- 1Department of Marine Biomedicine and Polar Medicine, Naval Medical Center, Naval Medical University (Second Military Medical University), Shanghai, China
- 2Key Laboratory of Medical Electrophysiology of Ministry of Education, Medical Electrophysiology Key Lab of Sichuan Province, Institute of Cardiovascular Research, Southwest Medical University, Luzhou, China
Cardiovascular disease is the leading cause of death globally among non-communicable diseases, which imposes a serious socioeconomic burden on patients and the healthcare system. Therefore, finding new strategies for preventing and treating cardiovascular diseases is of great significance in reducing the number of deaths and disabilities worldwide. Dipeptidyl peptidase 3 (DPP3) is the first zinc-dependent peptidase found among DPPs, mainly distributes within the cytoplasm. With the unique HEXXGH catalytic sequence, it is associated with the degradation of oligopeptides with 4 to 10 amino acids residues. Accumulating evidences have demonstrated that DPP3 plays a significant role in almost all cellular activities and pathophysiological mechanisms. Regarding the role of DPP3 in cardiovascular diseases, it is currently mainly used as a biomarker for poor prognosis in patients with cardiovascular diseases, suggesting that the level of DPP3 concentration in plasma is closely linked to the mortality of diseases such as cardiogenic shock and heart failure. Interestingly, it has been reported recently that DPP3 regulates blood pressure by interacting with the renin-angiotensin system. In addition, DPP3 also participates in the processes of pain signaling, inflammation, and oxidative stress. But the exact mechanism by which DPP3 affects cardiovascular function is not clear. Hence, this review summarizes the recent advances in the structure and catalytic activity of DPP3 and its extensive biological functions, especially its role as a therapeutic target in cardiovascular diseases. It will provide a theoretical basis for exploring the potential value of DPP3 as a therapeutic target for cardiovascular diseases.
Introduction
Cardiovascular disease is a major cause of disability and premature death worldwide, taking the lives of 17.9 million people in 2019, of which 81% occur in developing countries, and over one-third are premature deaths (1–3). Meanwhile, they impose a tremendous socioeconomic burden on patients and the healthcare system, especially in low-income and middle-income countries (4). Although health consciousness and medical practices have been improved gradually, the treatment and prognosis of cardiovascular diseases remain unsatisfactory (5). Therefore, finding new strategies for preventing and treating cardiovascular disease is of great significance in reducing the number of deaths and disabilities worldwide.
Dipeptidyl peptidases (DPPs) are a class of proteolytic enzyme family involved in nearly all aspects of cellular activities and physiological functions (6). They play a crucial role in a variety of physiological and pathological processes. There are eight distinct subtypes in this family, including DPP1 (7, 8), DPP2 (9, 10), DPP3 (11–13), DPP4 (14–16), DPP6 (17), DPP8 (18, 19), DPP9 (20, 21), and DPP10 (22, 23) (Table 1). DPPs are involved in a variety of physiological processes, including oligopeptide N-terminus processing, bio-active peptide degradation, cell cycle regulation, protein maturation, and viral infection (24–26). Contemporary studies have shown that DPPs inhibitors can be effective in treating several diseases, such as diabetes, tumors, and hematological diseases (27). Among them, DPP4 inhibitors, such as vildagliptin and sitagliptin, can reduce the level of blood glucose by enhancing the effects of the insulin-stimulating peptides glucagon-like peptide-1 (GLP-1) and glucose-dependent insulin-stimulating polypeptide (GIP). Furthermore, this class of drugs is used to treat type 2 diabetes and improve glycemic control (28). Similarly, a non-selective inhibitor of DPP, Val-boroPro (talabostat), can also treat prostate cancer by affecting fibroblast activation protein via reducing angiogenesis and inhibiting tumor proliferation and invasion (29). And the activity of DPP2 and the ratio of DPP2/DPP4 in serum may be diagnostic indicators for rheumatoid arthritis, systemic lupus erythematosus, cancer, Parkinson's disease, and other diseases (30, 31).
Dipeptidyl peptidase 3 (DPP3), one of the main members of the DPPs family, is highly conserved among animals. Its hydrolysis of 4–10 amino acid residues plays an important part in metabolism (32). The molecular weight of the purified DPP3 homologs is between 69 and 89 kDa (33), with the D. melanogaster DPP3 isoform (82–89 kDa) and cockroach DPP3 isoform (76–80 kDa) also found and verified (34). And DPP3 has multiple isoforms, including the classical DPP3 variant 1 (UniProtKB Q9NY33-1), variant 2 (UniProtKB Q9NY33-2), and variant 4 (UniProtKB Q9NY33-4). Variant 2 has no peptidase activity due to the lack of a catalytic sequence, while variant 4 lacks amino acids 91–120 from variant 1, but still has a catalytic function (35). Since the discovery of DPP3, its role in various physiological and pathological processes has attracted widespread attention from scientists. Studies have found that DPP3 can participate in protein turnover (32), oxidative stress (35, 36), pain (37), ovarian cancer tissue invasiveness (38), colorectal cancer progression (39), the maintenance of bone homeostasis (40) and inflammation (41, 42). Moreover, DPP3 is also closely related to high mortality in patients with sepsis (43, 44), cardiogenic shock (45), and acute kidney injury (46). Especially in cardiovascular diseases, DPP3 is regarded as a marker of more severe disease with higher activity of renin-angiotensin system (RAS) (47). However, there is still a lack of unified understanding of the role of DPP3 in diagnosing and treating cardiovascular diseases. Therefore, this review will summarize the biological characteristics of DPP3 and its research progresses in cardiovascular diseases, which aims to provide a theoretical basis for exploring potential value of DPP3 as a therapeutic target for cardiovascular diseases.
Biological properties
Distribution of DPP3
DPP3 is widely distributed among organisms. It was firstly identified in the bovine pituitary in 1967 (48). And this enzyme is the third to be found in the DPPs family, hence naming it DPP3. It can hydrolyze the terminal dipeptidyl amino residue from polypeptides containing at least four residues (49). According to a rat RNA-Seq transcriptomic results across 11 organs and four developmental stages (50, 51), DPP3 can highly expresses in the cardiovascular organs including heart and blood vessels. Moreover, accumulating studies have demonstrated that DPP3 exists in the other cardiovascular related organs, such as the adrenal gland, brain (52), and liver (53). Of course, the expression of DPP3 has also been reported in other organs that are not closely related to cardiovascular function, for instance, red blood cells (54), and cataractous lens (55). In addition, DPP3 has been found to be expressed not only in rats and humans, but also in yeast cells (56) and Drosophila (57). In 2000, Abramić et al. (58) found a high similarity and conservation of DPP3 between human erythrocytes and rat liver by mass spectrometry. Similar conclusions have been drawn in other studies (59–61).
DPPs are widely distributed among different tissues and were detected in the blood plasma (62), cerebrospinal fluid (26) and other body fluid (63). However, DPP3 is originally thought to be a cytoplasmic peptidase at the sub-cellular level, because it can be purified from the soluble fraction of most mammalian tissue homogenates (64). After that, DPP3 was extracted from the cell membrane of the Alzheimer's disease mouse model and detected by mass spectrometry, which confirmed that the peptidase is also distributed in mammalian cell membranes (65). Furthermore, DPP3 has also been found in extracellular fluids such as postplacental serum, human seminal, and cerebrospinal fluid, except in cells (66, 67). However, the mechanism of DPP3 targeting cell membranes or secretion into extracellular fluids is unclear. Recently, DPP3 activity was detected in human HK-2 cell culture medium (68), suggesting that intracellular DPP3 may be secreted or released into the extracellular fluids (69). Meanwhile, after cell death mediated by the anti-Fas receptor (CD95) antibody, DPP3 activity was significantly increased in the medium due to disrupting the cytoplasmic membrane (70). As cell death is a significant pathological change in a disease state, intracellular DPP3 can enter the circulation due to massive cell death (71). A recent clinical trial also found a close relationship between progressive cell death and the high level of DPP3 in plasma during the shock of various etiologies (72). It is precisely the wide distribution of DPP3 inside and outside cells that make it participating in various physiological and pathological processes, such as oxidative stress (73–75), RAS over-activation (45, 76), and inflammation (77, 78). Therefore, the above evidence fully indicates that DPP3 is widely distributed in multiple organisms and tissues and may have a broader range of biological functions.
Catalytic specificity of DPP3
Although DPP3 is widely distributed, its substrate and catalytic mode have strong specificity (79). DPP3 consists of two lobes separated by a wide clef, one is a α-helix-rich upper lobe, and the other is a lower lobe that mixes α-helices and β sheets (80, 81). Regardless of the fact that enzyme specificity exists between species, additional helical loops of amino acid residues are commonly observed in loops and the surface of both structures (82). In 1999, for the first time (83), it was verified that the DPP3 family (M49 family) has a unique HEXXGH conserved motif, and the catalytic motif (HEXXGH) and the secondary motif (EECRAR/D) are part of the upper lobe, while there are substrate binding sites between the upper and lower lobes. Two histidine residues on this motif contribute to the binding of divalent metal ions (mainly Zn2+, partly Mn2+, Co2+, Ni2+, and Cu2+). Among them, the Zn2+is located at the conserved binding site in the upper part of the two structures. And it is crucial for the catalytic activity of DPP3, where the glutamine acid provides the catalytic base with the histidine residue coordinating Zn2+ (84). In addition, DPP3 has a series of conserved arginine called “arginine anchors”, which are located at different positions from the catalytic Zn2+ (85). This allows substrates of different lengths to form a salt bridge between their C-terminus and the guanidine group of the positioned arginine anchor, thereby ensuring that the peptide bond cleaved by DPP3 is in the correct position, making DPP3-substrate binding easier (82). DPP3 has a wide range of active sites and flexible conformations, and the binding sites can be adjusted to different lengths and substrate binding, while 4–8 peptides are the most suitable hydrolysis substrates for DPP3. Among many polypeptides, angiotensin II (Ang II), endorphins and enkephalins can be efficiently cleaved by DPP3 (86).
DPP3 and enkephalin
Methionine-enkephalin (YGGFM) and leucine-enkephalin (YGGFL) are endogenous opioid neurotransmitters in the brain and spinal cord of many animals, including humans. And G. G. HADDAD and colleagues (87) found enkephalin analogs given intravenously or intra-arterially induce a biphasic response in MAP. While Li et al. (88) demonstrated that increased enkephalin in the rostral ventrolateral medulla after electro acupuncture decreases blood pressure. N terminal of both enkephalin are anchored to DPP3 via hydrogen bonding and electrostatic interactions of the tyrosine-318, glutamate-316, and asparagine-394 side chains and cleaved by DPP3 (89). Furthermore, leucine-enkephalin binds to inactive DPP3 isoform, but the difference in the C-terminal residue between the two structures of enkephalin is not significant. According to the results above, DPP3 may play a significant role in the regulation of cardiovascular function through its relationship with enkephalin.
DPP3 and endorphin 2
Endorphins are opioid peptides that play an essential role as neurotransmitters or neuromodulators in mammals (90), whose main functions include analgesia and endothelial cell-dependent vasodilation. Endorphins can be divided into two types according to their amino acid composition: Endorphin 1 (YPWF-NH2) and endorphin 2 (YPFF-NH2), both of which have amidated C-terminal (91). Kassab et al. (92) found endorphin is involved in the responses of blood pressure and heart rate to pain in sleep-deprived rats. In the sino-aortic denervated rat, the content of beta-endorphin and leu-enkephalin were decreased in hypothalamus and medulla oblongata (93). As DPP3 has now turned out to be a post-proline peptidase (38), substrates containing proline are more easily cleaved by peptidases. The binding mode of endorphin 2 to DPP3 was demonstrated, which mainly binds and interacts with the conserved residues aspartate-316, asparagine-391, and asparagine-394 of peptidase through the N-terminus with micromolar affinity (94). However, whether DPP3 is involved in the regulation of endorphins on cardiovascular function is still unclear.
DPP3 and synthetic morphorphins
Tyrosine (valine-valine-tyrosine-proline-tryptophan), a derivative of rotorphanin (95), has been shown to inhibit the activity of purified DPP3 in the brain of monkey (96). By synthesizing orphanoid pentapeptides containing aliphatic or aromatic amino acids at the N-terminus, such as VVYPW, LVYPW, IVYPW, YVYPW, FVYPW, and WVYPW, it was found that among these pentapeptides, IVYPW is a stronger inhibitor than casomorphin agent, and inhibits the activity of rat DPP3 with nanomolar affinity (42, 97).
DPP3 and RAS
Ang II is a potent vasoconstrictor of octapeptides, primarily involved in the humoral regulation of cardiovascular activity. In the treatment of hypertension, angiotensin-converting enzyme (ACE) inhibitors, Ang II type 1 receptor (AT1R) antagonists, and mineralocorticoid receptor antagonists are cornerstones in blocking RAS. Although Ang II is not an opioid peptide, DPP3 can cleave Ang II in vitro. Zhang et al. (98) demonstrated that Arg421-Lys423 of DPP3 could form an α-helix with the presence of Ang II. Thus, like other opioid peptides, the binding of Ang II to DPP3 is an endothermic process driven by entropy changes. Ang II forms a cis-peptide in a wheel-like conformation between histidine-6 and proline-7 during hydrolysis (99), meanwhile the binding site of DPP3 can bend to fully accommodate upon binding to Ang II and catalytic substrate. It was found that purified angiotensin-(1–7) [Ang-(1–7)] peptidase and DPP3 exhibited the same Ang-(1–7) hydrolysis profile, and both enzymatic activities were inhibited by the metallopeptidase inhibitor JMV-390 (100). DPP3 can sequentially hydrolyze Ang-(1–7) to Ang-(3–7) and rapidly convert Ang-(3–7) to Ang-(5–7) (101). At the same time, the kinetic analysis showed that the hydrolysis rate of Ang-(3–7) was higher than that of Ang-(1–7), and the Km value of Ang-(3–7) was lower than that of Ang-(1–7). Finally, it was found that chronic treatment of HK-2 cells with 20 nM of JMV-390 decreased intracellular DPP3 activity and increased cellular levels of Ang-(1–7) (69). Therefore, DPP3 can not only cleave Ang II but also participate in the hydrolysis of Ang-(1–7) and Ang-(3–7), thereby affecting the balance between Ang II and Ang-(1–7) in RAS.
General functions of DPP3
As DPP3 cleaves dipeptides sequentially from the N-terminus of various bioactive peptide substrates, it has a very wide range of biological functions. In 2001, Zhan et al. (32) have found DPP3 participates in the intracellular turnover of proteins. In the same year, DPP3 was found to be able to remove the N-terminal dipeptide from the myotropic neuropeptide proctolin in vitro (34). On the basis of DPP3's substrate specificity, recent research indicates that it plays a role in the regulation of blood pressure and pain (102). Since studies identified the DPP3 expression and activity in cells of the innate immune system, such as polymorphonuclear granulocytes and neutrophils, it has been reported that DPP3 involves in regulating the body's immune function (103). What's more, accumulating evidences have demonstrated that the high expression of DPP3 was associated with the pathogenesis of cancers such as multiple myeloma, colorectal cancer, and ER-positive breast cancer (12, 39, 104–106).
DPP3 and cardiovascular diseases
DPP3 and hypertension
Current studies have confirmed that abnormally increased Ang II can lead to elevated blood pressure by directly causing vasoconstriction, sympathetic hyperexcitation, and increased aldosterone release (107, 108). Additionally, the rostral ventrolateral medulla and paraventricular nucleus can also produce Ang II that stimulates the Ang II type 1 receptor (AT1R), thereby causing sympathetic excitation to increase blood pressure (109, 110). Not only in the brain, circulatory Ang II can enter the peri-ventricular organs such as subfornical organ and endplate vascular nodes to inhibit the baroreflex activity, resulting in increased vascular tone. And Ang II can also promote a wide range of tissue responses, such as apoptosis, inflammation, and fibrosis through activation of AT1R (111, 112). Therefore, angiotensin-converting enzyme inhibitors and AT1R antagonists, which target Ang II, are currently the first-line treatments for hypertension, especially in patients with hypertension complicated by diabetes or renal function failure (113, 114).
Based on the theory that DPP3, as a highly efficient hydrolase of angiotensin, can participate in the regulation of RAS (115), Xiaoling Pang and colleagues (11) in 2016 found that injecting DPP3 into Ang II-induced hypertensive mice through the tail vein significantly reduced blood pressure. A novel function of DPP3 and its potential therapeutic use in hypertension were revealed for the first time. However, Kumar et al. (86) found that DPP3 knockout mice showed no change in blood pressure using the tail artery cuff method in the same year. Although this study has some limitations due to the use of the tail artery cuff method to measure blood pressure (for example, the effect of stress on blood pressure in mice), the unchanged blood pressure also suggests that there may be other cardiovascular compensatory mechanisms after DPP3 knockout. Angiotensin II, III, and IV can be rapidly removed by exogenous intravenous injection of DPP3, and the dipeptide released by the substrate also has an inhibitory effect on ACE. Therefore, theoretically, DPP3 can lower levels of functional angiotensin, ultimately lowering blood pressure (33, 81). However, further studies are still required to verify whether DPP3 can be used as a biomarker of hypertension or participate in the occurrence and development of hypertension.
DPP3 and cardiogenic shock
Recent studies have reported that known biomarkers such as brain natriuretic peptide (BNP) (116), N-terminal pro-brain natriuretic peptide (NT-proBNP) (117), growth stimulation expressed gene 2 (ST2) (118), and troponin (119) are not of high value in the prediction of cardiogenic shock, while DPP3 as a recently discovered biomarker is attracting researchers' attention (77, 120). To elucidate the effects of circulating DPP3 on cardiac function and renal hemodynamics, Benjamin Deniau and colleagues (121) measured the circulatory level of DPP3 of 174 patients with acute heart failure and found that a high level of circulatory DPP3 was associated with short-term mortality risk and severe organ dysfunction. Additionally, a rapid decline in the level of DPP3 within 24 h after acute heart failure correlated with a better outcome. In 2020, Dépret et al. (122) also found that the concentration of DPP3 in plasma on admission was closely associated with an increased risk of death and circulatory collapse in severely burned patients. Later, in 2021, Boorsma et al. (47) measured the level of DPP3 in the serum samples of 2,156 patients with acute heart failure using luminescence immunoassay and found that the concentration of DPP3 was increased in patients with worsening heart failure, which may exacerbate acute heart failure. On the contrary, prilizumab, as a specific antibody against DPP3, has a certain potential therapeutic value in patients with acute heart failure. In 2022, Pavo et al. (45) found that the level of circulatory DPP3 was elevated only in patients with advanced heart failure with reduced ejection fraction (HFrEF), which could not only serve as a biomarker of cardiogenic shock, but also help identify end-stage patients with HFrEF. In animal experiments, Deniau et al. (121) also found that intravenous injection of DPP3 in healthy mice can lead to myocardial depression and impaired renal hemodynamics. In contrast, the level of oxidative stress and inflammation was rapidly reduced through injection of procilizumab, an inhibitor of DPP3, which significantly normalized cardiac function and renal hemodynamics in the mouse model of acute heart failure. The above studies show that circulatory DPP3 plays an important role in the diagnosis and staging of patients with cardiogenic shock caused by acute heart failure. However, the mechanism of the elevated level of DPP3 in pathological conditions and whether it is involved in the occurrence and development of cardiogenic shock is still unclear.
DPP3 and chronic heart failure
Unlike acute cardiogenic shock, the exogenous administration of DPP3 showed a protective effect on the development of myocardial fibrosis and chronic heart failure. Given that excess Ang II can damage organs such as the heart and kidneys, it was found that exogenous intravenous administration of DPP3 for 4 weeks significantly reduced the degree of Ang II-induced cardiac fibrosis and exerted a protective effect (11). At the same time, Komeno et al. (46) also found that the cardiac inflammatory cell infiltration and myocardial fibrosis levels were significantly reduced, and diastolic cardiac dysfunction was also improved after 8 weeks of intravenous recombinant DPP3 treatment in the type 2 diabetes model db/db mice, but it has no significant effects on blood glucose. The above results have shown that DPP3 can prevent the occurrence and development of chronic heart failure by inhibiting myocardial inflammation and fibrosis. Although the specific mechanism of DPP3 protection against chronic heart failure is still unclear, these results suggest that DPP3 may be a potential therapeutic target for cardiovascular diseases.
Possible mechanisms of DPP3 involved in cardiovascular diseases
Imbalance of RAS
RAS is a peptide hormone system composed of various components such as enzymes, inactive peptides, and active peptides, which play an essential role in regulating blood pressure and body fluid homeostasis. Traditionally, angiotensinogen produced in the liver is hydrolyzed by renin from paraglomerular cells to produce angiotensin I (10-peptide), which is then converted by ACE to the biologically active Ang II (8-peptide). Ang II is a highly efficient hydrolysis substrate of DPP3 and is also the principal effector peptide of RAS. It can virtually participate in the functional regulation of most organs, including the heart, kidney, and vascular system, and has crucial pathophysiological significance (123).
Angiotensin-converting enzyme 2 (ACE2) can directly catalyze the hydrolysis of Ang II to generate Ang-(1–7), but Ang-(1–7) has the opposite biological effect to Ang II (124). By binding to Mas receptors, Ang-(1–7) can promote vasodilation, anti-proliferation, and anti-hypertrophy (125). At the same time, Ang-(1–7) can also be cleaved by DPP3 to generate Ang-(3–7). Studies have shown that Ang-(3–7) can promote the release of dopamine and γ-aminobutyric acid in the striatum (126). It plays a vital role in regulating blood pressure in the rostral ventrolateral medulla (127), which suggests that DPP3 may play an important role in treating Parkinson's disease and hypertension, respectively. As research progressed, Blet et al. (43) found that a low level of DPP3 (median 15 ng/mL) was also present in the circulatory system of healthy individuals. And in the pathological process of sepsis-induced multiple organ failure, the higher the circulatory DPP3 on admission is associated with the more prolonged need for supportive therapy such as vasopressors and mechanical ventilation. Meanwhile, DPP3 inhibits the signal transduction of the Ang II-AT1 receptor complex by affecting intracellular G protein-coupled receptor-dependent Ca2+ in HEK293T cells (128). This laid the theoretical basis for DPP3 to affect RAS and the hemodynamics and development of cardiovascular diseases.
Oxidative stress
Besides the over-activation of RAS, oxidative stress may also be a critical mediator between DPP3 and cardiovascular diseases (129). Increased reactive oxygen species (ROS) can adversely affect cellular molecules, such as DNA, RNA, proteins, lipids, and carbohydrates, leading to cell damage and death (130). In contrast, the cellular antioxidant system [superoxide dismutase (SOD), peroxidase, and antioxidant vitamins] can maintain the balance of these two systems by preventing the accumulation of ROS (131). When there is an imbalance between ROS production and antioxidants, oxidative stress will disrupt redox signaling and further lead to endothelial damage, cardiovascular remodeling, renal dysfunction, sympathetic nervous system excitation, and immune cell activation (132, 133). Under normal physiological conditions, Nuclear factor-erythroid-2-related factor 2 (Nrf2) is a leucine zipper transcription factor located in the cytoplasm that binds to the inhibitor of Nrf2 [INrf2, or Kelch-like ECH-associated protein 1 (KEAP1)], which up-regulates a series of antioxidant enzymes. After oxidative damage or phosphorylation of Nrf2 at the serine 40 site by protein kinase C or phosphatidylinositol 3 kinase, Nrf2 is released from the complex with INrf2 and migrates to the nucleus. Afterward, nuclear Nrf2 up-regulates the expression of antioxidant enzymes by binding to the antioxidant response element (ARE) in its promoter (134). However, it is found that elevated ROS levels can promote DPP3 expression through the transcriptional regulator E26 avian erythroblastosis virus transcription factor-1, and elevated DPP3 can mediate the release and migration of Nrf2 to the nucleus, thereby up-regulating the expression of antioxidant enzymes (131). In 2017, Lu et al. (12) found an interaction between endogenous DPP3 and KEAP1, and hydrogen peroxide can strongly induce the DPP3-KEAP1 interaction. In comparison, DPP3 is required for Nrf2 induction and nuclear accumulation in estrogen receptor-positive MCF7 breast cancer cells (135). In addition, a high level of DPP3 mRNA is associated with an increase in expression of Nrf2 downstream genes and a poor prognosis for estrogen receptor-positive breast cancer. After that, Ren et al. (78) in 2021 also found that DPP3 can protect hippocampus neurons by modulating the neuronal KEAP1/Nrf2 signaling pathway, inhibiting apoptosis, oxidative stress, and inflammation in the pathological state of the cerebral ischemia/reperfusion injury.
Inflammation
Inflammation is a protective response of the body to injury or infection, but excessive long-term inflammation can lead to the development of cardiovascular diseases (136, 137). A large amount of evidence shows that long-term abnormal changes in inflammatory cells such as macrophages and immune molecules such as tumor necrosis factor-α (TNF-α) can promote the development of myocardial fibrosis and chronic heart failure. While excessive infiltration of inflammatory cells is associated with acute deterioration of cardiac function (138). In addition, a large number of clinical trials have shown that compared with normotensive patients, the levels of interleukin-6 (IL-6) (103), interleukin-1β (IL-1β) (139), and TNF-α (140, 141) in plasma were higher in hypertensive patients. Some studies have reported that DPP3 also has another function, which is involved in inflammation and immune responses. And DPP3 has been detected in the innate and acquired immune systems, such as granulocytes, monocytes, and lymphocytes (142). Moreover, deletion of DPP3 affects not only the production of proinflammatory cytokines, but also the anti-inflammatory cytokines (82, 143). Up-regulation of proinflammatory cytokines TNF-α, IL-1β, and IL-6 were also observed in DPP3-knockout myeloid cells and macrophages (16). These results suggest that DPP3 may be involved in the initiation and maintenance of immune responses, and it may significantly impact the regulation of immune function.
Summary
Globally, cardiovascular diseases are the leading cause of death. More than four-fifths of death in patients with cardiovascular diseases is caused by heart attack or stroke. Hypertension, as an essential risk factor for cardiovascular diseases, substantially increases the risk of cardiovascular and cerebrovascular diseases. Hence, identifying the risk of cardiovascular diseases and ensuring patients receive treatment early are the main strategies to prevent death in patients with cardiovascular diseases. Moreover, the foremost method is the comprehensive use of indicators, including patient history, imaging, and histology. Among them, the diagnostic evaluation of biomarkers in plasma and the targeted administration of cardiovascular drugs are particularly important for accurate diagnosis and prevention of heart attack and stroke. At present, the concentration and activity of DPP3 in circulation can accurately predict the severity of acute cardiogenic shock patients, which undoubtedly provides an important basis for the prevention and diagnosis of cardiovascular diseases. With the in-depth exploration of DPP3, a large amount of evidence shows that it is not only a biomarker of cardiovascular disease, but also participates in the occurrence and development of cardiovascular diseases through pathways such as RAS, oxidative stress, and inflammation. And DPP3 plays an essential role in the pathogenesis of cardiovascular diseases such as hypertension and heart failure (Figure 1), which will contribute to its transformation from biomarker to therapeutic target.
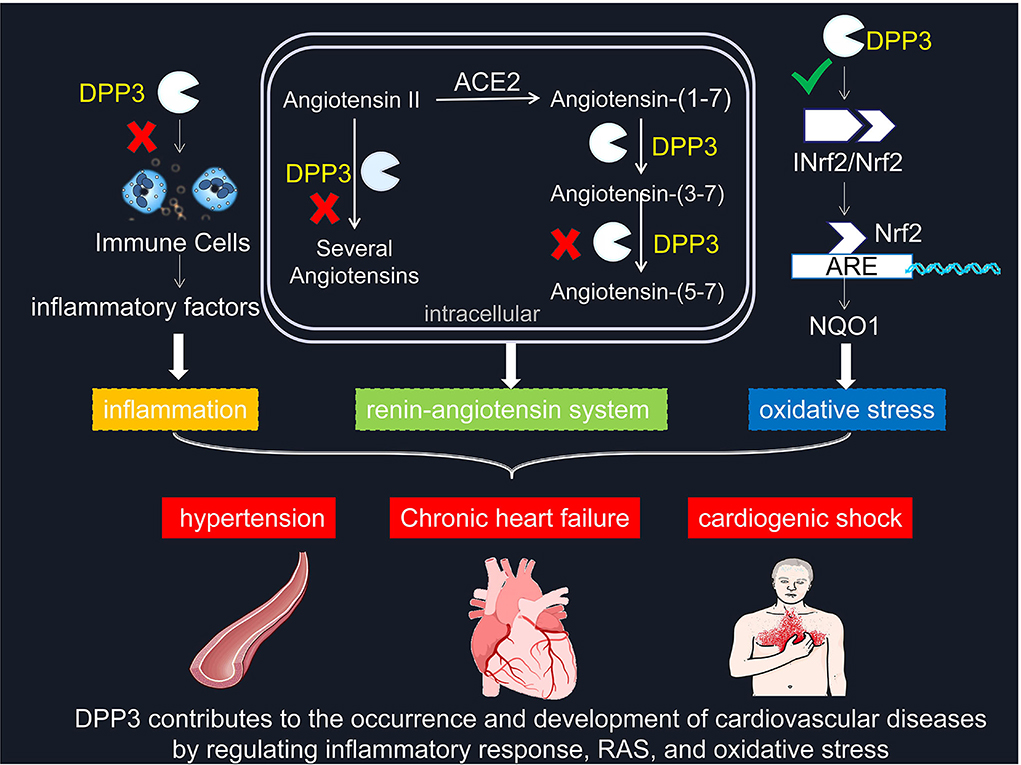
Figure 1. DPP3 contributes to the occurrence and development of cardiovascular diseases by regulating inflammatory response, RAS, and oxidative stress. × represents blocking this pathway. √ means promoting this mechanism. DPP3, Dipeptidyl peptidases 3; RAS, renin-angiotensin system; Nrf2, Nuclear factor-erythroid-2-related factor 2; INrf2, inhibitor of Nrf2; NQO1, quinone oxidoreductase 1.
Perspective
Although the mystery of DPP3 has been gradually unveiled, the results of animal experiments have shown that exogenous intravenous injection of DPP3 inhibitor procilizumab in cardiogenic shock improves cardiac function. In high-risk diseases such as hypertension and diabetes, DPP3 can prevent the occurrence and development of chronic heart failure by inhibiting myocardial inflammation and myocardial fibrosis. The precise mechanism remains elusive for the difference in the role of DPP3 in chronic cardiac failure and cardiogenic shock. At the same time, the level of circulatory DPP3 has been helpful in the diagnosis of diseases such as cardiogenic shock in clinical studies, but whether it can be further used to identify patients sensitive to hemodynamic treatment strategies remains to be explored. Therefore, it is still of great significance to clarify the efficacy of DPP3 as a biomarker and to explore its potential therapeutic value in patients with cardiovascular disease for the diagnosis and prevention of cardiovascular disease.
Author contributions
PY and WD designed the manuscript. XT and W-ZW edited the manuscript. Y-QL and Y-KW revised the manuscript. All authors contributed to manuscript revision, read, and approved the submitted version.
Funding
This work was supported by the Natural Science Foundation of Shanghai (No. 22ZR1478400) and the National Natural Science Foundation of China (Nos. 81970354, 81630012, and 81800366).
Conflict of interest
The authors declare that the research was conducted in the absence of any commercial or financial relationships that could be construed as a potential conflict of interest.
Publisher's note
All claims expressed in this article are solely those of the authors and do not necessarily represent those of their affiliated organizations, or those of the publisher, the editors and the reviewers. Any product that may be evaluated in this article, or claim that may be made by its manufacturer, is not guaranteed or endorsed by the publisher.
References
1. Tsao CW, Aday AW, Almarzooq ZI, Alonso A, Beaton AZ, Bittencourt MS, et al. Heart disease and stroke statistics-2022 update: a report from the American Heart Association. Circulation. (2022) 145:e153–639. doi: 10.1161/CIR.0000000000001052
2. WHO. Who List of Priority Medical Devices for Management of Cardiovascular Diseases and Diabetes. Licence:CCBY-NC-SA 30 IGO. Geneva (2021).
3. Liu W, Leong DP, Hu B, AhTse L, Rangarajan S, Wang Y, et al. The association of grip strength with cardiovascular diseases and all-cause mortality in people with hypertension: findings from the prospective urban rural epidemiology China study. J Sport Health Sci. (2021) 10:629–36. doi: 10.1016/j.jshs.2020.10.005
4. Wang W, Zhang M, Xu CD, Ye PP, Liu YN, Huang ZJ, et al. Hypertension prevalence, awareness, treatment, and control and their associated socioeconomic factors in China: a spatial analysis of a national representative survey. Biomed Environ Sci. (2021) 34:937–51. doi: 10.3967/bes2021.130
5. Yuyun MF, Sliwa K, Kengne AP, Mocumbi AO, Bukhman G. Cardiovascular diseases in Sub-Saharan Africa compared to high-income countries: an epidemiological perspective. Glob Heart. (2020) 15:15. doi: 10.5334/gh.403
6. Sato A, Ogita H. Pathophysiological implications of dipeptidyl peptidases. Curr Protein Pept Sci. (2017) 18:843–9. doi: 10.2174/1389203718666170329104936
7. Roustaei H, Kiamanesh Z, Askari E, Sadeghi R, Aryana K, Treglia G. Could fibroblast activation protein (fap)-specific radioligands be considered as pan-tumor agents? Contrast Media Mol Imaging. (2022) 2022:3948873. doi: 10.1155/2022/3948873
8. Kuyumcu S, Sanli Y, Subramaniam RM. Fibroblast-activated protein inhibitor Pet/Ct: cancer diagnosis and management. Front Oncol. (2021) 11:758958. doi: 10.3389/fonc.2021.758958
9. Mentlein R, Struckhoff G. Purification of two dipeptidyl aminopeptidases II from rat brain and their action on proline-containing neuropeptides. J Neurochem. (1989) 52:1284–93. doi: 10.1111/j.1471-4159.1989.tb01877.x
10. Lynn KR. The isolation and some properties of dipeptidyl peptidases II and III from porcine spleen. Int J Biochem. (1991) 23:47–50. doi: 10.1016/0020-711X(91)90007-A
11. Pang X, Shimizu A, Kurita S, Zankov DP, Takeuchi K, Yasuda-Yamahara M, et al. Novel therapeutic role for dipeptidyl peptidase III in the treatment of hypertension. Hypertension. (2016) 68:630–41. doi: 10.1161/HYPERTENSIONAHA.116.07357
12. Lu K, Alcivar AL, Ma J, Foo TK, Zywea S, Mahdi A, et al. Nrf2 induction supporting breast cancer cell survival is enabled by oxidative stress-induced Dpp3-Keap1 interaction. Cancer Res. (2017) 77:2881–92. doi: 10.1158/0008-5472.CAN-16-2204
13. Ballou SP, Lozanski G. Induction of inflammatory cytokine release from cultured human monocytes by C-reactive protein. Cytokine. (1992) 4:361–8. doi: 10.1016/1043-4666(92)90079-7
14. Mentlein R, Gallwitz B, Schmidt WE. Dipeptidyl-peptidase Iv hydrolyses gastric inhibitory polypeptide, glucagon-like peptide-1(7-36)amide, peptide histidine methionine and is responsible for their degradation in human serum. Eur J Biochem. (1993) 214:829–35. doi: 10.1111/j.1432-1033.1993.tb17986.x
15. Augstein P, Berg S, Heinke P, Altmann S, Salzsieder E, Demuth HU, et al. Efficacy of the dipeptidyl peptidase IV inhibitor isoleucine thiazolidide (P32/98) in fatty zucker rats with incipient and manifest impaired glucose tolerance. Diabetes Obes Metab. (2008) 10:850–61. doi: 10.1111/j.1463-1326.2007.00813.x
16. Pederson RA, White HA, Schlenzig D, Pauly RP, McIntosh CH, Demuth HU. Improved glucose tolerance in Zucker fatty rats by oral administration of the dipeptidyl peptidase IV inhibitor isoleucine thiazolidide. Diabetes. (1998) 47:1253–8. doi: 10.2337/diab.47.8.1253
17. Demine S, Garcia Ribeiro R, Thevenet J, Marselli L, Marchetti P, Pattou F, et al. A nanobody-based nuclear imaging tracer targeting dipeptidyl peptidase 6 to determine the mass of human beta cell grafts in mice. Diabetologia. (2020) 63:825–36. doi: 10.1007/s00125-019-05068-5
18. Liu HF, Xie YK, Zhong BY, Zhang JH, Song CX, Liu YW, et al. Dipeptidyl peptidase-8 induces sorafenib resistance via binding with C-Rel to mediate Nf-?b signaling in hepatocellular carcinoma. Cell Biol Int. (2022) 46:213–21. doi: 10.1002/cbin.11719
19. Sato T, Tatekoshi A, Takada K, Iyama S, Kamihara Y, Jawaid P, et al. Dpp8 is a novel therapeutic target for multiple myeloma. Sci Rep. (2019) 9:18094. doi: 10.1038/s41598-019-54695-w
20. Sharif H, Hollingsworth LR, Griswold AR, Hsiao JC, Wang Q, Bachovchin DA, et al. Dipeptidyl peptidase 9 sets a threshold for Card8 inflammasome formation by sequestering its active C-terminal fragment. Immunity. (2021) 54:1392–404.e10. doi: 10.1016/j.immuni.2021.04.024
21. Wu QQ, Zhao M, Huang GZ, Zheng ZN, Chen Y, Zeng WS, et al. Fibroblast activation protein (Fap) overexpression induces epithelial-mesenchymal transition (Emt) in oral squamous cell carcinoma by down-regulating dipeptidyl peptidase 9 (Dpp9). Onco Targets Ther. (2020) 13:2599–611. doi: 10.2147/OTT.S243417
22. Ni H, Rajamani S, Giles WR. Novel regulation of the mammalian cardiac Na(+) channel by dipeptidyl peptidase 10 interactions: an editorial comment. Int J Cardiol. (2019) 284:74–6. doi: 10.1016/j.ijcard.2019.02.011
23. Belau F, Metzner K, Christ T, Ravens U, Schaefer M, Künzel S, et al. Dpp10 Is a new regulator of Nav15 channels in human heart. Int J Cardiol. (2019) 284:68–73. doi: 10.1016/j.ijcard.2018.12.072
24. Prajapati SC, Chauhan SS. Dipeptidyl peptidase III: a multifaceted oligopeptide n-End cutter. FEBS J. (2011) 278:3256–76. doi: 10.1111/j.1742-4658.2011.08275.x
25. Korkmaz B, Lesner A, Letast S, Mahdi YK, Jourdan ML, Dallet-Choisy S, et al. Neutrophil proteinase 3 and dipeptidyl peptidase I (Cathepsin C) as pharmacological targets in granulomatosis with polyangiitis (Wegener Granulomatosis). Semin Immunopathol. (2013) 35:411–21. doi: 10.1007/s00281-013-0362-z
26. Nagatsu T. Prolyl oligopeptidase and dipeptidyl peptidase II/dipeptidyl peptidase IV ratio in the cerebrospinal fluid in Parkinson's disease: historical overview and future prospects. J Neural Transm. (2017) 124:739–44. doi: 10.1007/s00702-016-1604-8
27. Deacon CF. Dipeptidyl peptidase 4 inhibitors in the treatment of type 2 diabetes mellitus. Nat Rev Endocrinol. (2020) 16:642–53. doi: 10.1038/s41574-020-0399-8
28. Chen CC, Chen RF, Wang YC Li YT, Chuang JH, Kuo YR. Combination of a Cd26 inhibitor, G-Csf, and short-term immunosuppressants modulates allotransplant survival and immunoregulation in a rodent hindlimb allotransplant model. Transplantation. (2021) 105:1250–60. doi: 10.1097/TP.0000000000003504
29. Fujita K, Hagihara M, Nagatsu T, Iwata H, Miura T. The activity of dipeptidyl peptidase II and dipeptidyl peptidase IV in mice immunized with type II collagen. Biochem Med Metab Biol. (1992) 48:227–34. doi: 10.1016/0885-4505(92)90069-B
30. Hagihara M, Sekiguchi K, Fujiwara M, Aoyagi T, Takeuchi T, Nagatsu T. Effects of deoxyspergualin on dipeptidyl peptidase-II and -IV in the spleen of Bxsb Mice and Mrl/Lpr mice during the development of the lupus erythematosus-like syndrome. Biochem Pharmacol. (1992) 43:1380–3. doi: 10.1016/0006-2952(92)90517-M
31. Sohar N, Sohar I, Hammer H. Lysosomal enzyme activities: new potential markers for Sjögren's syndrome. Clin Biochem. (2005) 38:1120–6. doi: 10.1016/j.clinbiochem.2005.09.003
32. Zhan H, Yamamoto Y, Shumiya S, Kunimatsu M, Nishi K, Ohkubo I, et al. Peptidases play an important role in cataractogenesis: an immunohistochemical study on lenses derived from shumiya cataract rats. Histochem J. (2001) 33:511–21. doi: 10.1023/A:1014943522613
33. Dhanda S, Singh H, Singh J, Singh TP. Isolation, purification and characterization of a Dpp-III homologue from goat brain. Protein Expr Purif. (2007) 52:297–305. doi: 10.1016/j.pep.2006.10.004
34. Mazzocco C, Fukasawa KM, Raymond AA, Puiroux J. Purification, partial sequencing and characterization of an insect membrane dipeptidyl aminopeptidase that degrades the insect neuropeptide proctolin. Eur J Biochem. (2001) 268:4940–9. doi: 10.1046/j.1432-1327.2001.02425.x
35. Mazzocco C, Gillibert-Duplantier J, Neaud V, Fukasawa KM, Claverol S, Bonneu M, et al. Identification and characterization of two dipeptidyl-peptidase III isoforms in drosophila melanogaster. FEBS J. (2006) 273:1056–64. doi: 10.1111/j.1742-4658.2006.05132.x
36. Jaramillo MC, Zhang DD. The emerging role of the Nrf2-Keap1 signaling pathway in cancer. Genes Dev. (2013) 27:2179–91. doi: 10.1101/gad.225680.113
37. Chiba T, Li YH, Yamane T, Ogikubo O, Fukuoka M, Arai R, et al. Inhibition of recombinant dipeptidyl peptidase III by synthetic hemorphin-like peptides. Peptides. (2003) 24:773–8. doi: 10.1016/S0196-9781(03)00119-0
38. Barsun M, Jajcanin N, Vukelić B, Spoljarić J, Abramić M. Human dipeptidyl peptidase III acts as a post-proline-cleaving enzyme on endomorphins. Biol Chem. (2007) 388:343–8. doi: 10.1515/BC.2007.039
39. Tong Y, Huang Y, Zhang Y, Zeng X, Yan M, Xia Z, et al. Dpp3/Cdk1 contributes to the progression of colorectal cancer through regulating cell proliferation, cell apoptosis, and cell migration. Cell Death Dis. (2021) 12:529. doi: 10.1038/s41419-021-03796-4
40. Menale C, Robinson LJ, Palagano E, Rigoni R, Erreni M, Almarza AJ, et al. Absence of dipeptidyl peptidase 3 increases oxidative stress and causes bone loss. J Bone Miner Res. (2019) 34:2133–48. doi: 10.1002/jbmr.3829
41. Simaga S, Babić D, Osmak M, Sprem M, Abramić M. Tumor cytosol dipeptidyl peptidase III activity is increased with histological aggressiveness of ovarian primary carcinomas. Gynecol Oncol. (2003) 91:194–200. doi: 10.1016/S0090-8258(03)00462-1
42. Simaga S, Babić D, Osmak M, Ilić-Forko J, Vitale L, Milicić D, et al. Dipeptidyl peptidase III in malignant and non-malignant gynaecological tissue. Eur J Cancer. (1998) 34:399–405. doi: 10.1016/S0959-8049(97)00401-2
43. Blet A, Deniau B, Santos K, van Lier DPT, Azibani F, Wittebole X, et al. Monitoring circulating dipeptidyl peptidase 3 (Dpp3) predicts improvement of organ failure and survival in sepsis: a prospective observational multinational study. Crit Care. (2021) 25:61. doi: 10.1186/s13054-021-03471-2
44. Frigyesi A, Lengquist M, Spångfors M, Annborn M, Cronberg T, Nielsen N, et al. Circulating dipeptidyl peptidase 3 on intensive care unit admission is a predictor of organ dysfunction and mortality. J Intens Care. (2021) 9:52. doi: 10.1186/s40560-021-00561-9
45. Pavo N, Prausmüller S, Spinka G, Goliasch G, Bartko PE, Arfsten H, et al. Circulating dipeptidyl peptidase (Cdpp3)-a marker for end-stage heart failure? J Intern Med. (2022) 291:886–90. doi: 10.1111/joim.13449
46. Komeno M, Pang X, Shimizu A, Molla MR, Yasuda-Yamahara M, Kume S, et al. Cardio- and reno-protective effects of dipeptidyl peptidase III in diabetic mice. J Biol Chem. (2021) 296:100761. doi: 10.1016/j.jbc.2021.100761
47. Boorsma EM, Ter Maaten JM, Damman K, van Veldhuisen DJ, Dickstein K, Anker SD, et al. Dipeptidyl peptidase 3, a marker of the antagonist pathway of the renin-angiotensin-aldosterone system in patients with heart failure. Eur J Heart Fail. (2021) 23:947–53. doi: 10.1002/ejhf.2158
48. Ellis S, Nuenke JM. Dipeptidyl arylamidase III of the pituitary. Purification and characterization. J Biol Chem. (1967) 242:4623–9. doi: 10.1016/S0021-9258(18)99503-7
49. Hashimoto J, Yamamoto Y, Kurosawa H, Nishimura K, Hazato T. Identification of dipeptidyl peptidase III in human neutrophils. Biochem Biophys Res Commun. (2000) 273:393–7. doi: 10.1006/bbrc.2000.2827
50. Yu Y, Fuscoe JC, Zhao C, Guo C, Jia M, Qing T, et al. A rat Rna-Seq transcriptomic bodymap across 11 organs and 4 developmental stages. Nat Commun. (2014) 5:3230. doi: 10.1038/ncomms4230
51. Yu Y, Zhao C, Su Z, Wang C, Fuscoe JC, Tong W, et al. Comprehensive Rna-Seq transcriptomic profiling across 11 organs, 4 ages, and 2 sexes of Fischer 344 rats. Sci Data. (2014) 1:140013. doi: 10.1038/sdata.2014.13
52. Lee CM, Snyder SH. Dipeptidyl-aminopeptidase III of rat brain. Selective affinity for enkephalin and angiotensin. J Biol Chem. (1982) 257:12043–50. doi: 10.1016/S0021-9258(18)33674-3
53. Fukasawa K, Fukasawa KM, Iwamoto H, Hirose J, Harada M. The Hellgh motif of rat liver dipeptidyl peptidase III is involved in zinc coordination and the catalytic activity of the enzyme. Biochemistry. (1999) 38:8299–303. doi: 10.1021/bi9904959
54. Abramić M, Zubanović M, Vitale L. Dipeptidyl peptidase III from human erythrocytes. Biol Chem Hoppe Seyler. (1988) 369:29–38. doi: 10.1515/bchm3.1988.369.1.29
55. Swanson AA, Davis RM, McDonald JK. Dipeptidyl peptidase III of human cataractous lenses. Part Purif Curr Eye Res. (1984) 3:287–91. doi: 10.3109/02713688408997211
56. Watanabe Y, Kumagai Y, Fujimoto Y. Presence of a dipeptidyl aminopeptidase III in saccharomyces cerevisiae. Chem Pharm Bull. (1990) 38:246–8. doi: 10.1248/cpb.38.246
57. Mazzocco C, Fukasawa KM, Auguste P, Puiroux J. Characterization of a functionally expressed dipeptidyl aminopeptidase III from drosophila melanogaster. Eur J Biochem. (2003) 270:3074–82. doi: 10.1046/j.1432-1033.2003.03689.x
58. Abramić M, Schleuder D, Dolovcak L, Schröder W, Strupat K, Sagi D, et al. Human and rat dipeptidyl peptidase III: biochemical and mass spectrometric arguments for similarities and differences. Biol Chem. (2000) 381:1233–43. doi: 10.1515/BC.2000.151
59. Jajcanin-Jozic N, Abramic M. Hydrolysis of dipeptide derivatives reveals the diversity in the M49 family. Biol Chem. (2013) 394:767–71. doi: 10.1515/hsz-2012-0347
60. Hirose J, Hata T, Kawaoka C, Ikeura T, Kitahara S, Horii K, et al. Flexibility of the coordination geometry around the cupric ions in Cu(Ii)-rat dipeptidyl peptidase III is important for the expression of enzyme activity. Arch Biochem Biophys. (2012) 525:71–81. doi: 10.1016/j.abb.2012.05.018
61. Gamrekelashvili J, Kapanadze T, Han M, Wissing J, Ma C, Jaensch L, et al. Peptidases released by necrotic cells control Cd8+ T cell cross-priming. J Clin Invest. (2013) 123:4755–68. doi: 10.1172/JCI65698
62. Kamori M, Hagihara M, Nagatsu T, Iwata H, Miura T. Activities of dipeptidyl peptidase II, dipeptidyl peptidase IV, prolyl endopeptidase, and collagenase-like peptidase in synovial membrane from patients with rheumatoid arthritis and osteoarthritis. Biochem Med Metab Biol. (1991) 45:154–60. doi: 10.1016/0885-4505(91)90016-E
63. Rao AJ, Hagihara M, Nagatsu T, Yanagita N. Presence of dipeptidyl peptidase II, dipeptidyl peptidase IV, and prolyl endopeptidase in effusion from patients with serous otitis media. Biochem Med Metab Biol. (1990) 43:276–82. doi: 10.1016/0885-4505(90)90035-Y
64. Shimamori Y, Watanabe Y, Fujimoto Y. Purification and characterization of dipeptidyl aminopeptidase III from human placenta. Chem Pharm Bull. (1986) 34:3333–40. doi: 10.1248/cpb.34.3333
65. Chadwick W, Brenneman R, Martin B, Maudsley S. Complex and multidimensional lipid raft alterations in a murine model of Alzheimer's disease. Int J Alzheimers Dis. (2010) 2010:604792. doi: 10.4061/2010/604792
66. Sato H, Kimura K, Yamamoto Y, Hazato T. Activity of Dpp III in human cerebrospinal fluid derived from patients with pain. Masui. (2003) 52:257–63.
67. Kaufmann P, Muenzner M, Kästorf M, Santos K, Hartmann T, Dienelt A, et al. A novel and highly efficient purification procedure for native human dipeptidyl peptidase 3 from human blood cell lysate. PLoS ONE. (2019) 14:e0220866. doi: 10.1371/journal.pone.0220866
68. Wilson BA, Cruz-Diaz N, Marshall AC, Pirro NT, Su Y, Gwathmey TM, et al. An angiotensin-(1-7) peptidase in the kidney cortex, proximal tubules, and human Hk-2 epithelial cells that is distinct from insulin-degrading enzyme. Am J Physiol Renal Physiol. (2015) 308:F594–601. doi: 10.1152/ajprenal.00609.2014
69. Cruz-Diaz N, Wilson BA, Pirro NT, Brosnihan KB, Marshall AC, Chappell MC. Identification of dipeptidyl peptidase 3 as the angiotensin-(1-7) degrading peptidase in human Hk-2 renal epithelial cells. Peptides. (2016) 83:29–37. doi: 10.1016/j.peptides.2016.06.005
70. Wattiaux R. Wattiaux-de Coninck S, Thirion J, Gasingirwa MC, Jadot M. Lysosomes and Fas-mediated liver cell death. Biochem J. (2007) 403:89–95. doi: 10.1042/BJ20061738
71. Wang F, Gómez-Sintes R, Boya P. Lysosomal membrane permeabilization and cell death. Traffic. (2018) 19:918–31. doi: 10.1111/tra.12613
72. van Lier D, Kox M, Pickkers P. Promotion of vascular integrity in sepsis through modulation of bioactive adrenomedullin and dipeptidyl peptidase 3. J Intern Med. (2021) 289:792–806. doi: 10.1111/joim.13220
73. Malovan G, Hierzberger B, Suraci S, Schaefer M, Santos K, Jha S, et al. The emerging role of dipeptidyl peptidase 3 in pathophysiology. FEBS J. (2022) 10:125–9. doi: 10.1111/febs.16429
74. Deniau B, Blet A, Santos K, Vaittinada Ayar P, Genest M, Kastorf M, et al. Inhibition of circulating dipeptidyl-peptidase 3 restores cardiac function in a sepsis-induced model in rats: a proof of concept study. PLoS ONE. (2020) 15:e0238039. doi: 10.1371/journal.pone.0238039
75. Menale C, Tabacco G, Naciu AM, Schiavone ML, Cannata F, Morenghi E, et al. Dipeptidyl peptidase 3 activity as a promising biomarker of bone fragility in postmenopausal women. Molecules. (2022) 27:3929–40. doi: 10.3390/molecules27123929
76. Haznedaroglu IC, Malkan UY. Local Bone Marrow renin-angiotensin system in the genesis of leukemia and other malignancies. Eur Rev Med Pharmacol Sci. (2016) 20:4089–111. Available online at: https://www.europeanreview.org/article/11533
77. Iborra-Egea O, Montero S, Bayes-Genis A. An outlook on biomarkers in cardiogenic shock. Curr Opin Crit Care. (2020) 26:392–7. doi: 10.1097/MCC.0000000000000739vspace*2pt
78. Ren X, Yu J, Guo L, Ma H. Dipeptidyl-peptidase 3 protects oxygen-glucose deprivation/reoxygenation-injured hippocampal neurons by suppressing apoptosis, oxidative stress and inflammation via modulation of Keap1/Nrf2 signaling. Int Immunopharmacol. (2021) 96:107595. doi: 10.1016/j.intimp.2021.107595
79. Sabljic I, Tomin M, Matovina M, Sucec I, Tomasic Paic A, Tomic A, et al. The first dipeptidyl peptidase III from a thermophile: structural basis for thermal stability and reduced activity. PLoS ONE. (2018) 13:e0192488. doi: 10.1371/journal.pone.0192488
80. Sabljic I, Mestrovic N, Vukelic B, Macheroux P, Gruber K, Luic M, et al. Crystal structure of dipeptidyl peptidase III from the human gut symbiont bacteroides thetaiotaomicron. PLoS ONE. (2017) 12:e0187295. doi: 10.1371/journal.pone.0187295vspace*2pt
81. Agic D, Brkic H, Kazazic S, Tomic A, Abramic M. Aprotinin interacts with substrate-binding site of human dipeptidyl peptidase III. J Biomol Struct Dyn. (2019) 37:3596–606. doi: 10.1080/07391102.2018.1521343
82. Baral PK, Jajcanin-Jozic N, Deller S, Macheroux P, Abramic M, Gruber K. The first structure of dipeptidyl-peptidase III provides insight into the catalytic mechanism and mode of substrate binding. J Biol Chem. (2008) 283:22316–24. doi: 10.1074/jbc.M803522200
83. Lewis AP, Thomas PJ. A novel clan of zinc metallopeptidases with possible intramembrane cleavage properties. Protein Sci. (1999) 8:439–42. doi: 10.1110/ps.8.2.439
84. Tomic A, Kovacevic B, Tomic S. Concerted nitrogen inversion and hydrogen bonding to Glu451 are responsible for protein-controlled suppression of the reverse reaction in human Dpp Iii. Phys Chem Chem Phys. (2016) 18:27245–56. doi: 10.1039/C6CP04580D
85. Abramic M, Karacic Z, Semanjski M, Vukelic B, Jajcanin-Jozic N. Aspartate 496 from the subsite S2 drives specificity of human dipeptidyl peptidase III. Biol Chem. (2015) 396:359–66. doi: 10.1515/hsz-2014-0247
86. Kumar P, Reithofer V, Reisinger M, Wallner S, Pavkov-Keller T, Macheroux P, et al. Substrate complexes of human dipeptidyl peptidase III reveal the mechanism of enzyme inhibition. Sci Rep. (2016) 6:23787. doi: 10.1038/srep23787
87. Haddad GG, Jeng HJ, Lai TL. Effect of endorphins on heart rate and blood pressure in adult dogs. Am J Physiol. (1986) 250:H796–805. doi: 10.1152/ajpheart.1986.250.5.H796
88. Li M, Tjen ALSC, Guo ZL, Longhurst JC. Repetitive electroacupuncture attenuates cold-induced hypertension through enkephalin in the rostral ventral lateral medulla. Sci Rep. (2016) 6:35791. doi: 10.1038/srep35791
89. Ivkovic J, Jha S, Lembacher-Fadum C, Puschnig J, Kumar P, Reithofer V, et al. Efficient entropy-driven inhibition of dipeptidyl peptidase III by hydroxyethylene transition-state peptidomimetics. Chemistry. (2021) 27:14108–20. doi: 10.1002/chem.202102204
90. Zadina JE. Isolation and distribution of endomorphins in the central nervous system. Jpn J Pharmacol. (2002) 89:203–8. doi: 10.1254/jjp.89.203
91. Horvath G. Endomorphin-1 and endomorphin-2: pharmacology of the selective endogenous mu-opioid receptor agonists. Pharmacol Ther. (2000) 88:437–63. doi: 10.1016/S0163-7258(00)00100-5
92. Kassab S, Sachdeva U, Das N, Al-Shaibani T, Nayar U. Cardiovascular responses to tonic pain in rem sleep-deprived rats: role of melatonin and beta endorphin. Sultan Qaboos Univ Med J. (2006) 6:51–6.
93. Shan ZZ Dai SM, Fang F, Su DF. Changes of central norepinephrine, beta-endorphin, leu-enkephalin, peripheral arginine-vasopressin, and angiotensin II levels in acute and chronic phases of sino-aortic denervation in rats. J Cardiovasc Pharmacol. (2004) 43:234–41. doi: 10.1097/00005344-200402000-00011
94. Bezerra GA, Dobrovetsky E, Viertlmayr R, Dong A, Binter A, Abramic M, et al. Entropy-driven binding of opioid peptides induces a large domain motion in human dipeptidyl peptidase III. Proc Natl Acad Sci USA. (2012) 109:6525–30. doi: 10.1073/pnas.1118005109
95. Nishimura K, Hazato T. Isolation and identification of an endogenous inhibitor of enkephalin-degrading enzymes from bovine spinal cord. Biochem Biophys Res Commun. (1993) 194:713–9. doi: 10.1006/bbrc.1993.1880
96. Yamamoto Y, Hashimoto J, Shimamura M, Yamaguchi T, Hazato T. Characterization of tynorphin, a potent endogenous inhibitor of dipeptidyl peptidaseiii. Peptides. (2000) 21:503–8. doi: 10.1016/S0196-9781(00)00174-1
97. Tomic A, Tomic S. Demystifying Dpp III catalyzed peptide hydrolysis-computational study of the complete catalytic cycle of human Dpp III catalyzed tynorphin hydrolysis. Int J Mol Sci. (2022) 23:1858–65. doi: 10.3390/ijms23031858
98. Zhang S, Lv S, Fu X, Han L, Han W, Li W. Molecular dynamics simulations study of the interactions between human dipeptidyl-peptidase III and two substrates. Molecules. (2021) 26:6492–9. doi: 10.3390/molecules26216492
99. Tzakos AG, Bonvin AM, Troganis A, Cordopatis P, Amzel ML, Gerothanassis IP, et al. On the molecular basis of the recognition of angiotensin II (AII). NMR structure of AII in solution compared with the X-ray structure of AII bound to the Mab Fab131. Eur J Biochem. (2003) 270:849–60. doi: 10.1046/j.1432-1033.2003.03441.x
100. Abramić M, Agić D. Survey of dipeptidyl peptidase III inhibitors: from small molecules of microbial or synthetic origin to aprotinin. Molecules. (2022) 27:3006–12. doi: 10.3390/molecules27093006
101. Jha S, Taschler U, Domenig O, Poglitsch M, Bourgeois B, Pollheimer M, et al. Dipeptidyl peptidase 3 modulates the renin-angiotensin system in mice. J Biol Chem. (2020) 295:13711–23. doi: 10.1074/jbc.RA120.014183
102. Rehfeld L, Funk E, Jha S, Macheroux P, Melander O, Bergmann A. Novel methods for the quantification of dipeptidyl peptidase 3 (Dpp3) concentration and activity in human blood samples. J Appl Lab Med. (2019) 3:943–53. doi: 10.1373/jalm.2018.027995
103. Grdisa M, Vitale L. Types and localization of aminopeptidases in different human blood cells. Int J Biochem. (1991) 23:339–45. doi: 10.1016/0020-711X(91)90116-5
104. Choy TK, Wang CY, Phan NN, Khoa Ta HD, Anuraga G, Liu YH, et al. Identification of dipeptidyl peptidase (Dpp) family genes in clinical breast cancer patients via an integrated bioinformatics approach. Diagnostics. (2021) 11:1204–10. doi: 10.3390/diagnostics11071204
105. Li Y, Wang X, Vural S, Mishra NK, Cowan KH, Guda C. Exome analysis reveals differentially mutated gene signatures of stage, grade and subtype in breast cancers. PLoS ONE. (2015) 10:e0119383. doi: 10.1371/journal.pone.0119383
106. Miettinen JJ, Kumari R, Traustadottir GA, Huppunen ME, Sergeev P, Majumder MM, et al. Aminopeptidase expression in multiple myeloma associates with disease progression and sensitivity to melflufen. Cancers. (2021) 13:1527–34. doi: 10.3390/cancers13071527
107. Saxena PR. Interaction between the renin-angiotensin-aldosterone and sympathetic nervous systems. J Cardiovasc Pharmacol. (1992) 19(Suppl. 6):S80–8. doi: 10.1097/00005344-199219006-00013
108. Zucker IH, Xiao L, Haack KK. The central renin-angiotensin system and sympathetic nerve activity in chronic heart failure. Clin Sci. (2014) 126:695–706. doi: 10.1042/CS20130294
109. Wang W, Zou Z, Tan X, Zhang RW, Ren CZ, Yao XY, et al. Enhancement in tonically active glutamatergic inputs to the rostral ventrolateral medulla contributes to neuropathic pain-induced high blood pressure. Neural Plast. (2017) 2017:4174010. doi: 10.1155/2017/4174010
110. Wang WZ, Gao L, Wang HJ, Zucker IH, Wang W. Tonic glutamatergic input in the rostral ventrolateral medulla is increased in rats with chronic heart failure. Hypertension. (2009) 53:370–4. doi: 10.1161/HYPERTENSIONAHA.108.122598
111. Arnold AC, Gallagher PE, Diz DI. Brain renin-angiotensin system in the nexus of hypertension and aging. Hypertens Res. (2013) 36:5–13. doi: 10.1038/hr.2012.161
112. Benigni A, Cassis P, Remuzzi G. Angiotensin II revisited: new roles in inflammation, immunology and aging. EMBO Mol Med. (2010) 2:247–57. doi: 10.1002/emmm.201000080
113. Zhang ZY, Yu YL, Asayama K, Hansen TW, Maestre GE, Staessen JA. Starting antihypertensive drug treatment with combination therapy: controversies in hypertension - con side of the argument. Hypertension. (2021) 77:788–98. doi: 10.1161/HYPERTENSIONAHA.120.12858
114. Donnelly R, Manning G. Angiotensin-converting enzyme inhibitors and coronary heart disease prevention. J Renin Angiotensin Aldosterone Syst. (2007) 8:13–22. doi: 10.3317/jraas.2007.002
115. Picod A, Genest M, Assad N, Polidano E, Placier S, Gaudry S. Circulating dipeptidyl peptidase 3 modulates hemodynamics and the renin-angiotensin-aldosterone system in mice. Arch Cardiovasc Dis Suppl. (2022) 14:185. doi: 10.1016/j.acvdsp.2022.04.067
116. Veena V, Ganesh M, Silambanan S. A study of the correlation between brain-type natriuretic peptide (Bnp) levels & left ventricular ejection fraction (Lvef) in heart failure. New Front Med Res. (2021) 3:134–41. doi: 10.9734/bpi/nfmmr/v3/3514F
117. Rorth R, Jhund PS, Yilmaz MB, Kristensen SL, Welsh P, Desai AS, et al. Comparison of Bnp and Nt-Probnp in patients with heart failure and reduced ejection fraction. Circ Heart Fail. (2020) 13:e006541. doi: 10.1161/CIRCHEARTFAILURE.119.006541
118. Dikme R, Padak M, Isik M, Koyuncu I, Temiz E, Aydin MS, et al. Soluble St2 as a potential biomarker in pericardial fluid of coronary artery patients. Braz J Cardiovasc Surg. (2021) 36:677–84. doi: 10.21470/1678-9741-2020-0317
119. Aksoy A, Agirbasli M. Application of high-sensitivity troponin in suspected myocardial infarction. N Engl J Med. (2019) 381:2482–3. doi: 10.1056/NEJMc1913971
120. Van Diepen S, Katz JN, Albert NM, Henry TD, Jacobs AK, Kapur NK, et al. Contemporary management of cardiogenic shock: a scientific statement from the American Heart Association. Circulation. (2017) 136:e232–68. doi: 10.1161/CIR.0000000000000525
121. Deniau B, Rehfeld L, Santos K, Dienelt A, Azibani F, Sadoune M, et al. Circulating dipeptidyl peptidase 3 is a myocardial depressant factor: dipeptidyl peptidase 3 inhibition rapidly and sustainably improves haemodynamics. Eur J Heart Fail. (2020) 22:290–9. doi: 10.1002/ejhf.1601
122. Dépret F, Amzallag J, Pollina A, Fayolle-Pivot L, Coutrot M, Chaussard M, et al. Circulating dipeptidyl peptidase-3 at admission is associated with circulatory failure, acute kidney injury and death in severely ill burn patients. Crit Care. (2020) 24:168. doi: 10.1186/s13054-020-02888-5
123. Santos RAS, Oudit GY, Verano-Braga T, Canta G, Steckelings UM, Bader M. The Renin-Angiotensin System: Going Beyond the Classical Paradigms. Am J Physiol Heart Circ Physiol. (2019) 316:H958–70. doi: 10.1152/ajpheart.00723.2018
124. Deng Y, Tan X, Li ML, Wang WZ, Wang YK. Angiotensin-converting enzyme 2 in the rostral ventrolateral medulla regulates cholinergic signaling and cardiovascular and sympathetic responses in hypertensive rats. Neurosci Bull. (2019) 35:67–78. doi: 10.1007/s12264-018-0298-3
125. Santos RAS, Sampaio WO, Alzamora AC, Motta-Santos D, Alenina N, Bader M, et al. The Ace2/angiotensin-(1-7)/mas axis of the renin-angiotensin system: focus on angiotensin-(1-7). Physiol Rev. (2018) 98:505–53. doi: 10.1152/physrev.00023.2016
126. Stragier B, Hristova I, Sarre S, Ebinger G, Michotte Y. In Vivo Characterization of the angiotensin-(1-7)-induced dopamine and gamma-aminobutyric acid release in the striatum of the rat. Eur J Neurosci. (2005) 22:658–64. doi: 10.1111/j.1460-9568.2005.04188.x
127. Ferreira PM, Souza Dos Santos RA, Campagnole-Santos MJ. Angiotensin-(3-7) pressor effect at the rostral ventrolateral medulla. Regul Pept. (2007) 141:168–74. doi: 10.1016/j.regpep.2006.12.031
128. Prajapati SC, Singh R, Chauhan SS. Human dipeptidyl peptidase III regulates G-protein coupled receptor-dependent Ca2+ concentration in human embryonic kidney 293t cells. Biol Chem. (2016) 397:563–9. doi: 10.1515/hsz-2016-0117
129. Dinh QN, Drummond GR, Sobey CG, Chrissobolis S. Roles of inflammation, oxidative stress, and vascular dysfunction in hypertension. Biomed Res Int. (2014) 2014:406960. doi: 10.1155/2014/406960
130. Ghezzi P, Jaquet V, Marcucci F, Schmidt H. The oxidative stress theory of disease: levels of evidence and epistemological aspects. Br J Pharmacol. (2017) 174:1784–96. doi: 10.1111/bph.13544
131. Sies H. Oxidative stress: a concept in redox biology and medicine. Redox Biol. (2015) 4:180–3. doi: 10.1016/j.redox.2015.01.002
132. Mannaa A, Hanisch FG. Redox proteomes in human physiology and disease mechanisms. J Proteome Res. (2020) 19:1–17. doi: 10.1021/acs.jproteome.9b00586
133. Victorino VJ, Mencalha AL, Panis C. Post-translational modifications disclose a dual role for redox stress in cardiovascular pathophysiology. Life Sci. (2015) 129:42–7. doi: 10.1016/j.lfs.2014.11.008
134. Zhang Y, Duan X, Li J, Zhao S, Li W, Zhao L, et al. Inorganic arsenic induces Nrf2-regulated antioxidant defenses in both cerebral cortex and hippocampus in vivo. Neurochem Res. (2016) 41:2119–28. doi: 10.1007/s11064-016-1927-8
135. Matic S, Tomasic Paic A, Sobocanec S, Pinteric M, Pipalovic G, Martincic M, et al. Interdisciplinary study of the effects of dipeptidyl-peptidase iii cancer mutations on the Keap1-Nrf2 signaling pathway. Int J Mol Sci. (2022) 23:1994–9. doi: 10.3390/ijms23041994
137. Dick SA, Epelman S. Chronic heart failure and inflammation: what do we really know? Circ Res. (2016) 119:159–76. doi: 10.1161/CIRCRESAHA.116.308030
138. Fernandez-Real JM, Vayreda M, Richart C, Gutierrez C, Broch M, Vendrell J, et al. Circulating interleukin 6 levels, blood pressure, and insulin sensitivity in apparently healthy men and women. J Clin Endocrinol Metab. (2001) 86:1154–9. doi: 10.1210/jcem.86.3.7305
139. Furumoto T, Saito N, Dong J, Mikami T, Fujii S, Kitabatake A. Association of cardiovascular risk factors and endothelial dysfunction in Japanese hypertensive patients: implications for early atherosclerosis. Hypertens Res. (2002) 25:475–80. doi: 10.1291/hypres.25.475
140. Yu X, Yang Z, Yu M. Correlation of tumor necrosis factor alpha and interleukin 6 with hypertensive renal damage. Renal Fail. (2010) 32:475–9. doi: 10.3109/08860221003664280
141. Cicconetti P, Di Berardino A. Tortorelli D'Ambrosio M, Cacciafesta M. Resistant hypertension in the elderly. Recent Progr Med. (2017) 108:316–23. doi: 10.1701/2731.27837
142. Mortensen RF, Zhong W. Regulation of phagocytic leukocyte activities by C-reactive protein. J Leukoc Biol. (2000) 67:495–500. doi: 10.1002/jlb.67.4.495
Keywords: dipeptidyl peptidase 3, cardiovascular diseases, biomarker, therapeutic target, renin-angiotensin system
Citation: Ye P, Duan W, Leng Y-Q, Wang Y-K, Tan X and Wang W-Z (2022) DPP3: From biomarker to therapeutic target of cardiovascular diseases. Front. Cardiovasc. Med. 9:974035. doi: 10.3389/fcvm.2022.974035
Received: 20 June 2022; Accepted: 26 September 2022;
Published: 12 October 2022.
Edited by:
Claudia Penna, University of Turin, ItalyReviewed by:
Francesca Uberti, Università del Piemonte Orientale, ItalyYanfang Chen, Wright State University, United States
De-Pei Li, University of Missouri, United States
Copyright © 2022 Ye, Duan, Leng, Wang, Tan and Wang. This is an open-access article distributed under the terms of the Creative Commons Attribution License (CC BY). The use, distribution or reproduction in other forums is permitted, provided the original author(s) and the copyright owner(s) are credited and that the original publication in this journal is cited, in accordance with accepted academic practice. No use, distribution or reproduction is permitted which does not comply with these terms.
*Correspondence: Wei-Zhong Wang, d2Vpemhvbmd3YW5nQHNtbXUuZWR1LmNu; Xing Tan, dGFueGluZ19zbW11QDE2My5jb20=
†These authors have contributed equally to this work