRCIMAGING.108.797449
125. Halkos ME, Zhao ZQ, Kerendi F, Wang NP, Jiang R, Schmarkey LS, et al. Intravenous infusion of mesenchymal stem cells enhances regional perfusion and improves ventricular function in a porcine model of myocardial infarction. Basic Res Cardiol. (2008) 103:525–36. doi: 10.1007/s00395-008-0741-0
126. Hashemi SM, Ghods S, Kolodgie FD, Parcham-Azad K, Keane M, Hamamdzic D, et al. A placebo controlled, dose-ranging, safety study of allogenic mesenchymal stem cells injected by endomyocardial delivery after an acute myocardial infarction. Eur Heart J. (2008) 29:251–9. doi: 10.1093/eurheartj/ehm559
127. Perin EC, Silva GV, Assad JA, Vela D, Buja LM, Sousa AL, et al. Comparison of intracoronary and transendocardial delivery of allogeneic mesenchymal cells in a canine model of acute myocardial infarction. J Mol Cell Cardiol. (2008) 44:486–95. doi: 10.1016/j.yjmcc.2007.09.012
128. Qi CM, Ma GS, Liu NF, Shen CX, Chen Z, Liu XJ, et al. Transplantation of magnetically labeled mesenchymal stem cells improves cardiac function in a swine myocardial infarction model. Chin Med J. (2008) 121:544–50.
129. Schuleri KH, Amado LC, Boyle AJ, Centola M, Saliaris AP, Gutman MR, et al. Early improvement in cardiac tissue perfusion due to mesenchymal stem cells. Am J Physiol Heart Circ Physiol. (2008) 294:H2002–11.
130. Johnston PV, Sasano T, Mills K, Evers R, Lee ST, Smith RR, et al. Engraftment, differentiation, and functional benefits of autologous cardiosphere-derived cells in porcine ischemic cardiomyopathy. Circulation. (2009) 120:1075–83. doi: 10.1161/CIRCULATIONAHA.108.816058
131. Quevedo HC, Hatzistergos KE, Oskouei BN, Feigenbaum GS, Rodriguez JE, Valdes D, et al. Allogeneic mesenchymal stem cells restore cardiac function in chronic ischemic cardiomyopathy via trilineage differentiating capacity. Proc Natl Acad Sci U.S.A. (2009) 106:14022–7. doi: 10.1073/pnas.0903201106
132. Schuleri KH, Feigenbaum GS, Centola M, Weiss ES, Zimmet JM, Turney J, et al. Autologous mesenchymal stem cells produce reverse remodelling in chronic ischaemic cardiomyopathy. Eur Heart J. (2009) 30:2722–32.
133. Wang X, Jameel MN, Li Q, Mansoor A, Qiang X, Swingen C, et al. Stem cells for myocardial repair with use of a transarterial catheter. Circulation. (2009) 120:S238–46.
134. Yang YJ, Qian HY, Huang J, Li JJ, Gao RL, Dou KF, et al. Combined therapy with simvastatin and bone marrow-derived mesenchymal stem cells increases benefits in infarcted swine hearts. Arterioscler Thromb Vasc Biol. (2009) 29:2076–82. doi: 10.1161/ATVBAHA.109.189662
135. Jiang Y, Chen L, Tang Y, Ma G, Shen C, Qi C, et al. HO-1 gene overexpression enhances the beneficial effects of superparamagnetic iron oxide labeled bone marrow stromal cells transplantation in swine hearts underwent ischemia/reperfusion: an MRI study. Basic Res Cardiol. (2010) 105:431–42. doi: 10.1007/s00395-009-0079-2
136. Arslan F, Lai RC, Smeets MB, Akeroyd L, Choo A, Aguor EN, et al. Mesenchymal stem cell-derived exosomes increase ATP levels, decrease oxidative stress and activate PI3K/Akt pathway to enhance myocardial viability and prevent adverse remodeling after myocardial ischemia/reperfusion injury. Stem Cell Res. (2013) 10:301–12. doi: 10.1016/j.scr.2013.01.002
137. Agarwal U, George A, Bhutani S, Ghosh-Choudhary S, Maxwell JT, Brown ME, et al. Experimental, systems, and computational approaches to understanding the MicroRNA-Mediated reparative potential of cardiac progenitor cell-derived exosomes from pediatric patients. Circ Res. (2017) 120:701–12.
138. Gallet R, Dawkins J, Valle J, Simsolo E, de Couto G, Middleton R, et al. Exosomes secreted by cardiosphere-derived cells reduce scarring, attenuate adverse remodelling, and improve function in acute and chronic porcine myocardial infarction. Eur Heart J. (2017) 38:201–11. doi: 10.1093/eurheartj/ehw240
139. Liu L, Jin X, Hu CF, Li R, Zhou Z, Shen CX. Exosomes derived from mesenchymal stem cells rescue myocardial ischaemia/reperfusion injury by inducing cardiomyocyte autophagy via AMPK and Akt pathways. Cell Physiol Biochem. (2017) 43:52–68. doi: 10.1159/000480317
140. Adamiak M, Cheng G, Bobis-Wozowicz S, Zhao L, Kedracka-Krok S, Samanta A, et al. Induced pluripotent stem cell (iPSC)-Derived extracellular vesicles are safer and more effective for cardiac repair than iPSCs. Circ Res. (2018) 122:296–309. doi: 10.1161/CIRCRESAHA.117.311769
141. Ciullo A, Biemmi V, Milano G, Bolis S, Cervio E, Fertig ET, et al. Exosomal expression of CXCR4 targets cardioprotective vesicles to myocardial infarction and improves outcome after systemic administration. Int J Mol Sci. (2019) 20:468. doi: 10.3390/ijms20030468
Keywords: coronary chronic total occlusion, percutaneous coronary intervention, cardiac regeneration, cardiac remodeling, angiogenesis, optimal medical therapy
Citation: Liao R, Li Z, Wang Q, Lin H and Sun H (2022) Revascularization of chronic total occlusion coronary artery and cardiac regeneration. Front. Cardiovasc. Med. 9:940808. doi: 10.3389/fcvm.2022.940808
Received: 10 May 2022; Accepted: 05 August 2022;
Published: 25 August 2022.
Edited by:
Yuling Zhang, Sun Yat-sen Memorial Hospital, ChinaReviewed by:
Xiao Liu, Sun Yat-sen University, ChinaXiangkun Xie, Sun Yat-sen Memorial Hospital, China
Copyright © 2022 Liao, Li, Wang, Lin and Sun. This is an open-access article distributed under the terms of the Creative Commons Attribution License (CC BY). The use, distribution or reproduction in other forums is permitted, provided the original author(s) and the copyright owner(s) are credited and that the original publication in this journal is cited, in accordance with accepted academic practice. No use, distribution or reproduction is permitted which does not comply with these terms.
*Correspondence: Hairuo Lin, aGFpYmFvQHNtdS5lZHUuY29t, NTc0MDg3MzI0QHFxLmNvbQ==; Huijun Sun, c3VuaHVpanVuQGRtdS5lZHUuY24=