- 1Department of Cardiology Center, The First Hospital of Jilin University, Changchun, China
- 2Department of Endocrinology and Metabolism, The First Hospital of Jilin University, Changchun, China
Heart failure (HF) represents the terminal stage of cardiovascular disease and remains a leading cause of mortality. Epidemiological studies indicate a high prevalence and mortality rate of HF globally. Current treatment options primarily include pharmacological and non-pharmacological approaches. With the development of mesenchymal stem cell (MSC) transplantation technology, increasing research has shown that stem cell therapy and exosomes derived from these cells hold promise for repairing damaged myocardium and improving cardiac function, becoming a hot topic in clinical treatment for HF. However, this approach also presents certain limitations. This review summarizes the mechanisms of HF, current treatment strategies, and the latest progress in the application of MSCs and their exosomes in HF therapy.
1 Introduction
Heart failure (HF) is a clinical syndrome caused by impaired systolic and/or diastolic function of the heart, leading to pulmonary and/or systemic congestion and inadequate tissue perfusion. It primarily manifests as dyspnea, limited physical activity, and fluid retention (1, 2). The pathophysiology of HF is complex and involves multiple neurohormonal pathways, including the renin-angiotensin-aldosterone system (RAAS), sympathetic nervous system, and natriuretic peptide system (1, 3–5). The RAAS plays a central role in the pathogenesis and progression of HF, where activation of circulating and/or tissue RAAS leads to sodium and water retention, vasoconstriction, myocardial and vascular hypertrophy, fibrosis, and remodeling while enhancing the detrimental effects of other neurohormonal systems, such as the sympathetic nervous system (6–9). Heart failure is a terminal manifestation of cardiovascular disease and remains the primary cause of death, posing one of the two major challenges in cardiovascular medicine in the 21st century. According to epidemiological surveys, HF is rapidly emerging as a global public health issue, affecting over 64 million people worldwide. The incidence and mortality of HF continue to rise each year, placing a significant burden on healthcare systems (10). In Western countries, 1%–2% of hospitalizations are due to HF, making it the most common cause of hospital admission for patients over 65 years old. Approximately 30%–40% of HF patients have a history of hospitalization, with 50% being readmitted within a year of the initial diagnosis. HF is associated with high mortality, with 1-year mortality rates ranging from 15%–30% in certain populations and 5-year mortality rates reaching up to 75% (10). In Western countries, the annual healthcare cost per HF patient can reach €25,000, and the rising prevalence of HF is expected to further escalate these costs, even for developed nations (10). The expanding population of HF patients and the associated complications severely impact patients' quality of life. Current clinical treatments for HF primarily include pharmacological and non-pharmacological approaches. Traditional pharmacotherapy involves diuretics, β-blockers, angiotensin-converting enzyme inhibitors (ACEI) or angiotensin receptor blockers (ARB), and inotropic agents (11). Despite recent advancements in pharmacotherapy, traditional treatments still face certain limitations (12, 13). Non-pharmacological treatments mainly include interventions like cardiac resynchronization therapy (CRT) and implantable cardioverter-defibrillators (ICD), which have improved survival rates in some patients, though long-term prognosis remains poor (14, 15). Meanwhile, heart transplantation has offered hope for some critically ill patients, but challenges remain, including limited donor availability, severe post-operative complications, and high medical costs (16–18). Given that myocardial cells are permanent and non-regenerative, damaged myocardial cells and their microenvironment progressively deteriorate (19). Traditional pharmacological and non-pharmacological treatments can only alleviate symptoms but cannot reverse myocardial degeneration and necrosis or improve cardiac function fundamentally. With advancements in regenerative medicine, stem cell transplantation has emerged as a promising field. Under optimal conditions, stem cells can either differentiate directly into functional cardiomyocytes or indirectly promote cell survival through the secretion of exosomes. As such, stem cell-derived cardiomyocytes may replace dead myocardial cells and restore cardiac function, offering a novel and promising approach to HF treatment. Numerous studies have demonstrated the feasibility of MSCs transplantation for HF treatment. This review summarizes the mechanisms of HF, the current treatment landscape, and the latest developments in MSCs and exosome therapies for HF.
2 Mechanisms of heart failure
The most fundamental contraction of the heart is regulated by calcium ions. Calcium is released from the sarcoplasmic reticulum through ryanodine receptors, and the elevated intracellular Ca2+ concentration activates cardiac contraction. During diastole, Ca2+ is transported back into the sarcoplasmic reticulum via Ca2+-ATPase and sequestered. In HF, the activity of Ca2+-ATPase is reduced, leading to decreased uptake and storage of Ca2+ in the sarcoplasmic reticulum, which impairs cardiac repolarization and weakens myocardial contractility (20). Some studies indicate that increasing Ca2+ levels can enhance contractility in HF patients to some extent, yet other studies have shown that elevated Ca2+ can damage elastin in cardiomyocytes, increasing the risks of arrhythmia, myocardial hypertrophy, and apoptosis (21). These findings suggest that calcium dysregulation plays a key role in the pathogenesis of HF. Disrupted Ca2+ transport and homeostasis are associated with the development and progression of various diseases, including HF (22). Research also points out that serum Na+ and Ca2+ levels in HF patients are reduced, potentially contributing to impaired contractility and abnormal potassium channel expression, which exacerbates ventricular remodeling and leads to HF (23). Moreover, studies have revealed that the disruption of mitochondrial-sarcoplasmic reticulum junctions contributes to sinoatrial node dysfunction in HF, indicating that sarcoplasmic reticulum restructuring is involved in HF pathogenesis, further implying Ca2+ conversion abnormalities (24).
Oxidative stress (OS) has been demonstrated at every stage of HF development, and excess reactive oxygen species (ROS) can promote myocardial fibrosis, leading to cardiac remodeling, which in turn leads to HF and is involved in the progression of HF (25–27). ROS can be produced in the heart through various sources, including mitochondria, NADPH oxidase, uncoupled NO synthase (NOS), xanthine oxidase, cytochrome P450, and catecholamine autoxidation (28, 29). Excessive ROS production leads to mitochondrial oxidative stress and triggers mitochondrial dysfunction, including impairments in mitochondrial biogenesis, fatty acid metabolism, and antioxidant defense mechanisms. Increased mitochondrial ROS production has been demonstrated in myocardial cells in experimental models of HF and myocardial infarction (MI) (30). Furthermore, studies show that STAT3, a redox gene, protects mitochondrial integrity in cardiomyocytes, suppresses ROS formation, and regulates the expression of target genes like ErbB and Bcl-xL. However, STAT3 expression is reduced in damaged cardiomyocytes, which promotes cell death and fibrosis (31–33).
Inflammatory responses are crucial in the myocardial injury repair process. Leukocytes participate in the clearance of necrotic cardiomyocytes and initiate the myocardial repair process. Cardiomyocytes release adenosine triphosphate (ATP), which recruits macrophages to the site of inflammation to remove dead cells and debris (34). As macrophages digest apoptotic cells, various inflammatory cytokines, such as Tumor necrosis factor-α(TNF-α), Interleukin(IL)-1 β, IL-6, and transforming growth factor-β (TGF-β), may accumulate (35–37), promoting fibroblast activation, extracellular matrix (ECM) degradation, and increased matrix metalloproteinase (MMP) activity (38–40), further inducing cardiomyocyte apoptosis, autophagic damage, and fibrosis, eventually leading to cardiac hypertrophy (41). As ECM proteins are remodeled, the dead cardiomyocytes are replaced by scar tissue. Persistent inflammation, along with the continued infiltration of lymphocytes and macrophages into the scar tissue, exacerbates cardiac remodeling and reduces cardiac function, culminating in HF (41–43).
3 Current status of heart failure treatment
Traditionally, HF treatment includes general/basic therapy, pharmacological interventions, device-based therapies, and surgical interventions. In terms of general/basic therapy, patients must limit sodium intake (<5 g/day) and restrict fluid intake (<1–1.5 L/day) (44). Diuretics are used to alleviate symptoms of congestion, such as dyspnea and edema, with common medications including furosemide and spironolactone (45, 46). In recent years, the primary shift in HF pharmacotherapy has been towards the inhibition of excessive neurohormonal activation and its contribution to myocardial remodeling. Representative drugs include ACEI/ARB, β-blockers, and aldosterone receptor antagonists. In recent years, angiotensin receptor-neprilysin inhibitors (ARNIs) and sodium-glucose cotransporter-2 inhibitors (SGLT2i) have also emerged as prominent treatments. Renin-angiotensin system inhibitors, β-blockers, and aldosterone receptor antagonists can inhibit ventricular remodeling and reduce mortality in HF patients (47–50). ARNI, which combines ARB and enkephalinase inhibitor effects, raises levels of natriuretic peptides, bradykinin, and adrenomedullin, counteracting the vasoconstriction, sodium retention, and cardiac remodeling caused by excessive neurohormonal activation. The PARADIGM-HF trial demonstrated that sacubitril/valsartan sodium reduced the risk of the primary composite endpoint (cardiovascular death and HF hospitalization) by 20%, including a 20% reduction in sudden cardiac death, compared to enalapril (51). Subsequent studies such as TRANSITION and PIONEER-HF further validated the safety and efficacy of early initiation of ARNI therapy in hospitalized patients with acute decompensated HF (52, 53). SGLT2i, originally used as oral hypoglycemic agents by promoting the excretion of glucose and sodium through urine, have revealed unique cardiovascular effects. Studies have shown that SGLT2i reduces the risk of cardiovascular death and HF hospitalization in patients with type 2 diabetes (54), and their use has expanded to cardiovascular disease patients without diabetes. The DAPA-HF study confirmed that SGLT2i reduces the relative risk of HF worsening (hospitalization or emergency treatment with intravenous anti-HF medications) or cardiovascular death by 26% in HFrEF patients (55).
In addition to the aforementioned drugs, other medications like vericiguat, ivabradine, digitalis, and trimetazidine are also used in HF treatment. Vericiguat is the first oral soluble guanylate cyclase (sGC) stimulator approved for the treatment of symptomatic chronic HF with reduced ejection fraction (HFrEF) in adults (56). Vericiguat stimulates sGC independently of nitric oxide (NO) concentration and enhances NO sensitivity, increasing intracellular cyclic guanosine monophosphate (cGMP) levels, leading to smooth muscle relaxation and vasodilation, thereby improving cardiac structure and function. Increasing clinical studies have shown that vericiguat can reduce the incidence of cardiovascular death or HF hospitalization (57, 58). Ivabradine, by specifically inhibiting the cardiac pacemaker current in the sinoatrial node, reduces heart rate. The SHIFT study demonstrated that ivabradine could reduce the relative risk of cardiovascular death and hospitalization by 18%, while also significantly improving left ventricular function and quality of life in HF patients (59–61). Digitalis acts by inhibiting Na+/K+-ATPase, producing a positive inotropic effect, enhancing parasympathetic nerve activity, and reducing atrioventricular conduction. Studies have shown that long-term use of digoxin in HF patients does not affect mortality but reduces overall hospitalization rates and HF-related hospitalizations (62, 63). Trimetazidine improves myocardial mitochondrial function and glucose uptake through AMPK, alleviating pressure overload-induced HF (64). A recent meta-analysis demonstrated that trimetazidine significantly reduces cardiovascular mortality and HF hospitalization rates in HFrEF patients (65). Other emerging or experimental drugs include tafamidis for transthyretin amyloid cardiomyopathy (66), the myosin activator (omecamtiv mecarbil) (67) and the interleukin-1β inhibitor (canakinumab) (68), all of which hold promise for future clinical application.
Devices and surgical interventions also play a crucial role in HF treatment. Coronary artery bypass grafting (CABG) and percutaneous coronary intervention (PCI) can be performed to revascularize patients with HF. In the STICH trial, revascularization via CABG in patients with HFrEF was shown to reduce long-term mortality (69). However, in the REVIVED-BCIS2 trial, in patients with severe ischemic LV systolic dysfunction treated with optimal medications, revascularization by PCI did not reduce the incidence of death from any cause or hospitalization for HF (70). Currently, the most widely used cardiac implantable electronic devices mainly include CRT and ICD. CRT is used in HF patients with dyssynchronous ventricular contraction, improving atrioventricular and biventricular contraction synchrony, thus alleviating clinical symptoms, enhancing exercise tolerance and quality of life, reversing ventricular remodeling, improving cardiac function, and reducing HF-related hospitalization and mortality. It represents a milestone in device-based therapy for HF. Traditional biventricular pacing CRT has been used for the treatment of chronic HF for over 30 years. However, despite continual updates in CRT technology, approximately one-third of patients still experience treatment failure or non-response, resulting in no clinical benefit. In recent years, the emergence of conduction system pacing (CSP), as a new approach for CRT, has addressed some of these issues. CSP includes His bundle pacing (HBP) and left bundle branch area pacing (LBBAP), which directly stimulate the heart's native conduction system and offer greater benefits compared to traditional right ventricular pacing (71). Some recent clinical studies have demonstrated the feasibility and efficacy of CSP in CRT, showing not only improved synchrony of cardiac contraction in HF patients but also enhanced echocardiographic parameters and clinical outcomes (72–74). However, since CSP is a relatively new approach, its long-term clinical efficacy and safety still require further validation through large-scale, randomized controlled trials. The 2023 guidelines from the American Heart Rhythm Society raised the recommendation level for HBP and LBBAP in preventing and mitigating HF (75).CRT is limited to HF patients with widened QRS complexes and dyssynchronous ventricular contraction. The advent of the cardiac contractility modulation (CCM) device fills the gap in treating HF patients with narrow QRS complexes (76, 77). CCM is a novel device that treats chronic HF by delivering high-energy (approximately 7.5 V), long-duration (about 20 ms) electrical signals to the right ventricular septum during the absolute refractory period to enhance myocardial contractility. This stimulation does not generate an electrical activation in the myocardium but instead modulates calcium ion channels, increasing calcium ion regulation in cells, thus enhancing myocardial contractility and reversing ventricular remodeling (78). CCM represents a groundbreaking therapeutic device. Clinical studies have shown that CCM improves symptoms in HF patients, increases exercise tolerance, enhances quality of life, and reduces HF-related rehospitalization rates (79–82). However, there is a lack of prospective randomized controlled trials to assess whether long-term CCM therapy can reduce mortality in HF patients and improve long-term outcomes. It is also unclear whether the incidence of ventricular arrhythmias will increase, and further validation is needed. Moreover, all studies and trials on CCM have focused on left-sided HF, and no studies have yet evaluated the impact of CCM on patients with right-sided HF or diastolic HF, who currently lack effective treatment options. HF patients, particularly those with ischemic cardiomyopathy and significantly reduced left ventricular ejection fraction (LVEF ≤ 35%), face a high risk of sudden cardiac death (SCD) (83). Prophylactic ICD implantation can reduce the rate of SCD in HF patients (84). However, potential complications of traditional ICD implantation include device dislocation, pneumothorax, hematoma, and infection (85). To minimize these complications, the subcutaneous implantable cardioverter-defibrillator (S-ICD) has been developed. Compared to traditional ICDs, the S-ICD is safer, more effective, and more suitable for patients with difficulties in venous access, catheter-related complications, a high risk of infection, or those who are younger (86). Excessive sympathetic activation and reduced parasympathetic activity are compensatory mechanisms in HF that lead to cardiac damage, congestion, and decreased stroke volume. Therefore, neuromodulation has emerged as a new therapeutic approach for HF. Baroreflex activation therapy (BAT) involves electrical stimulation of the carotid baroreceptors, resulting in a centrally mediated reduction in sympathetic signals and an increase in parasympathetic signals, thereby improving the imbalance in autonomic regulation in HF patients and achieving therapeutic effects (87). Studies such as HOPE-4HF (88) and BeAT-HF (89) have confirmed that BAT significantly improves New York Heart Association (NYHA) functional classification, 6-min walk distance, and left ventricular ejection fraction (LVEF). Sleep apnea is a common comorbidity in HF patients, significantly affecting cardiovascular function (90). Sleep apnea can be classified into obstructive sleep apnea (OSA) and central sleep apnea (CSA).CSA is closely associated with HF, with 30%–50% of HF patients developing CSA, and its prevalence increases as HF severity worsens (91, 92). Phrenic nerve stimulation (PNS) has been shown to improve the apnea-hypopnea index and quality of life in CSA patients, while also demonstrating good safety (93, 94). Mechanical circulatory support (MCS) systems, particularly extracorporeal life support and extracorporeal membrane oxygenation (ECMO), can be used as “bridge therapies” for patients with acute and rapidly deteriorating HF or cardiogenic shock to stabilize hemodynamics, restore end-organ function, and buy time for further interventions such as heart transplantation or other MCS devices (95–97). For patients with end-stage HF, heart transplantation remains the only viable treatment option (16, 98).
Over the past few decades, significant advancements have been made in the treatment of HF. However, the prognosis for HF patients remains unsatisfactory. In the ESC-HF-LT study, the 1-year all-cause mortality rate for acute heart failure (AHF) was 23.6%, and for chronic heart failure, it was 6.4% (99). The combined endpoint of 1-year mortality or HF-related hospitalization was 36% for AHF and 14.5% for CHF (99). The 5-year survival rate for HF patients remains below 50% (100), and the mortality rate from HF is on the rise (101). There is still much to explore in the treatment of HF. Given that stem cells can differentiate into functional cardiomyocytes or enhance the survival of cardiomyocytes through paracrine functions, stem cell therapy holds potential for the treatment of HF.
4 Origin and biological characterization of mesenchymal stem cells
In the 1970s, Friedenstein et al. first described MSCs as spindle-shaped, adherent, non-hematopoietic stem cells located in mouse bone marrow (102). Later, in 1991, Caplan and colleagues named the cells isolated by Friedenstein as MSCs (103, 104). Since then, MSCs have become widely known as multipotent stem cells with self-renewal and immunomodulatory properties, attracting increasing attention from researchers due to their hidden clinical value. MSCs can be isolated from various adult tissues such as bone marrow, umbilical cord, placenta, adipose tissue, peripheral blood, liver, and dental pulp (105, 106), and they exhibit excellent self-renewal capacity in vitro, capable of persisting for over four months (107).
MSCs possess multipotent differentiation, low immunogenicity, immunomodulatory, and anti-inflammatory properties. When cultured MSCs are stimulated by certain cytokines, they can generate osteoblasts, chondrocytes, adipocytes, skeletal muscle cells, neurons, endothelial cells, and even cardiomyocytes (108–112). The International Society for Cellular Therapy (ISCT) has published the gold standard for MSCs: MSCs are distinguished from other cell types by expressing CD73, CD90, and CD105 on their surface while lacking expression of CD45, CD34, CD14, CD19, CD11b, and human leukocyte antigen (HLA)-DR (113, 114). Most importantly, MSCs exhibit low expression of major histocompatibility complex class II (MHC-II), the Fas ligand, and co-stimulatory moleculemolecules (B7-1, B7-2, CD40, and CD40l) on their surface (115, 116), indicating their low immunogenicity. Furthermore, MSCs inhibit the proliferation of T cells, B cells, natural killer (NK) cells, and dendritic cells (DCs). MSCs can block various immune cell functions, including cytokine secretion and cytotoxicity of T and NK cells, B cell maturation and antibody secretion, DC maturation and activation, and antigen presentation (117). This highlights the immunomodulatory activity of MSCs. MSCs protect tissues or organs from excessive inflammation through several mechanisms (118). First, MSCs express IL-1 receptor antagonist, downregulating IL-1 expression (119, 120). Second, MSCs establish a negative feedback loop in which macrophage-secreted TNF-α and other pro-inflammatory cytokines activate MSCs to secrete multifunctional anti-inflammatory proteins such as TNF-α-stimulated gene/protein 6 (TSG-6) and prostaglandin E2 (PGE2). TSG-6 reduces nuclear factor-κB (NF-κB) signaling in resident macrophages, and PGE2 stimulates the polarization of macrophages from a pro-inflammatory M1 phenotype to an anti-inflammatory M2 phenotype, thereby regulating the pro-inflammatory cytokine cascade (121). Third, MSCs establish a second negative feedback loop in which endotoxins, nitric oxide, or other damage-associated molecular patterns from injured tissues and macrophages activate MSCs to secrete PGE2, transforming macrophages into an IL-10-secreting phenotype (122). Thus, MSCs exhibit anti-inflammatory effects. In summary, the low immunogenicity, immunomodulatory, and anti-inflammatory properties of MSCs make them advantageous for cell therapy. These unique characteristics endow MSCs with potential and attractiveness in the field of regenerative medicine.
5 Different tissue sources of MSCs and their characteristics
5.1 Bone marrow mesenchymal stem cells (BM-MSCs)
Bone marrow-derived MSCs were the first MSCs to be described, known as BM-MSCs. BM-MSCs can be isolated from the sternum, vertebrae, iliac crest, and femoral shaft (123–125). Compared to other types of MSCs, the process of obtaining BM-MSCs is invasive. BM-MSCs are collected through specific bone marrow aspiration, and stem cell isolation can be quickly and efficiently performed using flow cytometry and MACS technology based on the expression of cell surface markers (126, 127). In addition to meeting the ISCT standards, BM-MSCs express other surface markers, such as STRO-1, CD146, SSEA-4, CD271, MSCA-1, and PDGFRα (128, 129). Among these, STRO-1 is highly expressed in fresh and young BM-MSCs and decreases during expansion cultures, making STRO-1-positive BM-MSCs suitable for clinical applications (129). BM-MSCs exist in very low quantities in bone marrow tissue and undergo rapid proliferation after seven days of culture, achieving a 500-fold expansion in 50 passages (130). Under phase-contrast microscopy, the dominant cells appear spindle-shaped or pool-like with abundant cytoplasm and large, dark nucleoli. After in vitro expansion, a sufficient number of cells can be obtained for therapeutic doses.
5.2 Adipose-derived mesenchymal stem cells (A-MSCs)
A-MSCs were first isolated from human adipose tissue through plastic adherence before MSCs were formally defined (131). In 2001, Zuk et al. identified MSCs in human adipose tissue and characterized them (132), which led to the recognition that MSCs isolated from adipose tissue could serve as an alternative to BM-MSCs. Adipose tissue consists mainly of adipocytes organized into lobules. It is a highly complex tissue composed of mature adipocytes that account for over 90% of the tissue volume, along with a heterogeneous group of cells surrounding and supporting the adipocytes. After enzymatic digestion and/or mechanical disruption, single-cell populations can be released from adipose tissue, forming the stromal vascular fraction (SVF) (133). Centrifugation separates the SVF from mature adipocytes. The supernatant contains low-density, floating adipocytes, while the SVF forms a denser cell pellet. The SVF is a heterogeneous mixture of various cell populations with different levels of maturity and function, including pericytes, smooth muscle cells, and A-MSCs (134). A-MSCs are further isolated from the SVF through expansion and plastic adherence, similar to BM-MSCs, to deplete most hematopoietic cell populations. Factors such as donor age, donor BMI, tissue type (white or brown adipose tissue), and tissue harvesting procedures and locations can affect the yield, proliferation rate, and differentiation capacity of A-MSCs (134–138). Compared to A-MSCs from older patients, A-MSCs from younger patients exhibit higher proliferation rates and more effectively differentiate into mature adipocytes (134). Increased BMI reduces A-MSCs proliferation and may impair in vitro osteogenic potential (135). Although there are two types of adipose tissue (brown and white), white adipose tissue is the source of A-MSCs used in most studies (136). Additionally, ultrasound-assisted liposuction reduces the proliferation frequency of A-MSCs and prolongs their population doubling time compared to excision methods (137). The yield of A-MSCs also depends on the tissue harvest site, with the abdomen being more suitable for A-MSCs collection than the hip/thigh region (138). A-MSCs possess the same characteristics as standard MSCs, meeting ISCT criteria. However, CD34 expression has been a unique feature of A-MSCs, with studies showing that a significant portion of A-MSCs are CD34-positive, although negative results for CD34 have also been reported (139). Evidence suggests that CD34 is expressed in tissue-resident A-MSCs, with negative findings being the result of cell culture, indicating that CD34 expression may be reversible (140). A report by Suga et al. indicated that CD34 expression is associated with immature, pro-angiogenic gene expression and greater replicative potential (141). It is speculated that CD34 is a niche-specific marker of immature/early progenitor cells that is lost under in vitro conditions (133, 142).
5.3 Placenta-derived mesenchymal stem cells (P-MSCs)
The placenta is a transient maternal-fetal organ that is highly abundant and easily accessible. Since it is discarded after delivery, its collection does not involve invasive procedures, making it more ethically acceptable. This has garnered significant interest in the placenta as a source of MSCs. P-MSCs can be isolated from various parts of the placenta, such as the chorion, basal decidua, top decidua, amnion, and amniotic fluid (143–146). P-MSCs are typically isolated using explant or enzymatic methods. In the explant method, tissue is dissected from the respective source, washed with cold phosphate-buffered saline to remove excess blood, minced into small pieces, and allowed to adhere to culture dishes. The plates are incubated at 37 °C with MSCs proliferation medium. In the enzymatic method, the tissue is similarly minced and treated with trypsin, DNase, and collagenase. The isolated cells are then plated and maintained similarly to explant cultures. P-MSCs exhibit high immunoregulatory properties by secreting soluble factors and modulating the immune functions of macrophages and dendritic cells, thereby inhibiting T cell activity and enhancing their immunosuppressive capabilities (144). Current literature suggests that P-MSCs hold great potential as a cell source for therapeutic angiogenesis. P-MSCs secrete a large number of cytokines and chemokines crucial for angiogenic signaling, promoting angiogenesis by modulating niche cells (147).
5.4 Umbilical cord mesenchymal stem cells (HUC-MSCs)
The human umbilical cord is an extra-embryonic connective tissue located between the mother and fetus, consisting of two arteries, one vein, vascular connective tissue, and umbilical epithelium. The connective tissue, also known as Wharton's jelly, is a spongy structure woven from collagen fibers, proteoglycans, and embedded stromal cells. The umbilical cord is a rich source of MSCs, with minimal ethical concerns. Its collection and isolation process is painless, minimally invasive, and the tissue is easy to store and transport (148). HUC-MSCs can be isolated from different parts of the umbilical cord, including blood, endothelial cells of the umbilical vein, and Wharton's jelly (149). Similar to P-MSCs, HUC-MSCs are typically isolated using explant or enzymatic methods (150). The surface markers of HUC-MSCs are similar to those defined by the ISCT for MSCs, although they are negative for CD133 (151). The phenotypic characteristics of HUC-MSCs may be influenced by the number of culture passages, culture medium, and methods used. HUC-MSCs can exert immunosuppressive effects by inhibiting the proliferation of T cells, B cells, and NK cells, and by directing monocytes and dendritic cells towards an immature state (152, 153).
5.5 Comparison of MSCs from four sources
Flow cytometric analysis using MSCs from different tissues revealed that all cell types exhibit similar immunophenotypes. MSCs are positive for CD29, CD44, CD73, CD90, and CD105, which are known MSC markers, with positive markers expressed across all cell types (154). These results confirm that MSCs from different sources express the surface markers defined by the ISCT. However, CD90, a typical MSC marker, is less pronounced in P-MSCs compared to other cells (154). In addition to surface markers, MSCs from different tissue sources have varying lineage differentiation capabilities. When MSCs from bone marrow, umbilical cord, adipose tissue, and placenta are cultured in vitro, BM-MSCs exhibit a longer population doubling time than other MSC types (154). P-MSCs can undergo more passages compared to other MSC types during passaging (154). In colony-forming unit (CFU) assays, BM-MSCs and HUC-MSCs produce more colonies than other MSC types when an equal number of MSCs are plated, indicating stronger self-renewal characteristics (154). After differentiation, BM-MSCs and AT-MSCs exhibit significant differentiation potential, generating osteoblasts, adipocytes, and chondrocytes, whereas MSCs derived from umbilical cord blood and placenta show weaker differentiation potential (154).
6 Mesenchymal stem cells (MSCs) and cardiomyocytes
The clinical application of MSCs began in the 1990s, initially focusing on the treatment of tumors, bone, and cartilage diseases, and demonstrated promising results. Studies have also shown the therapeutic potential and safety of using MSCs to treat various diseases. MSCs have gradually attracted attention from researchers in the field of cardiology. In preclinical models, MSCs have been used to improve heart injuries, and recent clinical trials on the safety and efficacy of MSCs therapies for heart injuries have yielded exciting and positive results. The mechanisms through which MSCs therapies benefit the heart are becoming clearer. Extensive research has shown that the beneficial effects of MSCs are not solely due to their ability to promote cardiomyocyte regeneration, which contributes only minimally. More importantly, MSCs exert paracrine effects, mediating immunomodulation and anti-inflammatory responses, thereby protecting the heart and promoting repair, while also influencing ECM remodeling favorably.
6.1 MSCs promote cardiomyocyte differentiation and regeneration
Early studies on the differentiation of MSCs into cardiomyocytes demonstrated that specific microenvironmental conditions, such as treatment with 5-azacytidine and platelet-derived growth factor-β, could induce MSCs differentiation into cardiomyocytes (155, 156). However, in another study, rat BM-MSCs failed to differentiate into cardiomyocytes following 5-azacytidine treatment (157). In fact, the regulatory mechanisms of MSCs multipotency are highly complex. Nevertheless, increasing evidence suggests that the activation or inhibition of certain transcription factors can guide MSCs towards specific lineage differentiation. Through exogenous overexpression or knockout of candidate genes, some key transcription factors, such as GATA4, Nkx2.5, myocardin, thioredoxin-1, and Notch1, can specifically promote the differentiation of MSCs into cardiomyocytes expressing ANP and cTnI (158, 159). Additionally, pre-treatment with TGF-β1 has been shown to enhance the differentiation of rat BM-MSCs into cardiomyocytes and improve cardiac function in a rat heart failure model (160). For stable and efficient cardiomyocyte generation from MSCs, co-treatment with 5-Aza, BMP-2, and DMSO can induce MSCs differentiation into cardiomyocyte-like cells within 24 h (161). Recent studies have shown that human amniotic MSCs (hAMSCs) can also be chemically induced to differentiate into cardiomyocyte-like cells in vitro using a chemical induction protocol that includes BMP4, Activin A, 5-azacytidine, CHIR99021, and IWP2 (162). These studies were conducted in vitro, but MSCs can also differentiate into functional cardiomyocytes in vivo under appropriate conditions. For instance, when human MSCs expressing the β-galactosidase reporter gene were transplanted into immunodeficient mice with experimental myocardial injury, β-galactosidase-expressing cardiomyocytes were found in the host myocardium, indicating that xenografted MSCs had differentiated into cardiomyocytes (163). Moreover, in gender-mismatched cell therapy experiments using porcine models of acute and chronic myocardial infarction, transplanted male MSCs were found to differentiate into cardiomyocytes in female host myocardium. This was confirmed by the co-localization of the Y chromosome with the cardiac transcription factors GATA-4, Nkx2.5, and the structural cardiac protein α-sarcomeric actin (164–166). These findings suggest the potential for MSCs to differentiate into cardiomyocytes in vivo.
6.1.1 Controversies and limitations of differentiation efficiency
Although multiple studies in rodent and porcine models have confirmed the potential of MSCs to differentiate into cardiomyocytes, their differentiation efficiency remains extremely low (engraftment rate <1%, differentiation proportion 10%–14%), with marked interspecies variability and instability of long-term engraftment. For instance, using β-galactosidase labeling, Toma et al. reported that only 0.44% of human MSCs injected into mouse hearts engrafted into myocardial tissue, and merely a small proportion of these cells exhibited cardiomyocyte-like phenotypes by expressing markers such as desmin, α-actin, and cardiac troponin T (163). In a porcine model of chronic ischemic cardiomyopathy, Y chromosome tracking revealed that only 14% of the engrafted MSCs expressed cardiac-specific markers (α-sarcomeric actin, troponin T, and transcription factors GATA-4/Nkx2.5), whereas 76% of the engrafted cells remained in an immature mesenchymal state (164). Species-specific differences further constrain their differentiation potential; in canine models, MSCs differentiated exclusively into vascular-like cells, with no detectable co-localization of cardiac troponin I (167). More critically, Dixon et al. demonstrated in a sheep myocardial infarction model that although transplanted MSCs were detectable within 1 h, they completely disappeared by week eight (Y chromosome PCR negative), indicating a fundamental deficiency in their capacity for long-term engraftment and integration (168).
Collectively, these findings suggest that the contribution of MSCs to cardiac functional improvement via direct differentiation into cardiomyocytes is minimal. Recent studies increasingly support the notion that the therapeutic effects of MSCs are primarily mediated through their robust paracrine activity—by secreting a repertoire of bioactive factors, including cytokines, growth factors, and extracellular vesicles (EVs), MSCs modulate the immune microenvironment, suppress inflammation and fibrosis, promote angiogenesis, and activate endogenous repair pathways. This paradigm shift provides a novel theoretical framework for optimizing stem cell-based therapeutic strategies.
6.2 The paracrine role of MSCs
Although MSCs can exert beneficial effects on the heart through various mechanisms, the paracrine activity of MSCs has recently become a focal point of therapeutic research. Multiple growth factors and cytokines are secreted together as part of the “secretome.” These factors can be water-soluble or encapsulated in EVs or exosomes cultured in “conditioned media.” The secreted paracrine factors have diverse effects, such as antifibrotic, anti-apoptotic, and angiogenic properties, which improve local vascularization and reduce cardiomyocyte death. The paracrine function of MSCs is primarily attributed to their derived exosomes, which will be discussed in detail later.
7 Exosomes derived from mesenchymal stem cells
7.1 Brief introduction to exosomes
Exosomes are EVs with diameters ranging from 40 to 160 nm (169). They are secreted by various cell types, including dendritic cells, mast cells, platelets, and MSCs, and are present in most human and animal body fluids, such as plasma, serum, saliva, amniotic fluid, breast milk, and urine (170). Exosomes were first discovered by Pan and Johnstone while studying how sheep reticulocytes mature into red blood cells (171). They carry crucial information and macromolecules from their source cells, playing a vital role in intercellular communication. These macromolecules, which include various proteins, enzymes, transcription factors, lipids, ECM proteins, receptors, and nucleic acids, can be found inside or on the surface of exosomes (170). Initially, exosomes were considered to be cellular debris or waste and were thought to be indicators of cell death (172, 173). Since their discovery, the biological properties, functions, and potential clinical applications of exosomes have been extensively studied. Typical biomarkers of exosomes include proteins such as CD9, CD81, CD63, ceramide, tumor susceptibility gene 101 (TSG101), and apoptosis-linked gene 2-interacting protein X (ALIX), all of which are involved in exosome origin and biogenesis (170, 174). The most common and traditional method for isolating exosomes is ultracentrifugation/differential ultracentrifugation, which separates exosomes based on size and density (175). Other methods include ultrafiltration, size-exclusion chromatography (SEC), and molecular weight-based exosome separation (175). Immunoaffinity chromatography and precipitation can also be used to enhance the purity of isolated exosomes (174).
MSCs promote angiogenesis and possess the ability to restore ischemic tissues, making them highly attractive for treating cardiovascular diseases (176). The beneficial effects of MSCs in tissue repair are largely attributed to paracrine signaling, including secreted vesicles, mainly exosomes (177). Since exosomes contain various regulatory RNAs, they have the potential to reduce tissue damage after myocardial infarction (178). Exosomes derived from different MSCs sources generally have common therapeutic effects, though their specific mechanisms vary slightly, likely due to differences in their microRNA (miRNA) content (179, 180). miRNAs, important gene expression regulators, are abundant in exosomes. They can suppress the expression of pro-apoptotic proteins, increase anti-apoptotic protein expression, improve angiogenic gene expression, mitigate oxidative stress, limit the proliferation of cardiac fibroblasts, create an anti-inflammatory microenvironment, and enhance cardiac function. These effects promote cardiomyocyte survival and improved function in various myocardial infarction or heart failure models (178, 181). Interestingly, recent studies have demonstrated that exosomes secreted by MSCs can replace MSCs-based stem cell therapy in various injury and disease models (182, 183). For example, MSC-derived exosomes (MSC-Exos) have been shown to induce repair in mouse models of wound healing and myocardial infarction (184–186). Our next aim is to elucidate the molecular mechanisms of MSC-derived exosomes to facilitate their clinical translation (as shown in Figures 1–4).
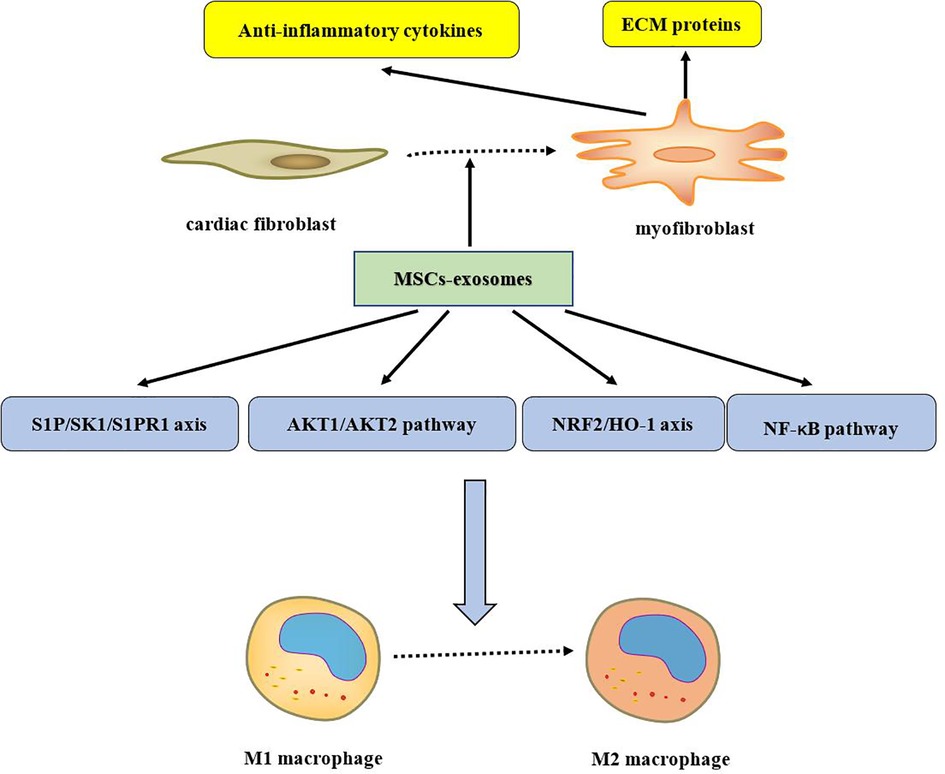
Figure 1. ECM, extracellular matrix; MSCs, mesenchymal stem cells; S1P/SK1/S1PR1, sphingosine-1-phosphate/sphingosine kinase 1/sphingosine-1-phosphate receptor 1; AKT, Ak strain transforming; NRF2/HO-1, nuclear factor erythroid 2-related factor 2/heme oxygenase-1; NF-κB, nuclear factor kappa-B.
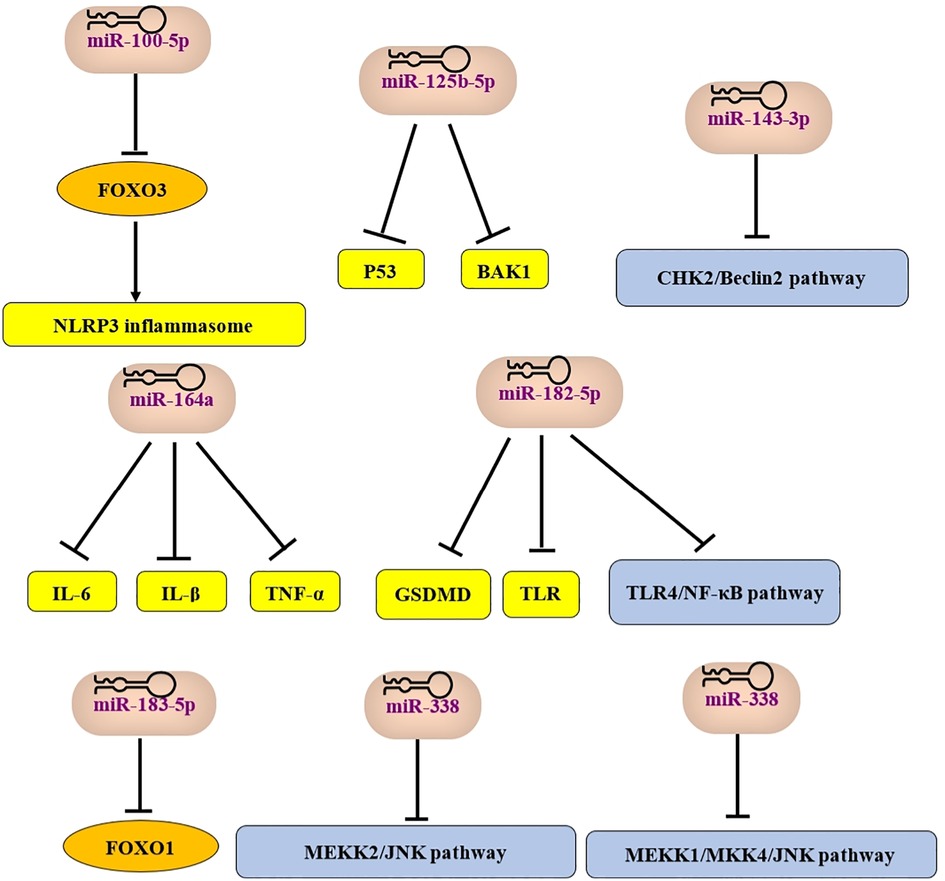
Figure 2. Mir, microRNA; FOXO, Forkhead box O; BAK, Bcl-2 homologous antagonist/killer; CHK2, checkpoint Kinase 2; IL, interleukin; TNF-α, tumor necrosis factor-α; GSDMD, gasdermin D; TLR, toll-like receptor; MEKK, Mitogen-Activated Protein Kinase Kinase Kinase 2; JNK, c-Jun N-terminal Kinase.
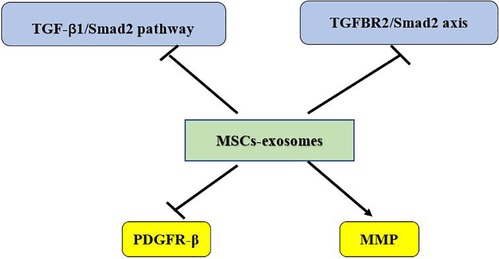
Figure 3. TGF, transforming growth factor; TGFBR2, transforming growth factor Beta receptor type II; PDGFR, platelet-derived growth factor receptor; MMP, matrix metalloproteinase.
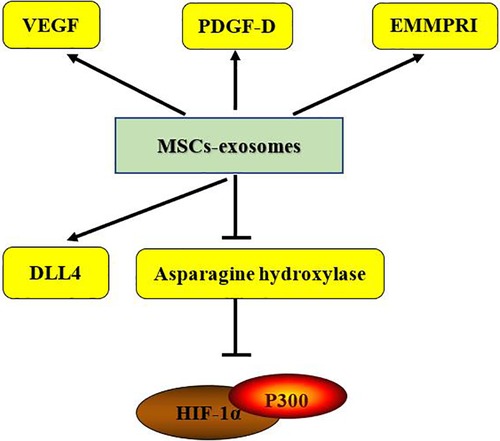
Figure 4. VEGF, vascular endothelial growth factor; PDGF, platelet-derived growth factor; EMMPRIN, extracellular matrix metalloproteinase inducer; DLL-4, delta-like 4; HIF, hypoxia-inducible factor.
7.2 Exosomes and cardiomyocytes
7.2.1 Cardioprotective effects
(The cardioprotective effects of MSCs-exosome are shown in Figure 1, where miRNA effects are shown in Figure 2).
Cardiomyocytes have a high oxygen demand, making them highly susceptible to hypoxia. During hypoxia and ischemia, cardiomyocytes are prone to apoptosis, oxidative stress, and excessive autophagy activation, which ultimately leads to impaired cardiac function. Increasing research has shown that exosomes derived from MSCs play a crucial role in anti-apoptotic, anti-oxidative stress, and autophagy regulation mechanisms.
Myocardial ischemia/reperfusion (I/R) injury can exacerbate apoptosis, necrosis, and cell death (187). During myocardial I/R injury, cardiomyocyte death activates inflammatory responses, which significantly impact the extent of myocardial damage. The activation of inflammatory cytokines can induce pyroptosis, leading to the release of intracellular contents into the extracellular environment. This, in turn, triggers further inflammatory responses, exacerbating myocardial damage in a vicious cycle (188). Prolonged or excessive inflammation degrades the ECM, leading to ventricular remodeling and eventually heart failure. Exosomes have been shown to inhibit inflammation by downregulating inflammatory cytokine expression and promoting the transition of macrophages from the pro-inflammatory M1 phenotype to the anti-inflammatory M2 phenotype. Macrophages play a critical role in both the progression and resolution of inflammation. M1 macrophages secrete large amounts of pro-inflammatory factors, while M2 macrophages produce various anti-inflammatory factors and growth factors (189). After myocardial injury, M1 macrophages are recruited to the damaged myocardium, exhibiting strong phagocytic and pro-inflammatory activities, after which M2 macrophages dominate to resolve inflammation and promote myocardial repair (190). Studies have shown that exosomes derived from BM-MSCs activate the AKT1/AKT2 signaling pathway and the nuclear factor erythroid 2-related factor 2/heme oxygenase-1 (NRF2/HO-1) axis, while inhibiting the NF-κB signaling pathway. This signaling shift promotes macrophage polarization from the M1 to the M2 phenotype, aiding in the resolution of post-myocardial injury inflammation (191, 192). Treating BM-MSCs with fibronectin type III domain-containing protein 5 (FNDC5) can further enhance the ability of exosomes to promote macrophage polarization (192). Similar to BM-MSC-derived exosomes, exosomes from A-MSCs also facilitate the M1-to-M2 transition and suppress inflammation after myocardial injury. However, the mechanism may differ, as this effect is achieved through the activation of the sphingosine-1-phosphate/sphingosine kinase 1/sphingosine-1-phosphate receptor 1 (S1P/SK1/S1PR1) axis (193). Not all exosomes regulate inflammation through macrophage polarization. For example, HUC-MSCs exosomes exert anti-inflammatory effects via cardiac fibroblasts. After myocardial injury, HUC-MSC-derived exosomes promote the differentiation of CFs into myofibroblasts, which secrete anti-inflammatory cytokines and ECM proteins, thereby reducing inflammation and stabilizing cardiac tissue (194).
MiRNAs in exosomes play a critical role in cardioprotection. MiRNAs within exosomes derived from BM-MSCs can coordinate the suppression of genes that drive cardiomyocyte apoptosis and cardiac injury. For example, in a mouse model of myocardial infarction, hypoxia-derived exosomal miR-125b-5p exhibited cardioprotective functions by inhibiting the expression of apoptotic genes p53 and BAK1, thereby enhancing cardiac repair and reducing cardiomyocyte apoptosis (195). Exosomes from BM-MSCs carrying miR-183-5p can inhibit the expression of FOXO1, thus reducing cardiomyocyte apoptosis and oxidative stress (196). Exosomal miR-182-5p from BM-MSCs suppresses the expression of the pyroptosis-related protein gasdermin D (GSDMD), toll-like receptor 4 (TLR4), and the TLR4/NF-κB signaling pathway, thereby exhibiting strong anti-inflammatory and anti-pyroptotic effects (197, 198). Notably, BM-MSC-derived exosomes contain miRNAs capable of regulating signaling pathways. For instance, miR-338 alleviates cardiomyocyte apoptosis via the MEKK2/JNK pathway (199), while miR-455-3p inhibits the MEKK1-MKK4-JNK signaling pathway (200). Furthermore, miR-143-3p regulates autophagy by inhibiting the CHK2/Beclin2 pathway, effectively suppressing cardiomyocyte apoptosis (201). Exosomes derived from miR-146a-modified A-MSCs have been shown to reduce local inflammation by inhibiting the release of pro-inflammatory factors (IL-6, IL-1β, and TNF-α), thus alleviating myocardial injury (202). The same study reported that exosomes from A-MSCs improve cardiac function by preventing apoptosis through downregulation of early growth response factor 1 (EGR1) (202). Research has also demonstrated that HUC-MSC-derived exosomes provide additional cardioprotection by promoting the expression of Smad7 through the inhibition of miR-125b-5p in the injured myocardium (203). Additionally, HUC-MSC-derived exosomes containing miR-100-5p can downregulate FOXO3, thereby inhibiting NLRP3 inflammasome activation and protecting cardiomyocytes from pyroptosis and damage (204).
7.2.2 Anti-myocardial fibrosis
(The antimyocardial fibrosis effect of MSCs-exosome is shown in Figure 3).
Following myocardial injury, ischemia and cell death of cardiomyocytes trigger various repair responses, among which myocardial fibrosis is a critical process. Although the initial fibrotic response helps prevent ventricular wall rupture after myocardial infarction, excessive fibrosis leads to progressive cardiac dysfunction and eventually heart failure. Therefore, reducing excessive myocardial fibrosis is a key research focus for improving heart failure outcomes.
Recent studies have demonstrated that MSCs-derived exosomes can significantly reduce infarct size, fibrosis area, and cardiomyocyte apoptosis in models of MI. Compared with control-treated infarcted pigs, intravenous administration of exosomes within 7 days resulted in a marked reduction in infarct size and preservation of cardiac function (205). Moreover, in mouse models of MI, exosome-treated mice exhibited higher survival rates. MSC-derived exosome treatment significantly decreased leukocyte accumulation in and around the infarct zone, as well as the expression of LOX-1, NLRP3 inflammasomes, and cell death markers (206). The underlying mechanism involves the inhibition of fibroblast-to-myofibroblast transformation mediated by TGF-β, thus reducing myocardial fibrosis. Further research has shown that MSC-derived exosomes inhibit the TGF-β1/Smad2 signaling pathway to alleviate myocardial injury and fibrosis (207). Exosomes from A-MSCs carry miR-671, which reduces myocardial fibrosis and inflammation by inactivating the TGFBR2/Smad2 axis (208). BM-MSC-derived exosomes upregulate PDGFR-β, enhancing microvascular regeneration, inhibiting fibrosis, and maintaining long-term cardiac function after I/R injury (209). Notably, BM-MSC-derived exosomes exhibit better therapeutic efficacy than BM-MSCs themselves, indicating their potential for clinical application in future stem cell transplantation technologie (209). The remodeling of ECM plays a crucial role in the development of ventricular dilation and heart failure. Among the many proteases present in cardiac ECM, MMPs drive the degradation of cardiac matrix during remodeling. Increased MMP activity may lead to collagen degradation, heightened inflammation, ECM remodeling, and progressive ventricular dilation and dysfunction (210). Exosomes derived from U-MSCs have been reported to contain TIMP2, an MMP inhibitor, which suppresses ECM remodeling and collagen deposition, thereby preventing adverse cardiac remodeling (187).
7.2.3 Promotion of angiogenesis
(The vascular regeneration-promoting effects of the MSCs-exosome are shown in Figure 4.)
After myocardial injury, the ability of the affected region to generate new blood vessels is limited. Severe impairments in angiogenesis can exacerbate myocardial hypoxia, further aggravating myocardial injury. Insufficient capillary formation during heart failure promotes the transition from compensatory hypertrophy to decompensation, eventually leading to heart failure (211). This process is closely linked to the regulation of angiogenesis by endothelial cells. Cardiac endothelial cells, when stimulated by angiogenic factors such as vascular endothelial growth factor (VEGF), platelet-derived growth factor, and fibroblast growth factor, activate angiogenic signaling pathways, promoting angiogenesis (212). When the secretion of these angiogenic factors is insufficient, endothelial cell proliferation and migration are suppressed, worsening the lack of angiogenesis and heart failure (213). Studies have shown that exosomes derived from human amniotic fluid mesenchymal stem cells (hAFMSC-Exos) promote angiogenesis in endothelial cells and enhance vascular formation during cardiac fibrosis, potentially through the upregulation of VEGF expression (214). Other studies indicate that exosomes from HUC-MSCs improve angiogenesis and cardiac function by activating platelet-derived growth factor D (PDGF-D) (215). These findings suggest that MSC-derived exosomes can stimulate endothelial cells by promoting the secretion of angiogenic factors, thereby enhancing angiogenesis. In addition, exosomes secreted by adipose-derived MSCs (A-MSC-Exo) transfer miR-125a to endothelial cells, promoting angiogenesis by inhibiting the angiogenesis inhibitor delta-like 4 (DLL4) (216). Thus, A-MSC-Exo may serve as a novel angiogenic factor and a potential candidate for heart failure therapy. Previous studies have shown that hypoxia-inducible factor 1-α (HIF-1α) overexpressing exosomes derived from mesenchymal stem cells rescued the impaired angiogenic capacity, migration, and proliferation of hypoxia-pretreated human umbilical vein endothelial cells in vitro and mediated cardioprotection through the up-regulation of pro-angiogenic factors and enhancement of neointima formation (217). Subsequently, exosomes extracted from A-MSCs were found to contain miR-31, which down-regulates asparagine hydroxylase, an enzyme that blocks the binding of HIF-1α to the coactivator p300, thereby “rescuing” angiogenic gene expression (218). In addition, ECM metalloproteinase inducer (EMMPRIN) has been shown to promote angiogenesis through hypoxia-inducible factor-2α (HIF-2α)-mediated regulation of soluble vascular endothelial growth factor isoforms and its receptor VEGFR-2 (219). Another study showed that MSC-derived exosomes contain comparable levels of VEGF and EMMPRIN, and MSC-derived exosomes can stimulate angiogenesis via EMMPRIN (220).
8 Existing challenges and limitations of MSCs and their exosomes in heart failure therapy
MSCs and their exosomes have emerged as promising therapeutic approaches for HF, with several clinical trials demonstrating encouraging results. However, significant challenges remain. These limitations can be broadly categorized into four areas: (1) heterogeneity in therapeutic efficacy and unclear mechanisms of action, (2) lack of standardized protocols for clinical translation, (3) concerns regarding long-term safety and production quality, and (4) obstacles in implementing individualized therapy.
8.1 Heterogeneity in efficacy and unclear mechanisms
One of the major challenges in MSCs-based therapy for HF is the pronounced heterogeneity in treatment outcomes and the incomplete understanding of the underlying mechanisms. Numerous clinical trials have reported variable results, likely stemming from a complex interplay among factors such as cell processing methods, therapeutic regimens, and patient-specific pathological conditions. For instance, the C-CURE trial utilized bone marrow-derived MSCs preconditioned with a cardiogenic cocktail, leading to notable improvements including a 7% increase in LVEF, a 24.8 ml reduction in end-systolic volume, and a 62-m gain in 6-min walk distance (221). In contrast, the CHART-1 trial, which employed a fixed high-dose regimen of bone marrow-derived MSCs in end-stage HF patients, failed to meet its primary endpoints and showed only limited benefit in certain subgroups (222, 223). Further analysis suggests that the cardiopoietic preconditioning strategy in C-CURE may have significantly enhanced the reparative potential of the cells, while individualized dosing based on body weight may be more advantageous than fixed dosing schemes. Additionally, the pathological microenvironment in end-stage HF may compromise the viability and functionality of transplanted cells.
Significant variation in therapeutic efficacy is also observed among MSCs derived from different tissue sources. In the RIMECARD trial, umbilical cord-derived MSCs (UC-MSCs) led to greater improvements in LVEF compared with placebo, and UC-MSCs were found to express hepatocyte growth factor (HGF) at levels 55 times higher than bone marrow-derived MSCs, suggesting that differences in the paracrine profiles of MSCs may influence therapeutic outcomes (224). The MSC-HF trial highlighted inter-individual variability in the effectiveness of autologous bone marrow-derived MSCs, which may be attributable to baseline patient characteristics such as age, comorbidities, and the etiology of heart failure (225). Notably, the POSEIDON trial found spatially specific effects of MSCs in improving regional contractile function within scarred myocardial zones, indicating that the local myocardial microenvironment may critically influence therapeutic efficacy (226).
Although the prevailing view is that MSCs exert their therapeutic benefits mainly through paracrine mechanisms, the precise molecular pathways involved remain poorly understood. The CONCERT-HF trial revealed cell-type-specific therapeutic effects: MSCs primarily improved patient-reported quality of life, whereas c-kit-positive cardiac progenitor cells (CPCs) demonstrated superiority in reducing major adverse cardiovascular events—a difference whose mechanism remains to be elucidated (227). While HGF is considered a key effector molecule, the downstream signaling pathways by which it orchestrates antifibrotic and proangiogenic responses require further clarification. Exosomes, as crucial paracrine mediators, pose additional challenges due to their compositional complexity, and current methodologies lack the sensitivity and specificity to reliably identify the key bioactive components responsible for therapeutic effects. These mechanistic uncertainties hamper the optimization and broader clinical application of MSCs-based therapies.
8.2 Challenges in standardization for clinical translation
The clinical translation of MSCs therapy for HF faces numerous challenges, among which the standardization of cell preparation processes is particularly prominent. Significant variability exists in the cell culture systems used across clinical trials, directly impacting cell quality and therapeutic outcomes. For instance, in the MSC-HF trial, the average culture period was 46.9 ± 10.5 days (225), whereas the C-CURE trial required an additional 4–6 weeks of cardiopoietic preconditioning. Furthermore, only 75% of patients in C-CURE met the release criteria (>600 × 106 cells) (221). In addition, there is no standardized method for exosome isolation; ultracentrifugation and commercial kits vary in yield, purity, and bioactivity. Optimal dosage and delivery routes (e.g., local injection vs. systemic administration) also remain controversial (228, 229). In the CONCERT-HF trial, 25% of MSCs and 16% of CPCs were excluded due to insufficient cell quantity or activity (227), highlighting the sensitivity of the manufacturing process and the critical importance of quality control.
In terms of delivery techniques, the demand for precision remains inadequately addressed. Intramyocardial injection methods, such as NOGA-guided endocardial delivery, are technically complex and operator-dependent. Approximately 20% of patients in the POSEIDON and CONCERT-HF trials were unable to receive the full injection protocol due to anatomical limitations (226, 227). Although the C-Cathez™ catheter used in the CHART-1 trial improved procedural efficiency, it still resulted in four cases of cardiac tamponade (222). Emerging technologies, such as real-time MRI-guided injections and robotic-assisted delivery systems, have shown promise in enhancing targeting accuracy; however, the associated costs are five to eight times higher than conventional approaches, limiting widespread adoption (230). Additionally, the application of exosomes in tissue repair is constrained by their low stability and short retention time. Studies have shown that exosomes are undetectable as early as 3 h post-myocardial injection (231). Moreover, systemic delivery faces targeting inefficiencies, with less than 1% of intravenously administered exosomes reaching the cardiac tissue (232, 233). Thus, future research should explore myocardial-specific modification strategies to improve delivery efficiency.
Another major barrier is the lack of consensus on endpoint measures, which limits comparability across clinical trials. Current studies use highly heterogeneous primary outcomes, including LVEF, left ventricular end-systolic volume (LVESV), scar size (assessed via MRI), and quality of life scores. However, some functional endpoints, such as the 6-min walk test, are susceptible to placebo effects—improvements of up to 30% have been observed in placebo groups in certain trials. Therefore, there is an urgent need to establish more objective and comprehensive composite endpoints that integrate imaging parameters (e.g., global longitudinal strain, GLS), biomarkers (e.g., NT-proBNP), and hard clinical outcomes (e.g., HF rehospitalization, cardiovascular mortality) to enhance the comparability and translational value of research findings.
8.3 Long-term safety and manufacturing challenges
The clinical translation of MSC therapy for HF is further hampered by long-term safety concerns and manufacturing limitations. Most current trials have relatively short follow-up durations (median 12–39 months), and potential risks such as tumorigenicity and immune rejection have yet to be fully elucidated. For example, in the RIMECARD trial, one patient treated with umbilical cord-derived MSCs was diagnosed with acute lymphoblastic leukemia 13 months later. Although trace-back analysis found no direct link to the treatment, the genomic instability that may result from replicative senescence during prolonged in vitro expansion of allogeneic MSCs remains a concern (224, 234, 235). While exosomes circumvent the risk of uncontrolled cell proliferation, their long-term immunomodulatory effects remain debatable. For instance, overexpression of programmed death-ligand 1 (PD-L1) may impair immune surveillance, a potential risk that warrants further validation through extended follow-up and larger sample sizes (236).
The scalability of the manufacturing process presents another critical bottleneck. Autologous MSCs therapies typically require 6–8 weeks from cell harvest to administration (as in the C-CURE trial), resulting in high costs and delayed treatment (223, 237). Donor-dependent variability in cell quality further complicates production. Compared with younger donors, MSCs from elderly patients show a 40%–60% reduction in proliferative capacity, leading to marked differences in therapeutic efficacy between batches (237–239). Although allogeneic umbilical cord-derived MSCs offer the advantage of cryopreserved “off-the-shelf” availability, post-thaw viability may decrease by 15%–30%, and the long-term effects of cryostorage on functional potency remain unclear (240). Exosome production faces its own set of technical limitations—fewer than 1 μg of exosomal protein can be harvested per milliliter of culture medium. Moreover, prolonged storage at −80 °C can compromise biological activity, hindering large-scale application (241–243).
The limitations of current quality control systems further exacerbate the complexity of clinical translation. Conventional assays (e.g., flow cytometry for phenotypic analysis) are time-consuming and insufficient for real-time clinical quality control. Although emerging technologies such as Raman spectroscopy offer real-time monitoring potential, they have not yet been standardized for clinical use (244–246). Exosome quality control is particularly challenging. Only a limited number of laboratories worldwide can simultaneously assess nanoparticle size distribution (via nanoparticle tracking analysis), purity markers (e.g., CD81/CD63), and functional potency (e.g., cell migration assays) (247, 248). Additionally, the absence of standardized functional evaluation systems—such as quantification of paracrine factors or assessments of tissue repair capacity—makes it difficult to ensure consistency between production batches (248, 249). These technical gaps not only compromise the predictability of therapeutic outcomes but also complicate regulatory approval processes. Moving forward, the development of automated quality control platforms and functional performance-based evaluation standards is crucial to overcoming current technological barriers and advancing stem cell therapies toward standardized and precise clinical application.
8.4 Barriers to personalized implementation
The personalized application of MSCs for heart failure remains hindered by multiple technical bottlenecks and translational challenges. Currently, patient stratification relies primarily on coarse indicators such as LVEF and the NYHA functional classification. However, post hoc analyses of the CHART-1 trial suggest that left ventricular end-diastolic volume (LVEDV) may serve as a more precise stratification marker (222).
The integration of multi-omics technologies offers promising avenues for precise stratification. Transcriptomic analysis has identified TNF-α as a key regulator of MSCs functionality; while TNF-α can activate the reparative and immunomodulatory capacities of MSCs via the inflammatory microenvironment, prolonged exposure or excessive concentrations may lead to functional exhaustion. Therefore, fine-tuning of TNF-α signaling is critical for optimizing MSC-based therapies (250). Proteomic profiling has revealed galectin-3 (Galectin-3) as a central mediator of the immunosuppressive and anti-inflammatory properties of MSCs, acting through regulation of macrophage polarization, T cell functionality, and fibrotic pathways (251–253). Future efforts are needed to delineate the dose-dependent effects and signaling networks of Galectin-3 to facilitate its targeted application in MSC therapy. Moreover, metabolomic data indicate that enhanced glycolysis and mitochondrial remodeling are tightly associated with the immunoregulatory function of MSCs. Targeting metabolic pathways may thus provide a novel strategy to modulate the immunosuppressive potential of MSCs (254). However, the clinical application of multi-omics technologies is limited by high per-test costs and the need for interdisciplinary expertise in data interpretation.
Dose optimization represents another critical challenge. Current preclinical studies demonstrate a non-linear, inverted U-shaped dose-response relationship, whereby both low and high doses result in inferior outcomes (255). The MSC-HF trial indicated that high doses (>100 × 106 cells) significantly improved cardiac function (225), whereas the POSEIDON trial found that the 20 × 106 cell group exhibited greater reduction in myocardial scar size compared to the 100 × 106 cell group (226). Such discrepancies may reflect heterogeneity in the pathological microenvironment—advanced heart failure patients often experience severe myocardial hypoxia and elevated inflammatory cytokines, which may lead to increased cell apoptosis at higher doses due to intensified competition for nutrients, whereas lower doses may survive better and exert paracrine effects in less hostile environments.
The determination of optimal treatment windows poses an additional clinical challenge. Preclinical studies have demonstrated that the therapeutic window for preventing I/R injury is narrow and requires early intervention before full activation of apoptotic pathways (255). The timing of MSC administration significantly affects therapeutic efficacy, though results remain inconsistent. In large animal models, administration 30 min post-reperfusion yielded variable outcomes—some studies reported improvements in infarct size or ejection fraction, while others found no benefit (256–258). In contrast, intravenous delivery 15 min post-reperfusion or delayed injection at 3 days to 3 months post-infarction facilitated functional recovery or remodeling (165, 259), whereas administration at 30 days post-MI may expose MSCs to detrimental microenvironments that compromise cell viability (260). Clinical studies further underscore the importance of timing; meta-analyses suggest that administration within one week of myocardial infarction, particularly 5–7 days PCI, enhances ejection fraction and myocardial perfusion. In contrast, very early administration (within 24 h) may reduce infarct size without improving overall cardiac function (261).
9 Optimization strategies for MSCs and their exosomes in heart failure therapy
The complex pathophysiology of heart failure necessitates further enhancement of the efficacy, targeting specificity, and durability of MSCs and exosome-based therapies. Recent advances in bioengineering, nanomedicine, and preclinical research have yielded multidimensional strategies to improve therapeutic outcomes. This section systematically outlines the most promising optimization approaches, including functional enhancement, innovative delivery systems, combinatorial therapeutic strategies, standardized manufacturing, and individualized treatment pathways, while discussing their translational potential based on the latest research findings.
9.1 Functional enhancement: genetic regulation and engineering modification
In recent years, genetic engineering and preconditioning strategies have achieved substantial progress in enhancing the therapeutic efficacy of MSCs and their exosomes. In the domain of genetic regulation, the application of CRISPR-Cas9 and viral vector technologies has enabled precise genetic modifications of MSCs, thereby significantly improving their reparative capacity (262–265). Genetic modifications have been shown to augment MSC efficacy in heart failure by promoting cell survival, enhancing differentiation and integration, and strengthening paracrine signaling (266). For instance, overexpression of heat shock protein 27 (HSP27) and heme oxygenase-1 (HO-1) has been reported to increase MSCs survival, reduce apoptosis, and improve left ventricular function (267, 268). Furthermore, overexpression of stromal cell-derived factor 1 (SDF-1) and its receptor CXCR4 enhances MSCs homing ability, reduces ventricular remodeling, and restores cardiac function (269, 270). Similarly, upregulation of IGF-1, HGF, and Ang-1 has been shown to promote angiogenesis, attenuate infarct size, and improve cardiac performance (271–273).
Preconditioning under hypoxic conditions (0.5%–3% O2) has also been demonstrated to activate the PI3K-Akt signaling pathway, enhance cellular survival, and upregulate key anti-apoptotic and pro-angiogenic factors including HIF-1α, VEGF, and Bcl-2, thereby reducing infarct size and improving cardiac outcomes (274). Exosome engineering represents another promising direction. For example, exosomes modified with ischemic myocardium-targeting peptides (IMTP-exosomes) have shown superior therapeutic efficacy compared to unmodified exosomes, significantly alleviating inflammation, reducing apoptosis and fibrosis, promoting angiogenesis, and enhancing cardiac function following myocardial infarction (275). Additionally, exosome microneedle patches loaded with microRNA-29b have been found to effectively prevent post-infarction cardiac fibrosis (276). Exosomes overexpressing CD47 have demonstrated improved targeting efficiency by evading immune clearance via the “don't eat me” signal (277, 278). These strategies provide powerful tools to optimize MSCs and exosome-based therapies, although further investigations are required to address challenges in clinical translation.
9.2 Innovative delivery systems: biomaterials-driven precision therapeutic strategies
Although substantial progress has been made in applying MSCs and their exosomes for cardiac repair, conventional intramyocardial injection remains hindered by limited cell viability, uneven distribution, and difficulties in maintaining therapeutic function. To overcome these limitations, researchers have developed a range of biomaterial-based innovative delivery systems that improve local retention, enable controlled release, and enhance the stability of bioactive molecules, thereby significantly boosting the therapeutic potential of MSCs-based interventions.
Hydrogel scaffolds, owing to their superior biocompatibility and biomimetic properties, have emerged as one of the most promising delivery vehicles. These scaffolds mimic the native ECM microenvironment, thereby enhancing MSCs retention and functional expression (279, 280). Specifically, injectable peptide and alginate hydrogels loaded with EVs have demonstrated improved retention at the site of myocardial injury, facilitated angiogenesis, and mitigated fibrotic progression (281). The incorporation of 3D culture technologies has further potentiated the cardioprotective effects of these systems, offering new avenues for myocardial regeneration (282, 283).
Microsphere and encapsulation technologies also offer unique advantages in delivery system design. MSCs-derived microspheres formed via self-assembly into 3D structures have been shown to enhance anti-apoptotic capacity and optimize paracrine signaling (284). Spheroids cultured on chitosan membranes have exhibited enhanced myocardial contractility in animal models, potentially mediated by angiogenesis-related microRNAs (285, 286). Semipermeable encapsulation platforms provide physical protection from immune rejection while allowing the exchange of nutrients and signaling molecules, offering an ideal foundation for integrating gene editing technologies and achieving controlled release of therapeutic factors (266, 287–289).
Recently, the development of magnetically responsive delivery systems has opened new frontiers for precision therapy. MSCs labeled with superparamagnetic iron oxide nanoparticles (SPIONs) have demonstrated significantly enhanced myocardial engraftment under magnetic guidance (290). Moreover, magnetic navigation technologies have been shown to increase targeting accuracy and therapeutic efficacy of MSCs and their exosomes in heart failure models (291, 292). Future research should further investigate the interplay between biomaterials and cellular functions, aiming to develop intelligent delivery platforms with targeted homing and dynamic responsiveness. Additionally, standardization and large-scale production of these technologies will be crucial for their clinical translation, providing more effective solutions for cardiovascular therapy. These innovative strategies represent a major step toward overcoming the limitations of traditional cell therapies and highlight the vast potential of tissue engineering in regenerative medicine.
9.3 Combined therapies: synergistic mechanisms and clinical translation
In recent years, combination strategies involving MSCs and their exosomes have achieved significant advances in the treatment of heart failure, primarily by leveraging synergistic mechanisms to amplify therapeutic efficacy. Among these, the co-administration of cells and pharmacological agents has emerged as a promising approach. Drug preconditioning with agents such as trimetazidine, melatonin, and statins has been shown to activate the Akt signaling pathway, reduce apoptosis, promote angiogenesis, and modulate immune responses—thereby significantly enhancing MSCs survival and tissue repair capacity (293–297). Currently, a clinical trial is underway investigating the combined application of atorvastatin and autologous BM-MSCs in patients undergoing PCI, aiming to improve cell survival through pharmacological dose optimization (298).
The therapeutic synergy of combination approaches is highly dependent on temporal coordination and vector design. As discussed in the previous section, combining MSCs with biomaterials (e.g., hydrogels or cell sheet technologies) enhances cell retention and facilitates myocardial repair. Notably, in a rat model of MI, sequential delivery of MSCs and their derived exosomes has demonstrated superior therapeutic effects (299). In this study, exosomes were administered 30 min post-MI to target the acute inflammatory phase, followed by MSC transplantation on day 3 to support regenerative processes (299). This precisely timed protocol significantly reduced infarct size and improved cardiac contractility, highlighting the potential advantages of temporally coordinated cell-exosome therapy.
More complex combination strategies involve co-transplantation of MSCs with CPCs. The CONCERT-HF trial has demonstrated that while CPCs primarily reduce clinical events and MSCs improve quality of life, their combined use yields additive benefits by targeting complementary aspects of cardiac repair (227). Additionally, the co-administration of MSCs with regulatory T cells (Tregs) has been shown to enhance MSC survival, proliferation, and pro-angiogenic potential, offering a novel avenue for cell-based combinatorial therapies (300).
Despite promising preclinical results, the clinical translation of combination therapies remains challenged by the need for optimized therapeutic regimens, thorough safety evaluations, and standardized manufacturing protocols. Future efforts should focus on developing intelligent delivery systems and conducting multicenter clinical trials to facilitate the adoption of combination strategies in clinical practice.
10 Conclusion and future prospects
Despite significant advancements in HF treatment, the five-year mortality rate for HF remains as high as 50%, making it a major global health concern. The pursuit of more effective therapeutic strategies for HF has long been the goal of many medical professionals. The discovery of MSCs and their exosome derivatives in the field of cardiac regenerative medicine has attracted widespread attention. Current evidence suggests that the use of MSCs and their exosomes can improve the ejection fraction in HF patients, with many preclinical trials showing promising results.
Although an increasing number of clinical trials have demonstrated the significant efficacy of MSCs and their exosomes in treating HF, and they hold considerable promise for clinical applications, several challenges remain. With longer follow-up periods, it is unclear whether the benefits of stem cell therapy will persist. Furthermore, the standardization of stem cell preparation, delivery methods, and dosing regimens has yet to be established. Additionally, most current studies have small sample sizes, limited evaluation methods for cardiac function, and follow-up periods of less than two years.
Therefore, future research should involve larger patient cohorts, longer follow-up periods, and more comprehensive evaluation methods. The promotion of personalized treatment should also be applied to MSCs transplantation, tailoring the choice of delivery methods, dosing, and follow-up strategies according to individual patient differences to achieve the best therapeutic outcomes. This represents another promising direction for future research on stem cell transplantation in the treatment of HF.
Author contributions
CC: Conceptualization, Writing – original draft. WZ: Methodology, Writing – original draft. HZ: Writing – original draft. WZ: Writing – original draft. YW: Visualization, Writing – original draft. BS: Supervision, Writing – review & editing.
Funding
The author(s) declare that no financial support was received for the research and/or publication of this article.
Conflict of interest
The authors declare that the research was conducted in the absence of any commercial or financial relationships that could be construed as a potential conflict of interest.
Generative AI statement
The author(s) declare that no Generative AI was used in the creation of this manuscript.
Publisher's note
All claims expressed in this article are solely those of the authors and do not necessarily represent those of their affiliated organizations, or those of the publisher, the editors and the reviewers. Any product that may be evaluated in this article, or claim that may be made by its manufacturer, is not guaranteed or endorsed by the publisher.
References
1. McDonagh TA, Metra M, Adamo M, Gardner RS, Baumbach A, Böhm M, et al. 2023 Focused update of the 2021 ESC guidelines for the diagnosis and treatment of acute and chronic heart failure. Eur Heart J. (2023) 44:3627–39. doi: 10.1093/eurheartj/ehad195
2. Metra M, Teerlink JR. Heart failure. Lancet. (2017) 390:1981–95. doi: 10.1016/S0140-6736(17)31071-1
3. Tanai E, Frantz S. Pathophysiology of heart failure. Compr Physiol. (2016) 6:187–214. doi: 10.1002/j.2040-4603.2016.tb00669.x
4. Špinar J, Špinarová L, Vítovec J. Pathophysiology, causes and epidemiology of chronic heart failure. Vnitr Lek. (2018) 64:834–8. doi: 10.36290/vnl.2018.114
5. Schrier RW, Abraham WT. Hormones and hemodynamics in heart failure. N Engl J Med. (1999) 341:577–85. doi: 10.1056/NEJM199908193410806
6. Dzau VJ, Colucci WS, Hollenberg NK, Williams GH. Relation of the renin-angiotensin-aldosterone system to clinical state in congestive heart failure. Circulation. (1981) 63:645–51. doi: 10.1161/01.CIR.63.3.645
7. Packer M, McMurray JJV. Importance of endogenous compensatory vasoactive peptides in broadening the effects of inhibitors of the renin-angiotensin system for the treatment of heart failure. Lancet. (2017) 389:1831–40. doi: 10.1016/S0140-6736(16)30969-2
8. Pugliese NR, Masi S, Taddei S. The renin-angiotensin-aldosterone system: a crossroad from arterial hypertension to heart failure. Heart Fail Rev. (2020) 25:31–42. doi: 10.1007/s10741-019-09855-5
9. Lytvyn Y, Burns KD, Testani JM, Lytvyn A, Ambinathan JPN, Osuntokun O, et al. Renal hemodynamics and renin-angiotensin-aldosterone system profiles in patients with heart failure. J Card Fail. (2022) 28:385–93. doi: 10.1016/j.cardfail.2021.08.015
10. Savarese G, Becher PM, Lund LH, Seferovic P, Rosano GMC, Coats AJS. Global burden of heart failure: a comprehensive and updated review of epidemiology. Cardiovasc Res. (2023) 118:3272–87. doi: 10.1093/cvr/cvac013
11. Bauersachs J. Heart failure drug treatment: the fantastic four. Eur Heart J. (2021) 42:681–3. doi: 10.1093/eurheartj/ehaa1012
12. Tamargo J, Caballero R, Delpón E. New drugs in preclinical and early stage clinical development in the treatment of heart failure. Expert Opin Investig Drugs. (2019) 28:51–71. doi: 10.1080/13543784.2019.1551357
13. Orso F, Herbst A, Pratesi A, Fattirolli F, Ungar A, Marchionni N, et al. New drugs for heart failure: what is the evidence in older patients? J Card Fail. (2022) 28:316–29. doi: 10.1016/j.cardfail.2021.07.011
14. Sapp JL, Sivakumaran S, Redpath CJ, Khan H, Parkash R, Exner DV, et al. Long-term outcomes of resynchronization-defibrillation for heart failure. N Engl J Med. (2024) 390:212–20. doi: 10.1056/NEJMoa2304542
15. Yafasova A, Doi SN, Thune JJ, Nielsen JC, Haarbo J, Bruun NE, et al. Effect of implantable cardioverter-defibrillators in nonischemic heart failure according to background medical therapy: extended follow-up of the DANISH trial. J Card Fail. (2024) 30(11):1411–20. doi: 10.1016/j.cardfail.2024.04.017
16. Bounader K, Flécher E. End-stage heart failure: the future of heart transplant and artificial heart. Presse Med. (2024) 53:104191. doi: 10.1016/j.lpm.2023.104191
17. Fuchs M, Schibilsky D, Zeh W, Berchtold-Herz M, Beyersdorf F, Siepe M. Does the heart transplant have a future? Eur J Cardiothorac Surg. (2019) 55:i38–48. doi: 10.1093/ejcts/ezz107
18. Shah KS, Kittleson MM, Kobashigawa JA. Updates on heart transplantation. Curr Heart Fail Rep. (2019) 16:150–6. doi: 10.1007/s11897-019-00432-3
19. Tang X, Li PH, Chen HZ. Cardiomyocyte senescence and cellular communications within myocardial microenvironments. Front Endocrinol (Lausanne). (2020) 11:280. doi: 10.3389/fendo.2020.00280
20. Dridi H, Kushnir A, Zalk R, Yuan Q, Melville Z, Marks AR. Intracellular calcium leak in heart failure and atrial fibrillation: a unifying mechanism and therapeutic target. Nat Rev Cardiol. (2020) 17:732–47. doi: 10.1038/s41569-020-0394-8
21. Yang C, Weiss AS, Tarakanova A. Changes in elastin structure and extensibility induced by hypercalcemia and hyperglycemia. Acta Biomater. (2023) 163:131–45. doi: 10.1016/j.actbio.2022.03.041
22. Valentim MA, Brahmbhatt AN, Tupling AR. Skeletal and cardiac muscle calcium transport regulation in health and disease. Biosci Rep. (2022) 42:BSR20211997. doi: 10.1042/BSR20211997
23. Primessnig U, Bracic T, Levijoki J, Otsomaa L, Pollesello P, Falcke M, et al. Long-term effects of Na(+)/Ca(2+) exchanger inhibition with ORM-11035 improves cardiac function and remodelling without lowering blood pressure in a model of heart failure with preserved ejection fraction. Eur J Heart Fail. (2019) 21:1543–52. doi: 10.1002/ejhf.1619
24. Ren L, Gopireddy RR, Perkins G, Zhang H, Timofeyev V, Lyu Y, et al. Disruption of mitochondria-sarcoplasmic reticulum microdomain connectomics contributes to sinus node dysfunction in heart failure. Proc Natl Acad Sci U S A. (2022) 119:e2206708119. doi: 10.1073/pnas.2206708119
25. Mancini A, Vergani E, Bruno C, Olivieri G, Di Segni C, Silvestrini A, et al. Oxidative stress as a possible mechanism underlying multi-hormonal deficiency in chronic heart failure. Eur Rev Med Pharmacol Sci. (2018) 22:3936–61. doi: 10.26355/eurrev_201806_15279
26. Wu C, Zhang Z, Zhang W, Liu X. Mitochondrial dysfunction and mitochondrial therapies in heart failure. Pharmacol Res. (2022) 175:106038. doi: 10.1016/j.phrs.2021.106038
27. Dey S, DeMazumder D, Sidor A, Foster DB, O’Rourke B. Mitochondrial ROS drive sudden cardiac death and chronic proteome remodeling in heart failure. Circ Res. (2018) 123:356–71. doi: 10.1161/CIRCRESAHA.118.312708
28. Cadenas S. ROS and redox signaling in myocardial ischemia-reperfusion injury and cardioprotection. Free Radic Biol Med. (2018) 117:76–89. doi: 10.1016/j.freeradbiomed.2018.01.024
29. Weissman D, Maack C. Redox signaling in heart failure and therapeutic implications. Free Radic Biol Med. (2021) 171:345–64. doi: 10.1016/j.freeradbiomed.2021.05.013
30. Bugger H, Pfeil K. Mitochondrial ROS in myocardial ischemia reperfusion and remodeling. Biochim Biophys Acta Mol Basis Dis. (2020) 1866:165768. doi: 10.1016/j.bbadis.2020.165768
31. Hulsurkar M, Quick AP, Wehrens XH. STAT3: a link between CaMKII-βIV-spectrin and maladaptive remodeling? J Clin Invest. (2018) 128:5219–21. doi: 10.1172/JCI124778
32. Kurdi M, Zgheib C, Booz GW. Recent developments on the crosstalk between STAT3 and inflammation in heart function and disease. Front Immunol. (2018) 9:3029. doi: 10.3389/fimmu.2018.03029
33. Kleinbongard P. Perspective: mitochondrial STAT3 in cardioprotection. Basic Res Cardiol. (2023) 118:32. doi: 10.1007/s00395-023-01003-3
34. Halade GV, Lee DH. Inflammation and resolution signaling in cardiac repair and heart failure. EBioMed. (2022) 79:103992. doi: 10.1016/j.ebiom.2022.103992
35. Hanna A, Frangogiannis NG. Inflammatory cytokines and chemokines as therapeutic targets in heart failure. Cardiovasc Drugs Ther. (2020) 34:849–63. doi: 10.1007/s10557-020-07071-0
36. Markousis-Mavrogenis G, Tromp J, Ouwerkerk W, Devalaraja M, Anker SD, Cleland JG, et al. The clinical significance of interleukin-6 in heart failure: results from the BIOSTAT-CHF study. Eur J Heart Fail. (2019) 21:965–73. doi: 10.1002/ejhf.1482
37. Frangogiannis NG. TGF-β as a therapeutic target in the infarcted and failing heart: cellular mechanisms, challenges, and opportunities. Expert Opin Ther Targets. (2024) 28:45–56. doi: 10.1080/14728222.2024.2316735
38. Bassiouni W, Valencia R, Mahmud Z, Seubert JM, Schulz R. Matrix metalloproteinase-2 proteolyzes mitofusin-2 and impairs mitochondrial function during myocardial ischemia-reperfusion injury. Basic Res Cardiol. (2023) 118:29. doi: 10.1007/s00395-023-00999-y
39. Lee HS, Kim WJ. The role of matrix metalloproteinase in inflammation with a focus on infectious diseases. Int J Mol Sci. (2022) 23:10546. doi: 10.3390/ijms231810546
40. Frangogiannis NG. Cardiac fibrosis: cell biological mechanisms, molecular pathways and therapeutic opportunities. Mol Aspects Med. (2019) 65:70–99. doi: 10.1016/j.mam.2018.07.001
41. Zhang H, Dhalla NS. The role of pro-inflammatory cytokines in the pathogenesis of cardiovascular disease. Int J Mol Sci. (2024) 25:1082. doi: 10.3390/ijms25021082
42. Carrillo-Salinas FJ, Ngwenyama N, Anastasiou M, Kaur K, Alcaide P. Heart inflammation: immune cell roles and roads to the heart. Am J Pathol. (2019) 189:1482–94. doi: 10.1016/j.ajpath.2019.04.009
43. Smolgovsky S, Theall B, Wagner N, Alcaide P. Fibroblasts and immune cells: at the crossroad of organ inflammation and fibrosis. Am J Physiol Heart Circ Physiol. (2024) 326:H303–16. doi: 10.1152/ajpheart.00545.2023
44. Mullens W, Damman K, Dhont S, Banerjee D, Bayes-Genis A, Cannata A, et al. Dietary sodium and fluid intake in heart failure. A clinical consensus statement of the heart failure association of the ESC. Eur J Heart Fail. (2024) 26:730–41. doi: 10.1002/ejhf.3244
45. Mullens W, Damman K, Harjola VP, Mebazaa A, Brunner-La Rocca HP, Martens P, et al. The use of diuretics in heart failure with congestion—a position statement from the heart failure association of the European Society of Cardiology. Eur J Heart Fail. (2019) 21:137–55. doi: 10.1002/ejhf.1369
46. Felker GM, Ellison DH, Mullens W, Cox ZL, Testani JM. Diuretic therapy for patients with heart failure: JACC state-of-the-art review. J Am Coll Cardiol. (2020) 75:1178–95. doi: 10.1016/j.jacc.2019.12.059
47. Fröhlich H, Henning F, Täger T, Schellberg D, Grundtvig M, Goode K, et al. Comparative effectiveness of enalapril, lisinopril, and ramipril in the treatment of patients with chronic heart failure: a propensity score-matched cohort study. Eur Heart J Cardiovasc Pharmacother. (2018) 4:82–92. doi: 10.1093/ehjcvp/pvx013
48. Foody JM, Farrell MH, Krumholz HM. beta-Blocker therapy in heart failure: scientific review. Jama. (2002) 287:883–9. doi: 10.1001/jama.287.7.883
49. Pitt B, Zannad F, Remme WJ, Cody R, Castaigne A, Perez A, et al. The effect of spironolactone on morbidity and mortality in patients with severe heart failure. Randomized aldactone evaluation study investigators. N Engl J Med. (1999) 341:709–17. doi: 10.1056/NEJM199909023411001
50. McMurray JJ, Ostergren J, Swedberg K, Granger CB, Held P, Michelson EL, et al. Effects of candesartan in patients with chronic heart failure and reduced left-ventricular systolic function taking angiotensin-converting-enzyme inhibitors: the CHARM-added trial. Lancet. (2003) 362:767–71. doi: 10.1016/S0140-6736(03)14283-3
51. McMurray JJ, Packer M, Desai AS, Gong J, Lefkowitz MP, Rizkala AR, et al. Angiotensin-neprilysin inhibition versus enalapril in heart failure. N Engl J Med. (2014) 371:993–1004. doi: 10.1056/NEJMoa1409077
52. Wachter R, Senni M, Belohlavek J, Straburzynska-Migaj E, Witte KK, Kobalava Z, et al. Initiation of sacubitril/valsartan in haemodynamically stabilised heart failure patients in hospital or early after discharge: primary results of the randomised TRANSITION study. Eur J Heart Fail. (2019) 21:998–1007. doi: 10.1002/ejhf.1498
53. Velazquez EJ, Morrow DA, DeVore AD, Duffy CI, Ambrosy AP, McCague K, et al. Angiotensin-neprilysin inhibition in acute decompensated heart failure. N Engl J Med. (2019) 380:539–48. doi: 10.1056/NEJMoa1812851
54. Zelniker TA, Wiviott SD, Raz I, Im K, Goodrich EL, Bonaca MP, et al. SGLT2 inhibitors for primary and secondary prevention of cardiovascular and renal outcomes in type 2 diabetes: a systematic review and meta-analysis of cardiovascular outcome trials. Lancet. (2019) 393:31–9. doi: 10.1016/S0140-6736(18)32590-X
55. McMurray JJV, Solomon SD, Inzucchi SE, Køber L, Kosiborod MN, Martinez FA, et al. Dapagliflozin in patients with heart failure and reduced ejection fraction. N Engl J Med. (2019) 381:1995–2008. doi: 10.1056/NEJMoa1911303
56. Markham A, Duggan S. Vericiguat: first approval. Drugs. (2021) 81:721–6. doi: 10.1007/s40265-021-01496-z
57. Armstrong PW, Pieske B, Anstrom KJ, Ezekowitz J, Hernandez AF, Butler J, et al. Vericiguat in patients with heart failure and reduced ejection fraction. N Engl J Med. (2020) 382:1883–93. doi: 10.1056/NEJMoa1915928
58. Armstrong PW, Roessig L, Patel MJ, Anstrom KJ, Butler J, Voors AA, et al. Placebo-controlled trial of the efficacy and safety of the oral soluble guanylate cyclase stimulator: the VICTORIA trial. JACC Heart Fail. (2018) 6:96–104. doi: 10.1016/j.jchf.2017.08.013
59. Swedberg K, Komajda M, Böhm M, Borer JS, Ford I, Dubost-Brama A, et al. Ivabradine and outcomes in chronic heart failure (SHIFT): a randomised placebo-controlled study. Lancet. (2010) 376:875–85. doi: 10.1016/S0140-6736(10)61198-1
60. Böhm M, Borer J, Ford I, Gonzalez-Juanatey JR, Komajda M, Lopez-Sendon J, et al. Heart rate at baseline influences the effect of ivabradine on cardiovascular outcomes in chronic heart failure: analysis from the SHIFT study. Clin Res Cardiol. (2013) 102:11–22. doi: 10.1007/s00392-012-0467-8
61. Thorup L, Simonsen U, Grimm D, Hedegaard ER. Ivabradine: current and future treatment of heart failure. Basic Clin Pharmacol Toxicol. (2017) 121:89–97. doi: 10.1111/bcpt.12784
62. G. Digitalis Investigation. The effect of digoxin on mortality and morbidity in patients with heart failure. N Engl J Med. (1997) 336:525–33. doi: 10.1056/NEJM199702203360801
63. Ahmed A, Rich MW, Fleg JL, Zile MR, Young JB, Kitzman DW, et al. Effects of digoxin on morbidity and mortality in diastolic heart failure: the ancillary digitalis investigation group trial. Circulation. (2006) 114:397–403. doi: 10.1161/CIRCULATIONAHA.106.628347
64. Shu H, Hang W, Peng Y, Nie J, Wu L, Zhang W, et al. Trimetazidine attenuates heart failure by improving myocardial metabolism via AMPK. Front Pharmacol. (2021) 12:707399. doi: 10.3389/fphar.2021.707399
65. Nassiri S, Van de Bovenkamp AA, Remmelzwaal S, Sorea O, de Man F, Handoko ML. Effects of trimetazidine on heart failure with reduced ejection fraction and associated clinical outcomes: a systematic review and meta-analysis. Open Heart. (2024) 11:e002579. doi: 10.1136/openhrt-2023-002579
66. Ghoneem A, Bhatti AW, Khadke S, Mitchell J, Liu J, Zhang K, et al. Real-world efficacy of tafamidis in patients with transthyretin amyloidosis and heart failure. Curr Probl Cardiol. (2023) 48:101667. doi: 10.1016/j.cpcardiol.2023.101667
67. Patel PH, Nguyen M, Rodriguez R, Surani S, Udeani G. Omecamtiv mecarbil: a novel mechanistic and therapeutic approach to chronic heart failure management. Cureus. (2021) 13:e12419. doi: 10.7759/cureus.12419
68. Everett BM, Cornel JH, Lainscak M, Anker SD, Abbate A, Thuren T, et al. Anti-inflammatory therapy with canakinumab for the prevention of hospitalization for heart failure. Circulation. (2019) 139:1289–99. doi: 10.1161/CIRCULATIONAHA.118.038010
69. Velazquez EJ, Lee KL, Jones RH, Al-Khalidi HR, Hill JA, Panza JA, et al. Coronary-artery bypass surgery in patients with ischemic cardiomyopathy. N Engl J Med. (2016) 374:1511–20. doi: 10.1056/NEJMoa1602001
70. Perera D, Clayton T, O’Kane PD, Greenwood JP, Weerackody R, Ryan M, et al. Percutaneous revascularization for ischemic left ventricular dysfunction. N Engl J Med. (2022) 387:1351–60. doi: 10.1056/NEJMoa2206606
71. Abdelrahman M, Subzposh FA, Beer D, Durr B, Naperkowski A, Sun H, et al. Clinical outcomes of his bundle pacing compared to right ventricular pacing. J Am Coll Cardiol. (2018) 71:2319–30. doi: 10.1016/j.jacc.2018.02.048
72. Upadhyay GA, Vijayaraman P, Nayak HM, Verma N, Dandamudi G, Sharma PS, et al. His corrective pacing or biventricular pacing for cardiac resynchronization in heart failure. J Am Coll Cardiol. (2019) 74:157–9. doi: 10.1016/j.jacc.2019.04.026
73. Huang W, Wu S, Vijayaraman P, Su L, Chen X, Cai B, et al. Cardiac resynchronization therapy in patients with nonischemic cardiomyopathy using left bundle branch pacing. JACC Clin Electrophysiol. (2020) 6:849–58. doi: 10.1016/j.jacep.2020.04.011
74. Zhang W, Huang J, Qi Y, Wang F, Guo L, Shi X, et al. Cardiac resynchronization therapy by left bundle branch area pacing in patients with heart failure and left bundle branch block. Heart Rhythm. (2019) 16:1783–90. doi: 10.1016/j.hrthm.2019.09.006
75. Chung MK, Patton KK, Lau CP, Dal Forno ARJ, Al-Khatib SM, Arora V, et al. 2023 HRS/APHRS/LAHRS guideline on cardiac physiologic pacing for the avoidance and mitigation of heart failure. J Arrhythm. (2023) 39:681–756. doi: 10.1002/joa3.12872
76. Kuschyk J, Rudic B, Borggrefe M, Akin I. Cardiac contractility modulation. Internist (Berl). (2018) 59:1021–7. doi: 10.1007/s00108-018-0493-3
77. Kuschyk J, Rudic B, Liebe V, Tülümen E, Borggrefe M, Akin I. Cardiac contractility modulation for treatment of chronic heart failure. Herzschrittmacherther Elektrophysiol. (2018) 29:369–76. doi: 10.1007/s00399-018-0600-0
78. Bazoukis G, Saplaouras A, Efthymiou P, Yiannikourides A, Liu T, Letsas KP, et al. Cardiac contractility modulation in patients with heart failure—a review of the literature. Heart Fail Rev. (2024) 29:689–705. doi: 10.1007/s10741-024-10390-1
79. Anker SD, Borggrefe M, Neuser H, Ohlow MA, Röger S, Goette A, et al. Cardiac contractility modulation improves long-term survival and hospitalizations in heart failure with reduced ejection fraction. Eur J Heart Fail. (2019) 21:1103–13. doi: 10.1002/ejhf.1374
80. Kloppe A, Mijic D, Schiedat F, Bogossian H, Mügge A, Rousso B, et al. A randomized comparison of 5 versus 12 h per day of cardiac contractility modulation treatment for heart failure patients: a preliminary report. Cardiol J. (2016) 23:114–9. doi: 10.5603/CJ.a2015.0073
81. Kuschyk J, Roeger S, Schneider R, Streitner F, Stach K, Rudic B, et al. Efficacy and survival in patients with cardiac contractility modulation: long-term single center experience in 81 patients. Int J Cardiol. (2015) 183:76–81. doi: 10.1016/j.ijcard.2014.12.178
82. Abraham WT, Kuck KH, Goldsmith RL, Lindenfeld J, Reddy VY, Carson PE, et al. A randomized controlled trial to evaluate the safety and efficacy of cardiac contractility modulation. JACC Heart Fail. (2018) 6:874–83. doi: 10.1016/j.jchf.2018.04.010
83. Theuns DA, Smith T, Hunink MG, Bardy GH, Jordaens L. Effectiveness of prophylactic implantation of cardioverter-defibrillators without cardiac resynchronization therapy in patients with ischaemic or non-ischaemic heart disease: a systematic review and meta-analysis. Europace. (2010) 12:1564–70. doi: 10.1093/europace/euq329
84. Hussein AA, Wilkoff BL. Cardiac implantable electronic device therapy in heart failure. Circ Res. (2019) 124:1584–97. doi: 10.1161/CIRCRESAHA.118.313571
85. Ezzat VA, Lee V, Ahsan S, Chow AW, Segal O, Rowland E, et al. A systematic review of ICD complications in randomised controlled trials versus registries: is our ‘real-world’ data an underestimation? Open Heart. (2015) 2:e000198. doi: 10.1136/openhrt-2014-000198
86. Nso N, Nassar M, Lakhdar S, Enoru S, Guzman L, Rizzo V, et al. Comparative assessment of transvenous versus subcutaneous implantable cardioverter-defibrillator therapy outcomes: an updated systematic review and meta-analysis. Int J Cardiol. (2022) 349:62–78. doi: 10.1016/j.ijcard.2021.11.029
87. Weaver FA, Abraham WT, Little WC, Butter C, Ducharme A, Halbach M, et al. Surgical experience and long-term results of baroreflex activation therapy for heart failure with reduced ejection fraction. Semin Thorac Cardiovasc Surg. (2016) 28:320–8. doi: 10.1053/j.semtcvs.2016.04.017
88. Abraham WT, Zile MR, Weaver FA, Butter C, Ducharme A, Halbach M, et al. Baroreflex activation therapy for the treatment of heart failure with a reduced ejection fraction. JACC Heart Fail. (2015) 3:487–96. doi: 10.1016/j.jchf.2015.02.006
89. Zile MR, Lindenfeld J, Weaver FA, Zannad F, Galle E, Rogers T, et al. Baroreflex activation therapy in patients with heart failure with reduced ejection fraction. J Am Coll Cardiol. (2020) 76:1–13. doi: 10.1016/j.jacc.2020.05.015
90. Piccirillo F, Crispino SP, Buzzelli L, Segreti A, Incalzi RA, Grigioni F. A state-of-the-art review on sleep apnea syndrome and heart failure. Am J Cardiol. (2023) 195:57–69. doi: 10.1016/j.amjcard.2023.02.020
91. Zeitler EP, Abraham WT. Novel devices in heart failure: BAT, atrial shunts, and phrenic nerve stimulation. JACC Heart Fail. (2020) 8:251–64. doi: 10.1016/j.jchf.2019.11.006
92. Oldenburg O, Lamp B, Faber L, Teschler H, Horstkotte D, Töpfer V. Sleep-disordered breathing in patients with symptomatic heart failure: a contemporary study of prevalence in and characteristics of 700 patients. Eur J Heart Fail. (2007) 9:251–7. doi: 10.1016/j.ejheart.2006.08.003
93. Costanzo MR, Ponikowski P, Coats A, Javaheri S, Augostini R, Goldberg LR, et al. Phrenic nerve stimulation to treat patients with central sleep apnoea and heart failure. Eur J Heart Fail. (2018) 20:1746–54. doi: 10.1002/ejhf.1312
94. Wang Y, Schoebel J, Han J, Kraemer JF, Toncar T, Siegert J, et al. Phrenic nerve stimulation for the treatment of central sleep apnea in patients with heart failure. Sleep Breath. (2023) 27:1027–32. doi: 10.1007/s11325-022-02699-8
95. Stewart GC, Givertz MM. Mechanical circulatory support for advanced heart failure: patients and technology in evolution. Circulation. (2012) 125:1304–15. doi: 10.1161/CIRCULATIONAHA.111.060830
96. Khalif A, Kanwar MK. ECMO as a bridge to advanced heart failure therapies: moving beyond ‘crash and burn’. J Heart Lung Transplant. (2023) 42:1072–3. doi: 10.1016/j.healun.2023.04.003
97. Chakaramakkil MJ, Sivathasan C. ECMO and short-term support for cardiogenic shock in heart failure. Curr Cardiol Rep. (2018) 20:87. doi: 10.1007/s11886-018-1041-4
98. Chun KH, Kang SM. Advanced heart failure: a contemporary approach. Korean J Intern Med. (2023) 38:471–83. doi: 10.3904/kjim.2023.159
99. Crespo-Leiro MG, Anker SD, Maggioni AP, Coats AJ, Filippatos G, Ruschitzka F, et al. European Society of Cardiology Heart Failure Long-Term Registry (ESC-HF-LT): 1-year follow-up outcomes and differences across regions. Eur J Heart Fail. (2016) 18:613–25. doi: 10.1002/ejhf.566
100. Bottle A, Newson R, Faitna P, Hayhoe B, Cowie MR. Changes in heart failure management and long-term mortality over 10 years: observational study. Open Heart. (2022) 9:e001888. doi: 10.1136/openhrt-2021-001888
101. Bozkurt B. Concerning trends of rising heart failure mortality rates. JACC Heart Fail. (2024) 12:970–2. doi: 10.1016/j.jchf.2024.04.001
102. Friedenstein AJ, Chailakhjan RK, Lalykina KS. The development of fibroblast colonies in monolayer cultures of Guinea-pig bone marrow and spleen cells. Cell Tissue Kinet. (1970) 3:393–403. doi: 10.1111/j.1365-2184.1970.tb00347.x
104. Wakitani S, Mera H, Nakamura N, Gobbi A. Review of caplan (1991) on cell-based therapeutic technology using mesenchymal stem cells. J Isakos. (2024) 9:426–30. doi: 10.1016/j.jisako.2023.08.010
105. Zuk PA, Zhu M, Ashjian P, De Ugarte DA, Huang JI, Mizuno H, et al. Human adipose tissue is a source of multipotent stem cells. Mol Biol Cell. (2002) 13:4279–95. doi: 10.1091/mbc.e02-02-0105
106. da Silva Meirelles L, Chagastelles PC, Nardi NB. Mesenchymal stem cells reside in virtually all post-natal organs and tissues. J Cell Sci. (2006) 119:2204–13. doi: 10.1242/jcs.02932
107. Qiao C, Xu W, Zhu W, Hu J, Qian H, Yin Q, et al. Human mesenchymal stem cells isolated from the umbilical cord. Cell Biol Int. (2008) 32:8–15. doi: 10.1016/j.cellbi.2007.08.002
108. Gupta S, Sharma A, Archana S, Verma RS. Mesenchymal stem cells for cardiac regeneration: from differentiation to cell delivery. Stem Cell Rev Rep. (2021) 17:1666–94. doi: 10.1007/s12015-021-10168-0
109. Hu L, Yin C, Zhao F, Ali A, Ma J, Qian A. Mesenchymal stem cells: cell fate decision to osteoblast or adipocyte and application in osteoporosis treatment. Int J Mol Sci. (2018) 19:360. doi: 10.3390/ijms19020360
110. Schaefer B, Beier JP, Ruhl T. Mesenchymal stem cells and the generation of neomuscle tissue. Surg Technol Int. (2020) 36:41–7. Available at: https://www.ncbi.nlm.nih.gov/pubmed/3224356532243565
111. Rahimi Darehbagh R, Seyedoshohadaei SA, Ramezani R, Rezaei N. Stem cell therapies for neurological disorders: current progress, challenges, and future perspectives. Eur J Med Res. (2024) 29:386. doi: 10.1186/s40001-024-01987-1
112. Crisan M. Transition of mesenchymal stem/stromal cells to endothelial cells. Stem Cell Res Ther. (2013) 4:95. doi: 10.1186/scrt306
113. Dominici M, Le Blanc K, Mueller I, Slaper-Cortenbach I, Marini F, Krause D, et al. Minimal criteria for defining multipotent mesenchymal stromal cells. The international society for cellular therapy position statement. Cytotherapy. (2006) 8:315–7. doi: 10.1080/14653240600855905
114. Mahla RS. Stem cells applications in regenerative medicine and disease therapeutics. Int J Cell Biol. (2016) 2016:6940283. doi: 10.1155/2016/6940283
115. Ryan JM, Barry FP, Murphy JM, Mahon BP. Mesenchymal stem cells avoid allogeneic rejection. J Inflamm (Lond). (2005) 2:8. doi: 10.1186/1476-9255-2-8
116. Halm D, Leibig N, Martens J, Stark GB, Groß T, Zimmermann S, et al. Direct comparison of the immunogenicity of major histocompatibility complex-I and -II deficient mesenchymal stem cells in vivo. Biol Chem. (2021) 402:693–702. doi: 10.1515/hsz-2020-0306
117. De Miguel P, Fuentes-Julián S, Blázquez-Martínez A, Pascual CY, Aller MA, Arias J, et al. Immunosuppressive properties of mesenchymal stem cells: advances and applications. Curr Mol Med. (2012) 12:574–91. doi: 10.2174/156652412800619950
118. Prockop DJ, Oh JY. Mesenchymal stem/stromal cells (MSCs): role as guardians of inflammation. Mol Ther. (2012) 20:14–20. doi: 10.1038/mt.2011.211
119. Ortiz LA, Dutreil M, Fattman C, Pandey AC, Torres G, Go K, et al. Interleukin 1 receptor antagonist mediates the antiinflammatory and antifibrotic effect of mesenchymal stem cells during lung injury. Proc Natl Acad Sci U S A. (2007) 104:11002–7. doi: 10.1073/pnas.0704421104
120. Cai Q, Yin F, Hao L, Jiang W. Research progress of mesenchymal stem cell therapy for severe COVID-19. Stem Cells Dev. (2021) 30:459–72. doi: 10.1089/scd.2020.0198
121. Liu Y, Zhang R, Yan K, Chen F, Huang W, Lv B, et al. Mesenchymal stem cells inhibit lipopolysaccharide-induced inflammatory responses of BV2 microglial cells through TSG-6. J Neuroinflamm. (2014) 11:135. doi: 10.1186/1742-2094-11-135
122. Guo Z, Zhou X, Li J, Meng Q, Cao H, Kang L, et al. Mesenchymal stem cells reprogram host macrophages to attenuate obliterative bronchiolitis in murine orthotopic tracheal transplantation. Int Immunopharmacol. (2013) 15:726–34. doi: 10.1016/j.intimp.2013.03.002
123. Dias LD, Casali KR, Ghem C, da Silva MK, Sausen G, Palma PB, et al. Mesenchymal stem cells from sternum: the type of heart disease, ischemic or valvular, does not influence the cell culture establishment and growth kinetics. J Transl Med. (2017) 15:161. doi: 10.1186/s12967-017-1262-0
124. Drela K, Stanaszek L, Snioch K, Kuczynska Z, Wrobel M, Sarzynska S, et al. Bone marrow-derived from the human femoral shaft as a new source of mesenchymal stem/stromal cells: an alternative cell material for banking and clinical transplantation. Stem Cell Res Ther. (2020) 11:262. doi: 10.1186/s13287-020-01697-5
125. Fragkakis EM, El-Jawhari JJ, Dunsmuir RA, Millner PA, Rao AS, Henshaw KT, et al. Vertebral body versus iliac crest bone marrow as a source of multipotential stromal cells: comparison of processing techniques, tri-lineage differentiation and application on a scaffold for spine fusion. PLoS One. (2018) 13:e0197969. doi: 10.1371/journal.pone.0197969
126. Jia Z, Liang Y, Xu X, Li X, Liu Q, Ou Y, et al. Isolation and characterization of human mesenchymal stem cells derived from synovial fluid by magnetic-activated cell sorting (MACS). Cell Biol Int. (2018) 42:262–71. doi: 10.1002/cbin.10903
127. Nery AA, Nascimento IC, Glaser T, Bassaneze V, Krieger JE, Ulrich H. Human mesenchymal stem cells: from immunophenotyping by flow cytometry to clinical applications. Cytometry A. (2013) 83:48–61. doi: 10.1002/cyto.a.22205
128. Purwaningrum M, Jamilah NS, Purbantoro SD, Sawangmake C, Nantavisai S. Comparative characteristic study from bone marrow-derived mesenchymal stem cells. J Vet Sci. (2021) 22:e74. doi: 10.4142/jvs.2021.22.e74
129. Samsonraj RM, Raghunath M, Nurcombe V, Hui JH, van Wijnen AJ, Cool SM. Concise review: multifaceted characterization of human mesenchymal stem cells for use in regenerative medicine. Stem Cells Transl Med. (2017) 6:2173–85. doi: 10.1002/sctm.17-0129
130. Nasef A, Fouillard L, El-Taguri A, Lopez M. Human bone marrow-derived mesenchymal stem cells. Libyan J Med. (2007) 2:190–201. doi: 10.3402/ljm.v2i4.4729
131. Van RL, Bayliss CE, Roncari DA. Cytological and enzymological characterization of adult human adipocyte precursors in culture. J Clin Invest. (1976) 58:699–704. doi: 10.1172/JCI108516
132. Zuk PA, Zhu M, Mizuno H, Huang J, Futrell JW, Katz AJ, et al. Multilineage cells from human adipose tissue: implications for cell-based therapies. Tissue Eng. (2001) 7:211–28. doi: 10.1089/107632701300062859
133. Bora P, Majumdar AS. Adipose tissue-derived stromal vascular fraction in regenerative medicine: a brief review on biology and translation. Stem Cell Res Ther. (2017) 8:145. doi: 10.1186/s13287-017-0598-y
134. Goncharov EN, Koval OA, Igorevich EI, Encarnacion Ramirez MJ, Nurmukhametov R, Valentinovich KK, et al. Analyzing the clinical potential of stromal vascular fraction: a comprehensive literature review. Medicina (Kaunas). (2024) 60:221. doi: 10.3390/medicina60020221
135. Frazier TP, Gimble JM, Devay JW, Tucker HA, Chiu ES, Rowan BG. Body mass index affects proliferation and osteogenic differentiation of human subcutaneous adipose tissue-derived stem cells. BMC Cell Biol. (2013) 14:34. doi: 10.1186/1471-2121-14-34
136. Minteer D, Marra KG, Rubin JP. Adipose-derived mesenchymal stem cells: biology and potential applications. Adv Biochem Eng Biotechnol. (2013) 129:59–71. doi: 10.1007/10_2012_146
137. Oedayrajsingh-Varma MJ, van Ham SM, Knippenberg M, Helder MN, Klein-Nulend J, Schouten TE, et al. Adipose tissue-derived mesenchymal stem cell yield and growth characteristics are affected by the tissue-harvesting procedure. Cytotherapy. (2006) 8:166–77. doi: 10.1080/14653240600621125
138. Jurgens WJ, Oedayrajsingh-Varma MJ, Helder MN, Zandiehdoulabi B, Schouten TE, Kuik DJ, et al. Effect of tissue-harvesting site on yield of stem cells derived from adipose tissue: implications for cell-based therapies. Cell Tissue Res. (2008) 332:415–26. doi: 10.1007/s00441-007-0555-7
139. Mildmay-White A, Khan W. Cell surface markers on adipose-derived stem cells: a systematic review. Curr Stem Cell Res Ther. (2017) 12:484–92. doi: 10.2174/1574888X11666160429122133
140. Lin CS, Ning H, Lin G, Lue TF. Is CD34 truly a negative marker for mesenchymal stromal cells? Cytotherapy. (2012) 14:1159–63. doi: 10.3109/14653249.2012.729817
141. Suga H, Matsumoto D, Eto H, Inoue K, Aoi N, Kato H, et al. Functional implications of CD34 expression in human adipose-derived stem/progenitor cells. Stem Cells Dev. (2009) 18:1201–10. doi: 10.1089/scd.2009.0003
142. Scherberich A, Di Maggio ND, McNagny KM. A familiar stranger: CD34 expression and putative functions in SVF cells of adipose tissue. World J Stem Cells. (2013) 5:1–8. doi: 10.4252/wjsc.v5.i1.1
143. Abomaray FM, Al Jumah MA, Alsaad KO, Jawdat D, Al Khaldi A, AlAskar AS, et al. Phenotypic and functional characterization of mesenchymal stem/multipotent stromal cells from Decidua Basalis of human term placenta. Stem Cells Int. (2016) 2016:5184601. doi: 10.1155/2016/5184601
144. Abumaree MH, Abomaray FM, Alshabibi MA, AlAskar AS, Kalionis B. Immunomodulatory properties of human placental mesenchymal stem/stromal cells. Placenta. (2017) 59:87–95. doi: 10.1016/j.placenta.2017.04.003
145. Díaz-Prado S, Muiños-López E, Hermida-Gómez T, Rendal-Vázquez ME, Fuentes-Boquete I, de Toro FJ, et al. Isolation and characterization of mesenchymal stem cells from human amniotic membrane. Tissue Eng Part C Methods. (2011) 17:49–59. doi: 10.1089/ten.tec.2010.0136
146. In’t Anker PS, Scherjon SA, Kleijburg-van der Keur C, Noort WA, Claas FH, Willemze R, et al. Amniotic fluid as a novel source of mesenchymal stem cells for therapeutic transplantation. Blood. (2003) 102:1548–9. doi: 10.1182/blood-2003-04-1291
147. Mathew SA, Naik C, Cahill PA, Bhonde RR. Placental mesenchymal stromal cells as an alternative tool for therapeutic angiogenesis. Cell Mol Life Sci. (2020) 77:253–65. doi: 10.1007/s00018-019-03268-1
148. Harris DT. Umbilical cord tissue mesenchymal stem cells: characterization and clinical applications. Curr Stem Cell Res Ther. (2013) 8:394–9. doi: 10.2174/1574888X11308050006
149. Yang Y, Zhang C, Sheng X. Isolation and culture of three kinds of umbilical cord mesenchymal stem cells. J Vis Exp. (2022) 186:e64065. doi: 10.3791/64065
150. Nagamura-Inoue T, He H. Umbilical cord-derived mesenchymal stem cells: their advantages and potential clinical utility. World J Stem Cells. (2014) 6:195–202. doi: 10.4252/wjsc.v6.i2.195
151. Ishige I, Nagamura-Inoue T, Honda MJ, Harnprasopwat R, Kido M, Sugimoto M, et al. Comparison of mesenchymal stem cells derived from arterial, venous, and Wharton’s jelly explants of human umbilical cord. Int J Hematol. (2009) 90:261–9. doi: 10.1007/s12185-009-0377-3
152. El Omar R, Beroud J, Stoltz JF, Menu P, Velot E, Decot V. Umbilical cord mesenchymal stem cells: the new gold standard for mesenchymal stem cell-based therapies? Tissue Eng Part B Rev. (2014) 20:523–44. doi: 10.1089/ten.teb.2013.0664
153. Uccelli A, Moretta L, Pistoia V. Mesenchymal stem cells in health and disease. Nat Rev Immunol. (2008) 8:726–36. doi: 10.1038/nri2395
154. Heo JS, Choi Y, Kim HS, Kim HO. Comparison of molecular profiles of human mesenchymal stem cells derived from bone marrow, umbilical cord blood, placenta and adipose tissue. Int J Mol Med. (2016) 37:115–25. doi: 10.3892/ijmm.2015.2413
155. Makino S, Fukuda K, Miyoshi S, Konishi F, Kodama H, Pan J, et al. Cardiomyocytes can be generated from marrow stromal cells in vitro. J Clin Invest. (1999) 103:697–705. doi: 10.1172/JCI5298
156. Xaymardan M, Tang L, Zagreda L, Pallante B, Zheng J, Chazen JL, et al. Platelet-derived growth factor-AB promotes the generation of adult bone marrow-derived cardiac myocytes. Circ Res. (2004) 94:E39–45. doi: 10.1161/01.RES.0000122042.51161.B6
157. Liu Y, Song J, Liu W, Wan Y, Chen X, Hu C. Growth and differentiation of rat bone marrow stromal cells: does 5-azacytidine trigger their cardiomyogenic differentiation? Cardiovasc Res. (2003) 58:460–8. doi: 10.1016/S0008-6363(03)00265-7
158. Yamada Y, Sakurada K, Takeda Y, Gojo S, Umezawa A. Single-cell-derived mesenchymal stem cells overexpressing Csx/Nkx2.5 and GATA4 undergo the stochastic cardiomyogenic fate and behave like transient amplifying cells. Exp Cell Res. (2007) 313:698–706. doi: 10.1016/j.yexcr.2006.11.012
159. Deng B, Wang JX, Hu XX, Duan P, Wang L, Li Y, et al. Nkx2.5 enhances the efficacy of mesenchymal stem cells transplantation in treatment heart failure in rats. Life Sci. (2017) 182:65–72. doi: 10.1016/j.lfs.2017.06.014
160. Lv Y, Liu B, Wang HP, Zhang L. Intramyocardial implantation of differentiated rat bone marrow mesenchymal stem cells enhanced by TGF-β1 improves cardiac function in heart failure rats. Braz J Med Biol Res. (2016) 49:e5273. doi: 10.1590/1414-431x20165273
161. Shen H, Wang Y, Zhang Z, Yang J, Hu S, Shen Z. Mesenchymal stem cells for cardiac regenerative therapy: optimization of cell differentiation strategy. Stem Cells Int. (2015) 2015:524756. doi: 10.1155/2015/524756
162. Shih HM, Chen YC, Yeh YT, Peng FS, Wu SC. Assessment of the feasibility of human amniotic membrane stem cell-derived cardiomyocytes in vitro. Heliyon. (2024) 10:e28398. doi: 10.1016/j.heliyon.2024.e28398
163. Toma C, Pittenger MF, Cahill KS, Byrne BJ, Kessler PD. Human mesenchymal stem cells differentiate to a cardiomyocyte phenotype in the adult murine heart. Circulation. (2002) 105:93–8. doi: 10.1161/hc0102.101442
164. Quevedo HC, Hatzistergos KE, Oskouei BN, Feigenbaum GS, Rodriguez JE, Valdes D, et al. Allogeneic mesenchymal stem cells restore cardiac function in chronic ischemic cardiomyopathy via trilineage differentiating capacity. Proc Natl Acad Sci U S A. (2009) 106:14022–7. doi: 10.1073/pnas.0903201106
165. Hatzistergos KE, Quevedo H, Oskouei BN, Hu Q, Feigenbaum GS, Margitich IS, et al. Bone marrow mesenchymal stem cells stimulate cardiac stem cell proliferation and differentiation. Circ Res. (2010) 107:913–22. doi: 10.1161/CIRCRESAHA.110.222703
166. Shake JG, Gruber PJ, Baumgartner WA, Senechal G, Meyers J, Redmond JM, et al. Mesenchymal stem cell implantation in a swine myocardial infarct model: engraftment and functional effects. Ann Thorac Surg. (2002) 73:1919–25; discussion 1926. doi: 10.1016/S0003-4975(02)03517-8
167. Silva GV, Litovsky S, Assad JA, Sousa AL, Martin BJ, Vela D, et al. Mesenchymal stem cells differentiate into an endothelial phenotype, enhance vascular density, and improve heart function in a canine chronic ischemia model. Circulation. (2005) 111:150–6. doi: 10.1161/01.CIR.0000151812.86142.45
168. Dixon JA, Gorman RC, Stroud RE, Bouges S, Hirotsugu H, Gorman JH 3rd, et al. Mesenchymal cell transplantation and myocardial remodeling after myocardial infarction. Circulation. (2009) 120:S220–9. doi: 10.1161/CIRCULATIONAHA.108.842302
169. Kalluri R, LeBleu VS. The biology, function, and biomedical applications of exosomes. Science. (2020) 367:eaau6977. doi: 10.1126/science.aau6977
170. Hade MD, Suire CN, Suo Z. Mesenchymal stem cell-derived exosomes: applications in regenerative medicine. Cells. (2021) 10:1959. doi: 10.3390/cells10081959
171. Pan BT, Johnstone RM. Fate of the transferrin receptor during maturation of sheep reticulocytes in vitro: selective externalization of the receptor. Cell. (1983) 33:967–78. doi: 10.1016/0092-8674(83)90040-5
172. Johnstone RM. The Jeanne Manery-Fisher Memorial Lecture 1991. Maturation of reticulocytes: formation of exosomes as a mechanism for shedding membrane proteins. Biochem Cell Biol. (1992) 70:179–90. doi: 10.1139/o92-028
173. Bobrie A, Colombo M, Raposo G, Théry C. Exosome secretion: molecular mechanisms and roles in immune responses. Traffic. (2011) 12:1659–68. doi: 10.1111/j.1600-0854.2011.01225.x
174. Ludwig AK, De Miroschedji K, Doeppner TR, Börger V, Ruesing J, Rebmann V, et al. Precipitation with polyethylene glycol followed by washing and pelleting by ultracentrifugation enriches extracellular vesicles from tissue culture supernatants in small and large scales. J Extracell Vesicles. (2018) 7:1528109. doi: 10.1080/20013078.2018.1528109
175. Foers AD, Chatfield S, Dagley LF, Scicluna BJ, Webb AI, Cheng L, et al. Enrichment of extracellular vesicles from human synovial fluid using size exclusion chromatography. J Extracell Vesicles. (2018) 7:1490145. doi: 10.1080/20013078.2018.1490145
176. Shafei AE, Ali MA, Ghanem HG, Shehata AI, Abdelgawad AA, Handal HR, et al. Mechanistic effects of mesenchymal and hematopoietic stem cells: new therapeutic targets in myocardial infarction. J Cell Biochem. (2018) 119:5274–86. doi: 10.1002/jcb.26637
177. Barreca MM, Cancemi P, Geraci F. Mesenchymal and induced pluripotent stem cells-derived extracellular vesicles: the new frontier for regenerative medicine? Cells. (2020) 9:1163. doi: 10.3390/cells9051163
178. Moghaddam AS, Afshari JT, Esmaeili SA, Saburi E, Joneidi Z, Momtazi-Borojeni AA. Cardioprotective microRNAs: lessons from stem cell-derived exosomal microRNAs to treat cardiovascular disease. Atherosclerosis. (2019) 285:1–9. doi: 10.1016/j.atherosclerosis.2019.03.016
179. Xu H, Wang Z, Liu L, Zhang B, Li B. Exosomes derived from adipose tissue, bone marrow, and umbilical cord blood for cardioprotection after myocardial infarction. J Cell Biochem. (2020) 121:2089–102. doi: 10.1002/jcb.27399
180. Sun SJ, Wei R, Li F, Liao SY, Tse HF. Mesenchymal stromal cell-derived exosomes in cardiac regeneration and repair. Stem Cell Rep. (2021) 16:1662–73. doi: 10.1016/j.stemcr.2021.05.003
181. Akbar N, Razzaq SS, Salim A, Haneef K. Mesenchymal stem cell-derived exosomes and their MicroRNAs in heart repair and regeneration. J Cardiovasc Transl Res. (2024) 17:505–22. doi: 10.1007/s12265-023-10449-8
182. Bjørge IM, Kim SY, Mano JF, Kalionis B, Chrzanowski W. Extracellular vesicles, exosomes and shedding vesicles in regenerative medicine—a new paradigm for tissue repair. Biomater Sci. (2018) 6:60–78. doi: 10.1039/C7BM00479F
183. Yin K, Wang S, Zhao RC. Exosomes from mesenchymal stem/stromal cells: a new therapeutic paradigm. Biomark Res. (2019) 7:8. doi: 10.1186/s40364-019-0159-x
184. Shao L, Chen Y, Li J, Chao J, Yang Z, Ding Y, et al. Hypoxia-elicited mesenchymal stem cell-derived small extracellular vesicles alleviate myocardial infarction by promoting angiogenesis through the miR-214/sufu pathway. Stem Cells Int. (2023) 2023:1662182. doi: 10.1155/2023/1662182
185. Furuta T, Miyaki S, Ishitobi H, Ogura T, Kato Y, Kamei N, et al. Mesenchymal stem cell-derived exosomes promote fracture healing in a mouse model. Stem Cells Transl Med. (2016) 5:1620–30. doi: 10.5966/sctm.2015-0285
186. Zhao J, Li X, Hu J, Chen F, Qiao S, Sun X, et al. Mesenchymal stromal cell-derived exosomes attenuate myocardial ischaemia-reperfusion injury through miR-182-regulated macrophage polarization. Cardiovasc Res. (2019) 115:1205–16. doi: 10.1093/cvr/cvz040
187. Ni J, Liu X, Yin Y, Zhang P, Xu YW, Liu Z. Exosomes derived from TIMP2-modified human umbilical cord mesenchymal stem cells enhance the repair effect in rat model with myocardial infarction possibly by the Akt/Sfrp2 pathway. Oxid Med Cell Longev. (2019) 2019:1958941. doi: 10.1155/2019/1958941
188. Toldo S, Mauro AG, Cutter Z, Abbate A. Inflammasome, pyroptosis, and cytokines in myocardial ischemia-reperfusion injury. Am J Physiol Heart Circ Physiol. (2018) 315:H1553–68. doi: 10.1152/ajpheart.00158.2018
189. Shapouri-Moghaddam A, Mohammadian S, Vazini H, Taghadosi M, Esmaeili SA, Mardani F, et al. Macrophage plasticity, polarization, and function in health and disease. J Cell Physiol. (2018) 233:6425–40. doi: 10.1002/jcp.26429
190. Kim Y, Nurakhayev S, Nurkesh A, Zharkinbekov Z, Saparov A. Macrophage polarization in cardiac tissue repair following myocardial infarction. Int J Mol Sci. (2021) 22:2715. doi: 10.3390/ijms22052715
191. Xu R, Zhang F, Chai R, Zhou W, Hu M, Liu B, et al. Exosomes derived from pro-inflammatory bone marrow-derived mesenchymal stem cells reduce inflammation and myocardial injury via mediating macrophage polarization. J Cell Mol Med. (2019) 23:7617–31. doi: 10.1111/jcmm.14635
192. Ning H, Chen H, Deng J, Xiao C, Xu M, Shan L, et al. Exosomes secreted by FNDC5-BMMSCs protect myocardial infarction by anti-inflammation and macrophage polarization via NF-κB signaling pathway and Nrf2/HO-1 axis. Stem Cell Res Ther. (2021) 12:519. doi: 10.1186/s13287-021-02591-4
193. Deng S, Zhou X, Ge Z, Song Y, Wang H, Liu X, et al. Exosomes from adipose-derived mesenchymal stem cells ameliorate cardiac damage after myocardial infarction by activating S1P/SK1/S1PR1 signaling and promoting macrophage M2 polarization. Int J Biochem Cell Biol. (2019) 114:105564. doi: 10.1016/j.biocel.2019.105564
194. Shi Y, Yang Y, Guo Q, Gao Q, Ding Y, Wang H, et al. Exosomes derived from human umbilical cord mesenchymal stem cells promote fibroblast-to-myofibroblast differentiation in inflammatory environments and benefit cardioprotective effects. Stem Cells Dev. (2019) 28:799–811. doi: 10.1089/scd.2018.0242
195. Zhu LP, Tian T, Wang JY, He JN, Chen T, Pan M, et al. Hypoxia-elicited mesenchymal stem cell-derived exosomes facilitates cardiac repair through miR-125b-mediated prevention of cell death in myocardial infarction. Theranostics. (2018) 8:6163–77. doi: 10.7150/thno.28021
196. Mao S, Zhao J, Zhang ZJ, Zhao Q. MiR-183-5p overexpression in bone mesenchymal stem cell-derived exosomes protects against myocardial ischemia/reperfusion injury by targeting FOXO1. Immunobiology. (2022) 227:152204. doi: 10.1016/j.imbio.2022.152204
197. Yue R, Lu S, Luo Y, Zeng J, Liang H, Qin D, et al. Mesenchymal stem cell-derived exosomal microRNA-182-5p alleviates myocardial ischemia/reperfusion injury by targeting GSDMD in mice. Cell Death Discov. (2022) 8:202. doi: 10.1038/s41420-022-00909-6
198. Sun C, Li W, Li Y, Chen J, An H, Zeng G, et al. MiR-182-5p mediated by exosomes derived from bone marrow mesenchymal stem cell attenuates inflammatory responses by targeting TLR4 in a mouse model of myocardial infraction. Immune Netw. (2022) 22:e49. doi: 10.4110/in.2022.22.e49
199. Fu DL, Jiang H, Li CY, Gao T, Liu MR, Li HW. MicroRNA-338 in MSCs-derived exosomes inhibits cardiomyocyte apoptosis in myocardial infarction. Eur Rev Med Pharmacol Sci. (2020) 24:10107–17. doi: 10.26355/eurrev_202010_23230 33090418
200. Wang Y, Shen Y. Exosomal miR-455-3p from BMMSCs prevents cardiac ischemia-reperfusion injury. Hum Exp Toxicol. (2022) 41:9603271221102508. doi: 10.1177/09603271221102508
201. Chen G, Wang M, Ruan Z, Zhu L, Tang C. Mesenchymal stem cell-derived exosomal miR-143-3p suppresses myocardial ischemia-reperfusion injury by regulating autophagy. Life Sci. (2021) 280:119742. doi: 10.1016/j.lfs.2021.119742
202. Pan J, Alimujiang M, Chen Q, Shi H, Luo X. Exosomes derived from miR-146a-modified adipose-derived stem cells attenuate acute myocardial infarction-induced myocardial damage via downregulation of early growth response factor 1. J Cell Biochem. (2019) 120:4433–43. doi: 10.1002/jcb.27731
203. Wang XL, Zhao YY, Sun L, Shi Y, Li ZQ, Zhao XD, et al. Exosomes derived from human umbilical cord mesenchymal stem cells improve myocardial repair via upregulation of Smad7. Int J Mol Med. (2018) 41:3063–72. doi: 10.3892/ijmm.2018.3496
204. Liang C, Liu Y, Xu H, Huang J, Shen Y, Chen F, et al. Exosomes of human umbilical cord MSCs protect against hypoxia/reoxygenation-induced pyroptosis of cardiomyocytes via the miRNA-100-5p/FOXO3/NLRP3 pathway. Front Bioeng Biotechnol. (2021) 8:615850. doi: 10.3389/fbioe.2020.615850
205. Charles CJ, Li RR, Yeung T, Mazlan SMI, Lai RC, de Kleijn DPV, et al. Systemic mesenchymal stem cell-derived exosomes reduce myocardial infarct size: characterization with MRI in a porcine model. Front Cardiovasc Med. (2020) 7:601990. doi: 10.3389/fcvm.2020.601990
206. Kore RA, Wang X, Ding Z, Griffin RJ, Tackett AJ, Mehta JL. MSC exosome-mediated cardioprotection in ischemic mouse heart comparative proteomics of infarct and peri-infarct areas. Mol Cell Biochem. (2021) 476:1691–704. doi: 10.1007/s11010-020-04029-6
207. Lin Y, Zhang F, Lian XF, Peng WQ, Yin CY. Mesenchymal stem cell-derived exosomes improve diabetes mellitus-induced myocardial injury and fibrosis via inhibition of TGF-β1/Smad2 signaling pathway. Cell Mol Biol (Noisy-le-grand). (2019) 65:123–6. doi: 10.14715/cmb/2019.65.7.21
208. Wang X, Zhu Y, Wu C, Liu W, He Y, Yang Q. Adipose-derived mesenchymal stem cells-derived exosomes carry MicroRNA-671 to alleviate myocardial infarction through inactivating the TGFBR2/Smad2 axis. Inflammation. (2021) 44:1815–30. doi: 10.1007/s10753-021-01460-9
209. Wang X, Bai L, Liu X, Shen W, Tian H, Liu W, et al. Cardiac microvascular functions improved by MSC-derived exosomes attenuate cardiac fibrosis after ischemia-reperfusion via PDGFR-β modulation. Int J Cardiol. (2021) 344:13–24. doi: 10.1016/j.ijcard.2021.09.017
210. Vanhoutte D, Heymans S. TIMPs and cardiac remodeling: ‘Embracing the MMP-independent-side of the family’. J Mol Cell Cardiol. (2010) 48:445–53. doi: 10.1016/j.yjmcc.2009.09.013
211. Wirth L, Erny E, Krane M, Lahm H, Hein L, Gilsbach R, et al. Gene expression networks in endothelial cells from failing human hearts. Am J Physiol Heart Circ Physiol. (2024) 327:H573–81. doi: 10.1152/ajpheart.00425.2024
212. Lewis FC, Kumar SD, Ellison-Hughes GM. Non-invasive strategies for stimulating endogenous repair and regenerative mechanisms in the damaged heart. Pharmacol Res. (2018) 127:33–40. doi: 10.1016/j.phrs.2017.08.016
213. Taimeh Z, Loughran J, Birks EJ, Bolli R. Vascular endothelial growth factor in heart failure. Nat Rev Cardiol. (2013) 10:519–30. doi: 10.1038/nrcardio.2013.94
214. Hu J, Chen X, Li P, Lu X, Yan J, Tan H, et al. Exosomes derived from human amniotic fluid mesenchymal stem cells alleviate cardiac fibrosis via enhancing angiogenesis in vivo and in vitro. Cardiovasc Diagn Ther. (2021) 11:348–61. doi: 10.21037/cdt-20-1032
215. Ma J, Zhao Y, Sun L, Sun X, Zhao X, Sun X, et al. Exosomes derived from akt-modified human umbilical cord mesenchymal stem cells improve cardiac regeneration and promote angiogenesis via activating platelet-derived growth factor D. Stem Cells Transl Med. (2017) 6:51–9. doi: 10.5966/sctm.2016-0038
216. Liang X, Zhang L, Wang S, Han Q, Zhao RC. Exosomes secreted by mesenchymal stem cells promote endothelial cell angiogenesis by transferring miR-125a. J Cell Sci. (2016) 129:2182–9. doi: 10.1242/jcs.170373
217. Sun J, Shen H, Shao L, Teng X, Chen Y, Liu X, et al. HIF-1α overexpression in mesenchymal stem cell-derived exosomes mediates cardioprotection in myocardial infarction by enhanced angiogenesis. Stem Cell Res Ther. (2020) 11:373. doi: 10.1186/s13287-020-01881-7
218. Zhu D, Wang Y, Thomas M, McLaughlin K, Oguljahan B, Henderson J, et al. Exosomes from adipose-derived stem cells alleviate myocardial infarction via microRNA-31/FIH1/HIF-1α pathway. J Mol Cell Cardiol. (2022) 162:10–9. doi: 10.1016/j.yjmcc.2021.08.010
219. Bougatef F, Quemener C, Kellouche S, Naïmi B, Podgorniak MP, Millot G, et al. EMMPRIN promotes angiogenesis through hypoxia-inducible factor-2alpha-mediated regulation of soluble VEGF isoforms and their receptor VEGFR-2. Blood. (2009) 114:5547–56. doi: 10.1182/blood-2009-04-217380
220. Vrijsen KR, Maring JA, Chamuleau SA, Verhage V, Mol EA, Deddens JC, et al. Exosomes from cardiomyocyte progenitor cells and mesenchymal stem cells stimulate angiogenesis via EMMPRIN. Adv Healthc Mater. (2016) 5:2555–65. doi: 10.1002/adhm.201600308
221. Bartunek J, Behfar A, Dolatabadi D, Vanderheyden M, Ostojic M, Dens J, et al. Cardiopoietic stem cell therapy in heart failure: the C-CURE (Cardiopoietic stem Cell therapy in heart failURE) multicenter randomized trial with lineage-specified biologics. J Am Coll Cardiol. (2013) 61:2329–38. doi: 10.1016/j.jacc.2013.02.071
222. Bartunek J, Terzic A, Davison BA, Filippatos GS, Radovanovic S, Beleslin B, et al. Cardiopoietic cell therapy for advanced ischaemic heart failure: results at 39 weeks of the prospective, randomized, double blind, sham-controlled CHART-1 clinical trial. Eur Heart J. (2017) 38:648–60. doi: 10.1093/eurheartj/ehw543
223. Teerlink JR, Metra M, Filippatos GS, Davison BA, Bartunek J, Terzic A, et al. Benefit of cardiopoietic mesenchymal stem cell therapy on left ventricular remodelling: results from the congestive heart failure cardiopoietic regenerative therapy (CHART-1) study. Eur J Heart Fail. (2017) 19:1520–9. doi: 10.1002/ejhf.898
224. Bartolucci J, Verdugo FJ, González PL, Larrea RE, Abarzua E, Goset C, et al. Safety and efficacy of the intravenous infusion of umbilical cord mesenchymal stem cells in patients with heart failure: a phase 1/2 randomized controlled trial (RIMECARD trial [randomized clinical trial of intravenous infusion umbilical cord mesenchymal stem cells on cardiopathy]). Circ Res. (2017) 121:1192–204. doi: 10.1161/CIRCRESAHA.117.310712
225. Mathiasen AB, Qayyum AA, Jørgensen E, Helqvist S, Kofoed KF, Haack-Sørensen M, et al. Bone marrow-derived mesenchymal stromal cell treatment in patients with ischaemic heart failure: final 4-year follow-up of the MSC-HF trial. Eur J Heart Fail. (2020) 22:884–92. doi: 10.1002/ejhf.1700
226. Suncion VY, Ghersin E, Fishman JE, Zambrano JP, Karantalis V, Mandel N, et al. Does transendocardial injection of mesenchymal stem cells improve myocardial function locally or globally?: an analysis from the percutaneous stem cell injection delivery effects on neomyogenesis (POSEIDON) randomized trial. Circ Res. (2014) 114:1292–301. doi: 10.1161/CIRCRESAHA.114.302854
227. Bolli R, Mitrani RD, Hare JM, Pepine CJ, Perin EC, Willerson JT, et al. A phase II study of autologous mesenchymal stromal cells and c-kit positive cardiac cells, alone or in combination, in patients with ischaemic heart failure: the CCTRN CONCERT-HF trial. Eur J Heart Fail. (2021) 23:661–74. doi: 10.1002/ejhf.2178
228. Zhang Y, Bi J, Huang J, Tang Y, Du S, Li P. Exosome: a review of its classification, isolation techniques, storage, diagnostic and targeted therapy applications. Int J Nanomed. (2020) 15:6917–34. doi: 10.2147/IJN.S264498
229. Gupta D, Zickler AM, El Andaloussi S. Dosing extracellular vesicles. Adv Drug Deliv Rev. (2021) 178:113961. doi: 10.1016/j.addr.2021.113961
230. Jang KW, Tu TW, Rosenblatt RB, Burks SR, Frank JA. MR-guided pulsed focused ultrasound improves mesenchymal stromal cell homing to the myocardium. J Cell Mol Med. (2020) 24:13278–88. doi: 10.1111/jcmm.15944
231. Han C, Zhou J, Liang C, Liu B, Pan X, Zhang Y, et al. Human umbilical cord mesenchymal stem cell derived exosomes encapsulated in functional peptide hydrogels promote cardiac repair. Biomater Sci. (2019) 7:2920–33. doi: 10.1039/C9BM00101H
232. Lai CP, Mardini O, Ericsson M, Prabhakar S, Maguire C, Chen JW, et al. Dynamic biodistribution of extracellular vesicles in vivo using a multimodal imaging reporter. ACS Nano. (2014) 8:483–94. doi: 10.1021/nn404945r
233. Wiklander OP, Nordin JZ, O’Loughlin A, Gustafsson Y, Corso G, Mäger I, et al. Extracellular vesicle in vivo biodistribution is determined by cell source, route of administration and targeting. J Extracell Vesicles. (2015) 4:26316. doi: 10.3402/jev.v4.26316
234. de Witte SFH, Lambert EE, Merino A, Strini T, Douben H, O’Flynn L, et al. Aging of bone marrow- and umbilical cord-derived mesenchymal stromal cells during expansion. Cytotherapy. (2017) 19:798–807. doi: 10.1016/j.jcyt.2017.03.071
235. Zhao H, Zhao H, Ji S. A mesenchymal stem cell aging framework, from mechanisms to strategies. Stem Cell Rev Rep. (2024) 20:1420–40. doi: 10.1007/s12015-024-10732-4
236. Gao Y, Chi Y, Chen Y, Wang W, Li H, Zheng W, et al. Multi-omics analysis of human mesenchymal stem cells shows cell aging that alters immunomodulatory activity through the downregulation of PD-L1. Nat Commun. (2023) 14:4373. doi: 10.1038/s41467-023-39958-5
237. Vizoso FJ, Costa LA, Eiro N. New era of mesenchymal stem cell-based medicine: basis, challenges and prospects. Rev Clin Esp (Barc). (2023) 223:619–28. doi: 10.1016/j.rce.2023.10.002
238. Wu LW, Wang YL, Christensen JM, Khalifian S, Schneeberger S, Raimondi G, et al. Donor age negatively affects the immunoregulatory properties of both adipose and bone marrow derived mesenchymal stem cells. Transpl Immunol. (2014) 30:122–7. doi: 10.1016/j.trim.2014.03.001
239. Kizilay Mancini O, Shum-Tim D, Stochaj U, Correa JA, Colmegna I. Age, atherosclerosis and type 2 diabetes reduce human mesenchymal stromal cell-mediated T-cell suppression. Stem Cell Res Ther. (2015) 6:140. doi: 10.1186/s13287-015-0127-9
240. Xu Y, Li-Ying Chan L, Chen S, Ying B, Zhang T, Liu W, et al. Optimization of UC-MSCs cold-chain storage by minimizing temperature fluctuations using an automatic cryopreservation system. Cryobiology. (2021) 99:131–9. doi: 10.1016/j.cryobiol.2020.11.010
241. Gurunathan S, Kang MH, Jeyaraj M, Qasim M, Kim JH. Review of the isolation, characterization, biological function, and multifarious therapeutic approaches of exosomes. Cells. (2019) 8:307. doi: 10.3390/cells8040307
242. Kimiz-Gebologlu I, Oncel SS. Exosomes: large-scale production, isolation, drug loading efficiency, and biodistribution and uptake. J Control Release. (2022) 347:533–43. doi: 10.1016/j.jconrel.2022.05.027
243. Jeyaram A, Jay SM. Preservation and storage stability of extracellular vesicles for therapeutic applications. AAPS J. (2018) 20:1. doi: 10.1208/s12248-017-0160-y
244. Jones EA, English A, Kinsey SE, Straszynski L, Emery P, Ponchel F, et al. Optimization of a flow cytometry-based protocol for detection and phenotypic characterization of multipotent mesenchymal stromal cells from human bone marrow. Cytometry B Clin Cytom. (2006) 70:391–9. doi: 10.1002/cyto.b.20118
245. van der Pol E, van Gemert MJ, Sturk A, Nieuwland R, van Leeuwen TG. Single vs. swarm detection of microparticles and exosomes by flow cytometry. J Thromb Haemost. (2012) 10:919–30. doi: 10.1111/j.1538-7836.2012.04683.x
246. Gualerzi A, Niada S, Giannasi C, Picciolini S, Morasso C, Vanna R, et al. Raman spectroscopy uncovers biochemical tissue-related features of extracellular vesicles from mesenchymal stromal cells. Sci Rep. (2017) 7:9820. doi: 10.1038/s41598-017-10448-1
247. Welsh JA, Goberdhan DCI, O’Driscoll L, Buzas EI, Blenkiron C, Bussolati B, et al. Minimal information for studies of extracellular vesicles (MISEV2023): from basic to advanced approaches. J Extracell Vesicles. (2024) 13:e12404. doi: 10.1002/jev2.12404
248. Lener T, Gimona M, Aigner L, Börger V, Buzas E, Camussi G, et al. Applying extracellular vesicles based therapeutics in clinical trials—an ISEV position paper. J Extracell Vesicles. (2015) 4:30087. doi: 10.3402/jev.v4.30087
249. Witwer KW, Goberdhan DC, O’Driscoll L, Théry C, Welsh JA, Blenkiron C, et al. Updating MISEV: evolving the minimal requirements for studies of extracellular vesicles. J Extracell Vesicles. (2021) 10:e12182. doi: 10.1002/jev2.12182
250. Li W, Liu Q, Shi J, Xu X, Xu J. The role of TNF-α in the fate regulation and functional reprogramming of mesenchymal stem cells in an inflammatory microenvironment. Front Immunol. (2023) 14:1074863. doi: 10.3389/fimmu.2023.1074863
251. Sioud M, Mobergslien A, Boudabous A, Fløisand Y. Mesenchymal stem cell-mediated T cell suppression occurs through secreted galectins. Int J Oncol. (2011) 38:385–90. doi: 10.3892/ijo.2010.869
252. Liu GY, Xu Y, Li Y, Wang LH, Liu YJ, Zhu D. Secreted galectin-3 as a possible biomarker for the immunomodulatory potential of human umbilical cord mesenchymal stromal cells. Cytotherapy. (2013) 15:1208–17. doi: 10.1016/j.jcyt.2013.05.011
253. Paganelli A, Diomede F, Marconi GD, Pizzicannella J, Rajan TS, Trubiani O, et al. Inhibition of LPS-induced inflammatory response of oral mesenchymal stem cells in the presence of galectin-3. Biomedicines. (2023) 11:1519. doi: 10.3390/biomedicines11061519
254. Liu Y, Yuan X, Muñoz N, Logan TM, Ma T. Commitment to aerobic glycolysis sustains immunosuppression of human mesenchymal stem cells. Stem Cells Transl Med. (2019) 8:93–106. doi: 10.1002/sctm.18-0070
255. Barrère-Lemaire S, Vincent A, Jorgensen C, Piot C, Nargeot J, Djouad F. Mesenchymal stromal cells for improvement of cardiac function following acute myocardial infarction: a matter of timing. Physiol Rev. (2024) 104:659–725. doi: 10.1152/physrev.00009.2023
256. Yang YJ, Qian HY, Huang J, Geng YJ, Gao RL, Dou KF, et al. Atorvastatin treatment improves survival and effects of implanted mesenchymal stem cells in post-infarct swine hearts. Eur Heart J. (2008) 29:1578–90. doi: 10.1093/eurheartj/ehn167
257. Song L, Yang YJ, Dong QT, Qian HY, Gao RL, Qiao SB, et al. Atorvastatin enhance efficacy of mesenchymal stem cells treatment for swine myocardial infarction via activation of nitric oxide synthase. PLoS One. (2013) 8:e65702. doi: 10.1371/journal.pone.0065702
258. Fan CQ, Leu S, Sheu JJ, Zhen YY, Tsai TH, Chen YL, et al. Prompt bone marrow-derived mesenchymal stem cell therapy enables early porcine heart function recovery from acute myocardial infarction. Int Heart J. (2014) 55:362–71. doi: 10.1536/ihj.14-007
259. Schuleri KH, Feigenbaum GS, Centola M, Weiss ES, Zimmet JM, Turney J, et al. Autologous mesenchymal stem cells produce reverse remodelling in chronic ischaemic cardiomyopathy. Eur Heart J. (2009) 30:2722–32. doi: 10.1093/eurheartj/ehp265
260. Tang XL, Wysoczynski M, Gumpert AM, Li Y, Wu WJ, Li H, et al. Effect of intravenous cell therapy in rats with old myocardial infarction. Mol Cell Biochem. (2022) 477:431–44. doi: 10.1007/s11010-021-04283-2
261. Attar A, Bahmanzadegan Jahromi F, Kavousi S, Monabati A, Kazemi A. Mesenchymal stem cell transplantation after acute myocardial infarction: a meta-analysis of clinical trials. Stem Cell Res Ther. (2021) 12:600. doi: 10.1186/s13287-021-02667-1
262. Cong L, Ran FA, Cox D, Lin S, Barretto R, Habib N, et al. Multiplex genome engineering using CRISPR/cas systems. Science. (2013) 339:819–23. doi: 10.1126/science.1231143
263. Zhang Z, Zhang Y, Gao F, Han S, Cheah KS, Tse HF, et al. CRISPR/Cas9 genome-editing system in human stem cells: current status and future prospects. Mol Ther Nucleic Acids. (2017) 9:230–41. doi: 10.1016/j.omtn.2017.09.009
264. Han AR, Shin HR, Kwon J, Lee SB, Lee SE, Kim EY, et al. Highly efficient genome editing via CRISPR-Cas9 ribonucleoprotein (RNP) delivery in mesenchymal stem cells. BMB Rep. (2024) 57:60–5. doi: 10.5483/BMBRep.2023-0113
265. Moeinabadi-Bidgoli K, Mazloomnejad R, Beheshti Maal A, Asadzadeh Aghdaei H, Kazem Arki M, Hossein-Khannazer N, et al. Genetic modification and preconditioning strategies to enhance functionality of mesenchymal stromal cells: a clinical perspective. Expert Opin Biol Ther. (2023) 23:461–78. doi: 10.1080/14712598.2023.2205017
266. Karpov AA, Udalova DV, Pliss MG, Galagudza MM. Can the outcomes of mesenchymal stem cell-based therapy for myocardial infarction be improved? Providing weapons and armour to cells. Cell Prolif. (2017) 50:e12316. doi: 10.1111/cpr.12316
267. McGinley LM, McMahon J, Stocca A, Duffy A, Flynn A, O’Toole D, et al. Mesenchymal stem cell survival in the infarcted heart is enhanced by lentivirus vector-mediated heat shock protein 27 expression. Hum Gene Ther. (2013) 24:840–51. doi: 10.1089/hum.2011.009
268. Tsubokawa T, Yagi K, Nakanishi C, Zuka M, Nohara A, Ino H, et al. Impact of anti-apoptotic and anti-oxidative effects of bone marrow mesenchymal stem cells with transient overexpression of heme oxygenase-1 on myocardial ischemia. Am J Physiol Heart Circ Physiol. (2010) 298:H1320–9. doi: 10.1152/ajpheart.01330.2008
269. Tang J, Wang J, Guo L, Kong X, Yang J, Zheng F, et al. Mesenchymal stem cells modified with stromal cell-derived factor 1 alpha improve cardiac remodeling via paracrine activation of hepatocyte growth factor in a rat model of myocardial infarction. Mol Cells. (2010) 29:9–19. doi: 10.1007/s10059-010-0001-7
270. Cheng Z, Ou L, Zhou X, Li F, Jia X, Zhang Y, et al. Targeted migration of mesenchymal stem cells modified with CXCR4 gene to infarcted myocardium improves cardiac performance. Mol Ther. (2008) 16:571–9. doi: 10.1038/sj.mt.6300374
271. Duan HF, Wu CT, Wu DL, Lu Y, Liu HJ, Ha XQ, et al. Treatment of myocardial ischemia with bone marrow-derived mesenchymal stem cells overexpressing hepatocyte growth factor. Mol Ther. (2003) 8:467–74. doi: 10.1016/S1525-0016(03)00186-2
272. Haider H, Jiang S, Idris NM, Ashraf M. IGF-1-overexpressing mesenchymal stem cells accelerate bone marrow stem cell mobilization via paracrine activation of SDF-1alpha/CXCR4 signaling to promote myocardial repair. Circ Res. (2008) 103:1300–8. doi: 10.1161/CIRCRESAHA.108.186742
273. Liu XH, Bai CG, Xu ZY, Huang SD, Yuan Y, Gong DJ, et al. Therapeutic potential of angiogenin modified mesenchymal stem cells: angiogenin improves mesenchymal stem cells survival under hypoxia and enhances vasculogenesis in myocardial infarction. Microvasc Res. (2008) 76:23–30. doi: 10.1016/j.mvr.2008.02.005
274. Hu X, Yu SP, Fraser JL, Lu Z, Ogle ME, Wang JA, et al. Transplantation of hypoxia-preconditioned mesenchymal stem cells improves infarcted heart function via enhanced survival of implanted cells and angiogenesis. J Thorac Cardiovasc Surg. (2008) 135:799–808. doi: 10.1016/j.jtcvs.2007.07.071
275. Wang X, Chen Y, Zhao Z, Meng Q, Yu Y, Sun J, et al. Engineered exosomes with ischemic myocardium-targeting peptide for targeted therapy in myocardial infarction. J Am Heart Assoc. (2018) 7:e008737. doi: 10.1161/JAHA.118.008737
276. Yuan J, Yang H, Liu C, Shao L, Zhang H, Lu K, et al. Microneedle patch loaded with exosomes containing MicroRNA-29b prevents cardiac fibrosis after myocardial infarction. Adv Healthc Mater. (2023) 12:e2202959. doi: 10.1002/adhm.202202959
277. Ben XY, Wang YR, Zheng HH, Li DX, Ren R, Ni PL, et al. Construction of exosomes that overexpress CD47 and evaluation of their immune escape. Front Bioeng Biotechnol. (2022) 10:936951. doi: 10.3389/fbioe.2022.936951
278. Liu S, Xiao X, Zhang L, Wang J, Zhao W, Liu H, et al. Reprogramming exosomes to escape from immune surveillance for mitochondrial protection in hepatic ischemia-reperfusion injury. Theranostics. (2024) 14:116–32. doi: 10.7150/thno.88061
279. Robinson ST, Douglas AM, Chadid T, Kuo K, Rajabalan A, Li H, et al. A novel platelet lysate hydrogel for endothelial cell and mesenchymal stem cell-directed neovascularization. Acta Biomater. (2016) 36:86–98. doi: 10.1016/j.actbio.2016.03.002
280. Ichihara Y, Kaneko M, Yamahara K, Koulouroudias M, Sato N, Uppal R, et al. Self-assembling peptide hydrogel enables instant epicardial coating of the heart with mesenchymal stromal cells for the treatment of heart failure. Biomaterials. (2018) 154:12–23. doi: 10.1016/j.biomaterials.2017.10.050
281. Lv K, Li Q, Zhang L, Wang Y, Zhong Z, Zhao J, et al. Incorporation of small extracellular vesicles in sodium alginate hydrogel as a novel therapeutic strategy for myocardial infarction. Theranostics. (2019) 9:7403–16. doi: 10.7150/thno.32637
282. Bou-Ghannam S, Kim K, Grainger DW, Okano T. 3D cell sheet structure augments mesenchymal stem cell cytokine production. Sci Rep. (2021) 11:8170. doi: 10.1038/s41598-021-87571-7
283. Bumroongthai K, Kavanagh DPJ, Genever P, Kalia N. Improving vasculoprotective effects of MSCs in coronary microvessels—benefits of 3D culture, sub-populations and heparin. Front Immunol. (2023) 14:1257497. doi: 10.3389/fimmu.2023.1257497
284. Ho SS, Murphy KC, Binder BY, Vissers CB, Leach JK. Increased survival and function of mesenchymal stem cell spheroids entrapped in instructive alginate hydrogels. Stem Cells Transl Med. (2016) 5:773–81. doi: 10.5966/sctm.2015-0211
285. Lee EJ, Park SJ, Kang SK, Kim GH, Kang HJ, Lee SW, et al. Spherical bullet formation via E-cadherin promotes therapeutic potency of mesenchymal stem cells derived from human umbilical cord blood for myocardial infarction. Mol Ther. (2012) 20:1424–33. doi: 10.1038/mt.2012.58
286. Liu BH, Yeh HY, Lin YC, Wang MH, Chen DC, Lee BH, et al. Spheroid formation and enhanced cardiomyogenic potential of adipose-derived stem cells grown on chitosan. Biores Open Access. (2013) 2:28–39. doi: 10.1089/biores.2012.0285
287. Levit RD, Landázuri N, Phelps EA, Brown ME, García AJ, Davis ME, et al. Cellular encapsulation enhances cardiac repair. J Am Heart Assoc. (2013) 2:e000367. doi: 10.1161/JAHA.113.000367
288. Wright EJ, Farrell KA, Malik N, Kassem M, Lewis AL, Wallrapp C, et al. Encapsulated glucagon-like peptide-1-producing mesenchymal stem cells have a beneficial effect on failing pig hearts. Stem Cells Transl Med. (2012) 1:759–69. doi: 10.5966/sctm.2012-0064
289. Yu J, Du KT, Fang Q, Gu Y, Mihardja SS, Sievers RE, et al. The use of human mesenchymal stem cells encapsulated in RGD modified alginate microspheres in the repair of myocardial infarction in the rat. Biomaterials. (2010) 31:7012–20. doi: 10.1016/j.biomaterials.2010.05.078
290. Silva LH, Cruz FF, Morales MM, Weiss DJ, Rocco PR. Magnetic targeting as a strategy to enhance therapeutic effects of mesenchymal stromal cells. Stem Cell Res Ther. (2017) 8:58. doi: 10.1186/s13287-017-0523-4
291. Liu S, Chen X, Bao L, Liu T, Yuan P, Yang X, et al. Treatment of infarcted heart tissue via the capture and local delivery of circulating exosomes through antibody-conjugated magnetic nanoparticles. Nat Biomed Eng. (2020) 4:1063–75. doi: 10.1038/s41551-020-00637-1
292. Zhang BF, Jiang H, Chen J, Hu Q, Yang S, Liu XP. Silica-coated magnetic nanoparticles labeled endothelial progenitor cells alleviate ischemic myocardial injury and improve long-term cardiac function with magnetic field guidance in rats with myocardial infarction. J Cell Physiol. (2019) 234:18544–59. doi: 10.1002/jcp.28492
293. Gong X, Fan G, Wang W, Wang G. Trimetazidine protects umbilical cord mesenchymal stem cells against hypoxia and serum deprivation induced apoptosis by activation of Akt. Cell Physiol Biochem. (2014) 34:2245–55. doi: 10.1159/000369667
294. Wisel S, Khan M, Kuppusamy ML, Mohan IK, Chacko SM, Rivera BK, et al. Pharmacological preconditioning of mesenchymal stem cells with trimetazidine (1-[2,3,4-trimethoxybenzyl]piperazine) protects hypoxic cells against oxidative stress and enhances recovery of myocardial function in infarcted heart through Bcl-2 expression. J Pharmacol Exp Ther. (2009) 329:543–50. doi: 10.1124/jpet.109.150839
295. Han D, Huang W, Li X, Gao L, Su T, Li X, et al. Melatonin facilitates adipose-derived mesenchymal stem cells to repair the murine infarcted heart via the SIRT1 signaling pathway. J Pineal Res. (2016) 60:178–92. doi: 10.1111/jpi.12299
296. Zhang Q, Wang H, Yang YJ, Dong QT, Wang TJ, Qian HY, et al. Atorvastatin treatment improves the effects of mesenchymal stem cell transplantation on acute myocardial infarction: the role of the RhoA/ROCK/ERK pathway. Int J Cardiol. (2014) 176:670–9. doi: 10.1016/j.ijcard.2014.07.071
297. Qu Z, Xu H, Tian Y, Jiang X. Atorvastatin improves microenvironment to enhance the beneficial effects of BMSCs therapy in a rabbit model of acute myocardial infarction. Cell Physiol Biochem. (2013) 32:380–9. doi: 10.1159/000354445
298. Xu JY, Qian HY, Huang PS, Xu J, Xiong YY, Jiang WY, et al. Transplantation efficacy of autologous bone marrow mesenchymal stem cells combined with atorvastatin for acute myocardial infarction (TEAM-AMI): rationale and design of a randomized, double-blind, placebo-controlled, multi-center, phase II TEAM-AMI trial. Regen Med. (2019) 14:1077–87. doi: 10.2217/rme-2019-0024
299. Huang P, Wang L, Li Q, Xu J, Xu J, Xiong Y, et al. Combinatorial treatment of acute myocardial infarction using stem cells and their derived exosomes resulted in improved heart performance. Stem Cell Res Ther. (2019) 10:300. doi: 10.1186/s13287-019-1353-3
Keywords: heart failure, treatment, mesenchymal stem cells, exosomes, cell transplantation
Citation: Chen C, Zhong W, Zheng H, Zhao W, Wang Y and Shen B (2025) Current state of heart failure treatment: are mesenchymal stem cells and their exosomes a future therapy? Front. Cardiovasc. Med. 12:1518036. doi: 10.3389/fcvm.2025.1518036
Received: 27 October 2024; Accepted: 16 April 2025;
Published: 28 April 2025.
Edited by:
William Carlson, MassGeneraHospital and Harvard Medical School, United StatesReviewed by:
Naresh Kumar, The Ohio State University, United StatesGentaro Ikeda, Stanford University, United States
Copyright: © 2025 Chen, Zhong, Zheng, Zhao, Wang and Shen. This is an open-access article distributed under the terms of the Creative Commons Attribution License (CC BY). The use, distribution or reproduction in other forums is permitted, provided the original author(s) and the copyright owner(s) are credited and that the original publication in this journal is cited, in accordance with accepted academic practice. No use, distribution or reproduction is permitted which does not comply with these terms.
*Correspondence: Botao Shen, c2hlbmJ0QGpsdS5lZHUuY24=