Vascular aging and cardiovascular disease: pathophysiology and measurement in the coronary arteries
- 1Kolling Institute of Medical Research, Royal North Shore Hospital, Sydney, NSW, Australia
- 2Menzies Institute for Medical Research, University of Tasmania, Hobart, TAS, Australia
- 3Northern Clinical School, Faculty of Medicine and Health, The University of Sydney, Sydney, NSW, Australia
- 4Imaging and Phenotyping Laboratory, Charles Perkins Centre and Faculty of Medicine and Health, University of Sydney, Sydney, NSW, Australia
- 5Department of Cardiology, Royal North Shore Hospital, Sydney, NSW, Australia
Age is a key risk factor for cardiovascular disease, including atherosclerosis. However, pathophysiological disease processes in the arteries are not an inevitable feature of aging. Large cohort studies with arterial phenotyping along with clinical and demographic data are essential to better understand factors related to the susceptibility or resilience to age-related vascular pathophysiology in humans. This review explores the mechanisms by which vascular structure and function alters with age, and how these changes relate to cardiovascular pathophysiology and disease. Features of vascular aging in the coronary arteries have historically been difficult to quantify pre-mortem due to their size and location. However, non-invasive imaging modalities including CT Coronary Angiogram are now being used to assess coronary vascular age, and further advances in imaging analysis such as the CT Fat Attenuation Index will help provide further measurement of features associated with coronary vascular aging. Currently, markers of vascular aging are not used as therapeutic targets in routine clinical practice, but non-pharmacological interventions including aerobic exercise and low salt diet, as well as anti-hypertensives have been demonstrated to reduce arterial stiffness. Advances in imaging technology, both in acquisition and advanced analysis, as well as harmonisation of measurements for researchers across the globe will be invaluable in understanding what constitutes healthy vascular aging and in identifying features of vascular aging that are associated with coronary artery disease and its adverse outcomes. Assessing such images in large cohorts can facilitate improved definitions of resilient and susceptible phenotypes to vascular aging in the coronary arteries. This is a critical step in identifying further risk factors and biomarkers within these groups and driving forward the development of novel therapies aimed at slowing or stopping age-related vascular changes in the coronary arteries.
1. Introduction
1.1. Age-related cardiovascular risk
Age is widely considered a key risk factor for cardiovascular disease (CVD) (1). However, CVD is not an inevitable feature of aging as evidenced by “resilient” elderly with no disease (2). Historically it has been a truism that aging is synonymous with disease (3), with the contribution of age to cardiovascular risk variously attributed to the time-dependent nature of CVD processes, or to cumulative exposure to cardiovascular risk factors over time (4). In recent years, the paradigm has evolved to assign “age-related risk” to a complex interplay between the mechanistic and molecular effects of age on cardiovascular structure and function (4), and the specific pathophysiological mechanisms which produce disease (3).
1.2. Trends in aging and CVD
Coronary artery disease (CAD) constitutes a large proportion of overall morbidity and mortality attributable to CVD and is the leading cause of death worldwide, both in the western world and developing countries (5, 6). This continues to be the case, despite public health efforts targeting the prevention and management of CAD which have resulted in the age-adjusted death rate falling across the developed world (7). However, there has been an absolute increase in both the overall population incidence and prevalence of CAD due to increased longevity of both men and women and increased prevalence of traditional risk factors (8). In Australia, the shift towards an older population is expected to continue, with the over 65 year old population projected to increase by 57% from 2015 to 2030, compared to an increase in the general population of only 23% (9). A combination of these demographic trends and increased awareness of how age alters the structure and function of the vascular tree (so called vascular aging) has led to an increased focus on exploring the pathophysiology and management of this process (10).
1.3. Vascular aging
In the context of identifiable age-driven changes in the structure and function of the arteries, the term vascular aging has been coined (3, 11). More recently, there has also been a focus on defining and identifying patient groups who demonstrate increased susceptibility or resilience to the effects of aging on the vasculature (12–15) by classifying them into early and healthy vascular aging phenotypes. Defining these cohorts is critical in allowing further analysis of novel risk factors and therapeutic targets, as well as blood-based biomarkers which characterise these groups. However, there is currently no agreed definition of the parameters which characterise these phenotypes.
1.4. Vascular aging in the coronary arteries
The conceptualisation of the relationship between CAD and age has evolved to acknowledge the interaction of atherosclerotic plaque development with the pathophysiological features of vascular aging, which is also accelerated by traditional risk factors for atherosclerosis (16). From this perspective, key age-associated features including endothelial dysfunction, arterial stiffening, and intimal thickening serve as the foundation for the later development of atherosclerosis, with age also mediating the composition of plaque (17). Whilst these features are part of vascular aging, not all people who display them develop CAD. However, there have been limited attempts at identifying the conditions in which these features constitute physiological or healthy vascular aging, and when they lead to susceptibility to the development of CAD and related adverse events. Further characterisation of these factors may be possible utilising widely available imaging markers including coronary artery calcium (CAC) detected by Computed Tomography Coronary Angiogram (CTCA) (13), in conjunction with recently developed imaging analytics that can identify age-associated changes in the coronary vasculature (18). In this context, this review explores how age alters the structure and function of the vasculature, and our current understanding of how these changes are associated with the development of atherosclerosis. It then explores how the clinical manifestations of these changes are measured, with a focus specifically in the coronary arteries, the unique challenges that arise due to their size and location in the body, the current state of therapeutics in vascular aging, and recent advances allowing in vivo assessment.
2. Features of vascular aging—pathophysiology and relationship with coronary artery disease
Figure 1 illustrates the known key contributors to vascular aging: (1) increased arterial stiffness, (2) intimal thickening, (3) chronic pro-inflammatory conditions, (4) endothelial dysfunction, (5) increased atherogenic conditions and formation of unstable plaques. Figure 2 outlines the cellular changes that drive these features of vascular aging.
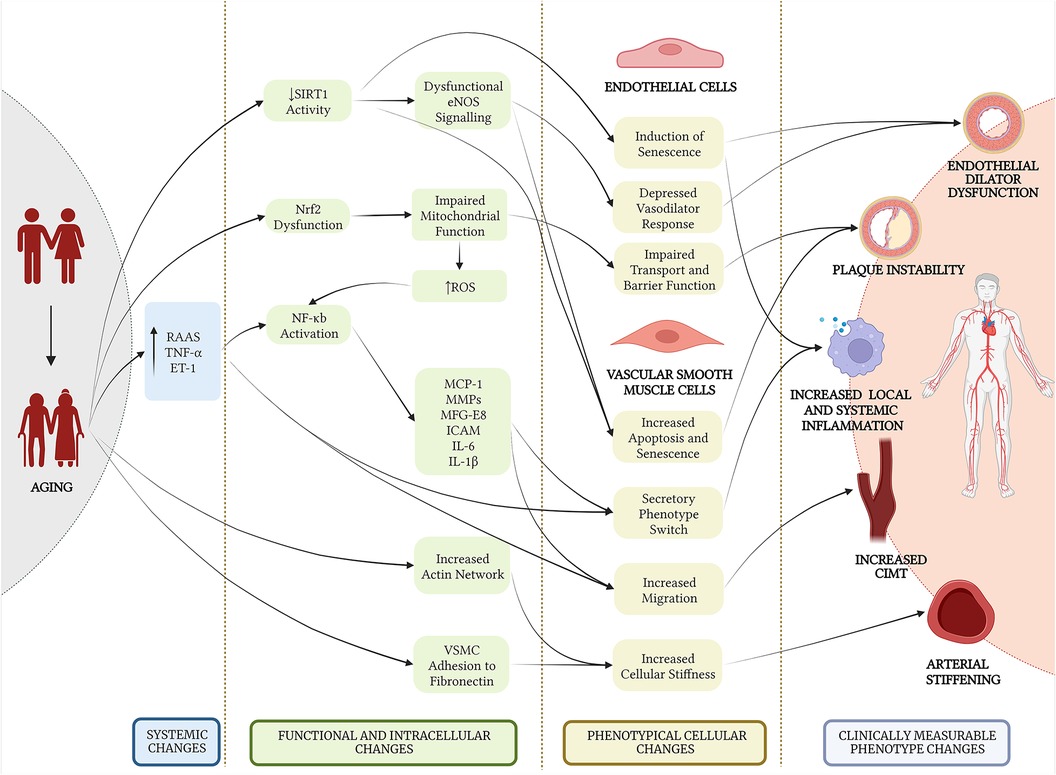
Figure 2. An outline of cellular changes of vascular aging. RAAS, renin-angiotensin-aldosterone system; TNF-α, tumor necrosis factor alpha; ET-1, enodthelin-1; eNOS, endothelial nitric oxide synthase; ROS, reactive oxygen species; MCP-1, monocyte chemoattractant protein-1; MMP, matrix metalloproteinase; MFG-E8, milk fat globule-EGF factor 8; ICAM, intracellular adhesion molecule; IL, interleukin; VSMC, vascular smooth muscle cell.
2.1. Arterial stiffness
Arterial stiffness is a measure of the resistance of the arterial wall to dilation from an increase in volume within the artery (19) and is one of the key features of vascular aging. It is most commonly measured by pulse wave velocity in the large, elastic arteries such as the aorta, common femoral and carotid arteries. Whilst increased arterial stiffness occurs in a variety of disease states including hypertension and diabetes, it is most commonly found to be associated with aging (20). Aging has been demonstrated to lead to increased arterial stiffness by affecting both the endothelial cells and vascular smooth muscle cells (VSMCs) (21, 22) and by modulating the composition of the extra-cellular matrix (ECM) (23), all of which are key in maintaining arterial compliance.
Aging causes arterial stiffening by increasing matrix metalloproteinase (MMP) production and secretion from VSMCs (24–26) and also MMP activation (26, 27). This process is mediated by numerous pro-inflammatory cytokines. MMPs and cytokines play a key role in promoting vascular remodelling through collagenolysis and elastolysis (28), as well as collagen formation (29). These processes are thought to play a role in observed histological changes in large aging arteries, namely the increase in collagen and the collagen to elastin ratio (30, 31).
Aging has also been demonstrated to modulate cell-ECM interactions and VSMC stiffness, and there has been an increased focus on these mechanisms as a cause of arterial stiffening. Crosslink products of elastin including desmosine and isodesmosine have been shown to decrease in age, whilst pyridoline, the crosslink product of collagen has been demonstrated to increase (32). The combination of increased MMP activity, crosslink degradation, and repeated mechanical and oxidative stress on the arteries contributes to the rupture of elastic lamellae that has been observed with aging (33). Moreover, the increase in collagen and the collagen to elastin ratio in aging leads to more of the pulsatile force from systole being transmitted onto collagen fibres, which are 100–1,000 times stiffer than elastin (34), promoting further rupture of the elastic lamellae in the arterial wall and enhancing its rigidity. The changing composition of the ECM and resulting stiffness also drives cellular stiffness of VSMCs (22). Aged VSMCs have been demonstrated to exhibit cytoskeletal changes with a significantly more extensive actin network (20). They also show increased adhesion to fibronectin in the ECM, one of the key interactions that form the basis of the ECM-integrin-cytoskeletal axis that mediates the tone and compliance of the vascular wall to changes in volume and pressure (20).
The age-related cellular changes that lead to increased arterial stiffening are well described and clinical studies have demonstrated a positive association between arterial stiffness and coronary artery calcium (35). However, it is still unclear what drives the relationship between arterial stiffness and atherosclerosis (36). To further elucidate this relationship, identification of populations who exhibit early vascular aging as measured by arterial stiffening, and atherosclerosis will be helpful. Assessment of the identifiable changes in the ECM, VSMCs and endothelial cells, as well as any associated compensatory mechanisms in this group would provide insights into the mechanism through which arterial stiffness and atherosclerosis are linked.
2.2. Intimal-Media thickening
Subclinical changes in the structure of the arterial wall from the effects of aging start to appear from the second decade of life (37, 38). As we increase in age, the tunica intima starts to thicken as a physiological response to the forces acting on the vessel walls. This phenomenon is known as intimal thickening, and it has been observed that a two to threefold increase in the intimal-media layer occurs between the ages of 20 and 90 (39). The process occurs both in the large arteries and also within arterioles in the microvasculature (40). In fact, intimal thickening in the arterioles has a proportionally increased effect on lumen diameter and may adversely reduce end-organ perfusion (41). This thickening is made up of layers of VSMCs which have migrated from the tunica media and are intercalated between collagen and elastic fibres (37, 42). This process has been demonstrated to be driven by age related increases in angiotensin II and MGF-E8 signalling (43).
Intimal thickening manifests differently in the coronary arteries, where it occurs in an eccentric rather than concentric manner as in the aorta (44–46). The outer layer of the thickened intima has been demonstrated to have an enriched level of proteoglycans, and particularly biglycan, which has a high affinity for lipoprotein binding, a key process in early atherogenesis (47). Intimal thickening also leads to increased permeability of the vascular wall, which results in cholesterol and phospholipid deposition in the sub-endothelial space (48). This increase in permeability may increase susceptibility to hypercholesterolaemia and the development of atherosclerotic plaque, as has been demonstrated in mouse models (49, 50).
The degree of intimal-medial thickening in the carotid artery has been demonstrated to be predictive of future clinical cardiovascular events (51). However, carotid intimal-medial thickness has a strong, positive linear association with age even in healthy populations free from CVD risk factors (52). Thus, further exploration of when intimal thickening is a physiological part of the aging process and when it causes or is a marker of susceptibility to the development of atherosclerosis is needed, both in the coronary arteries and the wider vascular system.
2.3. Pro-inflammatory state
Vascular inflammation has been acknowledged as a key feature in atherogenesis (53), and there have been recent advancements in our capability to quantify this process in the coronary arteries by assessing change in the surrounding perivascular fat, as measured by computed tomography coronary angiogram (CTCA) and the fat attenuation index (FAI) (54). There is strong experimental and clinical evidence that chronic, sterile, low-grade inflammation in the vasculature is a key characteristic of aging, and plays a critical role in driving phenotypic shifts of both VSMCs and endothelial cells which further perpetuate a pro-inflammatory state (55). This multi-faceted process occurs via changes in key signalling cascades including increased renin-angiotensin-aldosterone system and endoethlin-1 receptor A activation and increased advanced glycation end products (55). This drives activation of pro-inflammatory signalling pathways including NF-κb, induction of pro-inflammatory cytokines including monocyte chemo-attractant protein-1, interleukin (IL)-6, IL-1β and tumor necrosis factor α, and increased oxidative stress and generation of reactive oxygen species (25, 56, 57). Downstream pro-inflammatory transcription factors in these pathways are upregulated whereas protective factors from inflammation have been demonstrated to decrease with age (56, 58–60). This pro-inflammatory environment drives vascular endothelial dysfunction and a pro-atherogenic environment through impairment of cellular metabolism, increasing oxidative stress and increasing apoptosis (61–64). The increased generation of reactive oxygen species has also been strongly implicated in driving microvascular dysfunction (65), which plays a driving role in age-associated end-organ damage (41).
The chronic low-grade inflammation in aging has been demonstrated to be a driving force behind a switch in VSMC phenotype. The environmental changes caused by aging include chronic inflammation, mechano-stimuli, cell death, calcification, and epigenetic events (66). These changes, especially the angiotensin II mediated chronic inflammation drive a modification in the VSMC phenotype from “contractile” to “secretory” (21, 43, 58). In their normal contractile state, VSMCs have a high expression of genes involved in the synthesis of proteins which are critical for myofilament structure and function, including α-smooth muscle actin, smooth muscle myosin heavy chain, and calponin (22). The secretory phenotype exhibits reduced expression of these genes as well as an increase in the production of pro-inflammatory cytokines, chemokines and adhesion molecules (22, 24). This pro-inflammatory secretory phenotype has also been demonstrated to be driven in part due to increased signalling of toll-like receptor 4 and Myd88 (67). This phenotype is also observed in intimal layer VSMCs, indicating that SMCs may dedifferentiate during migration from the medial layer to the intimal layer during the intimal thickening process which also occurs in aging (37).
Whilst the mechanism of inflammation driving endothelial dysfunction and the switch in VSMC phenotype has been described, it is unclear at what levels these changes mediate the development of CAD. With the advances and development of imaging resolution and analysis such as CT FAI, it is now possible to quantify localised levels of inflammation in the coronary arteries (18). This may enable us to define levels of localised inflammation that drive morphological and functional changes in the vasculature that lead to susceptibility to the development of atherosclerosis and CAD.
Aging has also been demonstrated to accelerate endothelial cell senescence, a process which is mediated amongst other factors by down-regulation of bradykinin type 2 receptor expression (68). One other pathway of growing interest that has also been demonstrated to mediate endothelial senescence is the age related reduction in activity of the encoding gene SIRT1 (69). This gene encodes for the sirtuin enzyme Sirt1, which is a NAD + (nicotinamide adenine dinucleotide) related histone-deactylase enzyme, and has been implicated in regulation of endothelial nitrous oxide synthase (eNOS) activity and expression, p53 acetylation and earlier induction of the stress-induced premature senescence phenotype in endothelial cells (70). Cellular senescence is part of the aging process, and is in essence an irreversible withdrawal from the cell cycle (71). Indeed, increased levels of endothelial senescence has been observed even in healthy, older adults (72), so it is yet to be identified at which point increased senescence drives susceptibility to disease. Evidence suggests that vascular endothelial senescence is inversely related with endothelial function, and thus contributes to the increasing endothelial dysfunction as we age (72–74). Endothelial senescence has also been linked to upregulation of production and release of a range of pro-inflammatory cytokines, contributing to an inflammatory environment which is a hallmark of the aging process (75). On top of this, the quantity and function of endothelial progenitor cells (EPCs) decreases as we age, and thus dysfunctional or senescent endothelial cells are not replaced at the same rate, which further perpetuates and promotes endothelial dysfunction in aging (76).
2.4. Endothelial dysfunction
The vascular endothelium plays a critical role in the regulation of vasculogenesis, angiogenesis, vascular tone and inflammation, as well as providing barrier functions for the vessel wall (77, 78). Multiple studies have indicated that aging contributes to endothelial dysfunction by increasing oxidative stress through the generation of reactive oxygen species by nicotinamide adenine dinucleotide phosphate oxidases, increased levels of arginase activity, and decreased eNOS activity (79–81). This age-related dysfunction is also mediated by gender, and has been demonstrated to occur ten years later in women compared to men (82). Crucially, this dysfunction depresses the endothelium-dependent dilator response by reducing the bioavailability of nitrous oxide in response to vasoconstriction and shear forces (83) and we are consequently able to measure the degree of endothelial dysfunction by assessing the vascular vasodilatory response to an external stimulus. Apart from being a critical vasodilator to help modulate arterial flow to match metabolic demand, nitrous oxide has also been demonstrated to have strong anti-inflammatory effects and inhibit leukocyte adhesion and VSMC proliferation (84–86). Resultingly, it has been demonstrated that sufficient bioavailability of nitrous oxide is critical to maintain arterial function (87). In the microvasculature, these alterations in endothelial function lead to impaired vasodilatory function of arterioles and higher capillary pressures which causes hyperfiltration, protein leakage and oedema (40). As the microvasculature is the key interface for delivery of essential oxygen and nutrients to the tissues, these age-associated changes are observed by assessing reduced flow or damage to end-organs including the brain, heart and kidneys (40).
Aging also affects endothelial function not only by impairing mechanotransduction of shear stress, but also by reducing laminar shear stress through increases in arterial diameter and reductions in blood velocity (88–91). Laminar shear stress has been demonstrated to maintain endothelial cell quiescence and function, stimulate eNOS expression, suppress endothelial proliferation and promote the expression of atheroprotective genes (92, 93). Disturbed and reduced shear stress accelerates endothelial proliferation and turnover, and promotes expression of atherogenic and thrombotic genes (94, 95).
Understanding how age drives endothelial dysfunction is critical in identifying the mechanisms through which populations with and without disease adjust to these changes. One study has demonstrated that healthy older males have higher levels of phosphorylated eNOS expression in endothelial cells compared to younger (96), which may be a compensatory mechanism in healthy vascular aging against reduced nitrous oxide bioavailability. Measurement of endothelial function in populations with CAD may enable identification and characterisation of the tissue level processes occurring in endothelial cells in both resilient and susceptible individuals. Further measurement of endothelial dysfunction in patients with clinical microvascular dysfunction but no epicardial coronary artery disease [commonly termed ischemia with no obstructive arteries (97)] may also shed light on what drives resilience and susceptibility to vascular aging in the microvasculature compared to the microvasculature.
2.5. Pro-atherogenic state and plaque instability
Atherosclerosis is a complex pathological process, mediated by numerous risk factors, including traditional ones such as hypertension, dyslipidaemia, smoking, diabetes and metabolic syndrome (98). Age-driven changes in the vasculature not only create a pro-atherogenic state as described above, but also influence the composition and vulnerability of the atherosclerotic plaque that is formed in the disease process.
Aged VSMCs have been shown to be more susceptible to apoptosis in mouse models, a phenomenon which has been demonstrated to be linked to dysfunctional eNOS signalling which occurs in age (99). VSMC apoptotic indices increase as atherosclerotic lesions develop, and chronic VSMC apoptosis which occurs in aging has been shown to stimulate plaque development and progression (100). Atherosclerotic plaque stability depends on the thickness of the fibrous cap and the degree of cap inflammation, with the most common precursor lesion to plaque rupture being thin-cap fibroatheroma (TCFA) (101). VSMC apoptosis in atherosclerotic plaques has been demonstrated to induce cap-thinning, breakdown of collagen and enlargement of the necrotic core, all of which increase plaque vulnerability (102). Aged VSMCs also display a reduction in proliferation rates and significant increase in the population doubling time, which is similar to VSMCs taken from advanced atherosclerotic plaques (21). They are also more likely to enter senescence or irreversible growth arrest, which is mediated by increased angiotensin II signalling seen in the chronic inflammation pathway associated with age (103, 104). In vitro findings also suggest that plaque stability and progression may be mediated by enhanced VSMC proliferation (21), as successful plaque repair is dependent on VSMC proliferation and synthesisation of ECM (105).
Impairment of mitochondrial function in the vasculature has also been demonstrated to result from aging, due to increased amounts of mitochondrial DNA (mtDNA) mutations (106, 107) and Nrf2 dysfunction which limits the efficiency of mtDNA repair mechanisms (25). The efficacy of the electron transport chain decreases with age, resulting in electron leakage and reduced levels of ATP synthesis (108). This dysfunction leads to increased production of reactive oxygen species, which once above a certain threshold continue to promote and perpetuate age associated damage of the mitochondria and surrounding vasculature (109). This dysfunction impairs the functional processes of the vessel wall including membrane transport and barrier functions, which are highly dependent on normal energy metabolism (25), and also promotes vulnerable plaque formation in mouse models (110–112). This was also demonstrated in a recent human study where it was shown that mtDNA damage was associated with the incidence of TCFA in the coronary vessels (113).
Whilst these genetic and cellular changes may suggest that TCFA prevalence should increase with age, a recent analysis of plaque characteristics showed that TCFA prevalence increases significantly with age in women but not men (114). This highlights a need to further explore the levels of VSMC apoptosis and mtDNA mutations in older women and men, and how they relate to the observed clinical manifestations. This will allow a better understanding of how these cellular and genetic changes drive susceptibility to vulnerable plaque development and the mediating factors of this relationship.
3. Clinical assessment of vascular aging
3.1. Arterial anatomy—regional differences
Arteries across the vascular tree are structurally composed of three different layers, the tunica intima, media and adventitia. The tunica intima is made up of a single layer of endothelial cells and a supporting layer of elastic tissue, on the luminal side of the artery wall. The tunica media is made up of elastic and muscular tissue comprised of elastin, collagen and VSMCs in varying amounts, depending on vessel size and location (24). The tunica adventitia consists of fibrous tissue and provides structural support and shape to the artery (115).
Whilst arteries across the vascular tree share common structural and cellular elements, there is significant heterogeneity in their composition in accordance with arterial location and function (116). For example, larger, elastic arteries such as the aorta and carotids have significantly more musculo-elastic complexes, with higher amounts of elastin in the ECM to help convert pulsatile pressure from the pumping of the heart into continuous laminar flow in the peripheral arterioles (24). The coronary arteries have lesser amounts of elastic tissue and higher numbers of VSMCs than the larger elastic arteries (117). The more distal peripheral arteries have the lowest amount of elastic tissue and highest numbers of VSMCs, as their primary function is to regulate vasomotor tone. These factors need to be considered when measuring changes between individuals, or in the same individual over time.
3.2. Measurement of vascular aging
The differences in the composition of arteries across the vascular tree result in variability in the way that they are affected by aging (11, 118, 119). This impacts how assessment of vascular aging can be performed, as not all the pathophysiological phenomenon that occur are easily measurable in the clinical setting. Moreover, not all parts of the vascular tree are easily measurable, especially the deeper, smaller calibre arteries. There has been limited exploration of the congruence in the assessed vascular age of participants between different investigations and the different locations of the vasculature they are measuring (120–122), and the findings that exist are inconsistent. One study demonstrated largely different results when measuring vascular age by carotid intima-media thickness (CIMT) compared to carotid-femoral pulse wave velocity (cfPWV) (121), and another showed a significant difference in the calculated vascular age when using CIMT compared to CAD burden reflected by CAC based calculations (122). On the other hand, one other study showed similarities between vascular age derived from CAC scores and CIMT (120).
Currently, clinical assessment of vascular aging has focused predominantly on assessment of arteriosclerosis as measured by cfPWV, which measures the stiffness of the descending and thoracic aorta (123). An overall vascular age can then be extrapolated from this regionally based measurement technique (124). The heterogeneity in the effects of aging on the vasculature underscores the importance of measuring vascular aging at the local level in territories such as the coronary arteries which are implicated in many of the adverse clinical outcome of CVD. This would better inform territory specific phenotypes of resilience and susceptibility to vascular aging for further investigation.
There has been limited exploration of how best to measure coronary artery aging using current imaging modalities, and very few assessments of the healthy or early aging phenotype in the coronary arteries specifically. Studies to date have primarily focused on atherosclerosis and coronary artery calcium as a measurement from which to derive vascular age, given atherosclerosis is the more commonly observed and easily measurable age-related pathophysiological change in the coronary vessels (13, 125). Moreover, there has been little to no exploration of how other characteristics of the vasculature that are associated with age including arteriosclerosis, intimal thickening, endothelial dysfunction, and low-grade inflammation, may be included in this assessment Table 1.
3.3. Measurement of arterial stiffening
Arterial stiffening can be measured at the systemic, regional and local level. Whilst systemic arterial stiffness can only be estimated from models of the body's circulation, regional stiffness can be measured via the assessment of pulse wave velocity between two arterial sites. Assessment of coronary arterial stiffening requires imaging techniques that can measure stiffening at the local level. Ultrasonography can measure the stiffness of the arterial wall by observing the change in pressure related to the change in volume within the artery (126–128). It can also measure the degree of intimal thickening within an artery, providing insights into the relationship between intimal thickening and stiffening (123). However, due to its high technical requirements and duration as an investigation, ultrasonography to measure local arterial stiffness is currently reserved for mechanistic analyses rather than epidemiologic studies (123, 126). Previous attempts to measure local coronary artery stiffness have mostly encountered methodological issues that preclude them from being used in broader clinical practice and research (129, 130). Moreover, measurement of the necessary parameters of pressure and velocity to assess stiffness in the coronary arteries can only currently be performed by invasive angiogram (130). In terms of non-invasive assessment, MRI measurement of local arterial stiffness is a novel field, with proprietary software enabling assessment for localised measurements of the aorta (131), but not yet the coronary arteries. Thus, there is limited scope to assess coronary arterial stiffness non-invasively with current imaging techniques.
Outside of the coronary arteries, measurement of arterial stiffness is the most common assessment used to define phenotypes of early or healthy vascular aging. Pulse wave velocity between the common carotid artery and the common femoral artery is the gold standard for measuring arterial stiffness, as it incorporates the aortic and aortoiliac pathways which are exposed to the greatest haemodynamic load from left ventricular systole (123), and thus are more significantly affected by arterial stiffening (126). Meta-analyses of large cohort studies including the Rotterdam and Framingham studies, have clearly demonstrated aortic stiffness as measured by cfPWV has an association with cardiovascular events and mortality, even once traditional risk factors are accounted for (132, 133). An analysis of 17,635 participants demonstrated a hazard ratio (HR) of 1.30 [95% confidence interval (CI): 1.18–1.43] for cardiovascular events and 1.23 (95% CI: 1.11–1.35) for coronary events per 1-SD change in logeaPWV (133) after adjustment for age, sex and traditional risk factors, and another of 15,877 participants demonstrated a pooled relative risk (RR) increase in cardiovascular mortality of 1.15 (95% CI: 1.09–1.21) for every 1 m/s increase in aortic PWV (132). It has also been demonstrated to be an independent predictor of cardiovascular outcomes in a range of populations (132, 134–136). There are multiple ways of measuring the pulse wave at the common carotid artery and common femoral artery, including transcutaneous pressure transducers (137), doppler ultrasound (138) and also MRI (131). This results in cfPWV being a simple, non-invasive, and reproducible investigation which makes it reasonably easy to use in the clinical setting, and as a means of assessing participants in research studies.
3.4. Measurement of intimal thickening
Measurement of intimal thickening is most commonly performed by assessing the carotid artery and has also been utilised in studies to assess the degree of vascular aging in different populations (139–141). However, there has been limited exploration of assessing intimal thickening in the coronary arteries, with invasive methods such as intravascular ultrasound (IVUS) or intravascular optical coherence tomography (IV OCT) used in specific small study populations including Kawasaki disease and cardiac transplant recipients (142–144). IVUS and OCT are both accurate measurements of intimal thickness when compared to histological measurement (142, 145). However, the invasiveness of these procedures limits their broader use in assessing subclinical levels of intimal thickening. High resolution transthoracic echocardiography has only thus far been able to detect intimal thickening in the left main and left anterior descending arteries (146, 147) and thus would provide an incomplete assessment of the major coronary arteries. MRI has been used to measure wall thickness in patients with established CAD in a few small study populations (148, 149). However, there are limitations to its broader use because of the higher technical and patient requirements, due to the smaller lumen and effect of both cardiac and respiratory motion on the vessels themselves (148).
Outside of the coronary arteries, carotid ultrasonography is non-invasive, safe and the most widely used clinical investigation which can measure the degree of intimal-media thickening of the common, internal and external carotid arteries. Whilst common femoral artery intimal-media thickening has also been demonstrated to have a positive correlation with CAD, it is less widely used in both the clinical setting and in assessments of vascular aging. Multiple meta-analyses of large cohort studies have demonstrated an association between CIMT and adverse cardiovascular outcomes including stroke and myocardial infarction (51, 150). Most recently, a meta-analysis of 119 randomised control trials (RCTs) demonstrated an association between intervention effects on CIMT progression and the risk reduction in cardiovascular outcomes [pooled RR of 0.91 per 10 µm/y reduction of CIMT progression (95% CI: 0.87–0.94)] (151), supporting its use in clinical trials as a surrogate marker of CVD risk. The additional predictive value of CIMT above and beyond the commonly used clinical risk scores is unclear, as multiple studies (150, 152, 153) have found that there was no significant improvement in these models with the addition of CIMT. Interestingly, in the Multi-ethnic Study of Atherosclerosis (MESA) cohort, addition of CIMT improved prediction from a baseline model of traditional risk factors included in the Framingham Risk Score for CAD events [Net Reclassification Index (NRI): 4.2%, p = 0.035 for presence of plaque] but not for the wider endpoint of CVD events, which included stroke (154). CIMT may be useful in identifying patients who are likely to have CAD and therefore an increased vascular age based on assessment of their coronary arteries, as a recent systematic review demonstrated that CIMT increased linearly with severity of CAD irrespective of its significance, and also showed a moderate correlation between carotid and coronary artery stenosis (155).
3.5. Measurement of inflammation
Age has been demonstrated to contribute to an inflammatory vascular environment through activation of a range of pro-inflammatory processes (156). There is also some evidence to suggest that aging may exacerbate the vascular inflammation caused by other cardiovascular risk factors such as obesity and hypertension, although this has not been well explored (157). Given the widely acknowledged importance of vascular inflammation as part of the process of atherogenesis, there have been concerted efforts to develop non-invasive localised measurement methods to improve cardiovascular risk stratification (54).
Recent developments in CTCA image analysis have led to the development of the FAI, which quantifies coronary vessel inflammation based on the change in attenuation of the surrounding perivascular adipose tissue (54). This has been shown through elegant bed to bench experiments, to closely reflect levels of inflammation and cytokine release from inflamed atherosclerotic plaque, and has additional prognostic utility (18, 158, 159). It has also been demonstrated to have prognostic utility in risk stratifying individuals with low or no coronary artery calcium based on the identified “residual inflammatory risk” as measured by the FAI (18). In the initial CRISP-CT derivation and validation cohorts, a high CT FAI value (defined as greater than or equal to 70.1 Hounsfield Units) was demonstrated to be predictive of all-cause [HR 2.55 (95% CI 1.65–3.92), p < 0.0001] and cardiac mortality [HR 9.04 (95% CI 3.35–24.40), p < 0.0001] (18) after adjustment for age, sex and traditional risk factors. These findings were supported in the SCOT-HEART trial, where further development of this analysis resulted in the creation of the fat radiomic profile (FRP) biomarker, which showed a signification association with major adverse cardiac events [HR 1.12 per 0.01 increase in FRP (95% CI 1.08–1.15), p < 0.001] (158). These results have also been supported by smaller post-hoc analysis of cohort studies in Asian populations (160, 161). However, at present, there has not been further validation of CT FAI's association with CVD outcomes in larger cohort studies, and it has not been incorporated or used in assessing vascular aging. In addition to FAI, FDG positron emission tomography (PET) has also been proposed as a non-invasive modality to measure low grade peri-coronary inflammation (162). However, given the recency of the interest in peri-coronary adipose tissue (PCAT), there have been limited studies exploring this imaging modality. One study found that PCAT uptake overall was higher in patients with CAD compared to non-CAD controls (163). It also found that FDG uptake in PCAT was positively related to the degree of stenosis in the respective coronary artery in patients with a body mass index greater than 25 (163).
Outside of the coronary arteries, vascular inflammation has been measured at the systemic level through high-sensitivity C-reactive protein. Measurement of this inflammatory marker has been demonstrated to predict future vascular events and improve global risk reclassification (164, 165), however it is poorly associated with local vascular biological processes of atherogenesis and inflammation (166). FDG PET has been utilised in studies to measure levels of vascular inflammation (167–169), and in assessment of patients with central and peripheral vasculitis (170). However, there are limitations to its wider utilisation for measurement of sub-clinical vascular inflammation. These include large variations in the imaging protocol and methodology between studies, the influence of patient factors including BMI and pre-scan glucose on results and the lack of validation in larger cohorts (169, 171, 172).
3.6. Measurement of endothelial dysfunction
Endothelial dysfunction has been demonstrated to contribute to the pro-inflammatory environment in aged vessels through the reduced bio-availability of nitrous oxide (84–86). The most direct and also invasive method used to measure endothelial dysfunction is intracoronary artery infusions, which involves using quantitative coronary angiograms or intravascular ultrasound to measure arterial diameter and blood flow velocity in response to a vasoactive intra-arterial infusion, most commonly acetylcholine (173). Acetylcholine is commonly used as it causes coronary artery dilatation in healthy endothelium, and a paradoxical constriction in dysfunctional endothelium (173). Whilst coronary endothelial function as measured by quantitative coronary angiogram has been posited as a superior risk stratification tool to the widely used Framingham Risk Score (174), it is not feasible as a screening tool or for epidemiological or large cohort studies due to its invasiveness and associated risk (175).
Another measure of coronary endothelial function is the coronary flow reserve (CFR), which is defined as the ratio between the maximal hyperaemic coronary blood flow over the resting baseline level (176). Fractional flow reserve, which is a ratio of the distal to proximal pressure in a coronary artery across an epicardial lesion during maximal pharmacological vasodilation, and CFR are being more commonly used to assess stenosis severity of CAD (177). However unlike fractional flow reserve, CFR can assess microvascular dysfunction independent of epicardial CAD (176, 178). Currently, CFR is most commonly measured invasively during angiography, and a CFR of less than 2.5 is diagnostic for coronary microvascular dysfunction (CMD) (97). Non-invasive measurement of CFR can be performed by transthoracic doppler echocardiogram assessment of the left anterior descending artery (179, 180), however this is limited as it is highly operator dependent due to the technical considerations of the scan and only measures one of the major coronary vessels. Two studies using pharmacologically induced stress echocardiography found an association between age and decreased CFR (181, 182). However, this has yet to be incorporated in any measure of coronary vascular aging. Other non-invasive measures of CMD include PET and cardiac MRI, through measurement of myocardial blood flow to calculate the myocardial perfusion reserve. This is a ratio of the maximum blood flow through the myocardium in response to stress compared to baseline (183). However, these non-invasive measures have been limited primarily to research rather than clinical practice given their high cost, limited availability, and long scanning time (184).
Higher field 3.0 T MRI imaging has recently been demonstrated in a few small studies to be able to measure endothelial-dependent coronary vasoreactivity to isometric hand grip exercises in both healthy and diseased patients (185, 186). However, to date this has been limited to studies with small sample sizes, most likely due to the difficulty from both a technical and economic perspective in ascertaining large groups of cardiac MRIs. Several studies have demonstrated that assessment of coronary vasodilator function with PET is an independent predictor of cardiac mortality in patients with known or suspected CAD (187–189). However, this marker is yet to be incorporated in any assessments of vascular aging.
Outside of the coronary arteries, endothelial dysfunction is measured based on the vasodilatory effect of nitrous oxide. This can be done through a variety of methods, with the most widely used and validated being flow-mediated dilatation (FMD) of the brachial artery (190). This is primarily due to the fact that it is non-invasive, easy to perform and efficient (190). A meta-analysis of 17,280 participants demonstrated a pooled adjusted RR for a 1% increase in FMD of 0.88 (95% CI 0.84–0.91) (191), demonstrating that FMD was a significant predictor of cardiovascular events in both population and cohort studies. This was reinforced by four other meta-analyses, which also used pooled effect models adjusted for confounding risk factors with similar results (192–195). However, some of these studies note that the methodological quality of the studies, and poor standardisation of measurement make it difficult to definitively assess its predictive capability beyond current traditional models (192, 193). Indeed, this was reflected in the MESA cohort, where the addition of FMD did not improve the Framingham Risk Score in terms of discrimination but did improve classification (NRI: 0.29, p < 0.001) (196). These factors have led to a position paper by the European Society of Cardiology which underlines FMD's function primarily as a valuable research tool (136). Other minimally-invasive methods of measuring endothelial dysfunction include peripheral arterial tonometry, and quantification of EPCs via an EPC-colony forming unit assay and measurement of circulating EPCs (190). However, both methods have significant drawbacks, as peripheral arterial tonometry measures micro vessel vasodilatory response as opposed to large vessels, and EPC assay results are highly operator dependent (190).
3.7. Measurement of atherosclerosis and vulnerable plaque
There is robust evidence that aging is a major risk factor for the development of atherosclerotic plaque and plaque vulnerability (197).
Measurement of coronary artery plaque severity and composition has been assessed invasively using coronary angiography, IVUS and IV OCT. There have been a few large cohort studies utilising IVUS to assess plaque characteristics associated with age. A study of 1,009 Korean patients identified severe calcifications and negative remodelling in more elderly patients with CAD, and more unstable plaque morphology in younger patients (198). Another study of 990 patients demonstrated an association between age and the percentage of the calcium and lipid components of plaque (199). A third study of 697 reinforced these findings and also found higher rates of TCFA in patients over the age of 65 (200). IVUS has also been utilised in multiple RCTs of hundreds of patients investigating the impact of statin therapy on coronary atherosclerosis burden (201–203). IV OCT has been demonstrated to be superior than IVUS in quantifying coronary calcium and characteristics including thickness, area and volume due to its greater penetration (204). However, all these methods are difficult to use in population level community-based assessments of vascular age, given the invasive nature of the procedures and associated risks.
The most common non-invasive method of assessing coronary artery pathophysiology associated with aging is the measurement of coronary artery calcium deposition using a harmonised coronary artery calcium score. This is a standardised, non-invasive and low radiation study without contrast, providing a score reflecting coronary atherosclerotic burden that is strongly prognostic of future cardiovascular and coronary artery related events (205). CAC scoring using CTCA is widely available and relatively cost-effective in patients with suspected atherosclerosis (206–208). It also has the major advantages of being non-invasive, and able to visualise the vessel wall and high-risk positive remodelling rather than just luminal stenosis as may be assessed by invasive coronary angiography. The latter is particularly important when defining “healthy” aging, as diffuse non-obstructive CAD may not be well-appreciated by traditional invasive angiography (209). CAC scoring has been shown across multiple cohort studies including the Rotterdam Study [HR: 2.4 (95% CI 1.3–4.5)] for CAC 401–1,000 compared to CAC 0–100, MESA [HR: 3.61 (95% CI 1.96–6.65) for doubling of the CAC score], and the CAC consortium [HR: 1.45 (95% CI: 1.15–1.83) for CAC 1–100 vs. CAC 0] to be associated with an increased risk of adverse cardiovascular events (210–214), irrespective of ethnicity and independent of age, sex and traditional risk factors. This association was initially confirmed in middle-aged and older adults, and is also supported in adults as young as 30 years old by a significant association between CAC score and CVD mortality [HR for CAC > 100 vs. CAC 0: 3.3 (95% CI 1.8–6.2)] (215). CAC has also been demonstrated to add predictive value above traditional risk factors in the MESA [NRI: 0.25 (95% CI 0.16–0.34, p < 0.001)] and Heinz Nixdorf Recall (NRI: 0.224, p = 0.0009) studies (205, 207). Given this strong association with outcomes, randomised screening trials are now looking at the viability of using CAC to risk stratify patients for adverse coronary events instead of traditional risk scores, however the longer term outcome data is yet to be published (216, 217). Advances in high resolution CT have enabled measurement of coronary artery lumen and vessel wall dimensions as well as characterization of plaque composition (218, 219). This may enable more precise identification of patient phenotypes that are resilient or susceptible to the pathophysiological effects of aging on the vasculature through identification and quantification of vulnerable plaque and TCFA (207).
3.8. Risk factors of vascular aging
In the context of the heterogeneous speed of vascular aging across the population (220), it is important to understand the risk factors of vascular aging which may help in the identification of phenotypic groups. Non-modifiable risk factors that have been identified outside of chronological age include sex, ethnicity, family history, genetic factors and prenatal fetal growth (15, 221). Younger women have been observed to have slower rates of vascular aging, however, experience an increase in the rate of vascular aging in their 60’s as opposed to in the 70’s for men (220). This earlier increase has been thought to be associated with the onset of menopause (222). The sex differences in vascular aging have been shown to differ across vascular beds, highlighting the need for clearly defined measurement targets and assessment tools for localized vascular aging, including in the coronary arteries (222).
There are a wide range of modifiable risk factors that have been associated with early vascular aging including traditional cardiovascular risk factors (223–227), and there is also emerging evidence of association with depression in men and individual deprivation (228, 229). Individual risk factors do not necessarily affect all of the pathophysiological manifestations of vascular aging homogeneously, as the CRAVE study demonstrated that patients with hypertension and dyslipidemia had a four times higher rate of progression of cfPWV than those without, but nil effect on endothelial dysfunction as measured by FMD (230). Longitudinal data has also demonstrated the impact of early life pathology and even fetal stressors on vascular aging (231), and how ongoing exposure to risk factors can mediate its trajectory. In one study, metabolic syndrome in children and adolescents predicted arterial stiffness in middle aged adults (232). Another recent longitudinal study examining the trajectories of vascular aging as measured by a range of functional and structural indicators including CMIT and cfPWV found that lifestyle risk factors including smoking, diet, step count, BMI and HbA1c were associated with the trajectory of vascular aging over 11 years (220). They also reported a significant association between grip and leg strength and an increase in CIMT, and trunk flexibility and decreased cfPWV (220), which is consistent with prior studies (233, 234). Habitual aerobic exercise has also been demonstrated to mitigate the decline in endothelial function with age (235), however the effect of resistance or anaerobic training is more unclear.
Defining risk factors that are associated with coronary arterial aging is difficult with the currently available non-invasive assessment options, as it is difficult to delineate risk factors of atherosclerosis as an independent disease entity or as a marker of vascular aging. The heterogeneous effect of different risk factors on the clinically measurable manifestations of vascular aging highlight how characterization of indices of localized vascular aging in the coronaries outside of atherosclerosis is important in further understanding the disease process. Thus, incorporating novel, widely available, non-invasive measures such as CT FAI is integral in being able to perform assessments of the impact of risk factors of coronary arterial aging.
4. Measurement of coronary arterial age
Studies have used CTCA imaging data in different ways to assess the vascular age of the coronary arteries (121). The continuing absence of CAC has been used to define a healthy vascular aging phenotype in the Multi-Ethnic Study of Atherosclerosis (13) and Bogalusa Heart Study (12). McClelland et al. proposed a method of transforming the measurement of CAC from Agaston units into an arterial age, which they defined as the age at which the estimated coronary heart disease risk is the same for the observed CAC score (236). They demonstrated that using this CAC-derived arterial age instead of observed age resulted in a Framingham Risk Score that was more predictive of short-term incident coronary events based on data from the Multi-Ethnic Study of Atherosclerosis (236). Further studies using this calculation have also demonstrated that adding CAC-derived arterial age to a predictive model for stress-induced myocardial ischemia based on clinical data resulted in a higher net benefit than using chronological age (237). Another study however has suggested using an age adjusted segment involvement score as a surrogate marker of vascular age, which was demonstrated to have an incremental prognostic value to traditional risk factor evaluation based on data from the Coronary CT Angiography Evaluation for Clinical Outcomes: An International Multicentre study (238).
Expanding the assessment of coronary arterial age beyond atherosclerosis by incorporating other features of vascular aging is becoming possible with advances in imaging resolution and analysis. Whilst IVUS has been used to identify specific age-associated plaque morphology, these are yet to be incorporated into an assessment of coronary arterial age, given the necessity for an invasive procedure. CT FAI has made possible assessment of inflammation in the coronary arteries, however a standardised system of scoring has only recently been developed. Initial cohort studies of this scoring system demonstrate an increase in the FAI-score with age (239), as would be expected with the inflammation associated with vascular aging. Advanced imaging analysis (240) can also allow us to identify salient features on CTCA that are associated with aging and explore any prognostic significance they may have in being incorporated into an assessment of coronary arterial age.
5. Therapeutics of vascular aging
Currently available pharmacological therapies for vascular aging focus primarily on the effect on pulse wave velocity, as it the most common way vascular aging is measured and defined (15). Some studies have also assessed response via measurement of endothelial function through FMD (241, 242). However, there is limited evidence for using a reduction in cfPWV as a treatment target in hypertensive or diabetic populations, and no evidence to support therapies in non-hypertensive or non-diabetic populations (15). Whilst many assessments of pharmacological therapies have assessed their effect on major adverse cardiovascular events, there has been limited assessment of their effectiveness on vascular aging in the coronary arteries specifically. However, the proliferation of CTCA as a common non-invasive imaging modality and the addition of FAI for post imaging analysis provides opportunities to measure the subclinical vascular aging response to therapy in the coronary arteries. Formalising this imaging modality as a method to measure and define phenotypes of normal and abnormal coronary arterial aging will help to better allocate pharmacotherapy to prevent or manage vascular aging, similar to atherosclerotic coronary artery disease (243).
5.1. Non-pharmacological management
Multiple studies have assessed non-pharmacological methods of reducing arterial stiffness, mainly looking at the effects of exercise, caloric restriction and a reduction in salt consumption (15). Habitual aerobic exercise has been postulated to protect the vasculature from age associated changes by increasing nitrous oxide availability in response to increased laminar shear stress (244), improving mitochondrial biogenesis and anti-oxidant enzyme production (245), and stimulating both the production and release of endothelial progenitor cells from the bone marrow (246, 247). A recent meta-analysis demonstrated that aerobic exercise of greater than 4 weeks significantly improved central arterial PWV (pooled mean difference [PMD]: −0.47 m/s [95% CI −0.68 to −0.25]), and this is supported by sub-group analysis of a further meta-analysis, which found the same for aerobic exercise in healthy (weighted mean difference [WMD]: −0.41 m/s [95% CI −0.55 to −0.27]) and hypertensive adults [weighted mean difference: −0.66 m/s (95% CI −1.23 to −0.10)] (248, 249).
The evidence of the effects of resistance or anaerobic exercise on arterial stiffness is more unclear. One meta-analysis of resistance exercise found it made no difference to PWV [WMD: −0.04 m/s (95% CI −0.42 to 0.34)], and this was consistent after sub-group analysis by participant and study characteristics (249). However, another meta-analysis found that resistance exercise increased cf-PWV [WMD 0.42 m/s (95% CI: 0.17–0.66)] (250). The potential negative effect of acute resistance exercise has been postulated to be due to greater eccentric muscle damage causing secretion of inflammatory products (250) and angiotensin II (251), which mediate endothelial cell signaling and dilatation. Another study suggested that intense resistance exercise may increase sympathetic nervous system activity and therefore chronically increase adrenergic vasoconstrictor tone and arterial stiffness (252).
Regarding dietary sodium, a meta-analysis of eleven studies demonstrated that an average daily salt reduction of 5.2 g results in a PMD of −2.8% (95% CI −5.08 to −0.51) in cfPWV (253). Caloric restriction has also been investigated as a non-pharmacological intervention to ameliorate vascular aging, given the robust evidence that it extends lifespan in multiple animal models (254). Caloric restriction in mouse models has been demonstrated to enhance nitrous oxide bioavailability, prevent age-induced endothelial dysfunction (241), and reduce oxidative stress (255). Short term weight loss based on caloric restriction in overweight and obese adults has been demonstrated to reduce cfPWV (−187 ± 29 cm/s in intervention group vs. 15 ± 42 cm/s in control group), however it is difficult to assess to what degree the effect is mediated by weight loss as opposed to the dietary change itself (256–258). However, there has been limited research into longer-term caloric restriction due to adherence issues and potential adverse effects (259). A 2 year RCT of caloric restriction in humans demonstrated a decrease in F2-isoprostanes which are an index of accumulated oxidative injury (260, 261). However, this study did not include a direct measure of vascular aging. Further characterization of the beneficial impacts of these non-pharmacological interventions on coronary arterial aging may help both identification of resilient and susceptible individuals based on their lifestyle habits and exploration of novel therapeutic targets by identifying the pathways through which these interventions mediate the pathophysiological process of vascular aging.
5.2. Anti-hypertensive agents
Much of the literature to date has focused on the efficacy of different classes of anti-hypertensives in attenuating vascular aging (262), including angiotensin converting enzyme (ACE) inhibitors, angiotensin receptor blockers, beta blockers, calcium channel blockers, nitrates and diuretics and aldosterone antagonists. Whilst there is evidence suggesting that all the classes can reduce arterial stiffening as measured by PWV, ACE inhibitors which mediate the renin-angiotensin-aldosterone-system (RAAS) have been suggested to be the most effective given that they change the intrinsic properties of the arterial wall (262–266). A recent meta-analysis has also demonstrated that this effect from ACE inhibitors [PMD: −1.69 m/s (95% CI −2.05 to −1.33)] is independent of blood pressure reduction (263), through a direct influence on arterial wall structure, a reduction in oxidative stress and mediation of the pro-inflammatory state elucidated above (267). Further evidence is also emerging about the benefits of RAAS blockade in slowing aging via downregulation of mechanistic target of rapamycin and modulation of sirtuin expression (268).
5.3. Lipid-lowering agents
Statins have been demonstrated to have a benefit in preventing CVD events in moderate risk populations or those with a history of CVD [pooled RR: 0.72 (95% CI 0.64–0.81)] (269). This effect is primarily through a reduction in the amount of circulating low density lipoprotein (LDL), however there is also evidence that statins mediate inflammation independently of this as well (270). PCSK9 inhibitors can further improve outcomes in patients [pooled RR: 0.83 (95% CI 0.78–0.88)] already treated with standard therapy via further reduction of circulating LDL (271, 272). In patients who have signs of coronary arterial aging in the form of atherosclerosis as measured by CTCA, statins have been demonstrated to reduce major adverse cardiovascular events adjusted for traditional risk factors, age, sex and atrial fibrillation [HR: 0.76, (95% CI 0.60–0.95)], with the benefit being related to the degree of CAC (273). Numerous trials have also demonstrated that statins also confer a reduction in cfPWV in obese middle aged and older adults (treatment group: −163 ± 21 cm/s vs. control group: −48 ± 34 cm/s), independent of any changes in blood pressure (259, 274).
5.4. Diabetic agents
Whilst the evidence around the effect of glucose lowering on vascular outcomes in patients with type 2 diabetes is mixed (275, 276), certain classes of diabetic agents including metformin, GLP-1 agonists and DPP-4 inhibitors, have been demonstrated to improve mediate vascular aging through improving endothelial function and arterial stiffness, independent of glucose control (277–279). A recent meta-analysis demonstrated that SGLT-2 inhibitors significantly improve endothelial function as measured by FMD [PMD: 0.95% (95% CI 0.18–1.73)], and DPP-4 inhibitors [PMD: −0.18 m/s (95% CI −0.3 to −0.07)], SGLT-2 inhibitors [PMD: −1.30 m/s (95% CI −2.41 to −0.19)] and GLP-1 receptor agonists [PMD: −1.97 m/s (95% CI −2.65 to −1.30)] significantly decrease arterial stiffness in type 2 diabetic patients as measured by PWV (242, 278). There has been limited exploration of these novel diabetic agents in patients demonstrating coronary arterial aging and subclinical coronary atherosclerosis, however a pooled cohort analysis demonstrated that the effect modification of GLP-1 agonists on major adverse cardiovascular events was mediated by the degree of subclinical atherosclerosis (280).
5.5. Colchicine
Colchicine is a drug derived from the Colchicum Autumnale plant with notable anti-inflammatory effects through the inhibition of tubulin polymerization and microtubule generation (281). It is routinely used for the treatment of gout, familial Mediterranean fever and pericarditis (282). Given the significant role of inflammation in the development of atherosclerosis, the use of colchicine in the prevention of CVD events has been studied in three large RCTs. The LoDoCo and LoDoCo2 trials looked at the efficacy of colchicine in preventing major adverse cardiovascular events in patients with stable CAD (283, 284). Both the LoDoCo [HR: 0.33 (95% CI: 0.18–0.59); p < 0.0001] and LoDoCo2 [HR: 0.69 (95% CI: 0.57–0.83); P < 0.001] trial demonstrated that 0.5 mg of colchicine daily significantly decreased the risk of coronary events (285). The COLCOT trial explored if the impact of commencing colchicine in patients with a myocardial infarction on cardiovascular events. It found that there was a significant reduction in cardiovascular events over a median of 22.6 months [HR: 0.77 (95% CI: 0.61–0.96); p = 0.02] in patients who were commenced on 0.5 mg of colchicine daily within thirty days of their myocardial infarction (286).
There have also been small RCTs exploring the impact of colchicine on high sensitivity c-reactive protein (hs-CRP) in patients after an acute coronary syndrome, with mixed results. One study of 80 patients looked at the impact of giving patients 1 mg of colchicine daily to patients post acute coronary syndrome or stroke for thirty days and found no difference in hs-CRP (p = 0.22). However, another study of 80 patients looked at the impact of 0.5 mg of colchicine daily for 1 year and demonstrated a significant reduction in hs-CRP (treatment group: −37.3% vs. control group: −14.6%, p < 0.0001). To date, there have been no clinical trials looking at the effects of colchicine on PCAT inflammation, however this may change as CT FAI becomes more widespread.
5.6. Novel agents
The increasing focus on unravelling the mechanisms which drive vascular aging has resulted in testing of a range of novel therapeutic targets. These include senolytics, micro-RNA therapy, sirtuin activators, mTOR inhibitors, PPAR-gamma activators, antifibrotic agents and anti-inflammatory cytokine therapies (259, 266). Whilst many of these agents have clear therapeutic targets which are implicated in vascular aging, this has yet to be translated into clinical studies in human populations (259). mTOR inhibitors such as sirolimus and anti-proinflammatory cytokine therapies including tumor necrosis factor-alpha antagonists have demonstrated reductions in cfPWV in specific disease populations in which they are currently used (287–290). The CANTOS study looking at the effect of the IL-1β monoclonal antibody canakinumab also showed a reduction in major cardiovascular events with a three monthly 150 mg dose in patients with previous myocardial infarction [HR: 0.85 (95% CI 0.74–0.98)], providing support for further investigation of anti-proinflammatory therapies in patients with coronary arterial aging (291). Modulators of the sirtuin pathway including reservatrol and nicotinamide have shown some promise in animal models (292, 293), but clinical studies in human populations are scant (294). Similarly, senolytic and micro-RNA therapies have yet to be translated across to clinical trials in human populations, although a recent mouse model of senolytic therapy demonstrated senescent cells as a driver of the pro-inflammatory milieu, and a reduction in plaque growth and maladaptive plaque remodelling associated with treatment through transgenic and pharmacological approaches (295). Conversely, another recent study demonstrated that genetic senolysis of VSMCs did not change plaque size or composition, and pharmacological senolysis reduced atherogenesis in culture and in vivo, and senescent VSMCs in culture alone (296). There is currently no evidence regarding the effect of sirtuin modulators or senolytics on coronary arterial aging specifically, and thus it is critical to have a clearly defined biomarker of vascular aging for the coronary arteries to facilitate further study in this area Table 2.
6. Conclusion
The continuing demographic trend towards an aging population has driven a concerted effort to define the pathophysiological mechanisms by which aging changes the structure and function of the vasculature. Manifestations of these mechanisms including increased arterial stiffness, intimal thickening and the absence of atherosclerosis have started to be incorporated into definitions of phenotypes of early or healthy vascular aging. Currently, these definitions are not yet unified, but do not often involve any assessment of the coronary arteries as their location and size results in measurement limitations of processes of vascular aging.
Advancements in CTCA have enabled more detailed measurement of atherosclerosis and of localised coronary artery inflammation. Given its widespread availability and accessibility it is the optimal imaging modality for assessment of vascular aging in the coronaries in large epidemiological and cohort studies. Recognising these measurement modalities as a formal assessment of coronary arterial aging is essential in guiding therapeutic trials, as they provide the endpoints and criteria for how efficacy of therapy is assessed. Forming a consensus around the criteria to define healthy and early vascular aging phenotypes in the coronary arteries is the first step in a more detailed analysis of how age driven vascular changes mediate resilience and susceptibility specifically to CAD. It will provide a framework to identify the mechanisms and conditions where age-driven changes in the vasculature increase the risk of developing CAD as opposed to representing physiological aging. Furthermore, it will help to identify novel therapeutic targets to modulate this risk based on the observed phenotypes of resilience and susceptibility.
Defining the phenotypes of resilience and susceptibility to vascular aging in the coronary arteries will facilitate exploration of novel risk factors and blood-based biomarkers that define these cohorts. This is made possible by recent advances in high throughput multi-omic analysis platforms and increased access to a wide range of patient data through large cohort studies and healthy aging biobanks. Furthermore, developments in functional and anatomical phenotyping of coronary artery pathophysiology, and clearer measures of angiographically “normal” vessels make it feasible to design discovery efforts to unravel the missing mechanisms of resilience and susceptibility to arterial aging. These advances and developments in culmination will help to facilitate unbiased approaches to identifying new blood-based markers of resilience and susceptibility to CAD and coronary events.
Author contributions
GF, DC and RK contributed to the conception of the review. DC wrote the first draft of the manuscript. All authors contributed to the rewriting and editing of sections of the manuscript. All authors contributed to manuscript revision, read and approved the submitted version. All authors contributed to the article and approved the submitted version.
Funding
GF is supported by a National Health and Medical Research Council Practitioner Fellowship (GNT11359290), Heart Research Australia, and the New South Wales Office of Health and Medical Research. SG is supported by the Parker-Hughes Bequest, the New South Wales Office of Health and Medical Research, and the Frecker Family. RC is supported by the National Health and Medical Research Council of Australia (reference: 2009005) and by a National Heart Foundation Future Leader Fellowship (reference: 105636).
Conflict of interest
GF reports personal consulting fees from CSL, Novartis and grants from Abbott Diagnostic outside the submitted work. In addition, GF has a patent in biomarkers and oxidative stress awarded May 2017 in USA (US9638699B2), which was issued to the Northern Sydney Local Health District.
The remaining authors declare that the research was conducted in the absence of any commercial or financial relationships that could be construed as a potential conflict of interest.
Publisher's note
All claims expressed in this article are solely those of the authors and do not necessarily represent those of their affiliated organizations, or those of the publisher, the editors and the reviewers. Any product that may be evaluated in this article, or claim that may be made by its manufacturer, is not guaranteed or endorsed by the publisher.
References
1. D’Agostino RB, Vasan RS, Pencina MJ, Wolf PA, Cobain M, Massaro JM, et al. General cardiovascular risk profile for use in primary care. Circulation. (2008) 117(6):743–53. doi: 10.1161/CIRCULATIONAHA.107.699579
2. Dhingra R, Vasan RS. Age as a risk factor. Med Clin N Am. (2012) 96(1):87–91. doi: 10.1016/j.mcna.2011.11.003
3. Lakatta EG, Levy D. Arterial and cardiac aging: major shareholders in cardiovascular disease enterprises. Circulation. (2003) 107(1):139–46. doi: 10.1161/01.CIR.0000048892.83521.58
4. Najjar SS, Scuteri A, Lakatta EG. Arterial aging: is it an immutable cardiovascular risk factor? Hypertension. (2005) 46(3):454–62. doi: 10.1161/01.HYP.0000177474.06749.98
5. Vaduganathan M, Mensah George A, Turco Justine V, Fuster V, Roth Gregory A. The global burden of cardiovascular diseases and risk. J Am Coll Cardiol. (2022) 80(25):2361–71. doi: 10.1016/j.jacc.2022.11.005
6. Tsao CW, Aday AW, Almarzooq ZI, Anderson CAM, Arora P, Avery CL, et al. Heart disease and stroke statistics—2023 update: a report from the American heart association. Circulation. (2023) 147(8):e93–e621. doi: 10.1161/CIR.0000000000001123
7. Amini M, Zayeri F, Salehi M. Trend analysis of cardiovascular disease mortality, incidence, and mortality-to-incidence ratio: results from global burden of disease study 2017. BMC Public Health. (2021) 21(1):401. doi: 10.1186/s12889-021-10429-0
8. Fleg JL, Forman DE, Berra K, Bittner V, Blumenthal JA, Chen MA, et al. Secondary prevention of atherosclerotic cardiovascular disease in older adults. Circulation. (2013) 128(22):2422–46. doi: 10.1161/01.cir.0000436752.99896.22
9. Temple JB, McDonald PF. Population ageing and the labour force: 2000–2015 and 2015–2030. Australas J Ageing. (2017) 36(4):264–70. doi: 10.1111/ajag.12488
10. Liu Y, Chen K-j. Atherosclerosis, vascular aging and therapeutic strategies. Chin J Integr Med. (2012) 18(2):83–7. doi: 10.1007/s11655-012-0996-z
11. Laurent S. Defining vascular aging and cardiovascular risk. J Hypertens. (2012) 30:S3–S8. doi: 10.1097/HJH.0b013e328356a250
12. Razavi AC, Bazzano LA, He J, Krousel-Wood M, Chen J, Fernandez C, et al. Early contributors to healthy arterial aging versus premature atherosclerosis in young adults: the bogalusa heart study. J Am Heart Assoc. (2021) 10(12):e020774. doi: 10.1161/JAHA.121.020774
13. Whelton SP, Silverman MG, McEvoy JW, Budoff MJ, Blankstein R, Eng J, et al. Predictors of long-term healthy arterial aging: coronary artery calcium nondevelopment in the MESA study. JACC Cardiovasc Imaging. (2015) 8(12):1393–400. doi: 10.1016/j.jcmg.2015.06.019
14. Niiranen TJ, Lyass A, Larson MG, Hamburg NM, Benjamin EJ, Mitchell GF, et al. Prevalence, correlates, and prognosis of healthy vascular aging in a western community-dwelling cohort. Hypertension. (2017) 70(2):267–74. doi: 10.1161/HYPERTENSIONAHA.117.09026
15. Laurent S, Boutouyrie P, Cunha PG, Lacolley P, Nilsson PM. Concept of extremes in vascular aging. Hypertension. (2019) 74(2):218–28. doi: 10.1161/HYPERTENSIONAHA.119.12655
16. Lakatta EG. So! what’s aging? Is cardiovascular aging a disease? J Mol Cell Cardiol. (2015) 83:1–13. doi: 10.1016/j.yjmcc.2015.04.005
17. AlGhatrif M, Lakatta EG. The reality of aging viewed from the arterial wall. In: Safar ME, O’Rourke MF, Frohlich ED, editors. Blood pressure and arterial wall mechanics in cardiovascular diseases. London: Springer London (2014). p. 137–53.
18. Oikonomou EK, Marwan M, Desai MY, Mancio J, Alashi A, Hutt Centeno E, et al. Non-invasive detection of coronary inflammation using computed tomography and prediction of residual cardiovascular risk (the CRISP CT study): a post-hoc analysis of prospective outcome data. Lancet. (2018) 392(10151):929–39. doi: 10.1016/S0140-6736(18)31114-0
19. Gavish B, Izzo JL Jr. Arterial stiffness: going a step beyond. Am J Hypertens. (2016) 29(11):1223–33. doi: 10.1093/ajh/hpw061
20. Sehgel NL, Vatner SF, Meininger GA. “Smooth muscle cell stiffness syndrome”—revisiting the structural basis of arterial stiffness. Front Physiol. (2015) 6:335. doi: 10.3389/fphys.2015.00335
21. Bennett MR, Sinha S, Owens GK. Vascular smooth muscle cells in atherosclerosis. Circ Res. (2016) 118(4):692–702. doi: 10.1161/CIRCRESAHA.115.306361
22. Lacolley P, Regnault V, Avolio AP. Smooth muscle cell and arterial aging: basic and clinical aspects. Cardiovasc Res. (2018) 114(4):513–28. doi: 10.1093/cvr/cvy009
23. Jacob MP. Extracellular matrix remodeling and matrix metalloproteinases in the vascular wall during aging and in pathological conditions. Biomed Pharmacother. (2003) 57(5-6):195–202. doi: 10.1016/S0753-3322(03)00065-9
24. Lacolley P, Regnault V, Segers P, Laurent S. Vascular smooth muscle cells and arterial stiffening: relevance in development, aging, and disease. Physiol Rev. (2017) 97(4):1555–617. doi: 10.1152/physrev.00003.2017
25. Ungvari Z, Tarantini S, Donato AJ, Galvan V, Csiszar A. Mechanisms of vascular aging. Circ Res. (2018) 123(7):849–67. doi: 10.1161/CIRCRESAHA.118.311378
26. Li Z, Froehlich J, Galis ZS, Lakatta EG. Increased expression of matrix metalloproteinase-2 in the thickened intima of aged rats. Hypertension. (1999) 33(1):116–23. doi: 10.1161/01.HYP.33.1.116
27. Lakatta EG. Arterial and cardiac aging: major shareholders in cardiovascular disease enterprises: part III: cellular and molecular clues to heart and arterial aging. Circulation. (2003) 107(3):490–7. doi: 10.1161/01.CIR.0000048894.99865.02
28. Van Doren SR. Matrix metalloproteinase interactions with collagen and elastin. Matrix Biol. (2015) 44-46:224–31. doi: 10.1016/j.matbio.2015.01.005
29. Jiang L, Zhang J, Monticone RE, Telljohann R, Wu J, Wang M, et al. Calpain-1 regulation of matrix metalloproteinase 2 activity in vascular smooth muscle cells facilitates age-associated aortic wall calcification and fibrosis. Hypertension. (2012) 60(5):1192–9. doi: 10.1161/HYPERTENSIONAHA.112.196840
30. Gaballa MA, Jacob CT, Raya TE, Liu J, Simon B, Goldman S. Large artery remodeling during aging. Hypertension. (1998) 32(3):437–43. doi: 10.1161/01.HYP.32.3.437
31. Michel JB, Heudes D, Michel O, Poitevin P, Philippe M, Scalbert E, et al. Effect of chronic ANG I-converting enzyme inhibition on aging processes. II. Large arteries. Am J Physiol. (1994) 267(1):R124–35. doi: 10.1152/ajpregu.1994.267.1.R124
32. Watanabe M, Sawai T, Nagura H, Suyama K. Age-related alteration of cross-linking amino acids of elastin in human aorta. Tohoku J Exp Med. (1996) 180(2):115–30. doi: 10.1620/tjem.180.115
34. Duca L, Blaise S, Romier B, Laffargue M, Gayral S, El Btaouri H, et al. Matrix ageing and vascular impacts: focus on elastin fragmentation. Cardiovasc Res. (2016) 110(3):298–308. doi: 10.1093/cvr/cvw061
35. Oberoi S, Schoepf UJ, Meyer M, Henzler T, Rowe GW, Costello P, et al. Progression of arterial stiffness and coronary atherosclerosis: longitudinal evaluation by cardiac CT. Am J Roentgenol. (2013) 200(4):798–804. doi: 10.2214/AJR.12.8653
36. Palombo C, Kozakova M. Arterial stiffness, atherosclerosis and cardiovascular risk: pathophysiologic mechanisms and emerging clinical indications. Vasc Pharmacol. (2016) 77:1–7. doi: 10.1016/j.vph.2015.11.083
37. Piccirillo F, Carpenito M, Verolino G, Chello C, Nusca A, Lusini M, et al. Changes of the coronary arteries and cardiac microvasculature with aging: implications for translational research and clinical practice. Mech Ageing Dev. (2019) 184:111161. doi: 10.1016/j.mad.2019.111161
38. Olsen MH, Angell SY, Asma S, Boutouyrie P, Burger D, Chirinos JA, et al. A call to action and a lifecourse strategy to address the global burden of raised blood pressure on current and future generations: the lancet commission on hypertension. Lancet. (2016) 388(10060):2665–712. doi: 10.1016/S0140-6736(16)31134-5
39. O’Leary DHMD, Polak JFMDMPH, Kronmal RAP, Manolio TAMDMHS, Burke GLMDMS, Wolfson SKJMD. Carotid-artery intima and media thickness as a risk factor for myocardial infarction and stroke in older adults. N Engl J Med. (1999) 340(1):14–22. doi: 10.1056/NEJM199901073400103
40. Scioli MG, Bielli A, Arcuri G, Ferlosio A, Orlandi A. Ageing and microvasculature. Vasc Cell. (2014) 6(1):1–15. doi: 10.1186/2045-824X-6-19
41. Mitchell GF. Effects of central arterial aging on the structure and function of the peripheral vasculature: implications for end-organ damage. J Appl Physiol. (2008) 105(5):1652–60. doi: 10.1152/japplphysiol.90549.2008
42. Orlandi A, Bochaton-Piallat M-L, Gabbiani G, Spagnoli LG. Aging, smooth muscle cells and vascular pathobiology: implications for atherosclerosis. Atherosclerosis. (2006) 188(2):221–30. doi: 10.1016/j.atherosclerosis.2006.01.018
43. Fu Z, Wang M, Gucek M, Zhang J, Wu J, Jiang L, et al. Milk fat globule protein epidermal growth factor-8: a pivotal relay element within the angiotensin II and monocyte chemoattractant protein-1 signaling cascade mediating vascular smooth muscle cells invasion. Circ Res. (2009) 104(12):1337–46. doi: 10.1161/CIRCRESAHA.108.187088
44. Virmani R, Kolodgie FD, Burke AP, Farb A, Schwartz SM. Lessons from sudden coronary death: a comprehensive morphological classification scheme for atherosclerotic lesions. Arterioscler Thromb Vasc Biol. (2000) 20(5):1262–75. doi: 10.1161/01.ATV.20.5.1262
45. Stary HC. Macrophages, macrophage foam cells, and eccentric intimal thickening in the coronary arteries of young children. Atherosclerosis. (1987) 64(2):91–108. doi: 10.1016/0021-9150(87)90234-6
46. Movat HZ, More RH, Haust MD. The diffuse intimal thickening of the human aorta with aging. Am J Pathol. (1958) 34(6):1023–31.13583094
47. Nakashima Y, Wight TN, Sueishi K. Early atherosclerosis in humans: role of diffuse intimal thickening and extracellular matrix proteoglycans. Cardiovasc Res. (2008) 79(1):14–23. doi: 10.1093/cvr/cvn099
48. Virmani R, Kolodgie FD, Burke AP, Farb A, Schwartz SM. Lessons from sudden coronary death. Arterioscler, Thromb, Vasc Biol. (2000) 20(5):1262–75. doi: 10.1161/01.ATV.20.5.1262
49. Spagnoli LG, Orlandi A, Mauriello A, De Angelis C, Ramacci MT. Age-dependent increase of rabbit aortic atherosclerosis a morphometric approach. Pathol Res Pract. (1992) 188(4):637–42. doi: 10.1016/S0344-0338(11)80071-3
50. Spagnoli LG, Orlandi A, Mauriello A, Santeusanio G, de Angelis C, Lucreziotti R, et al. Aging and atherosclerosis in the rabbit: 1. Distribution, prevalence and morphology of atherosclerotic lesions. Atherosclerosis. (1991) 89(1):11–24. doi: 10.1016/0021-9150(91)90003-L
51. Lorenz MW, Markus HS, Bots ML, Rosvall M, Sitzer M. Prediction of clinical cardiovascular events with carotid intima-media thickness: a systematic review and meta-analysis. Circulation. (2007) 115(4):459–67. doi: 10.1161/CIRCULATIONAHA.106.628875
52. van den Munckhof IC, Jones H, Hopman MT, de Graaf J, Nyakayiru J, van Dijk B, et al. Relation between age and carotid artery intima-medial thickness: a systematic review. Clin Cardiol. (2018) 41(5):698–704. doi: 10.1002/clc.22934
53. Ross R. Atherosclerosis—an inflammatory disease. N Engl J Med. (1999) 340(2):115–26. doi: 10.1056/NEJM199901143400207
54. Antonopoulos AS, Sanna F, Sabharwal N, Thomas S, Oikonomou EK, Herdman L, et al. Detecting human coronary inflammation by imaging perivascular fat. Sci Transl Med. (2017) 9(398):eaal2658. doi: 10.1126/scitranslmed.aal2658
55. Wang M, Jiang L, Monticone RE, Lakatta EG. Proinflammation: the key to arterial aging. Trends Endocrinol Metab. (2014) 25(2):72–9. doi: 10.1016/j.tem.2013.10.002
56. Wang M, Khazan B, Lakatta E G. Central arterial aging and angiotensin II signaling. Curr Hypertens Rev. (2010) 6(4):266–81. doi: 10.2174/157340210793611668
57. Goel A, Su B, Flavahan S, Lowenstein CJ, Berkowitz DE, Flavahan NA. Increased endothelial exocytosis and generation of endothelin-1 contributes to constriction of aged arteries. Circ Res. (2010) 107(2):242–51. doi: 10.1161/CIRCRESAHA.109.210229
58. Wang M, Monticone RE, Lakatta EG. Arterial aging: a journey into subclinical arterial disease. Curr Opin Nephrol Hypertens. (2010) 19(2):201–7. doi: 10.1097/MNH.0b013e3283361c0b
59. Lakatta EG, Wang M, Najjar SS. Arterial aging and subclinical arterial disease are fundamentally intertwined at macroscopic and molecular levels. Med Clin N Am. (2009) 93(3):583–604. doi: 10.1016/j.mcna.2009.02.008
60. Lakatta EG. The reality of aging viewed from the arterial wall. Artery Res. (2013) 7(2):73–80. doi: 10.1016/j.artres.2013.01.003
61. Csiszar A, Labinskyy N, Smith K, Rivera A, Orosz Z, Ungvari Z. Vasculoprotective effects of anti-tumor necrosis factor-α treatment in aging. Am J Pathol. (2007) 170(1):388–98. doi: 10.2353/ajpath.2007.060708
62. Wang M, Zhang J, Jiang L-Q, Spinetti G, Pintus G, Monticone R, et al. Proinflammatory profile within the grossly normal aged human aortic wall. Hypertension. (2007) 50(1):219–27. doi: 10.1161/HYPERTENSIONAHA.107.089409
63. Arenas IA, Xu Y, Davidge ST. Age-associated impairment in vasorelaxation to fluid shear stress in the female vasculature is improved by TNF-α antagonism. Am J Physiol Heart Circ Physiol. (2006) 290(3):H1259–H63. doi: 10.1152/ajpheart.00990.2005
64. Ungvari Z, Kaley G, de Cabo R, Sonntag WE, Csiszar A. Mechanisms of vascular aging: new perspectives. J Gerontol A Biol Sci Med Sci. (2010) 65A(10):1028–41. doi: 10.1093/gerona/glq113
65. Dikalov S. Cross talk between mitochondria and NADPH oxidases. Free Radic Biol Med. (2011) 51(7):1289–301. doi: 10.1016/j.freeradbiomed.2011.06.033
66. Humphrey JD, Milewicz DM, Tellides G, Schwartz Martin A. Dysfunctional mechanosensing in aneurysms. Science. (2014) 344(6183):477–9. doi: 10.1126/science.1253026
67. Song Y, Shen H, Schenten D, Shan P, Lee PJ, Goldstein DR. Aging enhances the basal production of IL-6 and CCL2 in vascular smooth muscle cells. Arterioscler, Thromb, Vasc Biol. (2012) 32(1):103–9. doi: 10.1161/ATVBAHA.111.236349
68. Nurmi L, Heikkilä HM, Vapaatalo H, Kovanen PT, Lindstedt KA. Downregulation of bradykinin type 2 receptor expression in cardiac endothelial cells during senescence. J Vasc Res. (2012) 49(1):13–23. doi: 10.1159/000329615
69. Donato AJ, Magerko KA, Lawson BR, Durrant JR, Lesniewski LA, Seals DR. SIRT-1 and vascular endothelial dysfunction with ageing in mice and humans. J Physiol (Lond). (2011) 589(18):4545–54. doi: 10.1113/jphysiol.2011.211219
70. Ota H, Akishita M, Eto M, Iijima K, Kaneki M, Ouchi Y. Sirt1 modulates premature senescence-like phenotype in human endothelial cells. J Mol Cell Cardiol. (2007) 43(5):571–9. doi: 10.1016/j.yjmcc.2007.08.008
71. Muñoz-Espín D, Serrano M. Cellular senescence: from physiology to pathology. Nat Rev Mol Cell Biol. (2014) 15(7):482–96. doi: 10.1038/nrm3823
72. Rossman MJ, Kaplon RE, Hill SD, McNamara MN, Santos-Parker JR, Pierce GL, et al. Endothelial cell senescence with aging in healthy humans: prevention by habitual exercise and relation to vascular endothelial function. Am J Physiol Heart Circ Physiol. (2017) 313(5):H890–H5. doi: 10.1152/ajpheart.00416.2017
73. Minamino T, Miyauchi H, Yoshida T, Ishida Y, Yoshida H, Komuro I. Endothelial cell senescence in human atherosclerosis. Circulation. (2002) 105(13):1541–4. doi: 10.1161/01.CIR.0000013836.85741.17
74. Félétou M, Vanhoutte PM. Endothelial dysfunction: a multifaceted disorder (the wiggers award lecture). Am J Physiol Heart Circ Physiol. (2006) 291(3):H985–H1002. doi: 10.1152/ajpheart.00292.2006
75. Franceschi C, Bonafè M, Valensin S, Olivieri F, De Luca M, Ottaviani E, et al. Inflamm-aging: an evolutionary perspective on immunosenescence. Ann N Y Acad Sci. (2000) 908(1):244–54. doi: 10.1111/j.1749-6632.2000.tb06651.x
76. Ballard VL, Edelberg JM. Stem cells and the regeneration of the aging cardiovascular system. Circ Res. (2007) 100(8):1116–27. doi: 10.1161/01.RES.0000261964.19115.e3
77. Galley HF, Webster NR. Physiology of the endothelium. Br J Anaesth. (2004) 93(1):105–13. doi: 10.1093/bja/aeh163
78. Cines DB, Pollak ES, Buck CA, Loscalzo J, Zimmerman GA, McEver RP, et al. Endothelial cells in physiology and in the pathophysiology of vascular disorders. Blood. (1998) 91(10):3527–61. doi: 10.1161/01.RES.0000269183.13937.e8
79. Donato AJ, Eskurza I, Silver AE, Levy AS, Pierce GL, Gates PE, et al. Direct evidence of endothelial oxidative stress with aging in humans. Circ Res. (2007) 100(11):1659–66. doi: 10.1161/01.RES.0000269183.13937.e8
80. Csiszar A, Ungvari Z, Edwards JG, Kaminski P, Wolin MS, Koller A, et al. Aging-induced phenotypic changes and oxidative stress impair coronary arteriolar function. Circ Res. (2002) 90(11):1159–66. doi: 10.1161/01.RES.0000020401.61826.EA
81. Van Der Loo B, Labugger R, Skepper JN, Bachschmid M, Kilo J, Powell JM, et al. Enhanced peroxynitrite formation is associated with vascular aging. J Exp Med. (2000) 192(12):1731–44. doi: 10.1084/jem.192.12.1731
82. Celermajer DS, Sorensen KE, Spiegelhalter DJ, Georgakopoulos D, Robinson J, Deanfield JE. Aging is associated with endothelial dysfunction in healthy men years before the age-related decline in women. J Am Coll Cardiol. (1994) 24(2):471–6. doi: 10.1016/0735-1097(94)90305-0
83. Brandes RP, Fleming I, Busse R. Endothelial aging. Cardiovasc Res. (2005) 66(2):286–94. doi: 10.1016/j.cardiores.2004.12.027
84. Kubes P, Suzuki M, Granger D. Nitric oxide: an endogenous modulator of leukocyte adhesion. Proc Natl Acad Sci USA. (1991) 88(11):4651–5. doi: 10.1073/pnas.88.11.4651
85. Ganz P, Vita JA. Testing endothelial vasomotor function: nitric oxide, a multipotent molecule. Circulation. (2003) 108(17):2049–53. doi: 10.1161/01.CIR.0000089507.19675.F9
86. Moncada S. Nitric oxide: physiology, pathophysiology and pharmacology. Pharmacol Rev. (1991) 43:109–42.1852778
87. Levine AB, Punihaole D, Levine TB. Characterization of the role of nitric oxide and its clinical applications. Cardiology. (2012) 122(1):55–68. doi: 10.1159/000338150
88. Irace C, Carallo C, De Franceschi MS, Scicchitano F, Milano M, Tripolino C, et al. Human common carotid wall shear stress as a function of age and gender: a 12-year follow-up study. Age (Omaha). (2012) 34(6):1553–62. doi: 10.1007/s11357-011-9318-1
89. Bapir M, Untracht GR, Hunt JEA, McVey JH, Harris J, Skene SS, et al. Age-dependent decline in common femoral artery flow-mediated dilation and wall shear stress in healthy subjects. Life. (2022) 12(12):2023. doi: 10.3390/life12122023
90. Carallo C, Tripolino C, De Franceschi MS, Irace C, Xu XY, Gnasso A. Carotid endothelial shear stress reduction with aging is associated with plaque development in twelve years. Atherosclerosis. (2016) 251:63–9. doi: 10.1016/j.atherosclerosis.2016.05.048
91. Lantz J, Renner J, Länne T, Karlsson M. Is aortic wall shear stress affected by aging? An image-based numerical study with two age groups. Med Eng Phys. (2015) 37(3):265–71. doi: 10.1016/j.medengphy.2014.12.011
92. Peng Z, Shu B, Zhang Y, Wang M. Endothelial response to pathophysiological stress. Arterioscler Thromb Vasc Biol. (2019) 39(11):e233–e43. doi: 10.1161/ATVBAHA.119.312580
93. Gimbrone MA Jr, García-Cardeña G. Endothelial cell dysfunction and the pathobiology of atherosclerosis. Circ Res. (2016) 118(4):620–36. doi: 10.1161/CIRCRESAHA.115.306301
94. Chiu J-J, Chien S. Effects of disturbed flow on vascular endothelium: pathophysiological basis and clinical perspectives. Physiol Rev. (2011) 91(1):327–87. doi: 10.1152/physrev.00047.2009
95. Wentzel JJ, Chatzizisis YS, Gijsen FJH, Giannoglou GD, Feldman CL, Stone PH. Endothelial shear stress in the evolution of coronary atherosclerotic plaque and vascular remodelling: current understanding and remaining questions. Cardiovasc Res. (2012) 96(2):234–43. doi: 10.1093/cvr/cvs217
96. Donato AJ, Gano LB, Eskurza I, Silver AE, Gates PE, Jablonski K, et al. Vascular endothelial dysfunction with aging: endothelin-1 and endothelial nitric oxide synthase. Am J Physiol Heart Circ Physiol. (2009) 297(1):H425–H32. doi: 10.1152/ajpheart.00689.2008
97. Hansen B, Holtzman JN, Juszczynski C, Khan N, Kaur G, Varma B, et al. Ischemia with No obstructive arteries (INOCA): a review of the prevalence, diagnosis and management. Curr Probl Cardiol. (2023) 48(1):101420. doi: 10.1016/j.cpcardiol.2022.101420
98. Rafieian-Kopaei M, Setorki M, Doudi M, Baradaran A, Nasri H. Atherosclerosis: process, indicators, risk factors and new hopes. Int J Prev Med. (2014) 5(8):927–46.25489440
99. Wang J, Peng X, Lassance-Soares RM, Najafi AH, Alderman LO, Sood S, et al. Aging-Induced collateral dysfunction: impaired responsiveness of collaterals and susceptibility to apoptosis via dysfunctional eNOS signaling. J Cardiovasc Transl Res. (2011) 4(6):779–89. doi: 10.1007/s12265-011-9280-4
100. Clarke MC, Littlewood TD, Figg N, Maguire JJ, Davenport AP, Goddard M, et al. Chronic apoptosis of vascular smooth muscle cells accelerates atherosclerosis and promotes calcification and medial degeneration. Circ Res. (2008) 102(12):1529–38. doi: 10.1161/CIRCRESAHA.108.175976
101. Virmani R, Burke AP, Farb A, Kolodgie FD. Pathology of the vulnerable plaque. J Am Coll Cardiol. (2006) 47(8S):C13–C8. doi: 10.1016/j.jacc.2005.10.065
102. Clarke MC, Figg N, Maguire JJ, Davenport AP, Goddard M, Littlewood TD, et al. Apoptosis of vascular smooth muscle cells induces features of plaque vulnerability in atherosclerosis. Nat Med. (2006) 12(9):1075–80. doi: 10.1038/nm1459
103. McCrann D, Yang D, Chen H, Carroll S, Ravid K. Upregulation of Nox4 in the aging vasculature and its association with smooth muscle cell polyploidy. Cell Cycle. (2009) 8(6):902–8. doi: 10.4161/cc.8.6.7900
104. Yang D, McCrann DJ, Nguyen H, Hilaire CS, DePinho RA, Jones MR, et al. Increased polyploidy in aortic vascular smooth muscle cells during aging is marked by cellular senescence. Aging Cell. (2007) 6(2):257–60. doi: 10.1111/j.1474-9726.2007.00274.x
105. Wang JC, Bennett M. Aging and atherosclerosis. Circ Res. (2012) 111(2):245–59. doi: 10.1161/CIRCRESAHA.111.261388
106. Hayakawa M, Hattori K, Sugiyama S, Ozawa T. Age-associated oxygen damage and mutations in mitochondrial DNA in human hearts. Biochem Biophys Res Commun. (1992) 189(2):979–85. doi: 10.1016/0006-291X(92)92300-M
107. Hayakawa M, Torii K, Sugiyama S, Tanaka M, Ozawa T. Age-associated accumulation of 8-hydroxydeoxyguanosine in mitochondrial DNA of human diaphragm. Biochem Biophys Res Commun. (1991) 179(2):1023–9. doi: 10.1016/0006-291X(91)91921-X
108. Green DR, Galluzzi L, Kroemer G. Mitochondria and the autophagy–inflammation–cell death axis in organismal aging. Science. (2011) 333(6046):1109–12. doi: 10.1126/science.1201940
109. López-Otín C, Blasco MA, Partridge L, Serrano M, Kroemer G. The hallmarks of aging. Cell. (2013) 153(6):1194–217. doi: 10.1016/j.cell.2013.05.039
110. Tatarkova Z, Kuka S, Racay P, Lehotský J, Dobrota D, Mistuna D, et al. Effects of aging on activities of mitochondrial electron transport chain complexes and oxidative damage in rat heart. Physiol Res. (2011) 60(2):281. doi: 10.33549/physiolres.932019
111. Mercer JR, Cheng K-K, Figg N, Gorenne I, Mahmoudi M, Griffin J, et al. DNA Damage links mitochondrial dysfunction to atherosclerosis and the metabolic syndrome. Circ Res. (2010) 107(8):1021–31. doi: 10.1161/CIRCRESAHA.110.218966
112. Fujimoto H, Kobayashi H, Ohno M. Age-induced reduction in mitochondrial manganese superoxide dismutase activity and tolerance of macrophages against apoptosis induced by oxidized low density lipoprotein. Circ J. (2010) 74(2):353–60. doi: 10.1253/circj.CJ-09-0491
113. Yu E, Calvert PA, Mercer JR, Harrison J, Baker L, Figg NL, et al. Mitochondrial DNA damage can promote atherosclerosis independently of reactive oxygen species through effects on smooth muscle cells and monocytes and correlates with higher-risk plaques in humans. Circulation. (2013) 128(7):702–12. doi: 10.1161/CIRCULATIONAHA.113.002271
114. Seegers LM, Araki M, Nakajima A, Yonetsu T, Minami Y, Ako J, et al. Sex differences in culprit plaque characteristics among different age groups in patients with acute coronary syndromes. Circ: Cardiovasc Interventions. (2022) 15(6):e011612. doi: 10.1161/CIRCINTERVENTIONS.121.011612
115. Tucker WD, Arora Y, Mahajan K. Anatomy, blood vessels. Treasure Island (FL): StatPearls Publishing (2021).
116. Pfaltzgraff ER, Bader DM. Heterogeneity in vascular smooth muscle cell embryonic origin in relation to adult structure, physiology, and disease. Dev Dyn. (2015) 244(3):410–6. doi: 10.1002/dvdy.24247
117. Waller BF, Orr CM, Slack JD, Pinkerton CA, Van Tassel J, Peters T. Anatomy, histology, and pathology of coronary arteries: a review relevant to new interventional and imaging techniques—part I. Clin Cardiol. (1992) 15(6):451–7. doi: 10.1002/clc.4960150613
118. Jani B, Rajkumar C. Ageing and vascular ageing. Postgrad Med J. (2006) 82(968):357–62. doi: 10.1136/pgmj.2005.036053
119. McVeigh GE, Bratteli CW, Morgan DJ, Alinder CM, Glasser SP, Finkelstein SM, et al. Age-related abnormalities in arterial compliance identified by pressure pulse contour analysis. Hypertension. (1999) 33(6):1392–8. doi: 10.1161/01.HYP.33.6.1392
120. Khalil Y, Mukete B, Durkin MJ, Coccia J, Matsumura ME. A comparison of assessment of coronary calcium vs carotid intima media thickness for determination of vascular age and adjustment of the framingham risk score. Prev Cardiol. (2010) 13(3):117–21.20626666
121. Yurdadogan T, Malsch C, Kotseva K, Wood D, Leyh R, Ertl G, et al. Functional versus morphological assessment of vascular age in patients with coronary heart disease. Sci Rep. (2021) 11(1):18164. doi: 10.1038/s41598-021-96998-x
122. Miname MH, Bittencourt MS, Pereira AC, Jannes CE, Krieger JE, Nasir K, et al. Vascular age derived from coronary artery calcium score on the risk stratification of individuals with heterozygous familial hypercholesterolaemia. Eur Heart J Cardiovasc Imaging. (2020) 21(3):251–7. doi: 10.1093/ehjci/jez280
123. Laurent S, Marais L, Boutouyrie P. The noninvasive assessment of vascular aging. Can J Cardiol. (2016) 32(5):669–79. doi: 10.1016/j.cjca.2016.01.039
124. The Reference Values for Arterial Stiffness C. Determinants of pulse wave velocity in healthy people and in the presence of cardiovascular risk factors: ‘establishing normal and reference values’. Eur Heart J. 2010;31(19):2338–50. doi: 10.1093/eurheartj/ehq165
125. Vecsey-Nagy M, Szilveszter B, Kolossváry M, Boussoussou M, Vattay B, Merkely B, et al. Correlation between coronary artery calcium- and different cardiovascular risk score-based methods for the estimation of vascular age in Caucasian patients. J Clin Med. (2022) 11(4):1111. doi: 10.3390/jcm11041111
126. Laurent S, Cockcroft J, Van Bortel L, Boutouyrie P, Giannattasio C, Hayoz D, et al. Expert consensus document on arterial stiffness: methodological issues and clinical applications. Eur Heart J. (2006) 27(21):2588–605. doi: 10.1093/eurheartj/ehl254
127. Bossuyt J, Engelen L, Ferreira I, Stehouwer CD, Boutouyrie P, Laurent S, et al. Reference values for local arterial stiffness. Part B: femoral artery. J Hypertens. (2015) 33(10):1997–2009. doi: 10.1097/HJH.0000000000000655
128. Engelen L, Bossuyt J, Ferreira I, van Bortel LM, Reesink KD, Segers P, et al. Reference values for local arterial stiffness. Part A: carotid artery. J Hypertens. (2015) 33(10):1981–96. doi: 10.1097/HJH.0000000000000654
129. Davies JE, Whinnett ZI, Francis DP, Willson K, Foale RA, Malik IS, et al. Use of simultaneous pressure and velocity measurements to estimate arterial wave speed at a single site in humans. Am J Physiol Heart Circ Physiol. (2006) 290(2):H878–H85. doi: 10.1152/ajpheart.00751.2005
130. Aguado-Sierra J, Parker KH, Davies JE, Francis D, Hughes AD, Mayet J, editors. Arterial pulse wave velocity in coronary arteries. 2006 international conference of the IEEE engineering in medicine and biology society. New York: IEEE (2006) 30 Aug.-3 Sept. 2006. p. 867–70.
131. Herment A, Lefort M, Kachenoura N, De Cesare A, Taviani V, Graves MJ, et al. Automated estimation of aortic strain from steady-state free-precession and phase contrast MR images. Magn Reson Med. (2011) 65(4):986–93. doi: 10.1002/mrm.22678
132. Vlachopoulos C, Aznaouridis K, Stefanadis C. Prediction of cardiovascular events and all-cause mortality with arterial stiffness: a systematic review and meta-analysis. J Am Coll Cardiol. (2010) 55(13):1318–27. doi: 10.1016/j.jacc.2009.10.061
133. Ben-Shlomo Y, Spears M, Boustred C, May M, Anderson SG, Benjamin EJ, et al. Aortic pulse wave velocity improves cardiovascular event prediction: an individual participant meta-analysis of prospective observational data from 17,635 subjects. J Am Coll Cardiol. (2014) 63(7):636–46. doi: 10.1016/j.jacc.2013.09.063
134. Laurent S, Boutouyrie P, Asmar R, Gautier I, Laloux B, Guize L, et al. Aortic stiffness is an independent predictor of all-cause and cardiovascular mortality in hypertensive patients. Hypertension. (2001) 37(5):1236–41. doi: 10.1161/01.HYP.37.5.1236
135. Boutouyrie P, Tropeano AI, Asmar R, Gautier I, Benetos A, Lacolley P, et al. Aortic stiffness is an independent predictor of primary coronary events in hypertensive patients: a longitudinal study. Hypertension. (2002) 39(1):10–5. doi: 10.1161/hy0102.099031
136. Vlachopoulos C, Xaplanteris P, Aboyans V, Brodmann M, Cífková R, Cosentino F, et al. The role of vascular biomarkers for primary and secondary prevention. A position paper from the European society of cardiology working group on peripheral circulation: endorsed by the association for research into arterial structure and physiology (ARTERY) society. Atherosclerosis. (2015) 241(2):507–32. doi: 10.1016/j.atherosclerosis.2015.05.007
137. Asmar R, Benetos A, Topouchian J, Laurent P, Pannier B, Brisac A-M, et al. Assessment of arterial distensibility by automatic pulse wave velocity measurement. Hypertension. (1995) 26(3):485–90. doi: 10.1161/01.HYP.26.3.485
138. Cruickshank K, Riste L, Anderson SG, Wright JS, Dunn G, Gosling RG. Aortic pulse-wave velocity and its relationship to mortality in diabetes and glucose intolerance: an integrated index of vascular function? Circulation. (2002) 106(16):2085–90. doi: 10.1161/01.CIR.0000033824.02722.F7
139. Nilsson PM, Boutouyrie P, Laurent S. Vascular aging. Hypertension. (2009) 54(1):3–10. doi: 10.1161/HYPERTENSIONAHA.109.129114
140. Wadström BN, Fatehali A-AH, Engström G, Nilsson PM. A vascular aging index as independent predictor of cardiovascular events and total mortality in an elderly urban population. Angiology. (2019) 70(10):929–37. doi: 10.1177/0003319719857270
141. Morreale M, Mulè G, Ferrante A, D’ignoto F, Cottone S. Early vascular aging in normotensive patients with systemic lupus erythematosus: comparison with young patients having hypertension. Angiology. (2015) 67(7):676–82. doi: 10.1177/0003319715613917
142. Kume T, Akasaka T, Kawamoto T, Watanabe N, Toyota E, Neishi Y, et al. Assessment of coronary intima—media thickness by optical coherence tomography comparison with intravascular ultrasound. Circ J. (2005) 69(8):903–7. doi: 10.1253/circj.69.903
143. Tsuchihashi T, Kakimoto N, Takeuchi T, Suenaga T, Suzuki T, Shibuta S, et al. Intimal thickening and disruption of the media occur in the arterial walls of coronary arteries not associated with coronary arterial aneurysms in patients with kawasaki disease. BMC Cardiovasc Disord. (2021) 21(1):278. doi: 10.1186/s12872-021-02090-7
144. Kapadia SR, Nissen SE, Tuzcu EM. Impact of intravascular ultrasound in understanding transplant coronary artery disease. Curr Opin Cardiol. (1999) 14(2):140. doi: 10.1097/00001573-199903000-00011
145. Wong M, Edelstein J, Wollman J, Bond MG. Ultrasonic-pathological comparison of the human arterial wall. Verification of Intima-media Thickness. Arterioscler Thromb J Vasc Biol. (1993) 13(4):482–6. doi: 10.1161/01.ATV.13.4.482
146. Choi YS, Youn HJ, Park CS, Oh YS, Chung WS, Kim JH. High echogenic thickening of proximal coronary artery predicts the far advanced coronary atherosclerosis. Echocardiography. (2009) 26(2):133–9. doi: 10.1111/j.1540-8175.2008.00766.x
147. Gradus-Pizlo I, Feigenbaum H. Imaging of the left anterior descending coronary artery by high-frequency transthoracic and epicardial echocardiography. Am J Cardiol. (2002) 90(10):L28–31. doi: 10.1016/S0002-9149(02)02960-0
148. Makowski MR, Botnar RM. MR Imaging of the arterial vessel wall: molecular imaging from bench to bedside. Radiology. (2013) 269(1):34–51. doi: 10.1148/radiol.13102336
149. Gerretsen SC, Kooi ME, Kessels AG, Schalla S, Katoh M, van der Geest RJ, et al. Visualization of coronary wall atherosclerosis in asymptomatic subjects and patients with coronary artery disease using magnetic resonance imaging. PLoS One. (2010) 5(9):e12998. doi: 10.1371/journal.pone.0012998
150. van den Oord SCH, Sijbrands EJG, ten Kate GL, van Klaveren D, van Domburg RT, van der Steen AFW, et al. Carotid intima-media thickness for cardiovascular risk assessment: systematic review and meta-analysis. Atherosclerosis. (2013) 228(1):1–11. doi: 10.1016/j.atherosclerosis.2013.01.025
151. Willeit P, Tschiderer L, Allara E, Reuber K, Seekircher L, Gao L, et al. Carotid intima-media thickness progression as surrogate marker for cardiovascular risk. Circulation. (2020) 142(7):621–42. doi: 10.1161/CIRCULATIONAHA.120.046361
152. Den Ruijter HM, Peters SAE, Anderson TJ, Britton AR, Dekker JM, Eijkemans MJ, et al. Common carotid intima-media thickness measurements in cardiovascular risk prediction: a meta-analysis. JAMA. (2012) 308(8):796–803. doi: 10.1001/jama.2012.9630
153. Lorenz MW, Schaefer C, Steinmetz H, Sitzer M. Is carotid intima media thickness useful for individual prediction of cardiovascular risk? Ten-year results from the carotid atherosclerosis progression study (CAPS). Eur Heart J. (2010) 31(16):2041–8. doi: 10.1093/eurheartj/ehq189
154. Polak JF, Szklo M, Kronmal RA, Burke GL, Shea S, Zavodni AEH, et al. The value of carotid artery plaque and intima-media thickness for incident cardiovascular disease: the multi-ethnic study of atherosclerosis. J Am Heart Assoc. (2013) 2(2):e000087. doi: 10.1161/JAHA.113.000087
155. Bytyçi I, Shenouda R, Wester P, Henein MY. Carotid atherosclerosis in predicting coronary artery disease: a systematic review and meta-analysis. Arterioscler Thromb Vasc Biol. (2021) 41(4):e224–e37. doi: 10.1161/ATVBAHA.120.315747
156. Rea IM, Gibson DS, McGilligan V, McNerlan SE, Alexander HD, Ross OA. Age and age-related diseases: role of inflammation triggers and cytokines. Front Immunol. (2018) 9:586. doi: 10.3389/fimmu.2018.00586
157. Bailey-Downs LC, Tucsek Z, Toth P, Sosnowska D, Gautam T, Sonntag WE, et al. Aging exacerbates obesity-induced oxidative stress and inflammation in perivascular adipose tissue in mice: a paracrine mechanism contributing to vascular redox dysregulation and inflammation. J Gerontol A Bio Sci Med Sci. (2013) 68(7):780–92. doi: 10.1093/gerona/gls238
158. Oikonomou EK, Williams MC, Kotanidis CP, Desai MY, Marwan M, Antonopoulos AS, et al. A novel machine learning-derived radiotranscriptomic signature of perivascular fat improves cardiac risk prediction using coronary CT angiography. Eur Heart J. (2019) 40(43):3529–43. doi: 10.1093/eurheartj/ehz592
159. Oikonomou EK, Antoniades C. The role of adipose tissue in cardiovascular health and disease. Nat Rev Cardiol. (2019) 16(2):83–99. doi: 10.1038/s41569-018-0097-6
160. Dai X, Hou Y, Tang C, Lu Z, Shen C, Zhang L, et al. Long-term prognostic value of the serial changes of CT-derived fractional flow reserve and perivascular fat attenuation index. Quant Imaging Med Surg. (2022) 12(1):752. doi: 10.21037/qims-21-424
161. Hoshino M, Yang S, Sugiyama T, Zhang J, Kanaji Y, Yamaguchi M, et al. Prognostic value of peri-coronary adipose tissue attenuation and whole vessel and lesion plaque quantification on coronary computed tomography angiography. Eur Heart J. 2020;41(Suppl_2):ehaa946.0155. doi: 10.1093/ehjci/ehaa946.0155
162. Alavi A, Werner TJ, Al-Zaghal A. Detection of coronary inflammation. Lancet. (2019) 393(10187):2198. doi: 10.1016/S0140-6736(19)30224-7
163. Mazurek T, Kobylecka M, Zielenkiewicz M, Kurek A, Kochman J, Filipiak KJ, et al. PET/CT evaluation of 18F-FDG uptake in pericoronary adipose tissue in patients with stable coronary artery disease: independent predictor of atherosclerotic lesions’ formation? J Nucl Cardiol. (2017) 24(3):1075–84. doi: 10.1007/s12350-015-0370-6
164. Koenig W, Löwel H, Baumert J, Meisinger C. C-reactive protein modulates risk prediction based on the framingham score: implications for future risk assessment: results from a large cohort study in Southern Germany. Circulation. (2004) 109(11):1349–53. doi: 10.1161/01.CIR.0000120707.98922.E3
165. Pai JK, Pischon T, Ma J, Manson JE, Hankinson SE, Joshipura K, et al. Inflammatory markers and the risk of coronary heart disease in men and women. N Engl J Med. (2004) 351(25):2599–610. doi: 10.1056/NEJMoa040967
166. Lee R, Margaritis M, Channon KM, Antoniades C. Evaluating oxidative stress in human cardiovascular disease: methodological aspects and considerations. Curr Med Chem. (2012) 19(16):2504–20. doi: 10.2174/092986712800493057
167. Myers KS, Rudd JH, Hailman EP, Bolognese JA, Burke J, Pinto CA, et al. Correlation between arterial FDG uptake and biomarkers in peripheral artery disease. JACC Cardiovasc Imaging. (2012) 5(1):38–45. doi: 10.1016/j.jcmg.2011.08.019
168. Figueroa AL, Subramanian SS, Cury RC, Truong QA, Gardecki JA, Tearney GJ, et al. Distribution of inflammation within carotid atherosclerotic plaques with high-risk morphological features: a comparison between positron emission tomography activity, plaque morphology, and histopathology. Circ Cardiovasc Imaging. (2012) 5(1):69–77. doi: 10.1161/CIRCIMAGING.110.959478
169. Sadeghi MM. 18F-FDG PET and vascular inflammation: time to refine the paradigm? J Nucl Cardiol. (2015) 22(2):319–24. doi: 10.1007/s12350-014-9917-1
170. Zerizer I, Tan K, Khan S, Barwick T, Marzola MC, Rubello D, et al. Role of FDG-PET and PET/CT in the diagnosis and management of vasculitis. Eur J Radiol. (2010) 73(3):504–9. doi: 10.1016/j.ejrad.2010.01.021
171. Reddy AS, Uceda DE, Al Najafi M, Dey AK, Mehta NN. PET Scan with fludeoxyglucose/computed tomography in low-grade vascular inflammation. PET Clin. (2020) 15(2):207–13. doi: 10.1016/j.cpet.2019.11.009
172. Botkin CD, Osman MM. Prevalence, challenges, and solutions for 18F-FDG PET studies of obese patients: a technologist’s perspective. J Nucl Med Technol. (2007) 35(2):80–3. doi: 10.2967/jnmt.106.034918
173. Ludmer PL, Selwyn AP, Shook TL, Wayne RR, Mudge GH, Alexander RW, et al. Paradoxical vasoconstriction induced by acetylcholine in atherosclerotic coronary arteries. N Engl J Med. (1986) 315(17):1046–51. doi: 10.1056/NEJM198610233151702
174. Reriani M, Sara JD, Flammer A, Gulati R, Li MJ, Rihal C, et al. Coronary endothelial function testing provides superior discrimination compared to standard clinical risk scoring in prediction of cardiovascular events. Coron Artery Dis. (2016) 27(3):213. doi: 10.1097/MCA.0000000000000347
175. Brocq ML, Leslie SJ, Milliken P, Megson IL. Endothelial dysfunction: from molecular mechanisms to measurement, clinical implications, and therapeutic opportunities. Antioxid Redox Signaling. (2008) 10(9):1631–74. doi: 10.1089/ars.2007.2013
176. Garcia D, Harbaoui B, van de Hoef TP, Meuwissen M, Nijjer SS, Echavarria-Pinto M, et al. Relationship between FFR, CFR and coronary microvascular resistance–practical implications for FFR-guided percutaneous coronary intervention. PloS One. (2019) 14(1):e0208612. doi: 10.1371/journal.pone.0208612
177. Lachance P, Déry J-P, Rodés-Cabau J, Potvin J-M, Barbeau G, Bertrand OF, et al. Impact of fractional flow reserve measurement on the clinical management of patients with coronary artery disease evaluated with noninvasive stress tests prior to cardiac catheterization. Cardiovasc Revasc Med. (2008) 9(4):229–34. doi: 10.1016/j.carrev.2008.02.002
178. Petretta M, Costanzo P, Cuocolo A. Imaging techniques for the assessment of coronary flow reserve. European Cardiology. (2008) 4(1):37–40. 2008. doi: 10.15420/ecr.2008.4.1.37
179. Rigo F, Murer B, Ossena G, Favaretto E. Transthoracic echocardiographic imaging of coronary arteries: tips, traps, and pitfalls. Cardiovasc Ultrasound. (2008) 6(1):7. doi: 10.1186/1476-7120-6-7
180. Sicari R, Nihoyannopoulos P, Evangelista A, Kasprzak J, Lancellotti P, Poldermans D, et al. Stress echocardiography expert consensus statement: European Association of Echocardiography (EAE) (a registered branch of the ESC). Eur J Echocardiogr. (2008) 9(4):415–37. doi: 10.1093/ejechocard/jen175
181. Ramandika E, Kurisu S, Nitta K, Hidaka T, Utsunomiya H, Ishibashi K, et al. Effects of aging on coronary flow reserve in patients with no evidence of myocardial perfusion abnormality. Heart Vessels. (2020) 35:1633–9. doi: 10.1007/s00380-020-01643-8
182. Galderisi M, Rigo F, Gherardi S, Cortigiani L, Santoro C, Sicari R, et al. The impact of aging and atherosclerotic risk factors on transthoracic coronary flow reserve in subjects with normal coronary angiography. Cardiovasc Ultrasound. (2012) 10(1):20. doi: 10.1186/1476-7120-10-20
183. Larghat AM, Maredia N, Biglands J, Greenwood JP, Ball SG, Jerosch-Herold M, et al. Reproducibility of first-pass cardiovascular magnetic resonance myocardial perfusion. J Magn Reson Imaging. (2013) 37(4):865–74. doi: 10.1002/jmri.23889
184. Tonet E, Pompei G, Faragasso E, Cossu A, Pavasini R, Passarini G, et al. Coronary microvascular dysfunction: PET, CMR and CT assessment. J Clin Med. (2021) 10(9):1848. doi: 10.3390/jcm10091848
185. Hays AG, Hirsch GA, Kelle S, Gerstenblith G, Weiss RG, Stuber M. Noninvasive visualization of coronary artery endothelial function in healthy subjects and in patients with coronary artery disease. J Am Coll Cardiol. (2010) 56(20):1657–65. doi: 10.1016/j.jacc.2010.06.036
186. Iantorno M, Hays AG, Schär M, Krishnaswamy R, Soleimanifard S, Steinberg A, et al. Simultaneous noninvasive assessment of systemic and coronary endothelial function. Circ Cardiovasc Imaging. (2016) 9(3):e003954. doi: 10.1161/CIRCIMAGING.115.003954
187. Murthy VL, Naya M, Foster CR, Hainer J, Gaber M, Di Carli G, et al. Improved cardiac risk assessment with noninvasive measures of coronary flow reserve. Circulation. (2011) 124(20):2215–24. doi: 10.1161/CIRCULATIONAHA.111.050427
188. Ziadi MC, deKemp RA, Williams KA, Guo A, Chow BJW, Renaud JM, et al. Impaired myocardial flow reserve on rubidium-82 positron emission tomography imaging predicts adverse outcomes in patients assessed for myocardial ischemia. J Am Coll Cardiol. (2011) 58(7):740–8. doi: 10.1016/j.jacc.2011.01.065
189. Taqueti VR, Hachamovitch R, Murthy VL, Naya M, Foster CR, Hainer J, et al. Global coronary flow reserve is associated with adverse cardiovascular events independently of luminal angiographic severity and modifies the effect of early revascularization. Circulation. (2015) 131(1):19–27. doi: 10.1161/CIRCULATIONAHA.114.011939
190. Premer C, Kanelidis AJ, Hare JM, Schulman IH. Rethinking endothelial dysfunction as a crucial target in fighting heart failure. Mayo Clin Proc Innov Qual Outcomes. (2019) 3(1):1–13.30899903
191. Matsuzawa Y, Kwon TG, Lennon RJ, Lerman LO, Lerman A. Prognostic value of flow-mediated vasodilation in brachial artery and fingertip artery for cardiovascular events: a systematic review and meta-analysis. J Am Heart Assoc. (2015) 4(11):e002270. doi: 10.1161/JAHA.115.002270
192. Inaba Y, Chen JA, Bergmann SR. Prediction of future cardiovascular outcomes by flow-mediated vasodilatation of brachial artery: a meta-analysis. Int J Cardiovasc Imaging. (2010) 26(6):631–40. doi: 10.1007/s10554-010-9616-1
193. Green DJ, Jones H, Thijssen D, Cable NT, Atkinson G. Flow-mediated dilation and cardiovascular event prediction. Hypertension. (2011) 57(3):363–9. doi: 10.1161/HYPERTENSIONAHA.110.167015
194. Ras RT, Streppel MT, Draijer R, Zock PL. Flow-mediated dilation and cardiovascular risk prediction: a systematic review with meta-analysis. Int J Cardiol. (2013) 168(1):344–51. doi: 10.1016/j.ijcard.2012.09.047
195. Xu Y, Arora RC, Hiebert BM, Lerner B, Szwajcer A, McDonald K, et al. Non-invasive endothelial function testing and the risk of adverse outcomes: a systematic review and meta-analysis. Eur Heart J Cardiovasc Imaging. (2014) 15(7):736–46. doi: 10.1093/ehjci/jet256
196. Yeboah J, Folsom AR, Burke GL, Johnson C, Polak JF, Post W, et al. Predictive value of brachial flow-mediated dilation for incident cardiovascular events in a population-based study. Circulation. (2009) 120(6):502–9. doi: 10.1161/CIRCULATIONAHA.109.864801
197. Bourantas CV, Crake T, Zhang Y-J, Ozkor M, Ahmed J, Garcia-Garcia HM, et al. Intravascular imaging in cardiovascular ageing. Exp Gerontol. (2018) 109:31–7. doi: 10.1016/j.exger.2017.05.011
198. Hong YJ, Jeong MH, Ahn Y, Sim DS, Chung JW, Cho JS, et al. Age-related differences in intravascular ultrasound findings in 1,009 coronary artery disease patients. Circ J. (2008) 72(8):1270–5. doi: 10.1253/circj.72.1270
199. Philipp S, Böse D, Wijns W, Marso SP, Schwartz RS, König A, et al. Do systemic risk factors impact invasive findings from virtual histology? Insights from the international virtual histology registry. Eur Heart J. (2010) 31(2):196–202. doi: 10.1093/eurheartj/ehp428
200. Ruiz-García J, Lerman A, Weisz G, Maehara A, Mintz GS, Fahy M, et al. Age-and gender-related changes in plaque composition in patients with acute coronary syndrome: the PROSPECT study. EuroIntervention. (2012) 8(8):929–38. doi: 10.4244/EIJV8I8A142
201. Nissen SE, Nicholls SJ, Sipahi I, Libby P, Raichlen JS, Ballantyne CM, et al. Effect of very high-intensity statin therapy on regression of coronary atherosclerosis: the ASTEROID trial. JAMA. (2006) 295(13):1556–65. doi: 10.1001/jama.295.13.jpc60002
202. Nissen SE, Tuzcu EM, Schoenhagen P, Brown BG, Ganz P, Vogel RA, et al. Effect of intensive compared with moderate lipid-lowering therapy on progression of coronary atherosclerosis: a randomized controlled trial. JAMA. (2004) 291(9):1071–80. doi: 10.1001/jama.291.9.1071
203. Okazaki S, Yokoyama T, Miyauchi K, Shimada K, Kurata T, Sato H, et al. Early statin treatment in patients with acute coronary syndrome. Circulation. (2004) 110(9):1061–8. doi: 10.1161/01.CIR.0000140261.58966.A4
204. Mintz GS. Intravascular imaging of coronary calcification and its clinical implications. JACC Cardiovasc Imaging. (2015) 8(4):461–71. doi: 10.1016/j.jcmg.2015.02.003
205. Polonsky TS, McClelland RL, Jorgensen NW, Bild DE, Burke GL, Guerci AD, et al. Coronary artery calcium score and risk classification for coronary heart disease prediction. JAMA. (2010) 303(16):1610–6. doi: 10.1001/jama.2010.461
206. Sandfort V, Bluemke DA. CT Calcium scoring. History, current status and outlook. Diagn Interv Imaging. (2017) 98(1):3–10. doi: 10.1016/j.diii.2016.06.007
207. Erbel R, Möhlenkamp S, Moebus S, Schmermund A, Lehmann N, Stang A, et al. Coronary risk stratification, discrimination, and reclassification improvement based on quantification of subclinical coronary atherosclerosis. J Am Coll Cardiol. (2010) 56(17):1397–406. doi: 10.1016/j.jacc.2010.06.030
208. Dewey M, Hamm B. Cost effectiveness of coronary angiography and calcium scoring using CT and stress MRI for diagnosis of coronary artery disease. Eur Radiol. (2007) 17(5):1301–9. doi: 10.1007/s00330-006-0439-3
209. Maddox TM, Stanislawski MA, Grunwald GK, Bradley SM, Ho PM, Tsai TT, et al. Nonobstructive coronary artery disease and risk of myocardial infarction. JAMA. (2014) 312(17):1754–63. doi: 10.1001/jama.2014.14681
210. LaMonte MJ, FitzGerald SJ, Church TS, Barlow CE, Radford NB, Levine BD, et al. Coronary artery calcium score and coronary heart disease events in a large cohort of asymptomatic men and women. Am J Epidemiol. (2005) 162(5):421–9. doi: 10.1093/aje/kwi228
211. Detrano R, Guerci AD, Carr JJ, Bild DE, Burke G, Folsom AR, et al. Coronary calcium as a predictor of coronary events in four racial or ethnic groups. N Engl J Med. (2008) 358(13):1336–45. doi: 10.1056/NEJMoa072100
212. Vliegenthart R, Oudkerk M, Hofman A, Oei H-HS, van Dijck W, van Rooij FJ, et al. Coronary calcification improves cardiovascular risk prediction in the elderly. Circulation. (2005) 112(4):572–7. doi: 10.1161/CIRCULATIONAHA.104.488916
213. Yeboah J, Young R, McClelland RL, Delaney JC, Polonsky TS, Dawood FZ, et al. Utility of nontraditional risk markers in atherosclerotic cardiovascular disease risk assessment. J Am Coll Cardiol. (2016) 67(2):139–47. doi: 10.1016/j.jacc.2015.10.058
214. Grandhi GR, Mirbolouk M, Dardari ZA, Al-Mallah MH, Rumberger JA, Shaw LJ, et al. Interplay of coronary artery calcium and risk factors for predicting CVD/CHD mortality: the CAC consortium. JACC Cardiovasc Imaging. (2020) 13(5):1175–86. doi: 10.1016/j.jcmg.2019.08.024
215. Miedema MD, Dardari ZA, Nasir K, Blankstein R, Knickelbine T, Oberembt S, et al. Association of coronary artery calcium with long-term, cause-specific mortality among young adults. JAMA Netw Open. (2019) 2(7):e197440-e. doi: 10.1001/jamanetworkopen.2019.7440
216. Sandhu AT, Rodriguez F, Ngo S, Patel BN, Mastrodicasa D, Eng D, et al. Incidental coronary artery calcium: opportunistic screening of previous nongated chest computed tomography scans to improve statin rates (NOTIFY-1 project). Circulation. (2023) 147(9):703–14. doi: 10.1161/CIRCULATIONAHA.122.062746
217. van der Aalst CM, Denissen SJAM, Vonder M, Gratama JWC, Adriaansen HJ, Kuijpers D, et al. Screening for cardiovascular disease risk using traditional risk factor assessment or coronary artery calcium scoring: the ROBINSCA trial. Eur Heart J Cardiovasc Imaging. (2020) 21(11):1216–24. doi: 10.1093/ehjci/jeaa168
218. de Graaf MA, Broersen A, Kitslaar PH, Roos CJ, Dijkstra J, Lelieveldt BPF, et al. Automatic quantification and characterization of coronary atherosclerosis with computed tomography coronary angiography: cross-correlation with intravascular ultrasound virtual histology. Int J Cardiovasc Imaging. (2013) 29(5):1177–90. doi: 10.1007/s10554-013-0194-x
219. Voros S, Rinehart S, Qian Z, Vazquez G, Anderson H, Murrieta L, et al. Prospective validation of standardized, 3-dimensional, quantitative coronary computed tomographic plaque measurements using radiofrequency backscatter intravascular ultrasound as reference standard in intermediate coronary arterial lesions: results from the ATLANTA (assessment of tissue characteristics, lesion morphology, and hemodynamics by angiography with fractional flow reserve, intravascular ultrasound and virtual histology, and noninvasive computed tomography in atherosclerotic plaques) I study. JACC Cardiovasc Interv. (2011) 4(2):198–208. doi: 10.1016/j.jcin.2010.10.008
220. Watanabe D, Gando Y, Murakami H, Kawano H, Yamamoto K, Morishita A, et al. Longitudinal trajectory of vascular age indices and cardiovascular risk factors: a repeated-measures analysis. Sci Rep. (2023) 13(1):5401. doi: 10.1038/s41598-023-32443-5
221. Fung K, Ramírez J, Warren HR, Aung N, Lee AM, Tzanis E, et al. Genome-wide association study identifies loci for arterial stiffness index in 127,121 UK biobank participants. Sci Rep. (2019) 9(1):9143. doi: 10.1038/s41598-019-45703-0
222. Ji H, Kwan AC, Chen MT, Ouyang D, Ebinger JE, Bell SP, et al. Sex differences in myocardial and vascular aging. Circ Res. (2022) 130(4):566–77. doi: 10.1161/CIRCRESAHA.121.319902
223. Doonan RJ, Hausvater A, Scallan C, Mikhailidis DP, Pilote L, Daskalopoulou SS. The effect of smoking on arterial stiffness. Hypertens Res. (2010) 33(5):398–410. doi: 10.1038/hr.2010.25
224. Aroor AR, Jia G, Sowers JR. Cellular mechanisms underlying obesity-induced arterial stiffness. Am J Physiol Regul Integr Comp Physiol. (2018) 314(3):R387–R98. doi: 10.1152/ajpregu.00235.2016
225. Franklin SS. Arterial stiffness and hypertension: a two-way street? Hypertension. (2005) 45(3):349–51. doi: 10.1161/01.HYP.0000157819.31611.87
226. Nilsson PM, Boutouyrie P, Laurent S. Vascular aging: a tale of EVA and ADAM in cardiovascular risk assessment and prevention. Hypertension. (2009) 54(1):3–10. doi: 10.1161/HYPERTENSIONAHA.109.129114
227. Stehouwer C, Henry R, Ferreira I. Arterial stiffness in diabetes and the metabolic syndrome: a pathway to cardiovascular disease. Diabetologia. (2008) 51:527–39. doi: 10.1007/s00125-007-0918-3
228. Climie RE, Boutouyrie P, Perier M-C, Guibout C, van Sloten TT, Thomas F, et al. Individual and neighborhood deprivation and carotid stiffness. Hypertension. (2019) 73(6):1185–94. doi: 10.1161/HYPERTENSIONAHA.118.12186
229. Onete V, Henry RM, Sep SJ, Koster A, van der Kallen CJ, Dagnelie PC, et al. Arterial stiffness is associated with depression in middle-aged men—the Maastricht study. J Psychiatry Neurosci. (2018) 43(2):111–9. doi: 10.1503/jpn.160246
230. Terentes-Printzios D, Vlachopoulos C, Xaplanteris P, Ioakeimidis N, Aznaouridis K, Baou K, et al. Cardiovascular risk factors accelerate progression of vascular aging in the general population. Hypertension. (2017) 70(5):1057–64. doi: 10.1161/HYPERTENSIONAHA.117.09633
231. Mzayek F, Sherwin R, Hughes J, Hassig S, Srinivasan S, Chen W, et al. The association of birth weight with arterial stiffness at mid-adulthood: the bogalusa heart study. J Epidemiol Community Health. (2009) 63(9):729. doi: 10.1136/jech.2008.084475
232. Koivistoinen T, Hutri-Kähönen N, Juonala M, Aatola H, Kööbi T, Lehtimäki T, et al. Metabolic syndrome in childhood and increased arterial stiffness in adulthood—the cardiovascular risk in young finns study. Ann Med. (2011) 43(4):312–9. doi: 10.3109/07853890.2010.549145
233. Miyachi M, Kawano H, Sugawara J, Takahashi K, Hayashi K, Yamazaki K, et al. Unfavorable effects of resistance training on central arterial compliance: a randomized intervention study. Circulation. (2004) 110(18):2858–63. doi: 10.1161/01.CIR.0000146380.08401.99
234. Gando Y, Murakami H, Yamamoto K, Kawakami R, Ohno H, Sawada SS, et al. Greater progression of age-related aortic stiffening in adults with poor trunk flexibility: a 5-year longitudinal study. Front Physiol. (2017) 8:454. doi: 10.3389/fphys.2017.00454
235. Eskurza I, Myerburgh LA, Kahn ZD, Seals DR. Tetrahydrobiopterin augments endothelium-dependent dilatation in sedentary but not in habitually exercising older adults. J Physiol (Lond). (2005) 568(3):1057–65. doi: 10.1113/jphysiol.2005.092734
236. McClelland RL, Nasir K, Budoff M, Blumenthal RS, Kronmal RA. Arterial age as a function of coronary artery calcium (from the multi-ethnic study of atherosclerosis [MESA]). Am J Cardiol. (2009) 103(1):59–63. doi: 10.1016/j.amjcard.2008.08.031
237. Nappi C, Gaudieri V, Acampa W, Arumugam P, Assante R, Zampella E, et al. Coronary vascular age: an alternate means for predicting stress-induced myocardial ischemia in patients with suspected coronary artery disease. J Nucl Cardiol. (2019) 26(4):1348–55. doi: 10.1007/s12350-018-1191-1
238. Ayoub C, Kritharides L, Yeung Y, Chen L, Hossain A, Achenbach S, et al. Prognostic value of age adjusted segment involvement score as measured by coronary computed tomography: a potential marker of vascular age. Heart Vessels. (2018) 33(11):1288–300. doi: 10.1007/s00380-018-1188-3
239. Oikonomou EK, Antonopoulos AS, Schottlander D, Marwan M, Mathers C, Tomlins P, et al. Standardized measurement of coronary inflammation using cardiovascular computed tomography: integration in clinical care as a prognostic medical device. Cardiovasc Res. (2021) 117(13):2677–90. doi: 10.1093/cvr/cvab286
240. Heseltine TD, Murray SW, Ruzsics B, Fisher M. Latest advances in cardiac CT. Eur Cardiol Rev. (2020) 15. doi: 10.15420/ecr.2019.14.2
241. Donato AJ, Walker AE, Magerko KA, Bramwell RC, Black AD, Henson GD, et al. Life-long caloric restriction reduces oxidative stress and preserves nitric oxide bioavailability and function in arteries of old mice. Aging Cell. (2013) 12(5):772–83. doi: 10.1111/acel.12103
242. Batzias K, Antonopoulos AS, Oikonomou E, Siasos G, Bletsa E, Stampouloglou PK, et al. Effects of newer antidiabetic drugs on endothelial function and arterial stiffness: a systematic review and meta-analysis. J Diabetes Res. (2018) 2018:1232583. doi: 10.1155/2018/1232583
243. Maniar Y, Blumenthal RS, Alfaddagh A. The role of coronary artery calcium in allocating pharmacotherapy for primary prevention of cardiovascular disease: the ABCs of CAC. Clin Cardiol. (2022) 45(11):1107–13. doi: 10.1002/clc.23918
244. Green DJ, Maiorana A, O’Driscoll G, Taylor R. Effect of exercise training on endothelium-derived nitric oxide function in humans. J Physiol (Lond). (2004) 561(1):1–25. doi: 10.1113/jphysiol.2004.068197
245. Somani SM, Husain K. Exercise training alters kinetics of antioxidant enzymes in rat tissues. Biochem Mol Biol Int. (1996) 38(3):587–95.8829619
246. Kozakova M, Palombo C. Vascular ageing and aerobic exercise. Int J Environ Res Public Health. (2021) 18(20):10666. doi: 10.3390/ijerph182010666
247. Laufs U, Werner N, Link A, Endres M, Wassmann S, Jürgens K, et al. Physical training increases endothelial progenitor cells, inhibits neointima formation, and enhances angiogenesis. Circulation. (2004) 109(2):220–6. doi: 10.1161/01.CIR.0000109141.48980.37
248. Huang C, Wang J, Deng S, She Q, Wu L. The effects of aerobic endurance exercise on pulse wave velocity and intima media thickness in adults: a systematic review and meta-analysis. Scand J Med Sci Sports. (2016) 26(5):478–87. doi: 10.1111/sms.12495
249. Ashor AW, Lara J, Siervo M, Celis-Morales C, Mathers JC. Effects of exercise modalities on arterial stiffness and wave reflection: a systematic review and meta-analysis of randomized controlled trials. PLoS One. (2014) 9(10):e110034. doi: 10.1371/journal.pone.0110034
250. Pierce DR, Doma K, Leicht AS. Acute effects of exercise mode on arterial stiffness and wave reflection in healthy young adults: a systematic review and meta-analysis. Front Physiol. (2018) 9:73. doi: 10.3389/fphys.2018.00073
251. Barnes JN, Trombold JR, Dhindsa M, Lin H-F, Tanaka H. Arterial stiffening following eccentric exercise-induced muscle damage. J Appl Physiol. (2010) 109(4):1102–8. doi: 10.1152/japplphysiol.00548.2010
252. Motohiko M. Effects of resistance training on arterial stiffness: a meta-analysis. Br J Sports Med. (2013) 47(6):393. doi: 10.1136/bjsports-2012-090488
253. D’Elia L, Galletti F, La Fata E, Sabino P, Strazzullo P. Effect of dietary sodium restriction on arterial stiffness: systematic review and meta-analysis of the randomized controlled trials. J Hypertens. (2018) 36(4):734–43. doi: 10.1097/HJH.0000000000001604
254. Most J, Tosti V, Redman LM, Fontana L. Calorie restriction in humans: an update. Ageing Res Rev. (2017) 39:36–45. doi: 10.1016/j.arr.2016.08.005
255. Guo Z, Mitchell-Raymundo F, Yang H, Ikeno Y, Nelson J, Diaz V, et al. Dietary restriction reduces atherosclerosis and oxidative stress in the aorta of apolipoprotein E-deficient mice. Mech Ageing Dev. (2002) 123(8):1121–31. doi: 10.1016/S0047-6374(02)00008-8
256. Clifton PM, Keogh J, Foster P, Noakes M. Effect of weight loss on inflammatory and endothelial markers and FMD using two low-fat diets. Int J Obes. (2005) 29(12):1445–51. doi: 10.1038/sj.ijo.0803039
257. Keogh JB, Brinkworth GD, Noakes M, Belobrajdic DP, Buckley JD, Clifton PM. Effects of weight loss from a very-low-carbohydrate diet on endothelial function and markers of cardiovascular disease risk in subjects with abdominal obesity. Am J Clin Nutr. (2008) 87(3):567–76. doi: 10.1093/ajcn/87.3.567
258. Dengo AL, Dennis EA, Orr JS, Marinik EL, Ehrlich E, Davy BM, et al. Arterial destiffening with weight loss in overweight and obese middle-aged and older adults. Hypertension. (2010) 55(4):855–61. doi: 10.1161/HYPERTENSIONAHA.109.147850
259. Nowak KL, Rossman MJ, Chonchol M, Seals DR. Strategies for achieving healthy vascular aging. Hypertension. (2018) 71(3):389–402. doi: 10.1161/HYPERTENSIONAHA.117.10439
260. Ravussin E, Redman LM, Rochon J, Das SK, Fontana L, Kraus WE, et al. A 2-year randomized controlled trial of human caloric restriction: feasibility and effects on predictors of health span and longevity. J Gerontol A Biol Sci Med Sci. (2015) 70(9):1097–104. doi: 10.1093/gerona/glv057
261. Il'yasova D, Fontana L, Bhapkar M, Pieper CF, Spasojevic I, Redman LM, et al. Effects of 2 years of caloric restriction on oxidative status assessed by urinary F2-isoprostanes: the CALERIE 2 randomized clinical trial. Aging Cell. (2018) 17(2):e12719. doi: 10.1111/acel.12719
262. Janić M, Lunder M, Šabovič M. Arterial stiffness and cardiovascular therapy. BioMed Res Int. (2014) 2014:621437. doi: 10.1155/2014/621437
263. Shahin Y, Khan JA, Chetter I. Angiotensin converting enzyme inhibitors effect on arterial stiffness and wave reflections: a meta-analysis and meta-regression of randomised controlled trials. Atherosclerosis. (2012) 221(1):18–33. doi: 10.1016/j.atherosclerosis.2011.12.005
264. Williams B. CAFE Investigators; anglo-scandinavian cardiac outcomes trial investigators; CAFE steering committee and writing committee. Differential impact of blood pressure-lowering drugs on central aortic pressure and clinical outcomes: principal results of the conduit artery function evaluation (CAFE) study. Circulation. (2006) 113:1213–25. doi: 10.1161/CIRCULATIONAHA.105.606962
265. Safar ME. Arterial aging—hemodynamic changes and therapeutic options. Nat Rev Cardiol. (2010) 7(8):442–9. doi: 10.1038/nrcardio.2010.96
266. Chirinos JA, Segers P, Hughes T, Townsend R. Large-Artery stiffness in health and disease. J Am Coll Cardiol. (2019) 74(9):1237–63. doi: 10.1016/j.jacc.2019.07.012
267. Camici GG, Savarese G, Akhmedov A, Lüscher TF. Molecular mechanism of endothelial and vascular aging: implications for cardiovascular disease. Eur Heart J. (2015) 36(48):3392–403. doi: 10.1093/eurheartj/ehv587
268. de Cavanagh EMV, Inserra F, Ferder L. Angiotensin II blockade: how its molecular targets may signal to mitochondria and slow aging. Coincidences with calorie restriction and mTOR inhibition. Am J Physiol Heart Circ Physiol. 2015;309(1):H15–44. doi: 10.1152/ajpheart.00459.2014
269. Force UPST. Statin use for the primary prevention of cardiovascular disease in adults: uS preventive services task force recommendation statement. JAMA. (2022) 328(8):746–53. doi: 10.1001/jama.2022.13044
270. Libby P. The changing landscape of atherosclerosis. Nature. (2021) 592(7855):524–-33. doi: 10.1038/s41586-021-03392-8
271. Coppinger C, Movahed MR, Azemawah V, Peyton L, Gregory J, Hashemzadeh M. A comprehensive review of PCSK9 inhibitors. J Cardiovasc Pharmacol Ther. (2022) 27:10742484221100107. doi: 10.1177/10742484221100107
272. Turgeon RD, Tsuyuki RT, Gyenes GT, Pearson GJ. Cardiovascular efficacy and safety of PCSK9 inhibitors: systematic review and meta-analysis including the ODYSSEY OUTCOMES trial. Can J Cardiol. (2018) 34(12):1600–5. doi: 10.1016/j.cjca.2018.04.002
273. Mitchell JD, Fergestrom N, Gage BF, Paisley R, Moon P, Novak E, et al. Impact of statins on cardiovascular outcomes following coronary artery calcium scoring. J Am Coll Cardiol. (2018) 72(25):3233–42. doi: 10.1016/j.jacc.2018.09.051
274. Orr JS, Dengo AL, Rivero JM, Davy KP. Arterial destiffening with atorvastatin in overweight and obese middle-aged and older adults. Hypertension. (2009) 54(4):763–8. doi: 10.1161/HYPERTENSIONAHA.109.138248
275. Group AtCCRiDS. Effects of intensive glucose lowering in type 2 diabetes. N Engl J Med. (2008) 358(24):2545–59. doi: 10.1056/NEJMoa0802743
276. Group AC. Intensive blood glucose control and vascular outcomes in patients with type 2 diabetes. N Engl J Med. (2008) 358(24):2560–72. doi: 10.1056/NEJMoa0802987
277. Lambadiari V, Pavlidis G, Kousathana F, Varoudi M, Vlastos D, Maratou E, et al. Effects of 6-month treatment with the glucagon like peptide-1 analogue liraglutide on arterial stiffness, left ventricular myocardial deformation and oxidative stress in subjects with newly diagnosed type 2 diabetes. Cardiovasc Diabetol. (2018) 17(1):1–12. doi: 10.1186/s12933-017-0646-z
278. Solini A, Giannini L, Seghieri M, Vitolo E, Taddei S, Ghiadoni L, et al. Dapagliflozin acutely improves endothelial dysfunction, reduces aortic stiffness and renal resistive index in type 2 diabetic patients: a pilot study. Cardiovasc Diabetol. (2017) 16(1):1–9. doi: 10.1186/s12933-017-0621-8
279. Lunder M, Janić M, Japelj M, Juretič A, Janež A, Šabovič M. Empagliflozin on top of metformin treatment improves arterial function in patients with type 1 diabetes mellitus. Cardiovasc Diabetol. (2018) 17(1):153. doi: 10.1186/s12933-018-0797-6
280. Cainzos-Achirica M, Patel KV, Quispe R, Joshi PH, Khera A, Ayers C, et al. Coronary artery calcium for the allocation of GLP-1RA for primary prevention of atherosclerotic cardiovascular disease. JACC Cardiovasc Imaging. (2021) 14(7):1470–2. doi: 10.1016/j.jcmg.2020.12.024
281. Ravelli RB, Gigant B, Curmi PA, Jourdain I, Lachkar S, Sobel A, et al. Insight into tubulin regulation from a complex with colchicine and a stathmin-like domain. Nature. (2004) 428(6979):198–202. doi: 10.1038/nature02393
282. Zhang F-S, He Q-Z, Qin CH, Little PJ, Weng J-P, Xu S-W. Therapeutic potential of colchicine in cardiovascular medicine: a pharmacological review. Acta Pharmacol Sin. (2022) 43(9):2173–90. doi: 10.1038/s41401-021-00835-w
283. Nidorf SM, Fiolet ATL, Eikelboom JW, Schut A, Opstal TSJ, Bax WA, et al. The effect of low-dose colchicine in patients with stable coronary artery disease: the LoDoCo2 trial rationale, design, and baseline characteristics. Am Heart J. (2019) 218:46–56. doi: 10.1016/j.ahj.2019.09.011
284. Nidorf SM, Eikelboom JW, Budgeon CA, Thompson PL. Low-dose colchicine for secondary prevention of cardiovascular disease. J Am Coll Cardiol. (2013) 61(4):404–10. doi: 10.1016/j.jacc.2012.10.027
285. Nidorf SM, Fiolet ATL, Mosterd A, Eikelboom JW, Schut A, Opstal TSJ, et al. Colchicine in patients with chronic coronary disease. N Engl J Med. (2020) 383(19):1838–47. doi: 10.1056/NEJMoa2021372
286. Tardif J-C, Kouz S, Waters DD, Bertrand OF, Diaz R, Maggioni AP, et al. Efficacy and safety of low-dose colchicine after myocardial infarction. N Engl J Med. (2019) 381(26):2497–505. doi: 10.1056/NEJMoa1912388
287. Joannidès R, Monteil C, De Ligny B, Westeel P, Iacob M, Thervet E, et al. Immunosuppressant regimen based on sirolimus decreases aortic stiffness in renal transplant recipients in comparison to cyclosporine. Am J Transplant. (2011) 11(11):2414–22. doi: 10.1111/j.1600-6143.2011.03697.x
288. Wong M, Oakley SP, Young L, Jiang B, Wierzbicki A, Panayi G, et al. Infliximab improves vascular stiffness in patients with rheumatoid arthritis. Ann Rheum Dis. (2009) 68(8):1277–84. doi: 10.1136/ard.2007.086157
289. Angel K, Provan SA, Gulseth HL, Mowinckel P, Kvien TK, Atar D. Tumor necrosis factor-α antagonists improve aortic stiffness in patients with inflammatory arthropathies: a controlled study. Hypertension. (2010) 55(2):333–8. doi: 10.1161/HYPERTENSIONAHA.109.143982
290. Mäki-Petäjä KM, Hall FC, Booth AD, Wallace SM, Yasmin , Bearcroft PW, et al. Rheumatoid arthritis is associated with increased aortic pulse-wave velocity, which is reduced by anti–tumor necrosis factor-α therapy. Circulation. 2006;114(11):1185–92. doi: 10.1161/CIRCULATIONAHA.105.601641
291. Ridker PM, Everett BM, Thuren T, MacFadyen JG, Chang WH, Ballantyne C, et al. Antiinflammatory therapy with canakinumab for atherosclerotic disease. N Engl J Med. (2017) 377(12):1119–31. doi: 10.1056/NEJMoa1707914
292. de Picciotto NE, Gano LB, Johnson LC, Martens CR, Sindler AL, Mills KF, et al. Nicotinamide mononucleotide supplementation reverses vascular dysfunction and oxidative stress with aging in mice. Aging Cell. (2016) 15(3):522–30. doi: 10.1111/acel.12461
293. Mattison JA, Wang M, Bernier M, Zhang J, Park S-S, Maudsley S, et al. Resveratrol prevents high fat/sucrose diet-induced central arterial wall inflammation and stiffening in nonhuman primates. Cell Metab. (2014) 20(1):183–90. doi: 10.1016/j.cmet.2014.04.018
294. Martens C, Denman B, Mazzo M, Armstrong M, Reisdorph N, McQueen M, et al. NAA1 Nicotinamide riboside supplementation reduces aortic stiffness and blood pressure in middle-aged and older adults. Artery Res. (2017) 20(C):49. doi: 10.1016/j.artres.2017.10.021
295. Childs BG, Baker DJ, Wijshake T, Conover CA, Campisi J, van Deursen JM. Senescent intimal foam cells are deleterious at all stages of atherosclerosis. Science. (2016) 354(6311):472–7. doi: 10.1126/science.aaf6659
296. Garrido AM, Kaistha A, Uryga AK, Oc S, Foote K, Shah A, et al. Efficacy and limitations of senolysis in atherosclerosis. Cardiovasc Res. (2022) 118(7):1713–27. doi: 10.1093/cvr/cvab208
Keywords: coronary artery disease, vascular aging, cardiovascular imaging, healthy vascular aging
Citation: Cheng DCY, Climie RE, Shu M, Grieve SM, Kozor R and Figtree GA (2023) Vascular aging and cardiovascular disease: pathophysiology and measurement in the coronary arteries. Front. Cardiovasc. Med. 10:1206156. doi: 10.3389/fcvm.2023.1206156
Received: 15 April 2023; Accepted: 13 November 2023;
Published: 28 November 2023.
Edited by:
Stephen E. Greenwald, Queen Mary University of London, United KingdomReviewed by:
Nikolaos I. Vlachogiannis, National and Kapodistrian University of Athens, GreeceKensuke Nishimiya, Tohoku University, Japan
© 2023 Cheng, Climie, Shu, Grieve, Kozor and Figtree. This is an open-access article distributed under the terms of the Creative Commons Attribution License (CC BY). The use, distribution or reproduction in other forums is permitted, provided the original author(s) and the copyright owner(s) are credited and that the original publication in this journal is cited, in accordance with accepted academic practice. No use, distribution or reproduction is permitted which does not comply with these terms.
*Correspondence: Gemma A. Figtree gemma.figtree@sydney.edu.au
Abbreviations CVD, cardiovascular disease; CAD, coronary artery disease; CAC, coronary artery calcium; CTCA, computed tomography coronary angiogram; VSMC, vascular smooth muscle cell; ECM, extracellular matrix; MMP, matrix metalloprotein; FAI, fat attenuation index; IL, interleukin; NAD, nicotinamide adenine dinucleotide; eNOS, endothelial nitrous oxide synthase; EPC, endothelial progenitor cells; TCFA, thin-cap fibroatheroma; mtDNA, mitochondrial DNA; CIMT, carotid intima-media thickness; cfPWV, carotid-femoral pulse wave velocity; HR, hazard ratio; CI, confidence interval; RR, relative risk; IVUS, intravascular ultrasound; IV OCT, intravascular optical coherence tomography; RCT, randomised control trial; MESA, multi-ethnic study of atherosclerosis; NRI, net reclassification index; FRP, fat radiomic profile; PET, positron emission tomography; PCAT, peri-coronary adipose tissue; CFR, coronary flow reserve; CMD, coronary microvascular dysfunction; FMD, flow-mediated dilatation; PMD, pooled mean difference; WMD, weighted mean difference; ACE, angiotensin-converting enzyme; RAAS, renin-angiotensin-aldosterone-system; LDL, low density lipoprotein; hs-CRP, high sensitivity c-reactive protein.