- 1Department of Comprehensive Internal Medicine, The Affiliated Hospital of Qingdao University, Qingdao, Shandong, China
- 2School of Basic Medicine, Qingdao University, Qingdao, Shandong, China
- 3Institute for Translational Medicine, The Affiliated Hospital of Qingdao University, Qingdao Medical College, Qingdao University, Qingdao, Shandong, China
The limited capacity of adult mammalian cardiomyocytes to undergo cell division and proliferation is one of the key factors contributing to heart failure. In newborn mice, cardiac proliferation occurs during a brief window, but this proliferative capacity diminishes by 7 days after birth. Current studies on cardiac regeneration focused on elucidating changes in regulatory factors within the heart before and after this proliferative window, aiming to determine whether potential association between these factors and cell cycle arrest in cardiomyocytes. Facilitating the re-entry of cardiomyocytes into the cell cycle or reversing their exit from it represents a critical strategy for cardiac regeneration. This paper provides an overview of the role of cell cycle arrest in cardiac regeneration, briefly describes cardiomyocyte proliferation and cardiac regeneration, and systematically summarizes the regulation of the cell cycle arrest in cardiomyocytes, and the potential metabolic mechanisms underlying cardiomyocyte cycle arrest. Additionally, we highlight the development of cardiovascular disease drugs targeting cardiomyocyte cell cycle regulation and their status in clinical treatment. Our goal is to outline strategies for promoting cardiac regeneration and repair following cardiac injury, while also pointing toward future research directions that may offer new technologies and prospects for treating cardiovascular diseases, such as myocardial infarction, arrhythmia and heart failure.
1 Introduction
Cardiovascular disease (CVD), especially ischemic heart disease, has become a major global public health challenge and remains the leading cause of mortality worldwide, despite considerable advancements in prevention and treatment of CVDs (1). The limited regenerative capacity of the adult mammalian heart contributes to the progression of almost all CVDs to heart failure (HF). Studies have found that the renewal rate of human cardiomyocytes decreases significantly with age. From 1% annual renewal at age 25, it gradually decreases to 0.45% at age 75 (2). This diminished regenerative capacity further exacerbates the progression of HF. Cardiomyocytes lost due to cardiomyopathic injury, such as myocardial infarction (MI), can't be sufficiently replenished through proliferation and division in the adult mammalian heart, resulting in functional impairment at the site of injury and the formation of irreversible fibrotic scars. This heightened vulnerability increases the heart's susceptibility to subsequent damage (3). Currently, the prevailing clinical strategy for treating CVDs focuses on delaying disease progression rather than promoting repair or regeneration. For patients with end-stage HF, cardiac organ transplantation remains the only effective treatment option. However, the scarcity of transplantable organs falls far short of meeting the substantial clinical demand. Therefore, elucidating the regenerative mechanisms of endogenous cardiomyocytes represents a critical avenue for advancing CVD treatment.
The conventional belief is that the adult mammalian heart is a terminally differentiated organ in which cardiomyocytes have limited ability for mitosis and regeneration. However, in 2011, Porrello et al. demonstrated that neonatal mice exhibit significant endogenous cardiomyocyte proliferation and cardiac regenerative potential during the first seven days of life (P7). Notably, when P7 mice underwent apicoectomy, no myocardial regeneration occurred at the site of injury, and irreversible fibrosis developed, indicating a loss of regenerative capacity beyond this critical period (4). Consequently, what factors restrict cardiomyocyte proliferation in mammals, such as in P7 mice, thereby limiting cardiac regeneration? Therefore, elucidating the regulatory mechanisms governing cardiomyocyte proliferation and identifying key molecular switches controlling their cell cycle progression represents a central focus of cardiac regeneration regeneration.
The loss of cardiac regenerative capacity in adult mammals primarily results from the cessation of the cell cycle in cardiomyocytes, effectively closing the window for proliferation. First, there is a marked shift in energy metabolism between the embryonic and adult hearts. Specifically, embryonic cardiomyocytes predominantly rely on anaerobic glycolysis as their primary energy source, whereas adult cardiomyocytes primarily utilize oxygen-dependent mitochondrial oxidative phosphorylation (5). Second, the postnatal transition from anaerobic to aerobic respiration triggers an increase in reactive oxygen species (ROS) production and activates the DNA damage response pathway (DDR), ultimately leading to cell cycle arrest and maturation of cardiomyocytes (5–7). This raises the critical question of whether cell cycle withdrawal represents a cause or consequence of the diminished cardiac regenerative capacity. Taken together, these findings suggest that cell cycle activity plays a pivotal role in determining cardiac regenerative capacity.
In this paper, we comprehensively review the regulation of the cell cycle in cardiac regeneration, along with the regulators and mechanisms underlying myocardial cell cycle arrest that have been reported in recent years. Furthermore, we summarize cardiac regeneration strategies targeting the myocardial cell cycle, which provide innovative insights for cardiac regeneration and repair research.
2 Cardiomyocyte proliferation and cardiac regeneration
2.1 Source of neonatal cardiomyocytes
Cardiac regeneration has garnered substantial attention, with a central scientific question being the origin of newborn cardiomyocytes. Current research proposes potential sources, including the transdifferentiation from other cell types and the differentiation of progenitor cells, and dedifferentiation of pre-existing cardiomyocytes (Figure 1).
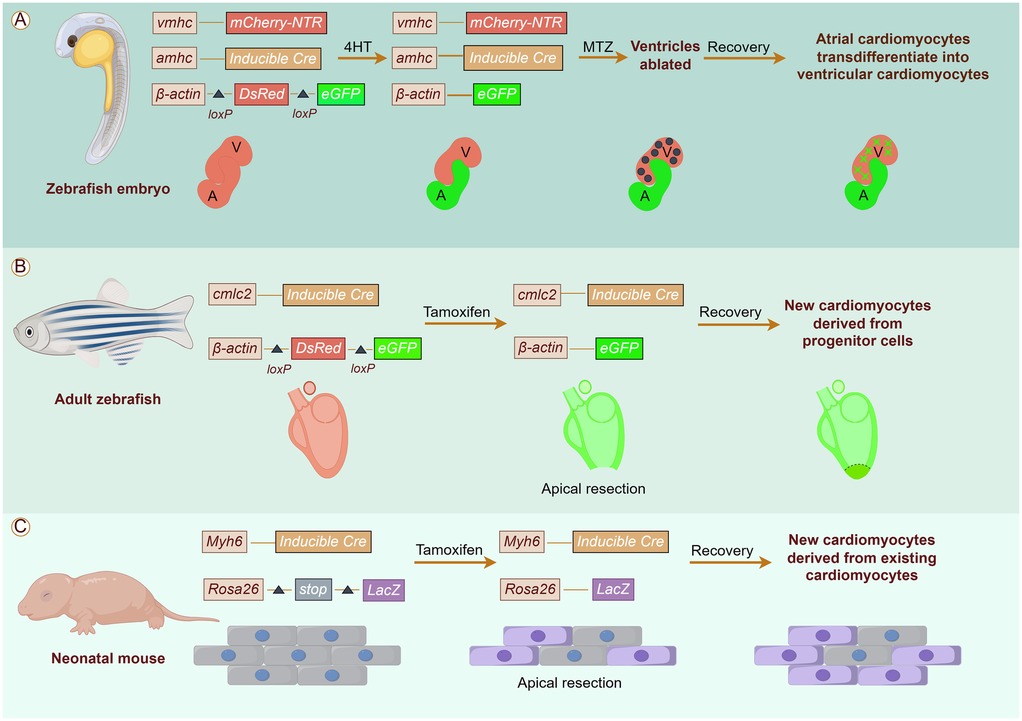
Figure 1. Origins of cardiomyocytes in cardiac regeneration. The potential sources of cardiomyocytes include (A) Transdifferentiation from other cell types; (B) Differentiation of progenitor cells; (C) Differentiation of progenitor cells.
The zebrafish heart has an amazing ability to regenerate throughout its life, thanks largely to the fact that its cardiomyocytes never fully exit the cell cycle and are able to proliferate in response to signals of injury to replenish lost myocardium (8). Fate mapping studies in zebrafish heart regeneration have demonstrated that fish replace lost cardiomyocytes primarily through the proliferation of undifferentiated cardiac precursor cells. By utilizing an EGFP dual fluorescent reporter gene driven by the cmlc2 promoter, researchers successfully traced the origin of newborn cardiomyocytes. Prior to cardiac injury, all cmlc2-expressing cardiomyocytes were labeled by eGFP expression, and most regenerating tissues exhibited eGFP expression 30 days after ventricular resection. These findings indicate that the newborn myocardium is predominantly derived from progenitor cells that subsequently differentiate into proliferative cardiomyocytes (9, 10). In recent years, studies have shown that transdifferentiation also contributes to cardiac regeneration. For instance, Zhang et al. discovered that differentiated atrial cardiomyocytes can transdifferentiate into ventricular cardiomyocytes using a combination of genetic fate mapping mapping and ventricle-specific genetic ablation system in zebrafish models. Notably, inhibition of Notch signaling blocked atrial-to-ventricular transdifferentiation and impaired cardiac regeneration (11). In addition, Poss et al. utilized a lineage-tracing method to fluorescently label zebrafish cardiomyocytes, confirming that neonatal cardiomyocytes are derived from pre-existing ones (12).
The switching of growth patterns in the mammalian heart during cardiac maturation is tightly controlled. Thus, mammalian cardiomyocytes regenerated after neonatal injury are derived from pre-existing cardiomyocytes, utilizing their proliferative activity that has not been completely lost, rather than transdifferentiated or induced stem cells (13). For example, Koudstaal et al. found by gene genealogical tracing techniques that cardiomyocytes can replenish damaged myocardium by de-differentiating and re-entering the cell cycle after injury in the neonatal mouse heart (14). The viewpoint was further substantiated by investigations into neonatal rat heart regeneration (4).
Overall, these investigations not only provide compelling evidence for cardiac plasticity during myocardial injury but also reveal multiple potential sources of cardiac resident cells for cardiac regeneration.
2.2 Cardiac regeneration in low-vertebrates and mammals
Lower vertebrates demonstrate superior cardiomyocyte regeneration capabilities compared to mammals. Zebrafish have exhibited successful regeneration and functional recovery in various cardiac injury models, including apical resection (AR), cryoinjury, and genetic cell ablation (12, 15–18). Amongst low-vertebrate species, tailless amphibians are also renowned for their remarkable cardiac regeneration capabilities. Following cardiac injury in salamanders, complete restoration of cardiac function without scarring can be achieved within three months (19–21). Additionally, it has been observed that adult frog cardiomyocytes limited some proliferative capacity and the ability to dedifferentiate. However, scar tissue formation occurs after cardiac injury, preventing complete cardiac regeneration (22).
The most significant difference between mammals and lower vertebrates lies in their capacity to complete the cell cycle (23, 24). In contrast to haploid cardiomyocytes, polyploid cardiomyocytes exhibit restricted proliferative capacity and typically fail to progress through the normal cell cycle (25). Furthermore, it has been demonstrated that mononuclear diploid cardiomyocytes play a crucial role in cardiac regeneration and repair due to their substantial proliferative potential (26). Unlike zebrafish mononuclear cardiomyocytes, which can re-enter the cell cycle during adulthood, adult mammalian cardiomyocytes transition from proliferative mononuclear diploid cells to nonproliferating binucleated diploid cells either before (27) or shortly after birth (28), thereby limiting their proliferative capacity (28, 29). Whether diploid or polyploid cardiomyocytes possess proliferate capabilities during cardiac regeneration and contribute to myocardial repair, as well as offering therapeutic strategies remains an area requiring further investigation.
Unlike zebrafish, adult mammalian hearts exhibit limited cardiac regeneration following injury (30). However, studies of neonatal cardiac regeneration in mice have demonstrated robust regenerative responses in injured areas after AR and MI surgery (4, 31). These findings highlight a specific time window during which the mammalian heart displays a strong regenerative response immediately after birth. However, Robledo et al. reported that the rat heart fails to achieve complete regeneration when subjected to burn injury 4–7 days after birth, underscoring the need for further investigation into the mechanisms underlying the differences in regenerative capacity between neonatal rats and mice (32). Interestingly, AR surgery performed on mice seven days after birth did not trigger a significant regenerative response (4), indicating that the heart's regenerative potential significantly diminishes shortly after birth. Recent reports on neonatal corrective cardiac surgery (33) and neonatal MI (34) suggest that human neonates may possess a higher regenerative capacity, enabling them to recove cardiac function effectively.
In the context of cardiac regeneration, lower vertebrates such as zebrafish exhibit complete restoration of the heart following injury, whereas mammals lose this regenerative capacity shortly after birth. Thus, what factors restrict cardiomyocyte proliferation, thereby reducing the regenerative potential in mammals? Consequently, elucidating the regulatory mechanisms that govern cardiomyocyte proliferation and identifying key regulators that orchestrate cardiac regeneration remain crucial objectives in cardiac regeneration research.
Overall, there are significant differences in the origin of neonatal cardiomyocytes and the regenerative capacity of the heart between lower vertebrates and mammals. Nevertheless, these studies provide an important theoretical foundation for the advancement of cardiac regenerative medicine. The development of cardiac regenerative medicine will emphasize multidisciplinary collaboration and innovation, integrating cell therapy, gene therapy, tissue engineering, and biomaterials to achieve effective treatment after MI. Future research will need to further optimize these techniques, address existing challenges, and facilitate the broader application of regenerative medicine in MI treatment.
3 Modulation of cell cycle on cardiomyocyte proliferation
Cell proliferation depends on the cell cycle, a complicated process that spans from the generation of daughter cells through one round of division to the subsequent preparation for new rounds of division. At the molecular level, this process is synergistically regulated by specific cellular cyclins (Cyclin) and cyclin-dependent kinases (CDKs) (35) (Figure 2).
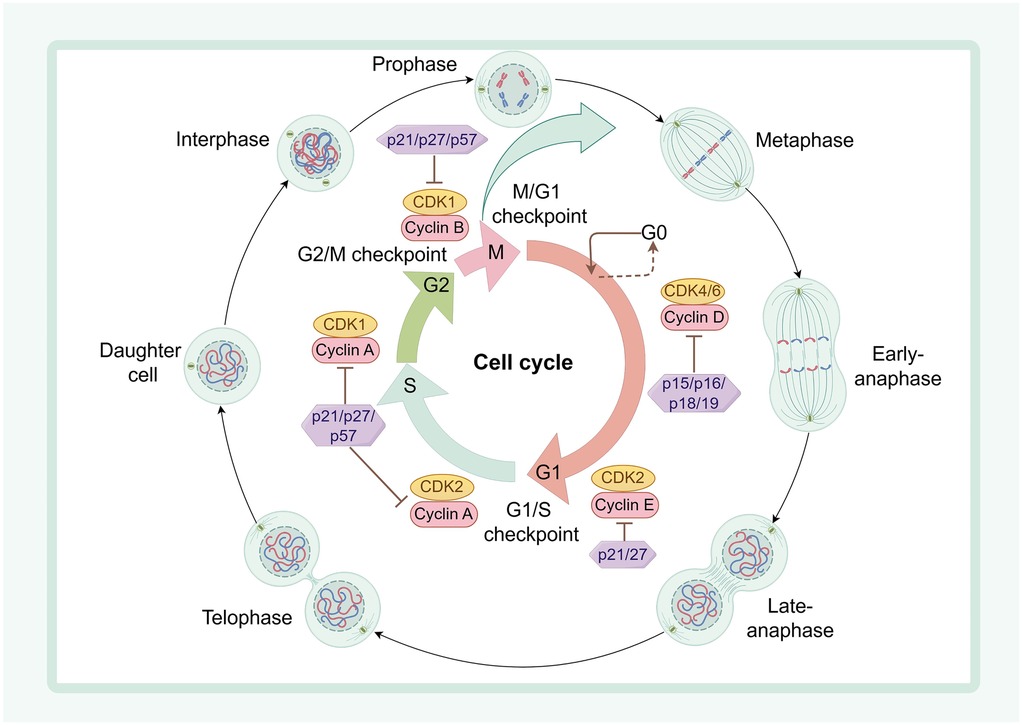
Figure 2. The regulatory mechanism of cell cycle in cardiac regeneration. Cyclin D interacts with CDK4/6 to initiate entry into G1 phase; late G1 CyclinE synergizes with CDK2 to enter S phase; CDK2, CDK1, and Cyclin A coordinate with each other to drive progression through S and G2 phases, respectively; and Cyclin B interacts with CDK1 to drive progression from late G2 to the end of mitosis. In contrast, two inhibitor families, the INK4 family (p15, p16, p18, and p19) and the WAF1/CIP/KIP family (p21, p27, and p57), interact with CDK to arrest the cell cycle. Cytoplasmic divisions are divided into interphase, prophase, metaphase, anaphase and telophase.
The activation of CDKs is dependent on the dynamic regulation of their associated cyclins (36). Notably, CDKs and specific cyclins play distinct roles during different phases of the cell cycle. Specifically, CDK1, CDK2, CDK4, and CDK6 are crucial for cell cycle progression (37–39). For instance, growth factors promote entry into the G1 phase, activate Cyclin D1, D2, and D3, and subsequently interact with CDK4 or CDK6 to drive cell cycle progression (40–42). It has been reported that overexpression of cyclin D2 in mice following MI induces DNA synthesis and cardiomyocyte proliferation while reducing infarct size (43). Furthermore, CDK1 and Cyclin B regulate progression from late G2 to the end of mitosis (38, 44). Overexpression of the Cyclin B1 complexed with cell division cycle kinase 2 (CDC2) facilitates cardiomyocyte division in adult mice, whereas deletion of this complex leads to G2/M phase arrest and inhibits proliferation in adult mouse cardiomyocytes (45). Additionally, overexpression of Cyclin A2 induces DNA synthesis and cardiomyocyte mitosis, thereby enhancing cardiac function in ischemically injured mice (46).
CDK-Cyclin activity is regulated by cyclin-dependent protein kinase inhibitory proteins (CKIs). A combination of four cell cycle regulators, CDk1 with CCNB and Cdk4 with CCND, activates cardiomyocyte proliferation. Furthermore, the use of Wee1 inhibitors in conjunction with TGF-β inhibitors promotes cardiomyocyte proliferation, reduces fibrosis area after cardiac injury, and improves cardiac function (47). Recent studies have revealed that adult mouse cardiomyocytes harbor multiple negative regulators in the form of CKI proteins, which restrict the cycling activity of these cells in adult mice, thereby suppressing their proliferative and division capabilities (48).
Changes in postnatal mammalian nutrient metabolism and ingestion lead to increased production of ROS and activation of the DDR (5, 6), both of which result in aberrant transcription of CDKs-Cyclin, leading to uncontrolled mitosis and cell cycle arrest in cardiomyocytes (49, 50). Modulating cell cycle regulators during cardiac growth and development to influence cardiomyocyte proliferation, thereby promoting cardiac regeneration, is anticipated as a prospective therapeutic strategy for cardiac repair.
In summary, cell cycle regulation is the key to cardiomyocyte proliferation, and future studies will explore its mechanism in depth through various strategies, such as gene therapy, metabolic intervention, cell transplantation and tissue engineering, and develop effective therapeutic means to promote myocardial regeneration and repair.
4 Regulators of cell cycle arrest in cardiomyocytes
Currently, an increasing number of studies in cardiac regeneration models have identified factors that inhibit cardiomyocyte proliferation by arresting cell cycle progression. These factors primarily include cell cycle proteins, coding and non-coding genes, as well as signaling pathways (Figure 3).
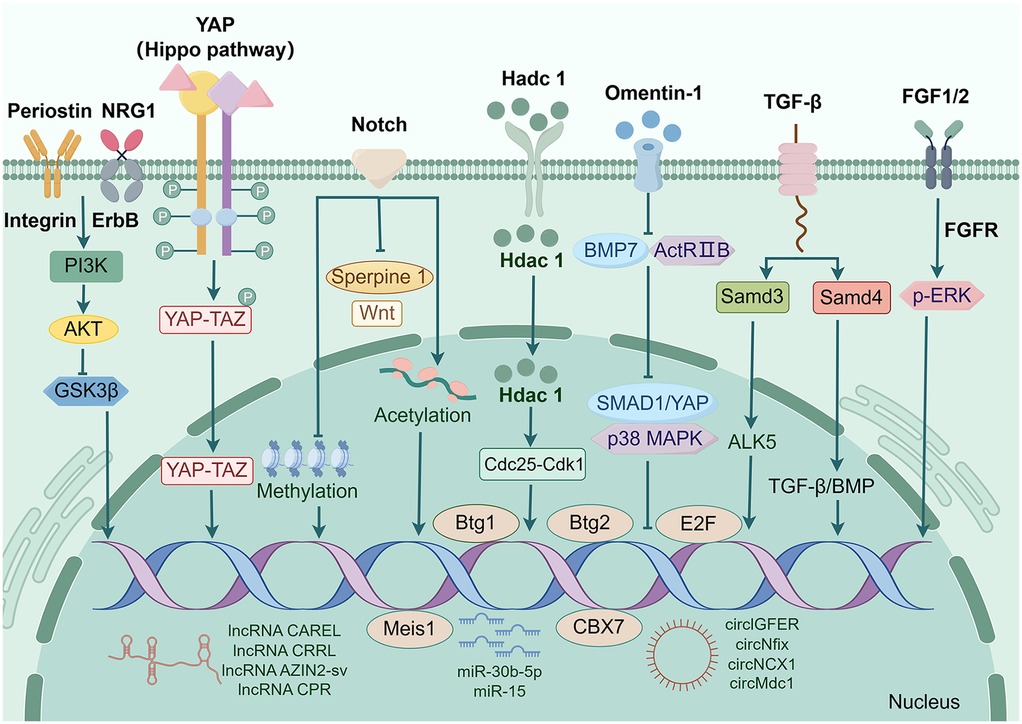
Figure 3. Factors that induce myocardial cell cycle arrest. Transcription factors including Btg1, Btg2, E2F, MEIS1, etc; Secretion factors including Hdac1, Omentin1, Periostin, NRG1, etc.; Signaling pathways including TGF-β pathway, Hippo pathway, Notch pathway, etc.; Non-coding genes including circGFER, circNCX1, miR-30b-5p, miR-15, lnc RNA CAREL, lnc RNACPR, etc.
4.1 Coding genes
In recent years, a multitude of coding genes that regulate the cell cycle in cardiac regeneration have been identified by researchers. These factors arrest the cardiomyocyte cell cycle through diverse pathways, thereby blocking both cardiomyocyte proliferation and cardiac regeneration (51).
Certain cell cycle regulators can suppress the proliferation of cardiomyocytes by directly binding to Cyclin or indirectly activating CDKs. For instance, the transcription factor E2F interacted with Cyclin to regulate both the proliferation and differentiation of cardiomyocytes (52). Deletion of E2F1, E2F2, and E2F3 has been shown to prevent cells from entering the S phase, thereby arresting the cell cycle of cardiomyocytes and inhibiting their capacity for proliferation (53). In 2013, Sadek et al. discovered that a transcription factor Meis1 played a crucial role in preventing heart muscle cells from dividing (54). Initially thought to act alone by Sadek's team during early studies where they knocked out its encoding gene in mice resulting in prolonged window for neonatal mouse cardiomyocyte division. However this prolongation was short-lived as these deficient cardiomyocytes eventually slowed down their division and ceased proliferating (54). Subsequently identified was Hoxb13 as a chaperone protein for Meis1; when both Meis1 and Hoxb13 were knocked out together in mice models it caused regression of cardiomyocytes back to an earlier developmental stage characterized by decreased size but increased number (54). Furthermore, they found that the CDK inhibitors p15, p16, and p21 are essential for transcriptional activation of Mesi1 as its transcriptional activation targets (55).
A study by Velayutham et al. revealed that the expression patterns of transcriptional co-regulators Btg1 and Btg2 during neonatal mouse cardiac development were consistent with the observed pattern of cell cycle of cardiomyocytes arrest. Btg1/2 constitutive double knockout cardiac tissues exhibited an increase in pH3+ mitotic cardiomyocytes at P7 but not at P30 (56). This finding indicates that suggest that Btg1 and Btg2 are novel factors involved in postnatal cell cycle of cardiomyocytes arrest. Zinc finger transcription factors are closely associated with cardiomyocyte proliferation. Mohammadi et al. demonstrated that specific knockdown of GATA4 in cardiomyocytes was found to reduce cardiomyocyte proliferation and myocardial angiogenesis in a rat model of cryoinjury, suggesting impaired cardiac regenerative capacity (57). CBX7 levels in the heart exhibit a continuous increase after birth and persist throughout adulthood. Overexpression of CBX7 in neonatal mouse cardiomyocytes has been shown to inhibit proliferation and promote multinucleation (58).
Secreted factors play important roles in cardiomyocyte cycle arrest and cardiac regeneration. Yang et al. identified omentin-1 as an adipokine that directly interacts with bone morphogenetic protein 7 (BMP7), regulating cardiomyocyte maturation. Omentin-1 prevents BMP7 from binding to activin type II receptor B (ActRIIB), subsequently inhibiting downstream signaling pathways involving DPP homolog 1 (SMAD1)/yes-associated protein (YAP) and p38 mitogen-activated protein kinase (p38 MAPK) (59). In another study, p38α negatively regulated adult zebrafish cardiomyocyte proliferation (60). Interestingly, Wu et al. showed that endogenous BMP signaling in zebrafish induced cell cycle arrest, thereby attenuating cardiomyocyte dedifferentiation and ultimately impairing myocardial regeneration (61). In a zebrafish regeneration model study, deficiency of histone deacetylase 1 (Hdac1) was found to affect the Cdc25-Cdk1 cell cycle axis, resulted in impaired progression through the G2/M phase and Cell cycle of cardiomyocytes arrest (62). Periostin, a secreted extracellular matrix protein, induces reentry into the cell cycle of already differentiated cardiomyocytes through the PI3K pathway, reduces post-infarction fibrosis, decreases infarct size, promotes angiogenesis and improves cardiac function (63). In addition, Chen et al. showed that Periostin mediates cardiomyocyte proliferation after myocardial infarction through PI3K/AKT/GSK3β signaling (64). NRG1, an epidermal growth factor, stimulates cardiomyocytes to re-enter the cell cycle and divide by activating downstream signaling pathways through its receptors ErbB2, ErbB3, and ErbB4 (65). However, although NRG1 transiently activates ErbB receptors to promote cardiomyocyte proliferation, it may inhibit overproliferation in adult myocardium through a negative feedback mechanism (66). Fibroblast growth factor FGF belongs to the family of secreted proteins. Studies have shown that activation of the FGF signaling pathway promotes cardiomyocyte proliferation and hypertrophy. For example, FGF10 regulates cell cycle reentry in cardiomyocytes by interacting with FGFR2b (embryonic stage) and FGFR1b (adult stage), thereby increasing ventricular wall thickness (67). However, chronic activation of the FGFR1 signaling pathway leads to cardiomyocyte hypertrophy and cardiac remodeling as evidenced by increased cardiomyocyte cross-sectional area, interstitial fibrosis, and disturbed myocyte alignment (68). In summary, secreted factors regulate cardiomyocyte cycle arrest through a complex network, a property that maintains cardiac homeostasis while limiting regenerative capacity. Future studies need to further resolve the spatiotemporal-specific roles of specific factors under pathological/physiological conditions to develop targeted intervention strategies.
Manipulating factors that induce cell cycle arrest during cardiac growth and development to influence cardiomyocyte proliferation, thereby promoting cardiac regeneration and repair, is anticipated as a prospective therapeutic strategy for cardiac restoration.
4.2 Non-coding genes
In recent years, an increasing number of evidence has demonstrated the involvement of noncoding RNAs (ncRNAs) in cardiac regeneration and their key roles in the pathogenesis of various CVDs. Specifically, circular RNAs (circRNAs) have emerged as crucial drivers of Cell cycle of cardiomyocytes arrest during adulthood. The Meis1-driven expression of circNfix exerts an inhibitory effect on cardiomyocyte proliferation. circNfix promoted the ubiquitin-dependent degradation of Y-box binding protein 1 (Ybx1), thereby suppressing the expression of Yap1 target genes Cyclin A2 and Cyclin B1, resulting in the inhibition of cardiomyocyte proliferation (69). Silencing of circMdc1 induced by oxidative stress allows reentry into the cell cycle of cardiomyocytes (70). Moreover, by competitively binding to proteins required for translation of its host gene, Mdc1, circMdc1 influenced oxidative DNA damage and controlled the window for cardiomyocyte cell cycle progression (70). In our previous work, we verified that circNCX1 plays a role in regulating cardiomyocyte proliferation by negatively regulating ubiquitination of the transcriptional activator BRG1 (71). The cyclic insulin circlGFIR interacted with miR-5-362p in the adult heart to inhibit cardiomyocyte proliferation, providing a crucial experimental basis for cardiac regeneration regulation (72). Long noncoding RNAs (lncRNAs) play a pivotal role in maintaining cellular homeostasis. Wang et al. discovered that the lncRNA CPR was an effective repressor of cardiomyocyte proliferation, offering a potential lncRNA-based therapeutic target for cardiac repair and regeneration (73). Furthermore, lncRNAs CAREL, CRRL, and AZIN2-sv have been shown to inhibit cardiomyocyte proliferation by interacting with micro RNAs (miRNAs) (74–76). MiRNAs are crucial regulators in cardiac development and involved in modulating various CVDs. In hearts of rats with MI, 85 differentially expressed miRNAs were identified, among which miR-30b-5p was down-regulated. Treatment of its inhibitor enabled reentry into the Cell cycle of cardiomyocytes while inhibiting cardiomyocyte apoptosis (77). Furthermore, cardiac-specific overexpression of miR-128 impaired cardiomyocyte proliferation and cardiac function, whereas miR-128 deletion inhibited p27 expression by enhancing SUZ12 expression, which further activated Cyclin E and CDK2 and contributed to cardiomyocyte cycle re-entry (78). The miR-15 family plays an important negative regulatory role in cardiomyocyte proliferation and cardiac regeneration. It has been shown that miR-15 family members (e.g., miR-195) are upregulated in the postnatal heart and promote cardiomyocyte proliferative arrest by repressing the expression of the cell cycle-associated gene Chek1 (79). chek1 plays a critical role in the G2/M phase transition and mitotic progression, and its expression is inhibited by the miR-15 family, leading to cardiomyocyte proliferation arrest (79). Meanwhile, the proliferative capacity of adult cardiomyocytes could be activated by inhibition of miR-15 family members and improved cardiac function in a myocardial infarction model (80). Thus, by inhibiting miR-15 family members, the proliferative capacity of cardiomyocytes can be activated, thus providing a new strategy for cardiac regeneration. These findings provide an important theoretical basis for the development of miRNA-based therapies for cardiac regeneration.
Collectively, these findings suggest that targeting ncRNA molecules holds great promise as a therapeutic strategy to promote cardiac repair processes and provides valuable insights for further investigations into the molecular mechanisms underlying cardiac regeneration.
4.3 Signaling pathways
4.3.1 Hippo-YAP signaling pathway
The Hippo signaling pathway plays a crucial role in regulating cardiomyocyte proliferation and cardiac regeneration (81). Heallen et al. demonstrated that Hippo signaling prevents postnatal re-entry of the cell cycle in cardiomyocytes, thereby inhibiting cardiomyocyte renewal and regeneration in adult mammals (82). YAP is highly activated in the heart before birth. However, its expression is suppressed after birth due to activation of Hippo signaling, consequently restraining cardiomyocyte proliferation (83). Consistent with this, overexpression of YAP during the postnatal period promotes the proliferation of postmitotic cardiomyocytes (83, 84). Collectively, these findings indicate that as cardiomyocytes progressively exit the cell cycle after birth, there is a substantial increase in Hippo signaling activity accompanied by a significant decrease in YAP activity. Therefore, targeting the Hippo-YAP signaling pathway has emerged as a promising strategy for achieving adult heart regeneration.
The role of the Hippo-YAP signaling pathway in cardiac injury has also been extensively investigated. Heallen et al. demonstrated that blockade of Hippo signaling through deletion of Sav1 or Lats1/2 facilitated cardiac regeneration in neonatal suckling mice subjected to AR and MI (82). However, depletion of Salvador, a key component of the Hippo pathway, in the hearts of mice with ischemic HF three weeks post-MI resulted in enhanced angiogenesis, reduced fibrosis, and restored cardiac function, ultimately promoting partial regeneration of the myocardium (85). These findings suggest that targeting the Hippo-YAP signaling pathway may reverse HF progression and provide therapeutic benefits for HF patients. Furthermore, adeno-associated virus subtype 9 (AAV9)-mediated overexpression of human YAP in the hearts of adult mice following MI significantly stimulated cardiomyocyte proliferation, improved cardiac function, and increased survival rates (86). Therefore, gene therapy utilizing AAV delivery vectors holds promise as a strategy for modifying outcomes after MI.
Our previous study demonstrated that YAP plays a crucial role in cardioprotection by targeting Parkin to suppress cardiotoxicity induced by the antitumor agent adriamycin (87). Furthermore, we confirmed the regulatory function of Parkin in cardiomyocyte necrosis (88). Based on these findings, we hypothesized Parkin may be involved in regulating cardiomyocyte cell cycle arrest through YAP signaling, thereby influencing cardiomyocyte proliferation and regeneration.
4.3.2 Notch signaling pathway
The Notch signaling pathway is a highly conserved signaling pathway that plays a critical role in cardiomyocyte proliferation and cardiac development (89). Early study on the zebrafish heart models revealed that msxB/C genes were significantly upregulated during cardiac regeneration, while the expression of Notch1 was notably increased prior to Msx activation, indicating early activation of the Notch pathway preceding zebrafish heart regeneration (90). Subsequent investigation focused on embryonic heart regeneration in zebrafish using a ventricle-specific gene ablation system. Notch signaling was activated in the atrial endocardium following ventricular ablation. Moreover, inhibition of Notch signaling blocked the conversion of atrial cardiomyocytes into ventricular cardiomyocytes, thereby affecting heart regeneration (11). In an adult zebrafish model of heart regeneration, Zhao et al. demonstrated that downregulation of Notch expression in the endocardium following ventricular amputation inhibited cardiomyocyte proliferation and triggered fibrosis. Upon injury to zebrafish hearts, Wnt signaling was activated, impairing cardiomyocyte proliferation and promoting scar formation. However, inhibition of the Wnt pathway partially restored the proliferation of Notch-suppressed cardiomyocytes in the endocardium (91), suggesting that Notch signaling promotes cardiomyocyte proliferation by inhibiting myocardial Wnt activity. Additionally, in a cryoinjury model of zebrafish hearts, it was observed that Serpine1, another endocardial molecule, exhibited strong expression during early stages after cryoinjury when Notch signaling was inhibited. However, its expression gradually decreased during later stages. These findings indicate that Notch signaling regulates endocardial maturation and cardiomyocyte proliferation by inhibiting Serpine1 (92).
In recent years, there has been an increasing attention on the investigation of Notch signaling in the mammalian heart. Collesi et al. were the first to observe a high expression level of Notch1 in immature ventricular cardiomyocytes, which decreased with age. Overexpression of Notch or activation of the Notch signaling pathway using the ligand Jagged1 significantly stimulated proliferative signaling and promoted cardiomyocyte proliferation in suckling mice (93). However, overexpressing Notch1 using adeno-associated viral vectors was largely ineffective in stimulating cardiac repair after MI in adult mice. Subsequent research revealed that DNA methylation modifications within promoter regions of genes targeted by Notch signaling in adult cardiomyocytes inhibited Notch activation (94). Additionally, acetylation modifications occurred in proliferating neonatal rat cardiomyocytes, thereby maintaining the stability of Notch signaling. Overexpression of Sirt1, a negative regulator of Notch1, reversed its acetylation level and decreased its stability, thus closing the proliferation window of cardiomyocytes. Viral vectors were utilized to enhance the expression of acetylation-modified Notch1, resulting in enhanced cardiac regenerative capacity following AR in suckling mice (95). Knockdown of Notch1 suppressed pathways involved in cardiomyocyte cell cycle progression and mitosis, leading to proliferation defects in early-stage human cardiomyocytes. Single-cell transcriptome analysis revealed that epicardial and second ventricular progenitor cells differentiated at the expense of first ventricular progenitor cells, suggesting that Notch1 inhibits second ventricular histiocyte proliferation under normal conditions (96).
4.3.3 TGF-β signaling pathway
The transforming growth factor-β (TGF-β) pathway plays a critical role in cardiac development and cardiomyocyte proliferation (97). Activation of TGF-β signaling primarily occurs through the Samd-dependent pathway. It has been demonstrated that the intracellular factor Smad4 mainly mediated TGF-β/BMP signaling. Specific knockdown of the Smad4 gene in the embryonic heart using the Cre-LoxP system resulted in a significant reduction in cardiomyocyte proliferation, severe defects in cardiac morphology, and stage-specific embryonic mortality. Furthermore, deletion of Smad4 affects the expression of TGF-β/BMP ligand, thereby disrupting its signaling cascade and ultimately impairing cardiac development while inhibiting embryonic cardiomyocyte proliferation (98). Smad3, a crucial marker of TGF-β signaling, induces the expression of TGF-β type I receptor ALK5 and initiates cardiomyocyte signaling in the injured region following cryoinjury of the zebrafish heart, thereby promoting cardiac regeneration. Inhibition of ALK5 using specific inhibitors reverses this process and completely impedes cardiac regeneration (99). Typically, TGF-β signaling activates ALK5 by recruiting type I receptors through type II receptors on the cell membrane for transphosphorylation. Moreover, in MI models, inhibition of ALK5 was found to attenuate Smad2 activation (100).
The activation of TGF-β signaling in cardiac regeneration can also be mediated by Samd-independent pathways. Although TGF-β can activate a wide range of signaling pathways, including the Rho/ROCK, p38 MAPK, and small GTPase pathways (101–104), little is currently known about the specific roles of these pathways in cardiac regeneration. Therefore, this represents an important future direction for research in cardiac regeneration.
In summary, regulators of cell cycle block in cardiomyocytes play a key role in cardiac regeneration. Future studies will deeply explore the regulatory mechanisms of cardiomyocyte proliferation through various strategies such as targeted therapy, gene editing, single-cell histology technology and improvement of the microenvironment, so as to provide new directions and methods for the development of cardiac regenerative medicine.
5 Mechanisms of metabolic regulation of cell cycle of cardiomyocytes blockade
Recently, changes in metabolic patterns during cardiac regeneration have become a hot research topic. In mammals, the fetal period is characterized by relative hypoxia, and cardiac energy is primarily derived from glycolysis (105–108). Seven days after birth, as cardiac energy demand increases, glycolysis gradually decreases and is replaced by a significant increase in fatty acid oxidation to maintain energy metabolism in cardiomyocytes (107). Consequently, this transition from glycolysis to oxidative metabolism leads to an elevation in mitochondrial ROS production and DDR, ultimately resulting in cell cycle arrest (5, 6). Collectively, alterations in oxygen levels at different developmental stages influence changes in the heart's energy source. Furthermore, modifications in metabolic patterns impact the state of the cardiomyocyte cell cycle and subsequently affect its proliferative capacity (Figure 4).
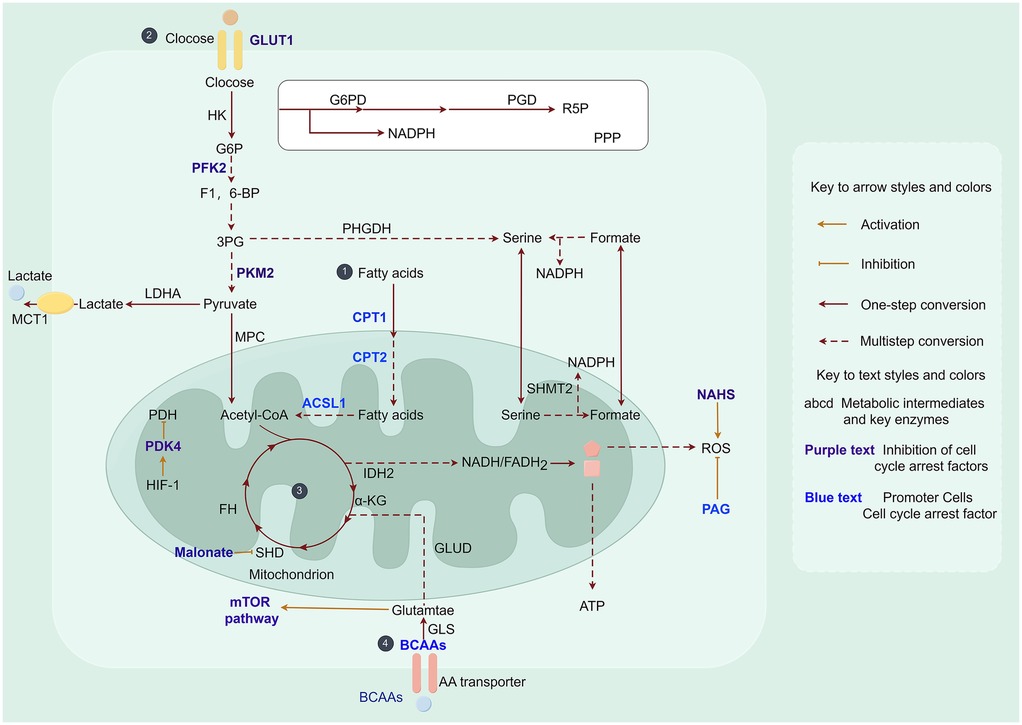
Figure 4. Metabolic pathways in myocardial cell cycle arrest. ① Fatty acid metabolism: ACSL1, acyl coenzyme A synthetase long chain family member 1; CPT, carnitine palmitoyltransferase. ② Glucose metabolism: GLUT, glucose transporter protein family; G6P, glucose-6-phosphate; PFK, phosphofructokinase; F1, 6-BP, 1, 6-bisphosphofructose; 3PG, 3-phosphoglycerate; PKM2, M2-pyruvate kinase; PDK, pyruvate dehydrogenase kinase; PDH, pyruvate dehydrogenase. ③ Tricarboxylic acid cycle: α-KG, α-ketoglutarate; SDH, succinate dehydrogenase; FH, fumarate hydratase; PAG, propylglycine; ROS, reactive oxygen species; NaHS, sodium bicarbonate. ④ Amino acid metabolism: BCAAs, branched-chain amino acids; mTOR, mammalian target of rapamycin. Acetyl-CoA is an intermediate product linking the three major nutrient metabolisms (fatty acid metabolism, glucose metabolism, and amino acid metabolism).
5.1 Fatty acid metabolism induces cardiomyocyte cell cycle arrest
The adult heart primarily derives its energy from the oxidation of fatty acids (109). Among all energy substrates, fatty acids produce the highest amount of ATP per 2 carbon units but also have the greatest oxygen demand. Consequently, fatty acids are considered the least metabolically efficient cardiac energy substrate in terms of total ATP produced/oxygen consumed (110).
Studies have shown that inhibition of fatty acids promotes cardiomyocyte proliferation and cardiac regeneration (111, 112). Neonatal mice fed with fatty acid-deficient milk exhibited an extended postnatal window for cardiomyocyte proliferation, but eventually progressed to cell cycle arrest (111). Carnitine palmitoyltransferase 1 (CPT1), a crucial enzyme in fatty acid oxidation, plays an indispensable role in regulating the metabolic switch from glycolysis to fatty acid oxidation. Li et al. demonstrated that inactivation of the b-type subunit of CPT1 (CPT1b) abolished fatty acid oxidation in cardiomyocytes, inducing a transition from a mature to an immature metabolic state and thereby reversing cardiomyocyte cell cycle arrest (112). Furthermore, etomoxir (ETO), an inhibitor of CPT1 activity, was found to suppress fatty acid oxidation and stimulate cardiomyocyte proliferation and cardiac regeneration in neonatal mice (113). Inhibition of carnitine palmitoyltransferase 2 (CPT2), another key enzyme in fatty acid oxidation, promoted cardiomyocyte proliferation in 2-week-old mice (114). Acyl coenzyme A synthetase long-chain family member 1 (ACSL1) is an essential regulator of lipid metabolism. Its expression level progressively increases after birth. Knockdown of ACSL1 significantly enhanced cardiac regeneration in primary mouse cardiomyocytes and efficiently restored cardiac function and muscle regeneration in adult mice following MI (115).
Phosphatidic acid plays a crucial role in lipid anabolism. Cao et al. unveiled the involvement of sphingolipid metabolism in cardiac regeneration, where SphK1 and SphK2, the two isozymes responsible for producing sphingosine-1-phosphate, exhibiting contrasting roles in repairing neonatal cardiac injury (116). Therefore, it is reasonable to speculate that ketoester metabolism also plays an indispensable role in regulating cardiac regeneration. Taken together, targeting fatty acid utilization in cardiomyocytes enhances proliferation and represents a promising therapeutic target for cardiac regenerative therapies (Table 1).
5.2 Glycolysis reverses cardiomyocyte cell cycle arrest
Glucose serves as a crucial energy source in the heart, where ATP production occurs through cytoplasmic glycolysis and mitochondrial oxidation of pyruvate derived from glycolysis. Glucose enters cardiomyocytes via glucose transporter protein 1 (GLUT1), where it is phosphorylated by hexokinase (HK) and subsequently participates in various metabolic processes. GLUT1 functions primarily as a glucose transporter protein in mammalian embryonic and neonatal hearts (117). Overexpression of GLUT1 in neonatal mouse hearts has been shown to enhance glycolytic efficiency (118) and facilitate cardiac regeneration following cryoinjury (119). These findings provide novel evidence supporting the notion that elevated expression of GLUT1 promotes cardiomyocyte proliferation and cardiac regeneration through enhanced glycolysis.
Key components of glycolysis are involved in the regulation of myocardial regeneration. Phosphofructokinase2 (PFK2), an essential enzyme in glycolysis, has been shown to enhance cardiomyocyte contractility when overexpressed in hypoxic mice (120), suggesting its potential cardioprotective function following cardiac injury. Pyruvate dehydrogenase kinase (PDK), another significant enzyme involved in glycolysis, exhibits significantly increased expression during cardiac development and further upregulation in adult hearts (121), consistent with the pattern of postnatal cardiomyocyte cell cycle arrest. Moreover, both zebrafish cryoinjury and mammalian MI models demonstrate substantial upregulation of PDK within the infarct zone (122, 123). Interestingly, overexpression of PDK3 promotes cardiomyocyte proliferation following zebrafish cardiac cryoinjury, but fails to influence scar repair (122). In contrast, cardiac-specific deletion of PDK4 facilitates cardiomyocyte proliferation and cardiac repair in the injured region after MI by enhancing glycolysis (124). Notably, as isoenzymes of PDK3 and PDK4 exhibit opposing roles in cardiac repair. This discrepancy may be attributed to their distinct distribution patterns, necessitating further investigation into the underlying mechanisms. The rate-limiting enzyme in the final step of glycolysis, pyruvate kinase muscle isoform 2 (PKM2), shows high expression levels in embryonic and neonatal mouse hearts. Overexpression of PKM2 promotes adult cardiomyocyte proliferation and myocardial regeneration by increasing glucose-6-phosphate dehydrogenase expression (125). Deletion of PKM2 inhibits glycolysis and reduces cardiomyocyte proliferation in zebrafish (126). Therefore, targeting specific glycolytic enzymes represents a promising therapeutic strategy for promoting cardiac repair and regeneration.
Collectively, these studies indicate that glycolysis plays a crucial role in regulating cardiomyocyte proliferation and cardiac regeneration following injury. Consequently, targeting glucose metabolism to reverse cardiomyocyte cell cycle arrest emerges as an efficacious strategy for promoting adult cardiac regeneration.
5.3 Tricarboxylic acid cycle metabolites regulate cardiomyocyte cell cycle arrest
The postnatal transition from glycolysis to fatty acid oxidation as the primary energy source in mammals is accompanied by a significant increase in mitochondrial abundance, which results in elevated levels of ROS during this period. The increased ROS levels subsequently induce DNA damage, leading to cell cycle arrest in cardiomyocytes (6). Therefore, understanding the role of mitochondrial metabolites in regulating this metabolic switch is crucial for developing research strategies focused on metabolic targeting for adult cardiac regeneration.
On one hand, inhibition of mitochondrial metabolism in cardiomyocytes leads to the reversal of cardiomyocyte cell cycle arrest. Succinate dehydrogenase (SDH) serves as a crucial link between the TAC and oxidative phosphorylation, playing a crucial role in cell cycle regulation and metabolic reprogramming (127). A study demonstrated that malonate-induced inhibition of SDH extended the window for cardiomyocyte proliferation in juvenile mice and promoted cardiomyocyte proliferation, hypertrophy, and cardiac regeneration in adult mice with MI. Notably, this inhibition was accompanied by an enhancement of glucose metabolism and a reduction in TAC cycle metabolism (128), consistent with the metabolic reprogramming process observed during the shift from oxidative phosphorylation to glycolysis in the adult heart, which facilitates cardiomyocyte proliferation and cardiac regeneration.
On the other hand, inhibition of oxidative pathways during mitochondrial metabolism leads to a reduction in ROS production. Martin et al. discovered that activation of antioxidant responses decreased ROS production and facilitated repair following cardiac injury (129). Sadek et al. reported that overexpression of mitochondrial peroxidase (mCAT) and the ROS scavenger, N-acetylcysteine (NAC) system, reduced DDR and promoted cardiomyocyte proliferation (6). Ge et al. demonstrated that Pitx2 deficiency failed to restore neonatal mouse hearts with AR due to the activation of genes associated with ROS scavenging by Pitx2 expression (129). Pei et al. demonstreated that the antioxidant hydrogen sulfide (H2S) eliminated ROS and reversed cardiomyocyte cell cycle arrest. Conversely, propylglycine (PAG), an inhibitor of H2S synthesis, was found to accumulate ROS, thereby suspending cardiomyocyte proliferation and cardiac regeneration in neonatal mice (130). In contrast, administration of NAD+ (NaHS), a hydrogen donor, attenuated H2S-mediated ROS accumulation and improved cardiac regeneration through enhanced cardiomyocyte proliferation and elimination of ROS after MI in P7 mice (130). These findings unveil a protective mechanism aimed at reducing mitochondrial oxidative stress-induced cardiomyocyte cell cycle arrest while providing a novel therapeutic strategy for HF.
The mitochondrial pyruvate carrier (MPC), located on the inner mitochondrial membrane, is responsible for transporting pyruvate from the cytoplasm to the mitochondrial matrix, where it participates in the tricarboxylic acid cycle, gluconeogenesis, and metabolism of lipids and amino acids to provide energy for the organism. The MPC plays a key role in the proliferation of cardiomyocytes and in the regulation of the cell cycle. Studies have shown that MPC1 deficiency leads to cardiomyocyte hypertrophy and heart failure, whereas MPC1 overexpression attenuates the hypertrophic response of cardiomyocytes (131). In addition, drugs modulating MPC activity may promote cardiomyocyte survival and functional recovery by improving energy metabolism and antioxidant capacity of cardiomyocytes. Currently, the MPC inhibitor UK5099 has been used to investigate its therapeutic potential in metabolic diseases (132), so could drugs modulating MPC activity further influence the cell cycle by regulating the metabolic level of cardiomyocytes, which could ultimately be applied in the clinical treatment of cardiovascular diseases such as MI?
Taken together, these studies provide compelling evidence for the crucial role of mitochondrial metabolites in modulating cardiac metabolism and highlight their promising therapeutic potential in facilitating adult cardiac regeneration (Table 1).
5.4 Amino acid oxidation promotes cardiomyocyte cell cycle arrest
Proteins play a pivotal role in the growth and maturation of cardiomyocytes, with enhanced protein synthesis leading to increased amino acid metabolism. To gain further insights into the involvement of amino acid oxidation in cardiac regeneration, studies have primarily focused on branched chain amino acids (BCAAs), namely leucine, isoleucine, and valine. BCAA levels exhibited dynamic fluctuations during mouse heart development. Specifically, valine, leucine, and isoleucine showed an increase during the postnatal phase, reaching a peak at day 9, followed by a decline in adulthood. Furthermore, most of the differentially expressed genes is directly associated with BCAA concentrations (133), indicating that amino acid metabolism plays an essential role in cardiomyocyte maturation. Therefore, we hypothesized that inhibiting enzymes involved in the catabolic pathway of BCAAs can enhance cardiomyocyte proliferation.
The mammalian target of rapamycin (mTOR) signaling pathway plays a critical role in cardiac development and growth (134, 135). Increased expression of glutamine, an amino acid transporter, in zebrafish and neonatal mouse cardiomyocytes activates the mTOR signaling pathway and regulates cardiomyocyte proliferation. Moreover, high concentrations of leucine and glutamine have been found to activate mTOR signaling and facilitate cardiac regeneration (136). Therefore, it is worth investigating whether the concentration of BCAAs also impacts the proliferative capacity of cardiomyocytes. Previous study has demonstrated that BCAAs activate the mTOR signaling pathway and induce metabolic reprogramming from fatty acid oxidative metabolism to glycolysis via HIF-1α (137), which is consistent with the metabolic reprogramming observed during cardiac regeneration. Conversely, inhibition of mTOR signaling blocks cardiac regeneration in zebrafish and promotes maturation of human pluripotent stem cell (iPSC)-derived cardiomyocytes (138, 139). Thus, it is evident that cardiomyocyte amino acid metabolism influences both cardiomyocyte proliferation and cardiac regeneration. However, further comprehensive investigations are warranted due to limited findings in this research area.
In summary, the blockage of metabolic regulatory mechanisms of the cardiomyocyte cycle represents an important research direction in cardiac regenerative medicine. Future studies will explore the regulatory mechanisms of cardiomyocyte regeneration in depth through metabolic intervention, epigenetic regulation, and multi-omics technology, thereby providing new strategies and methods for the treatment of cardiac diseases.
6 Drug development for cell cycle regulation and clinical treatment of CVDs
In recent years, despite significant advancements in the field of CVD treatment, there is an urgent need for novel therapeutic interventions. Among these potential options, targeting cell cycle regulation through drug development holds promise as a new strategy for CVD treatment. The following section provides a comprehensive summary of recent studies in drug discovery and clinical management of CVDs (Table 2), aiming to open new horizons in this field.
6.1 Metabolism-related drugs in CVD treatment
Myocardial energy metabolism plays a crucial role in regulating various pathological processes of CVDs, and interventions targeting myocardial energy metabolism hold promise as the next frontier for CVD prevention and treatment (140, 141).
Trimetazidine is used as an adjunct in the clinical treatment of HF to protect cardiomyocyte energy metabolism under hypoxic or ischemic conditions by reducing fatty acid beta oxidation and promoting glucose metabolism. In rat heart following I/R injury, trimetazidine has been shown to inhibit excessive glycolysis and enhance aerobic glucose oxidation (142). Consequently, trimetazidine exerts favorably influences the regulation of cardiomyocyte cell cycle through modulation of energy metabolism in the injured myocardium. Mitochondria, as the primary site of energy metabolism, have made the development of drugs targeting mitochondrial function regulation a major focus in the treatment of CVDs (143, 144). CoQ10, a naturally occurring compound in the human body closely associated with cardiac energy metabolism, exhibits notable antioxidant properties and effectively reduces the risk of cardiac morbidity (145). Nicotinamide adenine dinucleotide (NAD+), also known as coenzyme I, is a crucial central metabolite involved in redox reactions (146). Evidence from animal experiments has demonstrated that NAD+ levels are downregulated following cardiac I/R injury, and NAD+ supplementation reduces infarct size while reversing cardiomyocyte cell cycle arrest by regulating metabolic reprogramming (147–151). In recent years, NAD+ mediated myocardial metabolic disorders have been recognized as one of the pathogenic mechanisms underlying CVDs. Therefore, exploring the pharmacological role of NAD+ holds great promise as a therapeutic avenue.
Enhancing the energy supply through metabolism-related pathways plays a beneficial and complementary role in CVD treatment. Meanwhile, ensuring the judicious and rational utilization of myocardial metabolism-targeted drugs to maintain the homeostasis of energy metabolism represents a crucial next step in CVD drug discovery and development.
6.2 NcRNA drugs for the treatment of CVDs
As ongoing research progresses, an increasing number of evidence indicates that ncRNAs play a crucial role as regulatory molecules in modulating the cardiomyocyte cell cycle (152). In recent years, the development of multiple RNA-based therapies has further accelerated advancements in ncRNA-targeted drug discovery and development.
MiRNAs have emerged as promising targets for novel CVD-targeted therapeutics due to their short length, strong conservation, and high stability (153, 154). Currently, CDR132l, an antisense oligonucleotide drug targeting miR-132, has become the first therapeutic drug for HF and entered clinical trials. In a porcine model of HF after MI, CDR132l demonstrated significant improvements in HF severity, restoration of cardiomyocyte function, and reversal of cardiomyocyte cell cycle arrest within a reasonable dosage range (155). MRG-110, an antisense oligonucleotide drug for the treatment of cardiovascular diseases, promotes the growth of new blood vessels by inhibiting miR-92a, thereby exerting a therapeutic effect in accelerating wound healing (156). However, its clinical studies related to CVDs remain limited. Collectively, miRNA-based drugs hold promising prospects for treating MI and promoting myocardial cell cycle recovery following ischemic injury, thus representing novel biological targets worthy of further exploration in CVD treatment.
In comparison to miRNAs, lncRNAs and circRNAs have received relatively less attention in the field of CVD drug discovery and development owing to their limited in vivo stability, larger molecular size, and propensity to induce an immune response. Therefore, overcoming these challenges represents the subsequent crucial step for drug developers.
In summary, cell cycle regulation and metabolic regulation are important in the treatment of cardiovascular diseases. Future research will develop more effective and safer therapeutic drugs and methods through targeted therapy, nucleic acid-based therapeutic strategies, metabolic interventions, and clinical translation, providing new hope for the treatment of cardiovascular diseases.
7 Conclusion
Nowadays, CVD has become the leading cause of mortality worldwide, posing a severe threat to human life and health. Therefore, there is an urgent need for effective therapeutic strategies for CVD. Currently, the primary means of treating CVD in clinical practice is interventional therapy, which fails to achieve the renewal and regeneration of cardiomyocytes. The limited proliferative capacity of mature cardiomyocytes can be attributed to their terminal differentiation status and subsequent cell cycle arrest. Therefore, promoting cardiac regeneration by modulating cell cycle factors may represent a key strategy for curing cardiomyopathy in the future. In this review, we summarize the current state of research on myocardial regeneration and the mechanisms underlying cardiomyocyte cell cycle arrest, including recent findings and ongoing controversies.
The entry of cardiomyocytes into the cell cycle for division and proliferation represents the primary step in cardiac regeneration. To clearly delineate the critical role of cell cycle-related factors in cardiac regeneration, we mapped the regulators of cell cycle arrest before and after the proliferation window of cardiomyocytes (Figure 3). These regulators include both coding and noncoding genes, and as well as signaling pathways that regulate cardiomyocyte cell cycle exit or re-entry mainly by targeting cyclin-dependent factors. Notably, these regulators function independently, but instead form a complex network that orchestrates cell cycle homeostasis. Given this complexity, further elucidating the mechanisms of these pathways during homeostasis, disease, and regeneration in vivo is a crucial step toward targeting these pathways for therapeutic development.
The regulation of the cell cycle by metabolic reprogramming has been extensively studied in the context of tumor cells, while studies focusing on cardiomyocytes, which exhibit limited proliferative capacity, remain underexplored but hold immense potential. In this review, we summarize the current state of research on how myocardial metabolism regulates cell cycle arrest (Table 1). On this basis, modulating relevant metabolic pathways to promote cardiomyocyte cell cycle re-entry, proliferation, and regeneration may eventually evolve into an effective treatment for CVDs. However, several key questions require further investigation. For example, metabolic changes influence cardiomyocyte cell cycle progression; what are the underlying mechanisms by which these metabolic alterations ultimately affect cardiomyocyte proliferation and cardiac regeneration? Additionally, both cell cycle factors and metabolic pathways are involved in cardiac regeneration; are their interactions critical to cardiac regeneration?
In the clinical management of CVDs, drug therapy for cardiomyopathy has guided the direction of clinical research. Drugs such as trimetazidine, ranolazine, and CDR132l have been investigated in clinical trials for their ameliorative effects on cardiomyopathy (Table 2). In basic research, some of these drugs target cardiomyocyte cell cycle processes to exert their functions. For instance, malic acid in cardiac basal fluid exhibits antioxidant activity, scavenges ROS, and regulates cardiomyocyte cell cycle re-entry (157); trimetazidine affects cardiomyocyte cell cycle progression by regulating energy metabolism levels (142, 158–160). Therefore, it is essential to harness the pharmacological potential of validated pathways as drug candidates for inducing cardiac regeneration in adults. However, further exploration is required to determine the the population, side effects, and optimal dosage of therapeutic agents for cardiomyopathy (161).
So far, several key questions remain unresolved. It is well established that vascular endothelial growth factor (VEGF) binds to its receptor to promote vascular regeneration (162). However, the nascent vascular endothelium formed after MI has been shown to contribute to cardiomyocyte proliferation (163). It remains unclear whether VEGF, a well-characterized therapeutic factor for MI (164), can directly modulate cardiomyocyte cell cycle progression. Cardiac fibroblasts, which constitute the major cellular component of the heart, have previously been demonstrated to reprogrammed into cardiomyocytes through the action of specific transcription factors (165). In addition, cardiomyocytes can interact with endothelial cells and immune cells to influence the cell cycle and thus their proliferative potential (93, 166). However, lymphocytes also play an important role in cardiac inflammation and immune responses. So, do cardiomyocytes interact with lymphocytes to regulate cardiomyocyte proliferation? Stem cell therapy would be a potential cardioprotective strategy after MI (167). Previous studies have demonstrated that stem cells can act as immunomodulators and promote myocardial regeneration through anti-inflammatory effects (168, 169). However, the ability of stem cell therapies to reprogram cardiomyocyte cell cycle progression through the interaction of other cellular components (fibroblasts, endothelial cells, and platelet-derived growth factors, among others) remains to be further explored.
Currently, some progress has been made in the clinical treatment of myocardial regeneration. Canseco et al. found that cardiac load reduction by left ventricular assist device (LVAD) can induce the proliferation of cardiomyocytes (170). In addition, positive progress has been made in cell therapy trials, providing an important reference for the development of cardiac regenerative medicine (170). However, research on cardiomyocyte proliferation and regeneration still faces many challenges. For example, most of the current studies are in the stage of animal experiments, and the safety and efficacy of some of the technologies in human beings have yet to be further verified. In addition, the mechanism of cardiomyocyte proliferation and regeneration is complex and involves multiple cell types and signaling pathways, so how to accurately regulate the proliferation and regeneration of cardiomyocytes is still an urgent problem to be solved.
Despite some basic science advances in cardiac regeneration research, a number of controversies and questions remain. There is wide controversy in the academic community regarding the existence and function of cardiac stem cells. Early studies suggested that cardiac stem cells (e.g., c-Kit+ cells, Sca-1+ cells, etc.) have significant regenerative potential, but recent findings suggest that these cells have a very limited ability to differentiate into cardiomyocytes in vivo (171). Therefore, further studies are needed to investigate whether cardiac stem cells exist and the specific mechanisms of their role in cardiac regeneration. In addition, current studies have not fully elucidated whether cardiomyocyte regeneration is mainly dependent on the dedifferentiation of cardiomyocytes or on the reactivation of progenitor/stem cells in the heart, which is still controversial (170). Therefore, researchers need to further investigate the specific mechanisms of cardiomyocyte regeneration and how to promote endogenous cardiomyocyte regeneration by regulating cell cycle and metabolic pathways. Overall, the field of cardiac regeneration has made remarkable scientific progress, but there are still many problems, limitations, and research gaps. Future research needs to bridge the gap between basic science and clinical applications to provide a more solid theoretical foundation and feasible clinical protocols for cardiac regenerative therapies.
In this review, we systematically sort out the critical role of the cell cycle in cardiomyocyte proliferation and cardiac regeneration, discuss the precise regulation of the cell cycle by cell cycle regulators and metabolism, and further review the development and clinical applications of cell cycle-related drugs for cardiovascular diseases. This information will contribute to the treatment of cardiovascular diseases and provide promising directions for future research in the treatment of cardiovascular diseases, which will facilitate the exploration and utilization of new therapeutic strategies. In conclusion, we are optimistic that impressive progress in the direction of human cardiac regeneration is possible.
Author contributions
QX: Conceptualization, Writing – original draft. XC: Data curation, Writing – original draft. CZ: Data curation, Writing – original draft. YL: Data curation, Writing – review & editing. JW: Data curation, Writing – review & editing. XA: Conceptualization, Validation, Visualization, Writing – review & editing. WD: Conceptualization, Visualization, Writing – review & editing.
Funding
The author(s) declare that financial support was received for the research and/or publication of this article. This work was supported by National Natural Science Foundation of China (82270301), Natural Science Foundation of Shandong Province (ZR2019ZD28 and ZR2023MH299).
Conflict of interest
The authors declare that the research was conducted in the absence of any commercial or financial relationships that could be construed as a potential conflict of interest.
Generative AI statement
The author(s) declare that no Generative AI was used in the creation of this manuscript.
Publisher's note
All claims expressed in this article are solely those of the authors and do not necessarily represent those of their affiliated organizations, or those of the publisher, the editors and the reviewers. Any product that may be evaluated in this article, or claim that may be made by its manufacturer, is not guaranteed or endorsed by the publisher.
References
1. Roth GA, Mensah GA, Johnson CO, Addolorato G, Ammirati E, Baddour LM, et al. Global burden of cardiovascular diseases and risk factors, 1990–2019: update from the GBD 2019 study. J Am Coll Cardiol. (2020) 76:2982–3021. doi: 10.1016/j.jacc.2020.11.010
2. Bergmann O, Bhardwaj RD, Bernard S, Zdunek S, Barnabe-Heider F, Walsh S, et al. Evidence for cardiomyocyte renewal in humans. Science. (2009) 324:98–102. doi: 10.1126/science.1164680
4. Porrello ER, Mahmoud AI, Simpson E, Hill JA, Richardson JA, Olson EN, et al. Transient regenerative potential of the neonatal mouse heart. Science. (2011) 331:1078–80. doi: 10.1126/science.1200708
5. Girard J, Ferre P, Pegorier JP, Duee PH. Adaptations of glucose and fatty acid metabolism during perinatal period and suckling-weaning transition. Physiol Rev. (1992) 72:507–62. doi: 10.1152/physrev.1992.72.2.507
6. Puente BN, Kimura W, Muralidhar SA, Moon J, Amatruda JF, Phelps KL, et al. The oxygen-rich postnatal environment induces cardiomyocyte cell-cycle arrest through DNA damage response. Cell. (2014) 157:565–79. doi: 10.1016/j.cell.2014.03.032
7. Zhao Q, Sun Q, Zhou L, Liu K, Jiao K. Complex regulation of mitochondrial function during cardiac development. J Am Heart Assoc. (2019) 8:e012731. doi: 10.1161/JAHA.119.012731
8. Vasudevarao MD, Posadas Pena D, Ihle M, Bongiovanni C, Maity P, Geissler D, et al. BMP signaling promotes zebrafish heart regeneration via alleviation of replication stress. Nat Commun. (2025) 16:1708. doi: 10.1038/s41467-025-56993-6
9. Jopling C, Sleep E, Raya M, Marti M, Raya A, Izpisua Belmonte JC. Zebrafish heart regeneration occurs by cardiomyocyte dedifferentiation and proliferation. Nature. (2010) 464:606–9. doi: 10.1038/nature08899
10. Kikuchi K, Holdway JE, Werdich AA, Anderson RM, Fang Y, Egnaczyk GF, et al. Primary contribution to zebrafish heart regeneration by gata4(+) cardiomyocytes. Nature. (2010) 464:601–5. doi: 10.1038/nature08804
11. Zhang R, Han P, Yang H, Ouyang K, Lee D, Lin YF, et al. In vivo cardiac reprogramming contributes to zebrafish heart regeneration. Nature. (2013) 498:497–501. doi: 10.1038/nature12322
12. Poss KD, Wilson LG, Keating MT. Heart regeneration in zebrafish. Science. (2002) 298:2188–90. doi: 10.1126/science.1077857
13. Bouwman M, de Bakker DEM, Honkoop H, Giovou AE, Versteeg D, Boender AR, et al. Cross-species comparison reveals that Hmga1 reduces H3K27me3 levels to promote cardiomyocyte proliferation and cardiac regeneration. Nat Cardiovasc Res. (2025) 4:64–82. doi: 10.1038/s44161-024-00588-9
14. Koudstaal S, Jansen Of Lorkeers SJ, Gaetani R, Gho JM, van Slochteren FJ, Sluijter JP, et al. Concise review: heart regeneration and the role of cardiac stem cells. Stem Cells Transl Med. (2013) 2:434–43. doi: 10.5966/sctm.2013-0001
15. Chablais F, Veit J, Rainer G, Jazwinska A. The zebrafish heart regenerates after cryoinjury-induced myocardial infarction. BMC Dev Biol. (2011) 11:21. doi: 10.1186/1471-213X-11-21
16. Gonzalez-Rosa JM, Martin V, Peralta M, Torres M, Mercader N. Extensive scar formation and regression during heart regeneration after cryoinjury in zebrafish. Development. (2011) 138:1663–74. doi: 10.1242/dev.060897
17. Schnabel K, Wu CC, Kurth T, Weidinger G. Regeneration of cryoinjury induced necrotic heart lesions in zebrafish is associated with epicardial activation and cardiomyocyte proliferation. PLoS One. (2011) 6:e18503. doi: 10.1371/journal.pone.0018503
18. Wang J, Panakova D, Kikuchi K, Holdway JE, Gemberling M, Burris JS, et al. The regenerative capacity of zebrafish reverses cardiac failure caused by genetic cardiomyocyte depletion. Development. (2011) 138:3421–30. doi: 10.1242/dev.068601
19. Becker RO, Chapin S, Sherry R. Regeneration of the ventricular myocardium in amphibians. Nature. (1974) 248:145–7. doi: 10.1038/248145a0
20. Flink IL. Cell cycle reentry of ventricular and atrial cardiomyocytes and cells within the epicardium following amputation of the ventricular apex in the axolotl, amblystoma mexicanum: confocal microscopic immunofluorescent image analysis of bromodeoxyuridine-labeled nuclei. Anat Embryol (Berl). (2002) 205:235–44. doi: 10.1007/s00429-002-0249-6
21. Oberpriller JO, Oberpriller JC. Response of the adult newt ventricle to injury. J Exp Zool. (1974) 187:249–53. doi: 10.1002/jez.1401870208
22. Rumyantsev PP. Post-injury DNA synthesis, mitosis and ultrastructural reorganization of adult frog cardiac myocytes. An electron microscopic-autoradiographic study. Z Zellforsch Mikrosk Anat. (1973) 139:431–50. doi: 10.1007/BF00306596
23. Uygur A, Lee RT. Mechanisms of cardiac regeneration. Dev Cell. (2016) 36:362–74. doi: 10.1016/j.devcel.2016.01.018
24. Weinberger M, Riley PR. Animal models to study cardiac regeneration. Nat Rev Cardiol. (2024) 21:89–105. doi: 10.1038/s41569-023-00914-x
25. Secco I, Giacca M. Regulation of endogenous cardiomyocyte proliferation: the known unknowns. J Mol Cell Cardiol. (2023) 179:80–9. doi: 10.1016/j.yjmcc.2023.04.001
26. Patterson M, Barske L, Van Handel B, Rau CD, Gan P, Sharma A, et al. Frequency of mononuclear diploid cardiomyocytes underlies natural variation in heart regeneration. Nat Genet. (2017) 49:1346–53. doi: 10.1038/ng.3929
27. Jonker SS, Zhang L, Louey S, Giraud GD, Thornburg KL, Faber JJ. Myocyte enlargement, differentiation, and proliferation kinetics in the fetal sheep heart. J Appl Physiol (1985). (2007) 102:1130–42. doi: 10.1152/japplphysiol.00937.2006
28. Soonpaa MH, Kim KK, Pajak L, Franklin M, Field LJ. Cardiomyocyte DNA synthesis and binucleation during murine development. Am J Physiol. (1996) 271:H2183-9. doi: 10.1152/ajpheart.1996.271.5.H2183
29. Alkass K, Panula J, Westman M, Wu TD, Guerquin-Kern JL, Bergmann O. No evidence for cardiomyocyte number expansion in preadolescent mice. Cell. (2015) 163:1026–36. doi: 10.1016/j.cell.2015.10.035
30. Kikuchi K, Poss KD. Cardiac regenerative capacity and mechanisms. Annu Rev Cell Dev Biol. (2012) 28:719–41. doi: 10.1146/annurev-cellbio-101011-155739
31. Porrello ER, Mahmoud AI, Simpson E, Johnson BA, Grinsfelder D, Canseco D, et al. Regulation of neonatal and adult mammalian heart regeneration by the miR-15 family. Proc Natl Acad Sci U S A. (2013) 110:187–92. doi: 10.1073/pnas.1208863110
33. Fratz S, Hager A, Schreiber C, Schwaiger M, Hess J, Stern HC. Long-term myocardial scarring after operation for anomalous left coronary artery from the pulmonary artery. Ann Thorac Surg. (2011) 92:1761–5. doi: 10.1016/j.athoracsur.2011.06.021
34. Haubner BJ, Schneider J, Schweigmann U, Schuetz T, Dichtl W, Velik-Salchner C, et al. Functional recovery of a human neonatal heart after severe myocardial infarction. Circ Res. (2016) 118:216–21. doi: 10.1161/CIRCRESAHA.115.307017
35. Schafer KA. The cell cycle: a review. Vet Pathol. (1998) 35:461–78. doi: 10.1177/030098589803500601
36. Palacios-Blanco I, Martin-Castellanos C. Cyclins and CDKs in the regulation of meiosis-specific events. Front Cell Dev Biol. (2022) 10:1069064. doi: 10.3389/fcell.2022.1069064
37. Kaplon J, van Dam L, Peeper D. Two-way communication between the metabolic and cell cycle machineries: the molecular basis. Cell Cycle. (2015) 14:2022–32. doi: 10.1080/15384101.2015.1044172
38. Moncada S, Higgs EA, Colombo SL. Fulfilling the metabolic requirements for cell proliferation. Biochem J. (2012) 446:1–7. doi: 10.1042/BJ20120427
39. Kalucka J, Missiaen R, Georgiadou M, Schoors S, Lange C, De Bock K, et al. Metabolic control of the cell cycle. Cell Cycle. (2015) 14:3379–88. doi: 10.1080/15384101.2015.1090068
40. Sicinski P, Donaher JL, Parker SB, Li T, Fazeli A, Gardner H, et al. Cyclin D1 provides a link between development and oncogenesis in the retina and breast. Cell. (1995) 82:621–30. doi: 10.1016/0092-8674(95)90034-9
41. Rane SG, Dubus P, Mettus RV, Galbreath EJ, Boden G, Reddy EP, et al. Loss of Cdk4 expression causes insulin-deficient diabetes and Cdk4 activation results in beta-islet cell hyperplasia. Nat Genet. (1999) 22:44–52. doi: 10.1038/8751
42. Hochegger H, Takeda S, Hunt T. Cyclin-dependent kinases and cell-cycle transitions: does one fit all? Nat Rev Mol Cell Biol. (2008) 9:910–6. doi: 10.1038/nrm2510
43. Busk PK, Bartkova J, Strom CC, Wulf-Andersen L, Hinrichsen R, Christoffersen TE, et al. Involvement of cyclin D activity in left ventricle hypertrophy in vivo and in vitro. Cardiovasc Res. (2002) 56:64–75. doi: 10.1016/s0008-6363(02)00510-2
45. Tane S, Ikenishi A, Okayama H, Iwamoto N, Nakayama KI, Takeuchi T. CDK inhibitors, p21(Cip1) and p27(Kip1), participate in cell cycle exit of mammalian cardiomyocytes. Biochem Biophys Res Commun. (2014) 443:1105–9. doi: 10.1016/j.bbrc.2013.12.109
46. Chaudhry HW, Dashoush NH, Tang H, Zhang L, Wang X, Wu EX, et al. Cyclin A2 mediates cardiomyocyte mitosis in the postmitotic myocardium. J Biol Chem. (2004) 279:35858–66. doi: 10.1074/jbc.M404975200
47. Mohamed TMA, Ang YS, Radzinsky E, Zhou P, Huang Y, Elfenbein A, et al. Regulation of cell cycle to stimulate adult cardiomyocyte proliferation and cardiac regeneration. Cell. (2018) 173:104–16.e12. doi: 10.1016/j.cell.2018.02.014
48. Tane S, Okayama H, Ikenishi A, Amemiya Y, Nakayama KI, Takeuchi T. Two inhibitory systems and CKIs regulate cell cycle exit of mammalian cardiomyocytes after birth. Biochem Biophys Res Commun. (2015) 466:147–54. doi: 10.1016/j.bbrc.2015.08.102
49. Borghesan M, Hoogaars WMH, Varela-Eirin M, Talma N, Demaria M. A senescence-centric view of aging: implications for longevity and disease. Trends Cell Biol. (2020) 30:777–91. doi: 10.1016/j.tcb.2020.07.002
50. Delint-Ramirez I, Madabhushi R. DNA damage and its links to neuronal aging and degeneration. Neuron. (2025) 113:7–28. doi: 10.1016/j.neuron.2024.12.001
51. Gong R, Jiang Z, Zagidullin N, Liu T, Cai B. Regulation of cardiomyocyte fate plasticity: a key strategy for cardiac regeneration. Signal Transduct Target Ther. (2021) 6:31. doi: 10.1038/s41392-020-00413-2
52. Ahuja P, Sdek P, MacLellan WR. Cardiac myocyte cell cycle control in development, disease, and regeneration. Physiol Rev. (2007) 87:521–44. doi: 10.1152/physrev.00032.2006
53. Wu L, Timmers C, Maiti B, Saavedra HI, Sang L, Chong GT, et al. The E2F1-3 transcription factors are essential for cellular proliferation. Nature. (2001) 414:457–62. doi: 10.1038/35106593
54. Mahmoud AI, Kocabas F, Muralidhar SA, Kimura W, Koura AS, Thet S, et al. Meis1 regulates postnatal cardiomyocyte cell cycle arrest. Nature. (2013) 497:249–53. doi: 10.1038/nature12054
55. Hamada H, Nakanishi T, Markwald RR, Baldwin HS, Keller BB, Srivastava D, et al. Erratum to: etiology and morphogenesis of congenital heart disease. In Etiology and Morphogenesis of Congenital Heart Disease: From Gene Function and Cellular Interaction to Morphology. Tokyo: Springer Press (2018). p. E1. doi: 10.1007/978-4-431-54628-3_55
56. Velayutham N, Calderon MU, Alfieri CM, Padula SL, van Leeuwen FN, Scheijen B, et al. Btg1 and Btg2 regulate neonatal cardiomyocyte cell cycle arrest. J Mol Cell Cardiol. (2023) 179:30–41. doi: 10.1016/j.yjmcc.2023.03.016
57. Malek Mohammadi M, Kattih B, Grund A, Froese N, Korf-Klingebiel M, Gigina A, et al. The transcription factor GATA4 promotes myocardial regeneration in neonatal mice. EMBO Mol Med. (2017) 9:265–79. doi: 10.15252/emmm.201606602
58. Cho KW, Andrade M, Bae S, Kim S, Kim E, Jang J, et al. Polycomb group protein CBX7 represses cardiomyocyte proliferation through modulation of the TARDBP/RBM38 axis. Circulation. (2023) 147:1823–42. doi: 10.1161/CIRCULATIONAHA.122.061131
59. Yang H, Song S, Li J, Li Y, Feng J, Sun Q, et al. Omentin-1 drives cardiomyocyte cell cycle arrest and metabolic maturation by interacting with BMP7. Cell Mol Life Sci. (2023) 80:186. doi: 10.1007/s00018-023-04829-1
60. Jopling C, Sune G, Morera C, Izpisua Belmonte JC. P38alpha MAPK regulates myocardial regeneration in zebrafish. Cell Cycle. (2012) 11:1195–201. doi: 10.4161/cc.11.6.19637
61. Wu CC, Kruse F, Vasudevarao MD, Junker JP, Zebrowski DC, Fischer K, et al. Spatially resolved genome-wide transcriptional profiling identifies BMP signaling as essential regulator of zebrafish cardiomyocyte regeneration. Dev Cell. (2016) 36:36–49. doi: 10.1016/j.devcel.2015.12.010
62. Boos A, Gahr BM, Park DD, Braun V, Buhler A, Rottbauer W, et al. Hdac1-deficiency affects the cell cycle axis Cdc25-Cdk1 causing impaired G2/M phase progression and reduced cardiomyocyte proliferation in zebrafish. Biochem Biophys Res Commun. (2023) 665:98–106. doi: 10.1016/j.bbrc.2023.04.116
63. Kuhn B, del Monte F, Hajjar RJ, Chang YS, Lebeche D, Arab S, et al. Periostin induces proliferation of differentiated cardiomyocytes and promotes cardiac repair. Nat Med. (2007) 13:962–9. doi: 10.1038/nm1619
64. Chen Z, Xie J, Hao H, Lin H, Wang L, Zhang Y, et al. Ablation of periostin inhibits post-infarction myocardial regeneration in neonatal mice mediated by the phosphatidylinositol 3 kinase/glycogen synthase kinase 3beta/cyclin D1 signalling pathway. Cardiovasc Res. (2017) 113:620–32. doi: 10.1093/cvr/cvx001
65. Wang Y, Wei J, Zhang P, Zhang X, Wang Y, Chen W, et al. Neuregulin-1, a potential therapeutic target for cardiac repair. Front Pharmacol. (2022) 13:945206. doi: 10.3389/fphar.2022.945206
66. Wadugu B, Kuhn B. The role of neuregulin/ErbB2/ErbB4 signaling in the heart with special focus on effects on cardiomyocyte proliferation. Am J Physiol Heart Circ Physiol. (2012) 302:H2139–47. doi: 10.1152/ajpheart.00063.2012
67. Khosravi F, Ahmadvand N, Bellusci S, Sauer H. The multifunctional contribution of FGF signaling to cardiac development, homeostasis, disease and repair. Front Cell Dev Biol. (2021) 9:672935. doi: 10.3389/fcell.2021.672935
68. Cilvik SN, Wang JI, Lavine KJ, Uchida K, Castro A, Gierasch CM, et al. Fibroblast growth factor receptor 1 signaling in adult cardiomyocytes increases contractility and results in a hypertrophic cardiomyopathy. PLoS One. (2013) 8:e82979. doi: 10.1371/journal.pone.0082979
69. Huang S, Li X, Zheng H, Si X, Li B, Wei G, et al. Loss of super-enhancer-regulated circRNA nfix induces cardiac regeneration after myocardial infarction in adult mice. Circulation. (2019) 139:2857–76. doi: 10.1161/CIRCULATIONAHA.118.038361
70. Ma W, Wang X, Sun H, Xu B, Song R, Tian Y, et al. Oxidant stress-sensitive circRNA Mdc1 controls cardiomyocyte chromosome stability and cell cycle re-entry during heart regeneration. Pharmacol Res. (2022) 184:106422. doi: 10.1016/j.phrs.2022.106422
71. Yijian L, Weihan S, Lin Y, Heng Z, Yu W, Lin S, et al. CircNCX1 modulates cardiomyocyte proliferation through promoting ubiquitination of BRG1. Cell Signal. (2024) 120:111193. doi: 10.1016/j.cellsig.2024.111193
72. Zeng JH, Li HJ, Liu K, Liang CQ, Wu HY, Chen WY, et al. Loss of circIGF1R suppresses cardiomyocytes proliferation by sponging miR-362-5p. DNA Cell Biol. (2023) 42:399–410. doi: 10.1089/dna.2022.0590
73. Ponnusamy M, Liu F, Zhang YH, Li RB, Zhai M, Liu F, et al. Long noncoding RNA CPR (cardiomyocyte proliferation regulator) regulates cardiomyocyte proliferation and cardiac repair. Circulation. (2019) 139:2668–84. doi: 10.1161/CIRCULATIONAHA.118.035832
74. Cai B, Ma W, Ding F, Zhang L, Huang Q, Wang X, et al. The long noncoding RNA CAREL controls cardiac regeneration. J Am Coll Cardiol. (2018) 72:534–50. doi: 10.1016/j.jacc.2018.04.085
75. Chen G, Li H, Li X, Li B, Zhong L, Huang S, et al. Loss of long non-coding RNA CRRL promotes cardiomyocyte regeneration and improves cardiac repair by functioning as a competing endogenous RNA. J Mol Cell Cardiol. (2018) 122:152–64. doi: 10.1016/j.yjmcc.2018.08.013
76. Li X, He X, Wang H, Li M, Huang S, Chen G, et al. Loss of AZIN2 splice variant facilitates endogenous cardiac regeneration. Cardiovasc Res. (2018) 114:1642–55. doi: 10.1093/cvr/cvy075
77. Chi F, Feng L, Li Y, Zhao S, Yuan W, Jiang Y, et al. MiR-30b-5p promotes myocardial cell apoptosis in rats with myocardial infarction through regulating wnt/beta-catenin signaling pathway. Minerva Med. (2023) 114:476–84. doi: 10.23736/S0026-4806.20.06565-9
78. Huang W, Feng Y, Liang J, Yu H, Wang C, Wang B, et al. Loss of microRNA-128 promotes cardiomyocyte proliferation and heart regeneration. Nat Commun. (2018) 9:700. doi: 10.1038/s41467-018-03019-z
79. Porrello ER, Johnson BA, Aurora AB, Simpson E, Nam YJ, Matkovich SJ, et al. MiR-15 family regulates postnatal mitotic arrest of cardiomyocytes. Circ Res. (2011) 109:670–9. doi: 10.1161/CIRCRESAHA.111.248880
80. Zheng M, Wang M. A narrative review of the roles of the miR-15/107 family in heart disease: lessons and prospects for heart disease. Ann Transl Med. (2021) 9:66. doi: 10.21037/atm-20-6073
81. Wang J, Liu S, Heallen T, Martin JF. The hippo pathway in the heart: pivotal roles in development, disease, and regeneration. Nat Rev Cardiol. (2018) 15:672–84. doi: 10.1038/s41569-018-0063-3
82. Heallen T, Morikawa Y, Leach J, Tao G, Willerson JT, Johnson RL, et al. Hippo signaling impedes adult heart regeneration. Development. (2013) 140:4683–90. doi: 10.1242/dev.102798
83. von Gise A, Lin Z, Schlegelmilch K, Honor LB, Pan GM, Buck JN, et al. YAP1, the nuclear target of hippo signaling, stimulates heart growth through cardiomyocyte proliferation but not hypertrophy. Proc Natl Acad Sci U S A. (2012) 109:2394–9. doi: 10.1073/pnas.1116136109
84. Xin M, Kim Y, Sutherland LB, Qi X, McAnally J, Schwartz RJ, et al. Regulation of insulin-like growth factor signaling by yap governs cardiomyocyte proliferation and embryonic heart size. Sci Signal. (2011) 4:ra70. doi: 10.1126/scisignal.2002278
85. Leach JP, Heallen T, Zhang M, Rahmani M, Morikawa Y, Hill MC, et al. Hippo pathway deficiency reverses systolic heart failure after infarction. Nature. (2017) 550:260–4. doi: 10.1038/nature24045
86. Lin Z, von Gise A, Zhou P, Gu F, Ma Q, Jiang J, et al. Cardiac-specific YAP activation improves cardiac function and survival in an experimental murine MI model. Circ Res. (2014) 115:354–63. doi: 10.1161/CIRCRESAHA.115.303632
87. Xiao D, Chang W, Ding W, Wang Y, Fa H, Wang J. Enhanced mitophagy mediated by the YAP/parkin pathway protects against DOX-induced cardiotoxicity. Toxicol Lett. (2020) 330:96–107. doi: 10.1016/j.toxlet.2020.05.015
88. Sun T, Ding W, Xu T, Ao X, Yu T, Li M, et al. Parkin regulates programmed necrosis and myocardial ischemia/reperfusion injury by targeting cyclophilin-D. Antioxid Redox Signal. (2019) 31:1177–93. doi: 10.1089/ars.2019.7734
89. Bray SJ. Notch signalling in context. Nat Rev Mol Cell Biol. (2016) 17:722–35. doi: 10.1038/nrm.2016.94
90. Raya A, Koth CM, Buscher D, Kawakami Y, Itoh T, Raya RM, et al. Activation of notch signaling pathway precedes heart regeneration in zebrafish. Proc Natl Acad Sci U S A. (2003) 100(Suppl 1):11889–95. doi: 10.1073/pnas.1834204100
91. Zhao L, Ben-Yair R, Burns CE, Burns CG. Endocardial notch signaling promotes cardiomyocyte proliferation in the regenerating zebrafish heart through wnt pathway antagonism. Cell Rep. (2019) 26:546–54.e5. doi: 10.1016/j.celrep.2018.12.048
92. Munch J, Grivas D, Gonzalez-Rajal A, Torregrosa-Carrion R, de la Pompa JL. Notch signalling restricts inflammation and serpine1 expression in the dynamic endocardium of the regenerating zebrafish heart. Development. (2017) 144:1425–40. doi: 10.1242/dev.143362
93. Collesi C, Zentilin L, Sinagra G, Giacca M. Notch1 signaling stimulates proliferation of immature cardiomyocytes. J Cell Biol. (2008) 183:117–28. doi: 10.1083/jcb.200806091
94. Felician G, Collesi C, Lusic M, Martinelli V, Ferro MD, Zentilin L, et al. Epigenetic modification at notch responsive promoters blunts efficacy of inducing notch pathway reactivation after myocardial infarction. Circ Res. (2014) 115:636–49. doi: 10.1161/CIRCRESAHA.115.304517
95. Collesi C, Felician G, Secco I, Gutierrez MI, Martelletti E, Ali H, et al. Reversible Notch1 acetylation tunes proliferative signalling in cardiomyocytes. Cardiovasc Res. (2018) 114:103–22. doi: 10.1093/cvr/cvx228
96. Ye S, Wang C, Xu Z, Lin H, Wan X, Yu Y, et al. Impaired human cardiac cell development due to NOTCH1 deficiency. Circ Res. (2023) 132:187–204. doi: 10.1161/CIRCRESAHA.122.321398
97. Sorensen DW, van Berlo JH. The role of TGF-beta signaling in cardiomyocyte proliferation. Curr Heart Fail Rep. (2020) 17:225–33. doi: 10.1007/s11897-020-00470-2
98. Qi X, Yang G, Yang L, Lan Y, Weng T, Wang J, et al. Essential role of Smad4 in maintaining cardiomyocyte proliferation during murine embryonic heart development. Dev Biol. (2007) 311:136–46. doi: 10.1016/j.ydbio.2007.08.022
99. Chablais F, Jazwinska A. The regenerative capacity of the zebrafish heart is dependent on TGFbeta signaling. Development. (2012) 139:1921–30. doi: 10.1242/dev.078543
100. Tan SM, Zhang Y, Connelly KA, Gilbert RE, Kelly DJ. Targeted inhibition of activin receptor-like kinase 5 signaling attenuates cardiac dysfunction following myocardial infarction. Am J Physiol Heart Circ Physiol. (2010) 298:H1415–25. doi: 10.1152/ajpheart.01048.2009
101. Li Q, Xu Y, Li X, Guo Y, Liu G. Inhibition of rho-kinase ameliorates myocardial remodeling and fibrosis in pressure overload and myocardial infarction: role of TGF-beta1-TAK1. Toxicol Lett. (2012) 211:91–7. doi: 10.1016/j.toxlet.2012.03.006
102. Wang S, Sun A, Li L, Zhao G, Jia J, Wang K, et al. Up-regulation of BMP-2 antagonizes TGF-beta1/ROCK-enhanced cardiac fibrotic signalling through activation of Smurf1/Smad6 complex. J Cell Mol Med. (2012) 16:2301–10. doi: 10.1111/j.1582-4934.2012.01538.x
103. Matsumoto-Ida M, Takimoto Y, Aoyama T, Akao M, Takeda T, Kita T. Activation of TGF-beta1-TAK1-p38 MAPK pathway in spared cardiomyocytes is involved in left ventricular remodeling after myocardial infarction in rats. Am J Physiol Heart Circ Physiol. (2006) 290:H709–15. doi: 10.1152/ajpheart.00186.2005
104. Derynck R, Zhang YE. Smad-dependent and smad-independent pathways in TGF-beta family signalling. Nature. (2003) 425:577–84. doi: 10.1038/nature02006
105. Dawes GS, Mott JC, Widdicombe JG. The circulation of blood in the foetal lamb. J Physiol. (1954) 126:38P. doi: 10.1113/jphysiol.1954.sp005227
106. Lopaschuk GD, Spafford MA. Differences in myocardial ischemic tolerance between 1- and 7-day-old rabbits. Can J Physiol Pharmacol. (1992) 70:1315–23. doi: 10.1139/y92-184
107. Lopaschuk GD, Spafford MA, Marsh DR. Glycolysis is predominant source of myocardial ATP production immediately after birth. Am J Physiol. (1991) 261:H1698–705. doi: 10.1152/ajpheart.1991.261.6.H1698
108. Werner JC, Sicard RE. Lactate metabolism of isolated, perfused fetal, and newborn pig hearts. Pediatr Res. (1987) 22:552–6. doi: 10.1203/00006450-198711000-00016
109. Isu G, Robles Diaz D, Grussenmeyer T, Gaudiello E, Eckstein F, Brink M, et al. Fatty acid-based monolayer culture to promote in vitro neonatal rat cardiomyocyte maturation. Biochim Biophys Acta Mol Cell Res. (2020) 1867:118561. doi: 10.1016/j.bbamcr.2019.118561
110. Doenst T, Nguyen TD, Abel ED. Cardiac metabolism in heart failure: implications beyond ATP production. Circ Res. (2013) 113:709–24. doi: 10.1161/CIRCRESAHA.113.300376
111. Cardoso AC, Lam NT, Savla JJ, Nakada Y, Pereira AHM, Elnwasany A, et al. Mitochondrial substrate utilization regulates cardiomyocyte cell cycle progression. Nat Metab. (2020) 2:167–78. doi: 10.1038/s42255-020-0169-x
112. Li X, Wu F, Gunther S, Looso M, Kuenne C, Zhang T, et al. Inhibition of fatty acid oxidation enables heart regeneration in adult mice. Nature. (2023) 622:619–26. doi: 10.1038/s41586-023-06585-5
113. Cao T, Liccardo D, LaCanna R, Zhang X, Lu R, Finck BN, et al. Fatty acid oxidation promotes cardiomyocyte proliferation rate but does not change cardiomyocyte number in infant mice. Front Cell Dev Biol. (2019) 7:42. doi: 10.3389/fcell.2019.00042
114. Hirose K, Payumo AY, Cutie S, Hoang A, Zhang H, Guyot R, et al. Evidence for hormonal control of heart regenerative capacity during endothermy acquisition. Science. (2019) 364:184–8. doi: 10.1126/science.aar2038
115. Li Y, Yang M, Tan J, Shen C, Deng S, Fu X, et al. Targeting ACSL1 promotes cardiomyocyte proliferation and cardiac regeneration. Life Sci. (2022) 294:120371. doi: 10.1016/j.lfs.2022.120371
116. Ji X, Chen Z, Wang Q, Li B, Wei Y, Li Y, et al. Sphingolipid metabolism controls mammalian heart regeneration. Cell Metab. (2024) 36:839–56.e8. doi: 10.1016/j.cmet.2024.01.017
117. Shao D, Tian R. Glucose transporters in cardiac metabolism and hypertrophy. Compr Physiol. (2015) 6:331–51. doi: 10.1002/cphy.c150016
118. Liao R, Jain M, Cui L, D'Agostino J, Aiello F, Luptak I, et al. Cardiac-specific overexpression of GLUT1 prevents the development of heart failure attributable to pressure overload in mice. Circulation. (2002) 106:2125–31. doi: 10.1161/01.cir.0000034049.61181.f3
119. Fajardo VM, Feng I, Chen BY, Perez-Ramirez CA, Shi B, Clark P, et al. GLUT1 overexpression enhances glucose metabolism and promotes neonatal heart regeneration. Sci Rep. (2021) 11:8669. doi: 10.1038/s41598-021-88159-x
120. Wang Q, Donthi RV, Wang J, Lange AJ, Watson LJ, Jones SP, et al. Cardiac phosphatase-deficient 6-phosphofructo-2-kinase/fructose-2, 6-bisphosphatase increases glycolysis, hypertrophy, and myocyte resistance to hypoxia. Am J Physiol Heart Circ Physiol. (2008) 294:H2889–97. doi: 10.1152/ajpheart.91501.2007
121. Sugden MC, Langdown ML, Harris RA, Holness MJ. Expression and regulation of pyruvate dehydrogenase kinase isoforms in the developing rat heart and in adulthood: role of thyroid hormone status and lipid supply. Biochem J. (2000) 352(Pt 3):731–8. doi: 10.1042/bj3520731
122. Wilson JE. Isozymes of mammalian hexokinase: structure, subcellular localization and metabolic function. J Exp Biol. (2003) 206:2049–57. doi: 10.1242/jeb.00241
123. McCommis KS, Douglas DL, Krenz M, Baines CP. Cardiac-specific hexokinase 2 overexpression attenuates hypertrophy by increasing pentose phosphate pathway flux. J Am Heart Assoc. (2013) 2:e000355. doi: 10.1161/JAHA.113.000355
124. Pereira RO, Wende AR, Olsen C, Soto J, Rawlings T, Zhu Y, et al. Inducible overexpression of GLUT1 prevents mitochondrial dysfunction and attenuates structural remodeling in pressure overload but does not prevent left ventricular dysfunction. J Am Heart Assoc. (2013) 2:e000301. doi: 10.1161/JAHA.113.000301
125. Magadum A, Singh N, Kurian AA, Munir I, Mehmood T, Brown K, et al. Pkm2 regulates cardiomyocyte cell cycle and promotes cardiac regeneration. Circulation. (2020) 141:1249–65. doi: 10.1161/CIRCULATIONAHA.119.043067
126. Fukuda R, Marin-Juez R, El-Sammak H, Beisaw A, Ramadass R, Kuenne C, et al. Stimulation of glycolysis promotes cardiomyocyte proliferation after injury in adult zebrafish. EMBO Rep. (2020) 21:e49752. doi: 10.15252/embr.201949752
127. Martinez-Reyes I, Chandel NS. Mitochondrial TCA cycle metabolites control physiology and disease. Nat Commun. (2020) 11:102. doi: 10.1038/s41467-019-13668-3
128. Bae J, Salamon RJ, Brandt EB, Paltzer WG, Zhang Z, Britt EC, et al. Malonate promotes adult cardiomyocyte proliferation and heart regeneration. Circulation. (2021) 143:1973–86. doi: 10.1161/CIRCULATIONAHA.120.049952
129. Tao G, Kahr PC, Morikawa Y, Zhang M, Rahmani M, Heallen TR, et al. Pitx2 promotes heart repair by activating the antioxidant response after cardiac injury. Nature. (2016) 534:119–23. doi: 10.1038/nature17959
130. Pei J, Wang F, Pei S, Bai R, Cong X, Nie Y, et al. Hydrogen sulfide promotes cardiomyocyte proliferation and heart regeneration via ROS scavenging. Oxid Med Cell Longev. (2020) 2020:1412696. doi: 10.1155/2020/1412696
131. Cluntun AA, Badolia R, Lettlova S, Parnell KM, Shankar TS, Diakos NA, et al. The pyruvate-lactate axis modulates cardiac hypertrophy and heart failure. Cell Metab. (2021) 33:629–48.e10. doi: 10.1016/j.cmet.2020.12.003
132. He Z, Zhang J, Xu Y, Fine EJ, Suomivuori CM, Dror RO, et al. Structure of mitochondrial pyruvate carrier and its inhibition mechanism. Nature. (2025):1–8. doi: 10.1038/s41586-025-08667-y
133. Talman V, Teppo J, Poho P, Movahedi P, Vaikkinen A, Karhu ST, et al. Molecular atlas of postnatal mouse heart development. J Am Heart Assoc. (2018) 7:e010378. doi: 10.1161/JAHA.118.010378
134. Mazelin L, Panthu B, Nicot AS, Belotti E, Tintignac L, Teixeira G, et al. mTOR inactivation in myocardium from infant mice rapidly leads to dilated cardiomyopathy due to translation defects and p53/JNK-mediated apoptosis. J Mol Cell Cardiol. (2016) 97:213–25. doi: 10.1016/j.yjmcc.2016.04.011
135. Zhang P, Shan T, Liang X, Deng C, Kuang S. Mammalian target of rapamycin is essential for cardiomyocyte survival and heart development in mice. Biochem Biophys Res Commun. (2014) 452:53–9. doi: 10.1016/j.bbrc.2014.08.046
136. Xu X, Lu Z, Fassett J, Zhang P, Hu X, Liu X, et al. Metformin protects against systolic overload-induced heart failure independent of AMP-activated protein kinase alpha2. Hypertension. (2014) 63:723–8. doi: 10.1161/HYPERTENSIONAHA.113.02619
137. Hu J, Nie Y, Chen S, Xie C, Fan Q, Wang Z, et al. Leucine reduces reactive oxygen species levels via an energy metabolism switch by activation of the mTOR-HIF-1alpha pathway in porcine intestinal epithelial cells. Int J Biochem Cell Biol. (2017) 89:42–56. doi: 10.1016/j.biocel.2017.05.026
138. Chavez MN, Morales RA, Lopez-Crisosto C, Roa JC, Allende ML, Lavandero S. Autophagy activation in zebrafish heart regeneration. Sci Rep. (2020) 10:2191. doi: 10.1038/s41598-020-59106-z
139. Garbern JC, Helman A, Sereda R, Sarikhani M, Ahmed A, Escalante GO, et al. Inhibition of mTOR signaling enhances maturation of cardiomyocytes derived from human-induced pluripotent stem cells via p53-induced quiescence. Circulation. (2020) 141:285–300. doi: 10.1161/CIRCULATIONAHA.119.044205
140. Ajith TA, Jayakumar TG. Peroxisome proliferator-activated receptors in cardiac energy metabolism and cardiovascular disease. Clin Exp Pharmacol Physiol. (2016) 43:649–58. doi: 10.1111/1440-1681.12579
141. Chen S, Zou Y, Song C, Cao K, Cai K, Wu Y, et al. The role of glycolytic metabolic pathways in cardiovascular disease and potential therapeutic approaches. Basic Res Cardiol. (2023) 118:48. doi: 10.1007/s00395-023-01018-w
142. Kukes VG, Zhernakova NI, Gorbach TV, Romashchenko OV, Rumbesht VV. Efficiency of trimetazidine treatment of experimental ischemic heart disease in age aspect. Eksp Klin Farmakol. (2013) 76:9–12.23631276
143. Honka H, Solis-Herrera C, Triplitt C, Norton L, Butler J, DeFronzo RA. Therapeutic manipulation of myocardial metabolism: JACC state-of-the-art review. J Am Coll Cardiol. (2021) 77:2022–39. doi: 10.1016/j.jacc.2021.02.057
144. Ardehali H, Sabbah HN, Burke MA, Sarma S, Liu PP, Cleland JG, et al. Targeting myocardial substrate metabolism in heart failure: potential for new therapies. Eur J Heart Fail. (2012) 14:120–9. doi: 10.1093/eurjhf/hfr173
145. Singh U, Devaraj S, Jialal I. Coenzyme Q10 supplementation and heart failure. Nutr Rev. (2007) 65:286–93. doi: 10.1301/nr.2007.jun.286-293
146. Zapata-Perez R, Wanders RJA, van Karnebeek CDM, Houtkooper RH. NAD(+) homeostasis in human health and disease. EMBO Mol Med. (2021) 13:e13943. doi: 10.15252/emmm.202113943
147. Sukoyan GV, Kavadze IK. Effect of nadcin on energy supply system and apoptosis in ischemia-reperfusion injury to the myocardium. Bull Exp Biol Med. (2008) 146:321–4. doi: 10.1007/s10517-008-0268-2
148. Sukoyan GV, Andriadze NA, Guchua EI, Karsanov NV. Effect of NAD on recovery of adenine nucleotide pool, phosphorylation potential, and stimulation of apoptosis during late period of reperfusion damage to myocardium. Bull Exp Biol Med. (2005) 139:46–9. doi: 10.1007/s10517-005-0208-3
149. Liaudet L, Yang Z, Al-Affar EB, Szabo C. Myocardial ischemic preconditioning in rodents is dependent on poly (ADP-ribose) synthetase. Mol Med. (2001) 7:406–17. doi: 10.1007/BF03402187
150. Zhai X, Han W, Wang M, Guan S, Qu X. Exogenous supplemental NAD+ protect myocardium against myocardial ischemic/reperfusion injury in swine model. Am J Transl Res. (2019) 11:6066–74.31632574
151. Liu L, Wang Q, Zhao B, Wu Q, Wang P. Exogenous nicotinamide adenine dinucleotide administration alleviates ischemia/reperfusion-induced oxidative injury in isolated rat hearts via Sirt5-SDH-succinate pathway. Eur J Pharmacol. (2019) 858:172520. doi: 10.1016/j.ejphar.2019.172520
152. van der Ven CFT, Hogewoning BCR, van Mil A, Sluijter JPG. Non-coding RNAs in cardiac regeneration. Adv Exp Med Biol. (2020) 1229:163–80. doi: 10.1007/978-981-15-1671-9_9
153. Afify AY. A miRNA’s insight into the regenerating heart: a concise descriptive analysis. Heart Fail Rev. (2020) 25:1047–61. doi: 10.1007/s10741-019-09896-w
154. Wojciechowska A, Braniewska A, Kozar-Kaminska K. MicroRNA in cardiovascular biology and disease. Adv Clin Exp Med. (2017) 26:865–74. doi: 10.17219/acem/62915
155. Foinquinos A, Batkai S, Genschel C, Viereck J, Rump S, Gyongyosi M, et al. Preclinical development of a miR-132 inhibitor for heart failure treatment. Nat Commun. (2020) 11:633. doi: 10.1038/s41467-020-14349-2
156. Gallant-Behm CL, Piper J, Dickinson BA, Dalby CM, Pestano LA, Jackson AL. A synthetic microRNA-92a inhibitor (MRG-110) accelerates angiogenesis and wound healing in diabetic and nondiabetic wounds. Wound Repair Regen. (2018) 26:311–23. doi: 10.1111/wrr.12660
157. Wu JL, Wu QP, Yang XF, Wei MK, Zhang JM, Huang Q, et al. L-malate reverses oxidative stress and antioxidative defenses in liver and heart of aged rats. Physiol Res. (2008) 57:261–8. doi: 10.33549/physiolres.931161
158. Adamo L, Nassif ME, Novak E, LaRue SJ, Mann DL. Prevalence of lactic acidaemia in patients with advanced heart failure and depressed cardiac output. Eur J Heart Fail. (2017) 19:1027–33. doi: 10.1002/ejhf.628
159. Swietach P, Youm JB, Saegusa N, Leem CH, Spitzer KW, Vaughan-Jones RD. Coupled Ca2+/H+ transport by cytoplasmic buffers regulates local Ca2+ and H+ ion signaling. Proc Natl Acad Sci U S A. (2013) 110:E2064–73. doi: 10.1073/pnas.1222433110
160. Fang YH, Piao L, Hong Z, Toth PT, Marsboom G, Bache-Wiig P, et al. Therapeutic inhibition of fatty acid oxidation in right ventricular hypertrophy: exploiting Randle’s cycle. J Mol Med (Berl). (2012) 90:31–43. doi: 10.1007/s00109-011-0804-9
161. Dominguez-Rodriguez A, Abreu-Gonzalez P, de la Torre-Hernandez JM, Gonzalez-Gonzalez J, Garcia-Camarero T, Consuegra-Sanchez L, et al. Effect of intravenous and intracoronary melatonin as an adjunct to primary percutaneous coronary intervention for acute ST-elevation myocardial infarction: results of the melatonin adjunct in the acute myocaRdial infarction treated with angioplasty trial. J Pineal Res. (2017) 62(1):e12374. doi: 10.1111/jpi.12374
162. Apte RS, Chen DS, Ferrara N. VEGF In signaling and disease: beyond discovery and development. Cell. (2019) 176:1248–64. doi: 10.1016/j.cell.2019.01.021
163. Li M, Wu Y, Ye L. The role of amino acids in endothelial biology and function. Cells. (2022) 11(8):1372. doi: 10.3390/cells11081372
164. Kukula K, Urbanowicz A, Klopotowski M, Dabrowski M, Pregowski J, Kadziela J, et al. Long-term follow-up and safety assessment of angiogenic gene therapy trial VIF-CAD: transcatheter intramyocardial administration of a bicistronic plasmid expressing VEGF-A165/bFGF cDNA for the treatment of refractory coronary artery disease. Am Heart J. (2019) 215:78–82. doi: 10.1016/j.ahj.2019.06.009
165. Ieda M, Fu JD, Delgado-Olguin P, Vedantham V, Hayashi Y, Bruneau BG, et al. Direct reprogramming of fibroblasts into functional cardiomyocytes by defined factors. Cell. (2010) 142:375–86. doi: 10.1016/j.cell.2010.07.002
166. Aurora AB, Porrello ER, Tan W, Mahmoud AI, Hill JA, Bassel-Duby R, et al. Macrophages are required for neonatal heart regeneration. J Clin Invest. (2014) 124:1382–92. doi: 10.1172/JCI72181
167. Grajek S, Popiel M, Gil L, Breborowicz P, Lesiak M, Czepczynski R, et al. Influence of bone marrow stem cells on left ventricle perfusion and ejection fraction in patients with acute myocardial infarction of anterior wall: randomized clinical trial: impact of bone marrow stem cell intracoronary infusion on improvement of microcirculation. Eur Heart J. (2010) 31:691–702. doi: 10.1093/eurheartj/ehp536
168. Zhang Y, Mignone J, MacLellan WR. Cardiac regeneration and stem cells. Physiol Rev. (2015) 95:1189–204. doi: 10.1152/physrev.00021.2014
169. Vadivel S, Vincent P, Sekaran S, Visaga Ambi S, Muralidar S, Selvaraj V, et al. Inflammation in myocardial injury- stem cells as potential immunomodulators for myocardial regeneration and restoration. Life Sci. (2020) 250:117582. doi: 10.1016/j.lfs.2020.117582
170. Bois A, Grandela C, Gallant J, Mummery C, Menasche P. Revitalizing the heart: strategies and tools for cardiomyocyte regeneration post-myocardial infarction. NPJ Regen Med. (2025) 10:6. doi: 10.1038/s41536-025-00394-2
171. Chen X, Zhong X, Huang GN. Heart regeneration from the whole-organism perspective to single-cell resolution. NPJ Regen Med. (2024) 9:34. doi: 10.1038/s41536-024-00378-8
172. Teunissen BE, Smeets PJ, Willemsen PH, De Windt LJ, Van der Vusse GJ, Van Bilsen M. Activation of PPARdelta inhibits cardiac fibroblast proliferation and the transdifferentiation into myofibroblasts. Cardiovasc Res. (2007) 75:519–29. doi: 10.1016/j.cardiores.2007.04.026
173. Magadum A, Ding Y, He L, Kim T, Vasudevarao MD, Long Q, et al. Live cell screening platform identifies PPARdelta as a regulator of cardiomyocyte proliferation and cardiac repair. Cell Res. (2017) 27:1002–19. doi: 10.1038/cr.2017.84
174. Schulze PC, Drosatos K, Goldberg IJ. Lipid use and misuse by the heart. Circ Res. (2016) 118:1736–51. doi: 10.1161/CIRCRESAHA.116.306842
175. Kourampi I, Katsioupa M, Oikonomou E, Tsigkou V, Marinos G, Goliopoulou A, et al. The role of ranolazine in heart failure-current concepts. Am J Cardiol. (2023) 209:92–103. doi: 10.1016/j.amjcard.2023.09.066
176. Wang ZY, Liu YY, Liu GH, Lu HB, Mao CY. l-Carnitine and heart disease. Life Sci. (2018) 194:88–97. doi: 10.1016/j.lfs.2017.12.015
177. Sundell J, Knuuti J. Insulin and myocardial blood flow. Cardiovasc Res. (2003) 57:312–9. doi: 10.1016/s0008-6363(02)00718-6
178. Ivanov AV, Gorodetskaya EA, Kalenikova EI, Medvedev OS. Single intravenous injection of coenzyme Q10 protects the myocardium after irreversible ischemia. Bull Exp Biol Med. (2013) 155:771–4. doi: 10.1007/s10517-013-2249-3
Keywords: cardiomyocyte, proliferation, cardiac regeneration, cell cycle arrest, cardiovascular disease
Citation: Xu Q, Chen X, Zhao C, Liu Y, Wang J, Ao X and Ding W (2025) Cell cycle arrest of cardiomyocytes in the context of cardiac regeneration. Front. Cardiovasc. Med. 12:1538546. doi: 10.3389/fcvm.2025.1538546
Received: 3 December 2024; Accepted: 14 April 2025;
Published: 28 April 2025.
Edited by:
Kay-Dietrich Wagner, University of Nice Sophia Antipolis, FranceReviewed by:
Shafeeq Ahmed Mohammed, University Hospital Zürich, SwitzerlandVaibhav Deshmukh, Washington University in St. Louis, United States
Copyright: © 2025 Xu, Chen, Zhao, Liu, Wang, Ao and Ding. This is an open-access article distributed under the terms of the Creative Commons Attribution License (CC BY). The use, distribution or reproduction in other forums is permitted, provided the original author(s) and the copyright owner(s) are credited and that the original publication in this journal is cited, in accordance with accepted academic practice. No use, distribution or reproduction is permitted which does not comply with these terms.
*Correspondence: Xiang Ao, eGlhbmdhbzIwMTZAMTYzLmNvbQ==; Wei Ding, ZGluZ3dlaUBxZHUuZWR1LmNu