- 1National Dental Centre, Singapore, Singapore
- 2Faculty of Dentistry, National University of Singapore, Singapore, Singapore
- 3College of Pharmacy, Shenzhen Technology University, Shenzhen, China
- 4Department of Pharmacy, Faculty of Science, National University of Singapore, Singapore, Singapore
Periodontitis is a common chronic inflammatory disease driven by the interaction between a dysbiotic oral microbiome and the dysregulated host immune-inflammatory response. Naturally derived nutraceuticals, such as resveratrol and its analogs, are potential adjunctive therapies in periodontal treatment due to their antimicrobial and anti-inflammatory properties. Furthermore, different analogs of resveratrol and the choice of solvents used may lead to varying effects on therapeutic properties. This review presents the current findings and gaps in our understanding on the potential utility of resveratrol and its analogs in periodontal treatment.
Introduction
Advanced periodontitis is the sixth most prevalent health condition in the world (1). It affects the supporting tissues of the teeth, and disease consequences include tooth loss and impairment of daily functional activities, leading to reduced quality of life (2). Patients suffering from periodontitis are also at higher risk for systemic diseases such as diabetes (3) and cardiovascular disease (4).
The subgingival plaque biofilm is a prerequisite for triggering the host immune-inflammatory response leading to clinical disease in susceptible patients. This chronic and non-resolving inflammation results from the inability of the host immune-inflammatory response to resolve the microbial challenge in an effective manner. The microbial pathogenesis of periodontitis is based on the concept of dysbiosis involving shifts in composition of subgingival bacterial communities. In health, Gram-positive bacteria are abundant (5, 6), whereas shift toward disease involves an increase in proportions of Gram-negative anaerobes, including the classical red complex bacteria, and “keystone” pathogens such as Porphyromonas gingivalis (7). The dysbiotic microbiome and host inflammatory response stimulate each other in a self-perpetuating cycle (8, 9), and this interaction is further subject to environmental, genetic, and epigenetic modifying factors (10).
Periodontal treatment aims to control the levels of inflammation and prevent further disease progression. Therapeutic modalities primarily involve mechanical biofilm removal via root surface debridement with or without surgical intervention, as well as self-administered oral hygiene measures. Although the effectiveness of both non-surgical and surgical periodontal therapy is well-established (11), a spectrum of disease susceptibility and risk of disease progression exists across different patient groups (12, 13). Conventional periodontal treatment thus achieves varying degrees of success in different patient groups.
Consequently, in an effort to improve outcomes of periodontal treatment, especially for patients who do not respond well to conventional treatment modalities, adjunctive therapies targeting either the microbial challenge or modulation of the host response have been introduced. These, of course, come with limitations and disadvantages. Once proposed as potential host immunomodulatory agents, non-steroidal anti-inflammatory drugs are no longer considered a viable adjunctive treatment option because the long-term usage of aspirin can damage the stomach lining (14), and selective COX-2 inhibitors can result in cardiovascular complications (15, 16). Anti-cytokine therapy, as well as pro-resolvins and small molecule compounds like histone deacetylase inhibitors, remain an emerging area of research with a limited evidence base. Furthermore, because cytokines function in complex networks with overlapping roles and interactions (17), targeting individual cytokines in isolation may not achieve the desired clinical effects. With regard to the adjunctive use of systemic antibiotics in the treatment of periodontitis, additional benefits in clinical outcomes have been evaluated and reported in recent guidelines (18). However, this also needs to be weighed against the threat of global antimicrobial resistance associated with indiscriminate antibiotic usage.
In light of these considerations, natural compounds have received increasing attention for their potential as functional foods with combined anti-inflammatory and antimicrobial properties. These naturally derived nutraceuticals are promising candidates which circumvent the issue of antimicrobial resistance. Being dietary derivatives, there is also potential of improved patient compliance and acceptance. Furthermore, these functional foods may achieve multiple objectives, such as reducing oral inflammation while simultaneously conferring beneficial effects against systemic chronic inflammatory diseases, thereby increasing overall patient well-being. At the same time, the delivery vehicle for these functional foods should also be taken into consideration. This review highlights the limitations of current commonly used organic solvents and proposes an alternative solubility-enhancing excipient for use in future research.
Resveratrol
Resveratrol (RES) is a naturally occurring polyphenol that is found in foods such as grapes, cranberries, blueberries, peanuts and red wine (19–23). It is also a phytoalexin produced naturally by plants as a defense mechanism against insults from bacteria, fungi and chemicals.
The turn of the millennium saw a significant upsurge in published research on RES. This increased interest could be attributed to several key papers published in the 1990s. In 1992, Renaud and de Lorgeril discussed the “French paradox” – an observation in the French population that despite a diet containing high saturated fat levels, there was a relatively low mortality rate from cardiovascular disease (24). The authors hypothesized that this might be explained by the habits of wine consumption in this population. Subsequent publications highlighted the cardioprotective effects of RES, an active ingredient in red wine (25), as well as its cancer chemoprotective activity (26). These new discoveries sparked a wave of research interests in the potential benefits of RES.
Since then, a wide range of biologic properties attributed to RES has been elucidated. These include anti-inflammatory, antioxidant, anti-aging, anticarcinogenic and neuroprotective effects (27–29). RES is also well tolerated by humans (30, 31). As a result, its therapeutic potential for various medical conditions has been explored in numerous experimental and clinical studies. For example, its antioxidant and anti-inflammatory properties have been investigated in murine models of diabetes (32, 33), cardiovascular diseases (34, 35), arthritis (36) and chronic obstructive pulmonary disease (37, 38).
RES is a stilbene, which is a subcategory of the polyphenol family. It is made up of two phenol rings linked to each other by an ethylene bridge. RES also has different analogs such as pterostilbene (PTS; trans-3,5-dimethoxy-4-hydroxystilbene), oxyresveratrol (OXY; trans-2,3′,4,5′-tetrahydroxystilbene) and piceatannol (PIC; trans-3,4,3′,5′-tetrahydroxystilbene).
Similar to RES, the other three analogs all display anti-inflammatory and antioxidant properties (39–45). However, slight variations in chemical structure confer these analogs with different pharmacokinetic properties compared to RES (Figure 1). Further details are elaborated upon later.
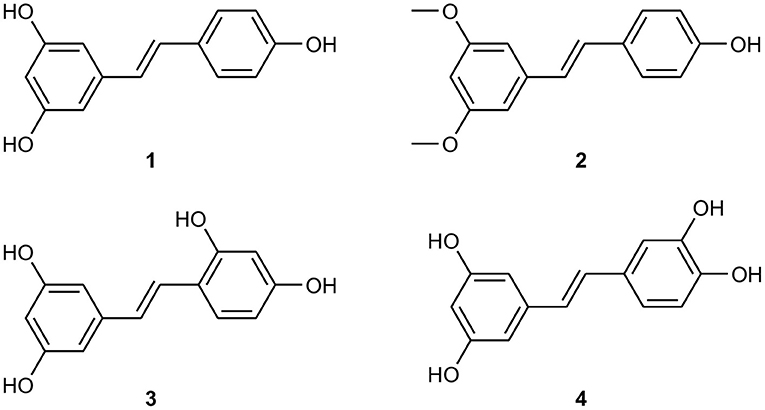
Figure 1. Chemical structures of (1) resveratrol (2) pterostilbene (3) oxyresveratrol (4) piceatannol.
The biotransformation of RES and PTS has been well elucidated (46). Phase II metabolic enzymes such as uridine 5′-diphospho-glucuronosyltransferase (UDP- glucuronosyltransferase) and sulfotransferase are the major in vivo metabolic mechanisms, while the gut microbiota also plays an important role in their biotransformation (46). Although the biotransformation pathways of PIC and OXY are still being examined, phase II metabolism and gut microbiota appear to determine their bio-conversion and elimination (47–49). The phase II metabolites of RES and its analogs are generally more polar, less bio-membrane permeable and less potent than their parent compounds. However, due to reversed metabolism mediated by glucuronidase and sulfatase, the phase II metabolites can be hydrolyzed and converted back to the aglycon form, thus serving as deposits of the parent compounds. The exact contribution of the metabolites of RES and its analogs on their biological activities remains largely unclear.
With regard to the safety profiles of RES and its analogs, animal studies showed that RES concentrations up to 300 mg/kg body weight showed no harmful effects in rats, although toxic effects were observed at ≥1,000 mg/kg body weight (50). In a clinical study on healthy human volunteers, oral doses of 5 g did not elicit adverse events (30). In a preclinical study on Swiss mice, high chronic doses of PTS (3,000 mg/kg) administered for 28 days similarly did not cause notable toxic side effects (51). A recent clinical trial conducted on patients with hypercholesterolemia demonstrated a favorable safety profile of PTS up to 250 mg per day (52). Although the clinical testing of PIC is still in its infancy, a clinical trial showed that an 8-week daily oral intake of 20 mg PIC increased insulin sensitivity and decreased blood pressure and heart rate in overweight men (53). Of note, similar adverse events were reported from participants in both test and placebo groups, thus suggesting an excellent safety profile of PIC.
In the past decade, the completely methoxylated resveratrol analogs have also attracted some interest in the biomedical community (54–57). Examples of these analogs include resveratrol trimethyl ether (RTE; trans-3,5,4′-trimethoxystilbene), oxyresveratrol trimethyl ether (OTE; trans-2,4,3′,5′-tetramethoxystilbene), trans-3,4,5,4′-tetramethoxystilbene (DMU-212) and trans-2,4,3′,4′,5′-pentamethoxystilbene. Compared with hydroxystilbenes such as RES, these completely methoxylated analogs lack antioxidant effects but display much stronger cytotoxic effects in transformed cells. However, since the completely methoxylated stilbenes have much poorer solubility, formulation into an appropriate dosage form for dental application is unlikely. Hence, they will not be discussed further in this review.
Anti-Inflammatory and Antioxidant Properties
Within the last decade, several studies have provided evidence of the potential utility of RES in the treatment of periodontitis. A study by Park et al. showed that RES rescued P. gingivalis lipopolysaccharide (LPS)-induced endothelial dysfunction (58). The underlying mechanism was a RES-mediated suppression of nuclear factor kappa B (NF-κB) activation in the endothelial cells. The authors further postulated that downregulation of Toll-like receptor (TLR) expression might be a possible underlying mechanism for the anti-inflammatory properties of RES. Although it should be noted that this study was not specifically designed to investigate RES usage in the context of periodontal treatment, nevertherless the findings shed light on the potential immunomodulation ability of RES against periodontal pathogens through its ability to modulate NF- κB, a key transcription factor regulating modulation of inflammatory host response pathway in periodontal disease pathogenesis (REF).
In an in vitro study utilizing human periodontal ligament cells (HPLCs), RES concentrations of up to 22.8 mg/mL led to a significant dose-dependent decrease in P. gingivalis lipopolysaccharide (LPS) induced nitric oxide (NO) production (59) investigated RES treatment of. In addition, RES was able to suppress production of pro-inflammatory cytokines including interleukins-1β (IL-1β), IL-6, IL-8, IL-12, and tumor necrosis factor (TNF)-α in HPLCs.
The therapeutic anti-inflammatory effect of RES in vivo has also been studied using experimental ligature-induced periodontitis in rats (60–62). Casati et al. found that the test group, which received 10 mg/kg of RES per day systemically administered for 30 days, had significantly less alveolar bone loss and gingival tissue IL-17 levels compared to controls. This was interpreted to be a positive modulation of the host immunoinflammatory response by RES. However, the authors were unable to demonstrate an immunomodulatory effect of RES on other selected cytokines, with no significant differences noted in IL-1β and IL-4 levels between the test and control groups. Using the same bead-based multiplex immunoenzymatic assay technique to measure cytokine levels in the gingival tissues, a subsequent study by the same research group (62) found 10-fold higher IL-4 levels in the test group (treated with RES) as compared to controls. A modulatory effect on IL-1β production in the test group was also noted, with ligated rats having comparable IL-1β levels to their non-ligated counterparts. Conversely, in the control group, ligated rats had statistically significantly higher IL-1β levels compared to the non-ligated subjects. However, when comparing the ligated rats between the test and control groups, no statistically significant differences were noted with regard to both IL-1β and TNF-α levels. These results should be interpreted with caution because the mean values were accompanied by large standard deviations. It is also worth mentioning that the solvents used for RES in the studies by Casati et al. and Correa et al. were different (ethanol and polysorbate, respectively). Effects from changing the solvent, if any, would compromise the validity of a direct comparison of the results from both studies. In any case, both studies showed that RES treatment resulted in reduced alveolar bone loss from ligature-induced periodontitis.
Similar findings in rats were demonstrated by Tamaki et al., who utilized micro-computed tomography (micro-CT) to show reduced alveolar bone loss with RES treatment (61). Compared to the aforementioned studies by Casati et al. and Correa et al., this study had a greater focus on investigating the pathways modulated by RES. Specifically, RES activated the nuclear factor E2-related factor 2 (Nrf2) antioxidant pathway, with an accompanying increase in expression of antioxidant genes such as heme oxygenase-1 (HO-1) and NAD(P)H:quinine oxidoreductase-1 (NQO-1), as well as reduced expression of inducible nitric oxide synthase (iNOS). Activation of the sirtuin 1 (Sirt1) pathway was also noted, in conjunction with an inhibitory effect on the NF-κB/MAPK (mitogen-activated protein kinase) pathway. The key limitation of this study was that although the concentration of RES used was similar to that used in previous studies (10 mg/kg/day), the test compound was not a pure RES isolate and also contained other derivatives such as isorhapontigenin. In general agreement with these findings, a recent study (63) showed a dose-dependent ability of RES (dissolved in ethanol) to modulate P. gingivalis-induced NF-κB activation, with significant inhibition noted from concentrations as low as 0.001 mg/mL. The study further showed that at concentrations of up to 0.00125 mg/mL, RES treatment resulted in a statistically significant downregulation of the expression of TREM-1 (triggering receptor expressed by myeloid cells) and reduced secretion of TNF-α.
Studies have also investigated the immunomodulatory ability of RES in the context of periodontitis associated with concomitant systemic conditions. In a preclinical study investigating the therapeutic effect of RES on experimental periodontitis in diabetic rats (64), a RES concentration of 20 mg/kg inhibited TLR-4 expression both in vitro and in vivo. The in vitro arm of the study showed that under high glucose conditions, treatment of gingival epithelial cells with 2.3 mg/mL RES downregulated the TLR-4-mediated production of pro-inflammatory cytokines IL-1β, IL-6, IL-8, and TNF-α, as well as the TLR-4-mediated activation of downstream signaling molecules such as the p65 subunit of NF-κB.
The ability of RES to act as a competitive antagonist to the receptor for aryl hydrocarbons (AhR) has also been studied (65). Aryl hydrocarbons are found in cigarette smoke and have been shown to impede osteogenesis and bone formation (66). In an in vitro study (67), RES supplementation was able to reverse osteogenesis inhibition, as measured by mineralized bone nodule formation by rat bone marrow cells, induced by the aryl hydrocarbon benzo[a]pyrene (BaP) and P. gingivalis-derived LPS. This ability of RES was further investigated in an animal study (68). This group investigated whether RES was able to modulate the effects of cigarette smoke inhalation on experimentally induced periodontitis in the rat. Morphometric and micro-CT measurements showed that, as compared to rats which were exposed to cigarette smoke alone, the test group which had also received daily administration of 10 mg/kg/day RES for 30 days (19 days before and 11 days after initiation of periodontitis) experienced less alveolar bone loss and higher bone interradicular density at periodontitis sites. It was also found that treatment with RES led to increased levels of IL-4, reduced levels of Th-17, and downregulated expression of receptor activator of NF-κB ligand (RANKL), a key mediator in osteoclastogenesis.
In summary, general trends may be noted with respect to an effect of RES in downregulating mRNA expression of certain pro-inflammatory cytokines (Figure 2). Such findings are both promising and clinically relevant in the context of periodontal disease, as these cytokines play important and interconnected roles in the pathogenesis of periodontal disease (17). However, in vitro data still constitute the bulk of current evidence, while the experimental studies available were largely conducted by a single research group. Furthermore, although results presented in terms of gene expression levels have mostly been positive (i.e., in support of an anti-inflammatory effect of RES), these need to be confirmed by downstream data based on protein levels. In addition, different studies have made use of different solvents for RES, which potentially adds a confounding factor and limits the ability to meaningfully compare results.
Antimicrobial Properties
There are relatively fewer studies on the potential antimicrobial properties of RES against bacteria implicated in the pathogenesis of periodontitis. In general, these have showed some promising results, but again, a variety of solvents have been used across the different studies. Also, some studies investigated planktonic bacteria, others biofilm bacteria, while one studied the effect on both. In an in vitro study utilizing the broth dilution method, O'Connor et al. found that RES dissolved in dimethyl sulfoxide (DMSO) had an inhibitory effect on planktonic periodontal pathogens, with minimum inhibitory concentration50 (MIC50) values of 1.28 and 0.08 mg/mL against A. actinomycetemcomitans and P. gingivalis, respectively (69). There were significant decreases in viable cell counts after just 1 h, thus indicating a rapid mode of antibacterial action. Another interesting observation was that A. actinomycetemcomitans proved more resistant to the antimicrobial effects, as evidenced by the 16-fold higher MIC50 concentration.
Another study investigated the effect of different RES concentrations on planktonic Fusobacterium nucleatum growth as well as biofilm formation (70). The MIC for planktonic F. nucleatum was 0.1 mg/mL. In their co-formed biofilm model, sub-MIC concentrations of RES (0.0015625–0.025 mg/mL) were all able to inhibit F. nucleatum biofilm formation, with statistically significant inhibitory effects achieved at 24, 36, and 48 h from onset of incubation. At these concentrations, RES was also able to induce F. nucleatum aggregation, with visible cell aggregation taking place at 0.025 mg/mL. Such aggregates resulted in formation of a thinner and “looser” biofilm. Unfortunately, the solvent used to dissolve RES was not reported, thus making it difficult to compare the obtained results with those from other research groups.
A recent study published by a Canadian group (63) also found antimicrobial effects of RES, dissolved in ethanol, against both planktonic and biofilm P. gingivalis. The MIC and minimum bactericidal concentration (MBC) values of RES against planktonic P. gingivalis were 0.25 and 0.5 mg/mL, respectively, with a 77.4% reduction of bacterial growth noted at a concentration of 0.125 mg/mL. The range of inhibition achieved with these values seems to be in agreement with that achieved by O'Connor et al., although one caveat is that the bacterial inoculum was not clearly reported in the study of Ben Lagha, which limits the validity of a direct comparison. In addition, Ben Lagha et al. also showed that treatment of 24-h preformed P. gingivalis biofilm with 0.25 mg/mL RES for an hour was able to decrease biofilm viability by slightly more than 50%, as measured by an adenosine triphosphate (ATP) luminescence assay. No significant effect on biofilm desorption nor detachment was noted, even at RES concentrations up to 1 mg/mL. This finding contrasts with the results obtained by He et al. (70), possibly due to the different biofilm model used (co-formed as compared to preformed). Considering these results in view of the limitations of early evidence obtained from in vitro models conducted in just a few studies, these suggest that any anti-biofilm effects of RES may be occurring via inhibitory mechanisms during the early stages of biofilm formation.
In contrast to the promising antimicrobial findings demonstrated by the aforementioned studies, a study which utilized a pre-formed 6-day mixed species biofilm model found that treatment with a RES concentration of 0.01% w/v for 30 min did not lead to significant alteration in biofilm species composition (71). Scanning electron microscopy (SEM) also demonstrated no apparent visual destabilization of the biofilm architecture. In their multi-species biofilm, Streptococcus mitis was the most dominant, followed by F. nucleatum, A. actinomycetemcomitans, and P. gingivalis, in decreasing order of proportion. Two key features of the methodology should be kept in mind when interpreting the results of this study. First, the use of distilled deionised water as the solvent for RES, which is an organic compound, might have resulted in undetectable precipitation of the compound with less of it available in its active form. Second, the short duration of exposure (30 min) to the test compound might have been insufficient to exert a measurable effect against a mature biofilm that had formed for 6 days.
An experimental study in rats was also unable to demonstrate antimicrobial effects of RES (72). The animals underwent experimental ligature-induced periodontitis with a test group receiving daily administration of 10 mg/kg RES for 30 days, and a control group receiving a placebo. Therapies commenced 19 days before periodontitis induction and continued for 11 days after. Microbiological evaluation of the ligature showed no statistically significant differences in levels of A. actinomycetemcomitans, P. gingivalis, and Tannerella forsythia between test and control groups, though there was a (non-significant) trend toward lower concentrations of these pathogens in the test group.
Mechanisms of Antimicrobial Action of RES
Antimicrobials can kill or inhibit bacterial growth through several mechanisms. For example, they may disrupt the bacterial cell membrane, or inhibit synthesis of various biological components critical for cell function and survival, such as its cell wall, proteins, and nucleic acids.
In the literature, the antimicrobial effects of RES and its analogs have been documented against both Gram-positive and Gram-negative bacteria. This non-specific antibacterial effect is thus independent of the unique characteristics ascribed to either type of bacteria. A number of mechanisms have been proposed, with most of the studies demonstrating some form of cell membrane disruption induced by the compounds. For example, Joung et al. demonstrated direct binding of OXY to the peptidoglycans on the bacterial cell wall of methicillin-resistant Staphylococcus aureus (73). Co-treatment with peptidoglycan conferred a rescue effect on the bacteria, as measured by increases in optical density (OD600) readings. The authors also used transmission electron microscopy (TEM) imaging of the bacterial cells to confirm the presence of cytoplasmic membrane disruption.
Other studies working with PTS have produced similar results. Using SEM imaging, one study showed that the bacterial cell membranes appeared to have more blebs and irregularities after PTS treatment (74). Yang et al. used TEM to show that the cytoplasm of bacterial cells appeared amorphous and empty after PTS treatment, thus indicating a loss of intracellular contents (75). Similarly, a recent study used SEM to demonstrate a more shriveled and pitted morphology of S. aureus and Escherichia coli cell membranes after PTS treatment, as well as TEM imaging to show that PTS caused cell wall destruction for both the Gram-negative and Gram-positive bacteria (76). In addition, the authors measured OD260 and OD280 values to demonstrate a dose-dependent leakage of intracellular nucleic acids and proteins as a result of the perturbation of membrane integrity from PTS treatment. Another research group used a membrane permeabilization assay based on release of calcein-AM from bacteria to show that RES was able to alter cell membrane permeability of P. gingivalis (63).
Alterations in gene and protein expression is another mechanism through which the compounds exert their antimicrobial effects. In the study by He et al., at sub-MIC (concentrations below MIC) RES concentrations of 0.0125 and 0.025 mg/mL, genes involved in quorum sensing, tolerance to environmental stresses and virulence factors, were found to be downregulated (70). This is in agreement with other studies showing that RES treatment causes biofilm inhibition of E. coli and S. aureus because of hindrance of quorum sensing activity (77, 78).
Similarly, Ren et al. reported that for both S. aureus and E. coli, PTS treatment downregulated the expression of genes related to cell wall synthesis (76). Changes in protein expression were shown by Yang et al. (75), who used SDS-polyacrylamide gel electrophoresis (SDS-PAGE) to demonstrate that chaperones and GAPDH (glyceraldehyde 3-phosphate dehydrogenase) were downregulated by PTS treatment. Chaperones help S. aureus adapt to and survive environmental stresses, hence downregulation of their expression could have maintained the bacteria in a stressed state, facilitating killing. GADPH is protective against oxidative stress, hence its downregulation by PTS would have made the bacteria more susceptible to oxidative damage. However, one puzzling finding from that study is that in contrast to PTS, RES actually upregulated the expression of these proteins. The authors postulated that the two compounds probably had different antibacterial mechanisms against S. aureus. Another plausible, although unsubstantiated, hypothesis might be an inferior potency of RES (as compared to PTS) in generation of oxidative stress against the bacterial cells. This would have given some allowance for the bacteria to cope with the stress and mount a feedback response, thus explaining the upregulated protein expressions of chaperones and GAPDH.
Next, it has also been shown that RES and its analogs are capable of generating oxidative stress in bacterial cells. Subramanian et al. proposed that RES induced cellular membrane oxidation (79), while the aforementioned study by Ren et al. showed that PTS treatment led to upregulation of genes associated with oxidative stress response mechanisms (76). This finding was supplemented by demonstration of increased production of intracellular reactive oxygen species (ROS) as detected by the fluorescent dye dihydrorhodamine 123 (DHR123). Although their study did not prove a direct causal link between the presence of ROS leading to bacterial cell death, the authors theorized that this was a reason behind the bactericidal activity of PTS, as intracellular ROS may stimulate apoptosis of bacterial cells.
Results from a 2015 study caution against the hasty conclusion that the antimicrobial effect is directly related to ROS production (80). In that study, RES treatment against E. coli increased intracellular production of ROS in a dose-dependent manner, but growth of RES-treated bacterial cells failed to recover even after rescue treatment with thiourea, which is a hydroxyl radical scavenger. Phase-contrast microscopy revealed morphological changes in bacterial cells treated by RES, namely dose-dependent cell elongation and filamentation, suggesting an inhibition of cell division by interfering with septum formation. Although it was additionally found that RES induced DNA fragmentation damage and upregulated the cell response to DNA damage, inhibition of FtsZ gene expression (and thus inhibition of Z-ring formation, which is a crucial step in bacterial cell division) was the direct cause of bacterial cell growth inhibition.
Taking all these findings together, it is clear that the RES compounds have the ability to interfere with the normal homeostatic mechanisms of bacterial cell function in several different ways. However, not all have a direct causative effect on bacterial cell death, and may also vary according to concentration the compound is administered at.
On a slightly different note, RES has also been shown to attenuate virulence properties of P. gingivalis in addition to inhibition of its growth (63). More specifically, RES treatment decreased bacterial adherence capability, attenuated P. gingivalis-induced loss of tight junction integrity in oral keratinocytes, and inhibited P. gingivalis degradation of Type I collagen.
Interactions of RES with Other Antimicrobials
There are only a handful of in vitro studies evaluating the interactions of RES or its analogs with other antimicrobials, against a select group of pathogens. There are none which focus on periodontal pathogens. A 2012 study showed that RES had a synergistic antimicrobial effect with ciprofloxacin and cefotaxime against Gram-positive (Bacillus subtilis, S. aureus) and Gram-negative (E. coli, Pseudomonas aeruginosa) bacteria (81). Synergism of RES has also been demonstrated against parasites, with increased activity noted when combined with amphotericin B against amastigotes (82). Another study investigating OXY demonstrated the potential of its combination with other antibiotics to suppress bacterial growth in vitro (83). Combination with gentamycin or ciprofloxacin achieved complete growth inhibition of S. aureus after 24 h. All the antibiotic combinations with OXY also had reduced MIC values as compared to when the antibiotics were used in isolation. Similar results were obtained with PTS in combination with gentamicin against S. aureus, E. coli and P. aeruginosa in a recent study (74).
Rationale for Increased Interest in RES Analogs
Despite the beneficial properties of RES and its promising applications, it has a relatively poor pharmacokinetic profile. The oral bioavailability of an administered drug compound is affected by properties such as its aqueous solubility, membrane permeability and metabolic stability (84). The inferior pharmacokinetic properties of RES are well-documented in the literature (27, 85). For example, RES has a short half-life (86, 87) and undergoes extensive phase II metabolism involving glucuronide or sulfate conjugation of its hydroxyl groups, with the consequent production of phase II metabolites in the form of glucuronides and sulfates (27, 88). It also suffers from poor oral bioavailability and undergoes rapid elimination (89). This problem of low bioavailability cannot be overcome simply by administering higher dosages of RES, due to concerns relating to financial costs and toxicity profiles. Assuming a weight of 75 kg, daily dose administration of 100 mg/kg (body weight) of RES would require 2.7 kg of RES in a year. This results in an estimated cost of about US$6,800 (27). Thus, these inherent limitations in the pharmacokinetic profile of RES have led to growing interest in its analogs as alternative therapeutic candidates instead, due to their superior pharmacokinetic properties.
One key reason behind the different pharmacokinetic profiles between RES and its analogs lies in the chemical structures of the individual compounds. A 2016 study by Chen et al. (90) on the comparative pharmacokinetic profiles of RES and OXY showed that after intravenous administration, OXY took a longer time to be eliminated than RES. After oral administration, OXY was absorbed and entered the systemic circulation rapidly, likely due to its increased aqueous solubility. OXY is a tetrahydroxystilbene and thus has an additional hydroxyl (-OH) group on its aromatic ring, as compared to RES, which is a trihydroxystilbene with only 3 hydroxyl groups. Although this additional hydroxyl group can also increase susceptibility to phase II metabolism, as documented by studies showing that OXY undergoes extensive first-pass metabolism in human and rat liver microsomes (91) and human intestinal microsomes (47), findings from Chen et al. showed that OXY had comparable oral plasma exposure to RES. The authors theorized that this could possibly be due to location of the hydroxyl groups, rather than quantity per se, which determined metabolic instability (90).
As for PTS, it has a methoxylated structure (3,-5-dimethoxy structure) and only a single hydroxyl group. This partial methylation makes PTS more resistant to phase II conjugation metabolism. It also confers a more favorable pharmacokinetic profile with regard to plasma exposure, half-life and rate of clearance. These have been supported by preclinical pharmacokinetic studies (92–95). Yeo et al. showed that after intravenous administration, PTS was eliminated more slowly and had longer mean transit time (MTT) than RES. Similarly, when given as an oral solution, PTS had lower peak serum concentration (Cmax) but 5–6 times higher plasma exposure and MTT than RES, evidencing its superior metabolic stability.
Due to the presence of additional methoxy groups, PTS is also more lipophilic than RES. Its moderate lipophilicity and non-polar characteristics (41, 96) potentially allow it to have better membrane permeability. This plausibly explains its superior biological potency over RES as shown in other studies. For example, Choo et al. (95) make the case for PTS as a more suitable candidate over RES for further development. Their in vitro study showed that PTS demonstrated greater potency than RES in the inhibition of LPS-induced NF-κB p65 nuclear accumulation, suppression of IL-6, IL-18 and vascular endothelial growth factor (VEGF) secretion, and anti-proliferative effects on human rheumatoid arthritic synovial fibroblasts (RASFs). In terms of biodistribution, PTS exhibited a high level of penetration to major organs after a single oral dose. Pharmacokinetics also remained favorable after repeated dosing, which led the authors to postulate that the distribution profile with a chronic PTS uptake should be comparable to that of a single administration. This has favorable implications for chronic disease prevention and treatment, as there would be a reduced need for dose adjustment.
Another example of a methoxylated analog possessing increased biological potency compared to RES is isorhapontigenin (ISO). ISO also exerts similar anti-inflammatory properties by acting on the NF-κB and MAPK pathways (97, 98). A recent study (99) demonstrated the superiority of ISO over RES as an anti-inflammatory agent for chronic obstructive pulmonary disease (COPD). ISO exerted an inhibitory effect on NF-κB transcriptional activity, as evidenced by a more than 75% inhibition of increase in NF-κB luciferase reporter gene expression after IL-1β stimulation. On the other hand, RES did not appear to have a significant effect. The greater biological effect of ISO, compared to RES, has been attributed to the presence of an additional meta-methoxy group in its chemical structure (100).
Choice of Solvents
Thus far, it is evident that the solvents used for RES and its analogs differ between studies. Being organic compounds, RES and its analogs have poor aqueous solubility, which in turn affects the rate and/or extent of oral bioavailability. Aqueous solubility is one of the key factors that determines oral absorption of xenobiotics (101). Lipid soluble drugs are less easily absorbed than water-soluble ones, especially for enteral administration. This means that the increased lipophilicity of certain methoxylated RES analogs like PTS is a double-edged sword. Although it grants greater potency of biological effects due to enhanced membrane permeability, it also reduces aqueous solubility of the compound (102), which in turn negatively affects oral bioavailability.
At the same time, usage of common organic solvents such as dimethyl sulfoxide (DMSO) to dissolve RES is not without its disadvantages. Since decades ago, it has been established that DMSO is capable of exerting cytotoxic effects. High dosages of DMSO can cause damage to blood cells, local irritation and necrosis (103), while another study warned of 50% mortality in humans weighing 80 kg when exposed to 880 g (applied on the skin) or 320 g (administered intravenously) of DMSO. At concentrations above 10% v/v, it has also been reported to induce cell apoptosis (104, 105). Due to these safety concerns, DMSO has to be used at sufficiently low concentrations (106–108).
Furthermore, recent in vitro studies have raised concerns about the toxic effects of DMSO observed even at concentrations below 10% v/v (109, 110). In particular, the study by Verheijen et al. utilized sensitive and high-throughput modern techniques to analyse the transcriptome, proteome and DNA methylation profiles of human cardiac and hepatic microtissues exposed to 0.1% DMSO for 2 weeks. They found that even at such a low concentration, DMSO could still affect cellular processes involved in metabolism and vesicle-mediated transport. DNA methylation mechanisms were also disrupted. Based on the capability of 0.1% DMSO to induce alterations in cellular processes, microRNA and epigenetics, the authors concluded that DMSO should only be used where absolutely necessary and in the lowest concentrations possible.
Consequently, the need to work with the lowest possible concentrations of DMSO puts a serious handicap on the ability to dissolve critical amounts of RES required for meaningful biological activity. Therefore, the second key challenge in translating the researched benefits of RES and its analogs to clinical applicability is choosing a non-toxic vehicle for these compounds.
Cyclodextrins and Their Pharmaceutical Applications
Cyclodextrins are manufactured from starch through degradation by glucosyltransferase enzymes, and were first isolated in 1891 by Antoine Villiers. They are cyclic oligosaccharides with a basic structure of linked D-glucopyranose units. The three most common natural cyclodextrins, also called “parent” cyclodextrins, are α-, β-, and γ-cyclodextrin (111). These parent cyclodextrins have 6, 7, and 8 linked D-glucopyranose units, respectively. The key characteristic of cyclodextrin molecules lies in their cone-shaped structure, so formed due to the chair-like structure of the individual glucopyranose units (112). This cone has a hydrophilic exterior and hydrophobic interior cavity. Cavity size is determined by the number of linked glucopyranose units, with α-, β-, and γ-cyclodextrin having cavity diameters of about 5.2, 6.6, and 8.4 Å, respectively (113). The three natural cyclodextrins (α-, β-, and γ-cyclodextrin) are all included in the Generally Recognized as Safe (GRAS) list by the US FDA (United States Food and Drug Administration) (114).
The first pharmaceutical patent for cyclodextrins was issued in Germany in 1953 (115), while the first pharmaceutical product containing cyclodextrin (prostaglandin E2 in β-cyclodextrin sublingual tablets) went on the market in 1976 (112). Today, cyclodextrins are widely utilized in the pharmaceutical, food and agrochemical industries (116). Their cone-shaped structure with a hydrophilic exterior and hydrophobic inner cavity confers the ability to behave as “transport vehicles,” carrying hydrophobic drug molecules to form an inclusion complex. Part, or all, of the molecule, may be encapsulated within the cavity. A dynamic equilibrium is thus formed between free cyclodextrins, free drug molecules, and inclusion complexes formed either in a 1:1 or 1:2 ratio. Large ring cyclodextrins with more than eight linked glucopyranose units are more expensive and do not form inclusion complexes as well as the smaller parent cyclodextrins. As a result, large ring cyclodextrins have less utility in the pharmaceutical industry (117).
Attempts have been made to measure the kinetics of drug release from drug-cyclodextrin inclusion complexes with fast kinetics techniques but to no avail, thus indicating that the process takes place faster than it can be measured. Using ultra-fast kinetics techniques, other groups have found that the rates approach diffusion-controlled limits (111). Thus, drug dissociation from and binding with cyclodextrin may be treated as simply a rapid equilibrium, in which simple dilution drives the drug release (118). Drug-protein binding, competitive drug displacement, and drug uptake into tissues inaccessible by free cyclodextrin molecules, may all also contribute to drug release post-administration (118).
Although the three most investigated cyclodextrins, α-, β-, and γ-cyclodextrin, appear to have rather similar complexation abilities (119), β-cyclodextrin has a cavity diameter (6–6.5 Å) of the optimal size for most chemical entities (120), including compounds with aromatic rings such as RES and its analogs. However, natural cyclodextrins have very poor aqueous solubility, with β-cyclodextrin being the least water soluble (~0.016 M at 25°C), compared to α- (0.12 M) and γ-cyclodextrin (0.17 M). The reason for its poor water solubility is due to formation of intramolecular hydrogen bonds between the C2- and C3- hydroxyl groups of neighboring glucose units, which makes the compound very stable (113).
In order to overcome this issue, the cyclodextrin is “functionalized” by chemically modifying the 2-, 3- or 6- hydroxyl groups of its glucose residues (116). This prevents intramolecular hydrogen bond formation and crystallization of the cyclodextrin. For example, the hydroxyl groups of β-cyclodextrin may be substituted with hydrophilic moieties such as hydroxypropyl groups to give hydroxypropyl-β-cyclodextrin (HPβCD). HPβCD has an aqueous solubility of ≥60% w/w and is very hydrophilic (121).
The safety profile of HPβCD has been evaluated in several human studies, with doses up to 200 mg/kg, given orally twice daily, being well tolerated. Single-dose intravenous administration of HPβCD up to 3 g also did not produce any toxic renal side-effects in healthy human volunteers (111). Its biocompatibility has allowed HPβCD to be a component of several US FDA approved products for human usage (116).
Cyclodextrins as a Solubility-Enhancing Excipient for RES
The oral bioavailability of a drug is dependent on its ease of dissolution and subsequent absorption through the gastrointestinal tract to the extent that sufficient drug concentrations are available at the pharmacologically active site for producing the necessary biological effect. In the Biopharmaceutical Classification System, drugs are classified into four categories based on their dissolution and permeability characteristics (122, 123). Type I compounds have the most ideal characteristics, with both high solubility and high permeability. In situations involving Type II compounds, which have high permeability but low aqueous solubility, the complexation ability of cyclodextrins makes them pharmaceutically useful as vehicles to increase the apparent aqueous solubility of Type II candidate molecules such that they approximate Type I characteristics, with a resulting increase in oral bioavailability (124, 125). To this end, HPβCD has been utilized in the pharmaceutical industry to enhance solubility of various nutraceuticals (126).
Although RES analogs like PTS have better pharmacokinetic profiles owing to differences in chemical structure, presence of methoxy groups also reduces the aqueous solubility (102). Utilizing HPβCD as the choice of solvent can address this issue. Yeo et al. showed that administering PTS with HPβCD as a solubility enhancing excipient helped to overcome the problem of its limited aqueous solubility, significantly increasing its oral absorption and bioavailability (94). Similar results were obtained for RES in an earlier study (89).
Cyclodextrins enhance the aqueous solubility of their guest molecules in a linear fashion, especially when 1:1 complexes are formed (111). This is in contrast to cosolvents or surfactants (such as DMSO and ethanol), which modify bulk properties of the solution, thus increasing aqueous solubility in an exponential manner. Consequently, upon dilution of the drug-solvent mixture with an aqueous solvent, the poorly water-soluble drug will tend to precipitate out. This is not an issue when HPβCD is utilized as the solvent for PTS, as shown by linear phase-solubility curves documented in the literature (94).
Utility of Cyclodextrin-RES Complexes in the Context of Periodontal Treatment
The comparative antimicrobial properties of RES and its analogs (OXY, PIC, and PTS), utilizing HPβCD as the solubility-enhancing excipient, were recently investigated (127) against F. nucleatum, which is a key bridging organism in plaque biofilm (128) and a potent instigator of the inflammatory response (129–132). Results from the study showed that PTS, complexed with HPβCD, was capable of conferring antimicrobial, anti-inflammatory and antioxidant effects in the presence of an F. nucleatum bacterial challenge (127). Complexation of PTS with HPβCD also led to increased antimicrobial potency as compared to the other analogs (RES, OXY, and PIC). These encouraging results support a focus for future research directed toward PTS, complexed with HPβCD, as a candidate nutraceutical for the adjunctive treatment of periodontitis.
In light of the global push for greater antibiotic stewardship, the combination of such antimicrobial, anti-inflammatory and antioxidant properties in a naturally derived compound positions it as an exciting potential addition to the current armamentarium of adjunctive therapies for periodontitis, particularly for patients who do not respond well to conventional root surface debridement. Furthermore, given that RES and its analogs have beneficial properties for other systemic conditions, their potential as adjunct therapies for periodontitis dovetails well with their utility as functional foods for comorbid conditions such as diabetes (32).
Discussion and Future Perspectives
Thus far, there is still an incomplete picture of the exact mechanisms through which RES and its analogs achieve their immunomodulatory and antimicrobial effects. With regard to immunomodulation, more studies are needed to shed light on how RES impacts the numerous biological mediators involved in the affected signaling pathways. Rather than a reductionist approach analyzing individual mediators, methodologies allowing for a more holistic evaluation may be considered. As for antimicrobial effects, research on mixed-species biofilms is lacking. There is also a dissonance from the existing literature in terms of the differing effects that RES has on developing vs. mature biofilms. More work will be needed to verify the exact nature of the interaction of RES with mature biofilms, as well as the biological mechanisms responsible if a difference truly exists. Also, to the best of our knowledge, there has been no study which investigated the nature of interactions, if any, of RES or its analogs with commonly used antibiotics. In addition, although recent results point to PTS complexed with HPβCD as a promising candidate for future research, pre-clinical studies in larger animal models are still needed, before moving on to clinical trials in humans to confirm any potential adjunctive benefits of PTS in the treatment of periodontitis. The lack of control of action for phytomedicine and nutraceuticals is a main limitation hindering their translation to clinical reality, hence consistent results from well-controlled clinical studies will be needed to address this issue. Last but not least, further work will be needed to develop and optimize user-friendly dosage and delivery forms (e.g., chewing gums, lozenges) of the compounds.
Author Contributions
YL wrote the manuscript. HL commented on and discussed the manuscript. PP and KT revised and finalized the manuscript. All authors contributed to the article and approved the submitted version.
Conflict of Interest
The authors declare that the research was conducted in the absence of any commercial or financial relationships that could be construed as a potential conflict of interest.
References
1. Kassebaum NJ, Bernabé E, Dahiya M, Bhandari B, Murray CJ, and Marcenes W. Global burden of severe periodontitis in 1990-2010: a systematic review and meta-regression. J Dent Res. (2014) 93:1045–53. doi: 10.1177/0022034514552491
2. Tonetti MS, Jepsen S, Jin L, and Otomo-Corgel J. Impact of the global burden of periodontal diseases on health, nutrition and wellbeing of mankind: a call for global action. J Clin Periodontol. (2017) 44:456–62. doi: 10.1111/jcpe.12732
3. Sanz M, Ceriello A, Buysschaert M, Chapple I, Demmer RT, Graziani F, et al. Scientific evidence on the links between periodontal diseases and diabetes: consensus report and guidelines of the joint workshop on periodontal diseases and diabetes by the International diabetes Federation and the European Federation of Periodontology. Diabetes Res Clin Pract. (2018) 137:231–41. doi: 10.1016/j.diabres.2017.12.001
4. Sanz M, Marco Del Castillo A, Jepsen S, Gonzalez-Juanatey JR, D'Aiuto F, Bouchard P, et al. Periodontitis and cardiovascular diseases: consensus report. J Clin Periodontol. (2020) 47:268–88. doi: 10.1111/jcpe.13189
5. Griffen AL, Beall CJ, Campbell JH, Firestone ND, Kumar PS, Yang ZK, et al. Distinct and complex bacterial profiles in human periodontitis and health revealed by 16S pyrosequencing. ISME J. (2012) 6:1176–85. doi: 10.1038/ismej.2011.191
6. Abusleme L, Dupuy AK, Dutzan N, Silva N, Burleson JA, Strausbaugh LD, et al. The subgingival microbiome in health and periodontitis and its relationship with community biomass and inflammation. ISME J. (2013) 7:1016–25. doi: 10.1038/ismej.2012.174
7. Hajishengallis G, Darveau RP, and Curtis MA. The keystone-pathogen hypothesis. Nat Rev Microbiol. (2012) 10:717–25. doi: 10.1038/nrmicro2873
8. Hajishengallis G, and Lamont RJ. Beyond the red complex and into more complexity: the polymicrobial synergy and dysbiosis (PSD) model of periodontal disease etiology. Mol Oral Microbiol. (2012) 27:409–19. doi: 10.1111/j.2041-1014.2012.00663.x
9. Bartold PM, and Van Dyke TE. An appraisal of the role of specific bacteria in the initial pathogenesis of periodontitis. J Clin Periodontol. (2019) 46:6–11. doi: 10.1111/jcpe.13046
10. Loos BG, and Van Dyke TE. The role of inflammation and genetics in periodontal disease. Periodontol 2000. (2020) 83:26–39. doi: 10.1111/prd.12297
11. Sanz M, Herrera D, Kebschull M, Chapple I, Jepsen S, Beglundh T, et al. Treatment of stage I–III periodontitis—The EFP S3 level clinical practice guideline. J Clin Periodontol. (2020) 47:4–60. doi: 10.1111/jcpe.13290
12. Loe H, Anerud A, Boysen H, and Morrison E. Natural history of periodontal disease in man. Rapid, moderate and no loss of attachment in Sri Lankan laborers 14 to 46 years of age. J Clin Periodontol. (1986) 13:431–45. doi: 10.1111/j.1600-051X.1986.tb01487.x
13. Papapanou PN, Sanz M, Buduneli N, Dietrich T, Feres M, Fine DH, et al. Periodontitis: Consensus report of workgroup 2 of the 2017 World Workshop on the classification of periodontal and peri-implant diseases and conditions. J Periodontol. (2018) 89(Suppl. 1):S173–82. doi: 10.1002/JPER.17-0721
14. Kimmey MB. Cardioprotective effects and gastrointestinal risks of aspirin: maintaining the delicate balance. Am J Med. (2004) 117(Suppl. 5A):72s−8s. doi: 10.1016/j.amjmed.2004.07.012
15. Mukherjee D, Nissen SE, and Topol EJ. Risk of cardiovascular events associated with selective COX-2 inhibitors. JAMA. (2001) 286:954–9. doi: 10.1001/jama.286.8.954
16. Davies NM, and Jamali F. COX-2 selective inhibitors cardiac toxicity: getting to the heart of the matter. J Pharm Pharm Sci. (2004) 7:332–6.
17. Preshaw PM, and Taylor JJ. How has research into cytokine interactions and their role in driving immune responses impacted our understanding of periodontitis? J Clin Periodontol. (2011) 38:60–84. doi: 10.1111/j.1600-051X.2010.01671.x
18. Teughels W, Feres M, Oud V, Martín C, Matesanz P, and Herrera D. Adjunctive effect of systemic antimicrobials in periodontitis therapy. A systematic review and meta-analysis. J Clin Periodontol. (2020) 22:257–281. doi: 10.1111/jcpe.13264
19. Romero-Pérez AI, Lamuela-Raventós RM, Waterhouse AL, and de la Torre-Boronat MC. Levels of cis- and trans-Resveratrol and their glucosides in White and Rosé Vitis vinifera wines from Spain. J Agric Food Chem. (1996) 44:2124–8. doi: 10.1021/jf9507654
20. Lyons MM, Yu C, Toma RB, Cho SY, Reiboldt W, Lee J, et al. Resveratrol in raw and baked blueberries and bilberries. J Agric Food Chem. (2003) 51:5867–70. doi: 10.1021/jf034150f
21. Feijóo O, Moreno A, and Falqué E. Content of trans- and cis-resveratrol in Galician white and red wines. J Food Compost Anal. (2008) 21:608–13. doi: 10.1016/j.jfca.2008.06.002
22. Zhang Q, Bian Y, Shi Y, Zheng S, Gu X, Zhang D, et al. An economical and efficient technology for the extraction of resveratrol from peanut (Arachis hypogaea) sprouts by multi-stage countercurrent extraction. Food Chem. (2015) 179:15–25. doi: 10.1016/j.foodchem.2015.01.113
23. Acuna-Avila PE, Vasquez-Murrieta MS, Franco Hernandez MO, and Lopez-Cortez Mdel S. Relationship between the elemental composition of grapeyards and bioactive compounds in the Cabernet Sauvignon grapes Vitis vinifera harvested in Mexico. Food Chem. (2016) 203:79–85. doi: 10.1016/j.foodchem.2016.02.031
24. Renaud S, and de Lorgeril M. Wine, alcohol, platelets, and the French paradox for coronary heart disease. Lancet. (1992) 339:1523–6. doi: 10.1016/0140-6736(92)91277-F
25. Siemann EH, and Creasy LL. Concentration of the phytoalexin resveratrol in wine. Am J Enol Vitic. (1992) 43:49–52.
26. Jang M, Cai L, Udeani GO, Slowing KV, Thomas CF, Beecher CW, et al. Cancer chemopreventive activity of resveratrol, a natural product derived from grapes. Science. (1997) 275:218–20. doi: 10.1126/science.275.5297.218
27. Baur JA, and Sinclair DA. Therapeutic potential of resveratrol: the in vivo evidence. Nat Rev Drug Discov. (2006) 5:493–506. doi: 10.1038/nrd2060
28. Whitlock NC, and Baek SJ. The anticancer effects of resveratrol: modulation of transcription factors. Nutr Cancer. (2012) 64:493–502. doi: 10.1080/01635581.2012.667862
29. Park EJ, and Pezzuto JM. The pharmacology of resveratrol in animals and humans. Biochim Biophys Acta. (2015) 1852:1071–113. doi: 10.1016/j.bbadis.2015.01.014
30. Boocock DJ, Faust GE, Patel KR, Schinas AM, Brown VA, Ducharme MP, et al. Phase I dose escalation pharmacokinetic study in healthy volunteers of resveratrol, a potential cancer chemopreventive agent. Cancer Epidemiol Biomarkers Prev. (2007) 16:1246–52. doi: 10.1158/1055-9965.EPI-07-0022
31. Cottart C-H, Nivet-Antoine V, and Beaudeux J-L. Review of recent data on the metabolism, biological effects, and toxicity of resveratrol in humans. Mol Nutr Food Res. (2014) 58:7–21. doi: 10.1002/mnfr.201200589
32. Soufi FG, Sheervalilou R, Vardiani M, Khalili M, and Alipour MR. Chronic resveratrol administration has beneficial effects in experimental model of type 2 diabetic rats. Endocr Regul. (2012) 46:83–90. doi: 10.4149/endo_2012_02_83
33. Zheng X, Zhu S, Chang S, Cao Y, Dong J, Li J, et al. Protective effects of chronic resveratrol treatment on vascular inflammatory injury in streptozotocin-induced type 2 diabetic rats: Role of NF-kappa B signaling. Eur J Pharmacol. (2013) 720:147–57. doi: 10.1016/j.ejphar.2013.10.034
34. Bhatt SR, Lokhandwala MF, and Banday AA. Resveratrol prevents endothelial nitric oxide synthase uncoupling and attenuates development of hypertension in spontaneously hypertensive rats. Eur J Pharmacol. (2011) 667:258–64. doi: 10.1016/j.ejphar.2011.05.026
35. Breen DM, Dolinsky VW, Zhang H, Ghanim H, Guo J, Mroziewicz M, et al. Resveratrol inhibits neointimal formation after arterial injury through an endothelial nitric oxide synthase-dependent mechanism. Atherosclerosis. (2012) 222:375–81. doi: 10.1016/j.atherosclerosis.2012.03.021
36. Xuzhu G, Komai-Koma M, Leung BP, Howe HS, McSharry C, McInnes IB, et al. Resveratrol modulates murine collagen-induced arthritis by inhibiting Th17 and B-cell function. Ann Rheum Dis. (2012) 71:129–35. doi: 10.1136/ard.2011.149831
37. Culpitt SV, Rogers DF, Fenwick PS, Shah P, De Matos C, Russell RE, et al. Inhibition by red wine extract, resveratrol, of cytokine release by alveolar macrophages in COPD. Thorax. (2003) 58:942–6. doi: 10.1136/thorax.58.11.942
38. Culpitt SV, Rogers DF, Shah P, De Matos C, Russell RE, Donnelly LE, et al. Impaired inhibition by dexamethasone of cytokine release by alveolar macrophages from patients with chronic obstructive pulmonary disease. Am J Respir Crit Care Med. (2003) 167:24–31. doi: 10.1164/rccm.200204-298OC
39. Chung KO, Kim BY, Lee MH, Kim YR, Chung HY, Park JH, et al. In-vitro and in-vivo anti-inflammatory effect of oxyresveratrol from Morus alba L. J Pharm Pharmacol. (2003) 55:1695–700. doi: 10.1211/0022357022313
40. Cichocki M, Paluszczak J, Szaefer H, Piechowiak A, Rimando AM, and Baer-Dubowska W. Pterostilbene is equally potent as resveratrol in inhibiting 12-O-tetradecanoylphorbol-13-acetate activated NFkappaB, AP-1, COX-2, and iNOS in mouse epidermis. Mol Nutr Food Res. (2008) 52(Suppl. 1):S62–70. doi: 10.1002/mnfr.200700466
41. Remsberg CM, Yanez JA, Ohgami Y, Vega-Villa KR, Rimando AM, and Davies NM. Pharmacometrics of pterostilbene: preclinical pharmacokinetics and metabolism, anticancer, antiinflammatory, antioxidant and analgesic activity. Phytother Res. (2008) 22:169–79. doi: 10.1002/ptr.2277
42. McCormack D, Schneider J, McDonald D, and McFadden D. The antiproliferative effects of pterostilbene on breast cancer in vitro are via inhibition of constitutive and leptin-induced Janus kinase/signal transducer and activator of transcription activation. Am J Surg. (2011) 202:541–4. doi: 10.1016/j.amjsurg.2011.06.020
43. Wang Y, Ding L, Wang X, Zhang J, Han W, Feng L, et al. Pterostilbene simultaneously induces apoptosis, cell cycle arrest and cyto-protective autophagy in breast cancer cells. Am J Transl Res. (2012) 4:44–51.
44. Ashraf MI, Shahzad M, and Shabbir A. Oxyresveratrol ameliorates allergic airway inflammation via attenuation of IL-4, IL-5, and IL-13 expression levels. Cytokine. (2015) 76:375–81. doi: 10.1016/j.cyto.2015.09.013
45. Yamamoto T, Li Y, Hanafusa Y, Yeh YS, Maruki-Uchida H, Kawakami S, et al. Piceatannol exhibits anti-inflammatory effects on macrophages interacting with adipocytes. Food Sci Nutr. (2017) 5:76–85. doi: 10.1002/fsn3.366
46. Wang P, and Sang S. Metabolism and pharmacokinetics of resveratrol and pterostilbene. Biofactors. (2018) 44:16–25. doi: 10.1002/biof.1410
47. Hu N, Mei M, Ruan J, Wu W, Wang Y, and Yan R. Regioselective glucuronidation of oxyresveratrol, a natural hydroxystilbene, by human liver and intestinal microsomes and recombinant UGTs. Drug Metab Pharmacokinet. (2014) 29:229–36. doi: 10.2133/dmpk.DMPK-13-RG-102
48. Jarosova V, Vesely O, Marsik P, Jaimes JD, Smejkal K, Kloucek P, et al. Metabolism of stilbenoids by human faecal microbiota. Molecules. (2019) 24:1155. doi: 10.3390/molecules24061155
49. Dai Y, Lim JX, Yeo SCM, Xiang X, Tan KS, Fu JH, et al. Biotransformation of piceatannol, a dietary resveratrol derivative: promises to human health. Mol Nutr Food Res. (2020) 64:e1900905. doi: 10.1002/mnfr.201900905
50. Crowell JA, Korytko PJ, Morrissey RL, Booth TD, and Levine BS. Resveratrol-associated renal toxicity. Toxicol Sci. (2004) 82:614–9. doi: 10.1093/toxsci/kfh263
51. Ruiz MJ, Fernandez M, Pico Y, Manes J, Asensi M, Carda C, et al. Dietary administration of high doses of pterostilbene and quercetin to mice is not toxic. J Agric Food Chem. (2009) 57:3180–6. doi: 10.1021/jf803579e
52. Riche DM, McEwen CL, Riche KD, Sherman JJ, Wofford MR, Deschamp D, et al. Analysis of safety from a human clinical trial with pterostilbene. J Toxicol. (2013) 2013:463595. doi: 10.1155/2013/463595
53. Kitada M, Ogura Y, Maruki-Uchida H, Sai M, Suzuki T, Kanasaki K, et al. The effect of piceatannol from passion fruit (Passiflora edulis) seeds on metabolic health in humans. Nutrients. (2017) 9:1142. doi: 10.3390/nu9101142
54. Sale S, Verschoyle RD, Boocock D, Jones DJ, Wilsher N, Ruparelia KC, et al. Pharmacokinetics in mice and growth-inhibitory properties of the putative cancer chemopreventive agent resveratrol and the synthetic analogue trans 3,4,5,4'-tetramethoxystilbene. Br J Cancer. (2004) 90:736–44. doi: 10.1038/sj.bjc.6601568
55. Lin HS, Choo QY, and Ho PC. Quantification of oxyresveratrol analog trans-2,4,3',5'-tetramethoxystilbene in rat plasma by a rapid HPLC method: application in a pre-clinical pharmacokinetic study. Biomed Chromatogr. (2010) 24:1373–8. doi: 10.1002/bmc.1454
56. Lin HS, and Ho PC. Preclinical pharmacokinetic evaluation of resveratrol trimethyl ether in sprague-dawley rats: the impacts of aqueous solubility, dose escalation, food and repeated dosing on oral bioavailability. J Pharm Sci. (2011) 100:4491–500. doi: 10.1002/jps.22588
57. Aldawsari FS, and Velazquez-Martinez CA. 3,4',5-trans-Trimethoxystilbene; a natural analogue of resveratrol with enhanced anticancer potency. Invest New Drugs. (2015) 33:775–86. doi: 10.1007/s10637-015-0222-x
58. Park HJ, Jeong SK, Kim SR, Bae SK, Kim WS, Jin SD, et al. Resveratrol inhibits Porphyromonas gingivalis lipopolysaccharide-induced endothelial adhesion molecule expression by suppressing NF-kappaB activation. Arch Pharm Res. (2009) 32:583–91. doi: 10.1007/s12272-009-1415-7
59. Rizzo A, Bevilacqua N, Guida L, Annunziata M, Romano Carratelli C, and Paolillo R. Effect of resveratrol and modulation of cytokine production on human periodontal ligament cells. Cytokine. (2012) 60:197–204. doi: 10.1016/j.cyto.2012.06.004
60. Casati MZ, Algayer C, Cardoso da Cruz G, Ribeiro FV, Casarin RC, Pimentel SP, et al. Resveratrol decreases periodontal breakdown and modulates local levels of cytokines during periodontitis in rats. J Periodontol. (2013) 84:e58–64. doi: 10.1902/jop.2013.120746
61. Tamaki N, Cristina Orihuela-Campos R, Inagaki Y, Fukui M, Nagata T, and Ito HO. Resveratrol improves oxidative stress and prevents the progression of periodontitis via the activation of the Sirt1/AMPK and the Nrf2/antioxidant defense pathways in a rat periodontitis model. Free Radic Biol Med. (2014) 75:222–9. doi: 10.1016/j.freeradbiomed.2014.07.034
62. Correa MG, Pires PR, Ribeiro FV, Pimentel SZ, Casarin RC, Cirano FR, et al. Systemic treatment with resveratrol and/or curcumin reduces the progression of experimental periodontitis in rats. J Periodontal Res. (2017) 52:201–9. doi: 10.1111/jre.12382
63. Ben Lagha A, Andrian E, and Grenier D. Resveratrol attenuates the pathogenic and inflammatory properties of Porphyromonas gingivalis. Mol Oral Microbiol. (2019) 34:118–30. doi: 10.1111/omi.12260
64. Zhen L, Fan DS, Zhang Y, Cao XM, and Wang LM. Resveratrol ameliorates experimental periodontitis in diabetic mice through negative regulation of TLR4 signaling. Acta Pharmacol Sin. (2015) 36:221–8. doi: 10.1038/aps.2014.131
65. Casper RF, Quesne M, Rogers IM, Shirota T, Jolivet A, Milgrom E, et al. Resveratrol has antagonist activity on the aryl hydrocarbon receptor: implications for prevention of dioxin toxicity. Mol Pharmacol. (1999) 56:784–90.
66. Singh SU, Casper RF, Fritz PC, Sukhu B, Ganss B, Girard BJr, et al. Inhibition of dioxin effects on bone formation in vitro by a newly described aryl hydrocarbon receptor antagonist, resveratrol. J Endocrinol. (2000) 167:183–95. doi: 10.1677/joe.0.1670183
67. Andreou V, D'Addario M, Zohar R, Sukhu B, Casper RF, Ellen RP, et al. Inhibition of osteogenesis in vitro by a cigarette smoke-associated hydrocarbon combined with Porphyromonas gingivalis lipopolysaccharide: reversal by resveratrol. J Periodontol. (2004) 75:939–48. doi: 10.1902/jop.2004.75.7.939
68. Ribeiro FV, Pino DS, Franck FC, Benatti BB, Tenenbaum H, Davies JE, et al. Resveratrol inhibits periodontitis-related bone loss in rats subjected to cigarette smoke inhalation. J Periodontol. (2017) 88:788–98. doi: 10.1902/jop.2017.170025
69. O'Connor DJ, Wong RW, and Rabie AB. Resveratrol inhibits periodontal pathogens in vitro. Phytother Res. (2011) 25:1727–31. doi: 10.1002/ptr.3501
70. He Z, Huang Z, Zhou W, Tang Z, Ma R, and Liang J. Anti-biofilm activities from resveratrol against Fusobacterium nucleatum. Front Microbiol. (2016) 7:1065. doi: 10.3389/fmicb.2016.01065
71. Millhouse E, Jose A, Sherry L, Lappin DF, Patel N, Middleton AM, et al. Development of an in vitro periodontal biofilm model for assessing antimicrobial and host modulatory effects of bioactive molecules. BMC Oral Health. (2014) 14:80. doi: 10.1186/1472-6831-14-80
72. Cirano FR, Casarin RC, Ribeiro FV, Casati MZ, Pimentel SP, Taiete T, et al. Effect of Resveratrol on periodontal pathogens during experimental periodontitis in rats. Braz Oral Res. (2016) 30:e128. doi: 10.1590/1807-3107bor-2016.vol30.0128
73. Joung DK, Mun SH, Choi SH, Kang OH, Kim SB, Lee YS, et al. Antibacterial activity of oxyresveratrol against methicillin-resistant Staphylococcus aureus and its mechanism. Exp Ther Med. (2016) 12:1579–84. doi: 10.3892/etm.2016.3486
74. Lee W, Basri D, and Ghazali A. Bactericidal effect of pterostilbene alone and in combination with gentamicin against human pathogenic bacteria. Molecules. (2017) 22:463. doi: 10.3390/molecules22030463
75. Yang S-C, Tseng C-H, Wang P-W, Lu P-L, Weng Y-H, Yen F-L, et al. Pterostilbene, a methoxylated resveratrol derivative, efficiently eradicates planktonic, biofilm, and intracellular MRSA by topical application. Front Microbiol. (2017) 8:1103. doi: 10.3389/fmicb.2017.01103
76. Ren X, An P, Zhai X, Wang S, and Kong Q. The antibacterial mechanism of pterostilbene derived from xinjiang wine grape: a novel apoptosis inducer in Staphyloccocus aureus and Escherichia coli. Lwt. (2019) 101:100–6. doi: 10.1016/j.lwt.2018.11.038
77. Lee JH, Cho HS, Joo SW, Chandra Regmi S, Kim JA, Ryu CM, et al. Diverse plant extracts and trans-resveratrol inhibit biofilm formation and swarming of Escherichia coli O157:H7. Biofouling. (2013) 29:1189–203. doi: 10.1080/08927014.2013.832223
78. Qin N, Tan X, Jiao Y, Liu L, Zhao W, Yang S, et al. RNA-Seq-based transcriptome analysis of methicillin-resistant Staphylococcus aureus biofilm inhibition by ursolic acid and resveratrol. Sci Rep. (2014) 4:5467. doi: 10.1038/srep05467
79. Subramanian M, Goswami M, Chakraborty S, and Jawali N. Resveratrol induced inhibition of Escherichia coli proceeds via membrane oxidation and independent of diffusible reactive oxygen species generation. Redox Biol. (2014) 2:865–72. doi: 10.1016/j.redox.2014.06.007
80. Hwang D, and Lim YH. Resveratrol antibacterial activity against Escherichia coli is mediated by Z-ring formation inhibition via suppression of FtsZ expression. Sci Rep. (2015) 5:10029. doi: 10.1038/srep10029
81. Kumar SN, Siji JV, Nambisan B, and Mohandas C. Activity and synergistic interactions of stilbenes and antibiotic combinations against bacteria in vitro. World J Microbiol Biotechnol. (2012) 28:3143–50. doi: 10.1007/s11274-012-1124-0
82. Ferreira C, Soares DC, Nascimento MT, Pinto-da-Silva LH, Sarzedas CG, Tinoco LW, et al. Resveratrol is active against Leishmania amazonensis: in vitro effect of its association with Amphotericin B. Antimicrob Agents Chemother. (2014) 58:6197–208. doi: 10.1128/AAC.00093-14
83. Joung DK, Choi SH, Kang OH, Kim SB, Mun SH, Seo YS, et al. Synergistic effects of oxyresveratrol in conjunction with antibiotics against methicillin-resistant Staphylococcus aureus. Mol Med Report. (2015) 12:663–7. doi: 10.3892/mmr.2015.3345
84. Helen Chan O, and Stewart BH. Physicochemical and drug-delivery considerations for oral drug bioavailability. Drug Discov Today. (1996) 1:461–73. doi: 10.1016/1359-6446(96)10039-8
85. Cottart CH, Nivet-Antoine V, Laguillier-Morizot C, and Beaudeux JL. Resveratrol bioavailability and toxicity in humans. Mol Nutr Food Res. (2010) 54:7–16. doi: 10.1002/mnfr.200900437
86. Asensi M, Medina I, Ortega A, Carretero J, Bano MC, Obrador E, et al. Inhibition of cancer growth by resveratrol is related to its low bioavailability. Free Radic Biol Med. (2002) 33:387–98. doi: 10.1016/S0891-5849(02)00911-5
87. Marier JF, Vachon P, Gritsas A, Zhang J, Moreau JP, and Ducharme MP. Metabolism and disposition of resveratrol in rats: extent of absorption, glucuronidation, and enterohepatic recirculation evidenced by a linked-rat model. J Pharmacol Exp Ther. (2002) 302:369–73. doi: 10.1124/jpet.102.033340
88. Walle T, Hsieh F, DeLegge MH, Oatis JE Jr, and Walle UK. High absorption but very low bioavailability of oral resveratrol in humans. Drug Metab Dispos. (2004) 32:1377–82. doi: 10.1124/dmd.104.000885
89. Das S, Lin HS, Ho PC, and Ng KY. The impact of aqueous solubility and dose on the pharmacokinetic profiles of resveratrol. Pharm Res. (2008) 25:2593–600. doi: 10.1007/s11095-008-9677-1
90. Chen W, Yeo SCM, Elhennawy MGAA, and Lin H-S. Oxyresveratrol: a bioavailable dietary polyphenol. J Funct Foods. (2016) 22(Suppl. C):122–31. doi: 10.1016/j.jff.2016.01.020
91. Mei M, Ruan JQ, Wu WJ, Zhou RN, Lei JP, Zhao HY, et al. In vitro pharmacokinetic characterization of mulberroside A, the main polyhydroxylated stilbene in mulberry (Morus alba L.), and its bacterial metabolite oxyresveratrol in traditional oral use. J Agric Food Chem. (2012) 60:2299–308. doi: 10.1021/jf204495t
92. Lin HS, Yue BD, and Ho PC. Determination of pterostilbene in rat plasma by a simple HPLC-UV method and its application in pre-clinical pharmacokinetic study. Biomed Chromatogr. (2009) 23:1308–15. doi: 10.1002/bmc.1254
93. Kapetanovic IM, Muzzio M, Huang Z, Thompson TN, and McCormick DL. Pharmacokinetics, oral bioavailability, and metabolic profile of resveratrol and its dimethylether analog, pterostilbene, in rats. Cancer Chemother Pharmacol. (2011) 68:593–601. doi: 10.1007/s00280-010-1525-4
94. Yeo SC, Ho PC, and Lin HS. Pharmacokinetics of pterostilbene in Sprague-Dawley rats: the impacts of aqueous solubility, fasting, dose escalation, and dosing route on bioavailability. Mol Nutr Food Res. (2013) 57:1015–25. doi: 10.1002/mnfr.201200651
95. Choo Q-Y, Yeo SCM, Ho PC, Tanaka Y, and Lin H-S. Pterostilbene surpassed resveratrol for anti-inflammatory application: potency consideration and pharmacokinetics perspective. J Funct Foods. (2014) 11:352–62. doi: 10.1016/j.jff.2014.10.018
96. Roupe KA, Remsberg CM, Yanez JA, and Davies NM. Pharmacometrics of stilbenes: seguing towards the clinic. Curr Clin Pharmacol. (2006) 1:81–101. doi: 10.2174/157488406775268246
97. Li HL, Wang AB, Huang Y, Liu DP, Wei C, Williams GM, et al. Isorhapontigenin, a new resveratrol analog, attenuates cardiac hypertrophy via blocking signaling transduction pathways. Free Radic Biol Med. (2005) 38:243–57. doi: 10.1016/j.freeradbiomed.2004.10.020
98. Gao G, Chen L, Li J, Zhang D, Fang Y, Huang H, et al. Isorhapontigenin (ISO) inhibited cell transformation by inducing G0/G1 phase arrest via increasing MKP-1 mRNA stability. Oncotarget. (2014) 5:2664–77. doi: 10.18632/oncotarget.1872
99. Yeo SCM, Fenwick PS, Barnes PJ, Lin HS, and Donnelly LE. Isorhapontigenin, a bioavailable dietary polyphenol, suppresses airway epithelial cell inflammation through a corticosteroid-independent mechanism. Br J Pharmacol. (2017) 174:2043–59. doi: 10.1111/bph.13803
100. Hasiah AH, Ghazali AR, Weber JF, Velu S, Thomas NF, and Inayat Hussain SH. Cytotoxic and antioxidant effects of methoxylated stilbene analogues on HepG2 hepatoma and Chang liver cells: implications for structure activity relationship. Hum Exp Toxicol. (2011) 30:138–44. doi: 10.1177/0960327110368739
101. Lin JH, and Lu AY. Role of pharmacokinetics and metabolism in drug discovery and development. Pharmacol Rev. (1997) 49:403–49.
102. Bethune SJ, Schultheiss N, and Henck J-O. Improving the poor aqueous solubility of nutraceutical compound pterostilbene through cocrystal formation. Cryst Growth Des. (2011) 11:2817–23. doi: 10.1021/cg1016092
103. Rubin LF. Toxicologic update of dimethyl sulfoxide. Ann NY Acad Sci. (1983) 411:6–10. doi: 10.1111/j.1749-6632.1983.tb47278.x
104. Notman R, Noro M, O'Malley B, and Anwar J. Molecular basis for dimethylsulfoxide (DMSO) action on lipid membranes. J Am Chem Soc. (2006) 128:13982–3. doi: 10.1021/ja063363t
105. de Menorval MA, Mir LM, Fernandez ML, and Reigada R. Effects of dimethyl sulfoxide in cholesterol-containing lipid membranes: a comparative study of experiments in silico and with cells. PLoS ONE. (2012) 7:e41733. doi: 10.1371/journal.pone.0041733
106. Hanslick JL, Lau K, Noguchi KK, Olney JW, Zorumski CF, Mennerick S, et al. Dimethyl sulfoxide (DMSO) produces widespread apoptosis in the developing central nervous system. Neurobiol Dis. (2009) 34:1–10. doi: 10.1016/j.nbd.2008.11.006
107. Sumida K, Igarashi Y, Toritsuka N, Matsushita T, Abe-Tomizawa K, Aoki M, et al. Effects of DMSO on gene expression in human and rat hepatocytes. Hum Exp Toxicol. (2011) 30:1701–9. doi: 10.1177/0960327111399325
108. Julien C, Marcouiller F, Bretteville A, El Khoury NB, Baillargeon J, Hebert SS, et al. Dimethyl sulfoxide induces both direct and indirect tau hyperphosphorylation. PLoS ONE. (2012) 7:e40020. doi: 10.1371/journal.pone.0040020
109. Galvao J, Davis B, Tilley M, Normando E, Duchen MR, and Cordeiro MF. Unexpected low-dose toxicity of the universal solvent DMSO. FASEB J. (2014) 28:1317–30. doi: 10.1096/fj.13-235440
110. Verheijen M, Lienhard M, Schrooders Y, Clayton O, Nudischer R, Boerno S, et al. DMSO induces drastic changes in human cellular processes and epigenetic landscape in vitro. Sci Rep. (2019) 9:4641. doi: 10.1038/s41598-019-40660-0
111. Stella VJ, and He Q. Cyclodextrins. Toxicol Pathol. (2008) 36:30–42. doi: 10.1177/0192623307310945
112. Loftsson T, and Brewster ME. Pharmaceutical applications of cyclodextrins: basic science and product development. J Pharm Pharmacol. (2010) 62:1607–21. doi: 10.1111/j.2042-7158.2010.01030.x
113. di Cagno M. The potential of cyclodextrins as novel active pharmaceutical ingredients: a short overview. Molecules. (2016) 22:1. doi: 10.3390/molecules22010001
114. Braga SS. Cyclodextrins: emerging medicines of the new millennium. Biomolecules. (2019) 9:801. doi: 10.3390/biom9120801
115. Loftsson T, and Duchene D. Cyclodextrins and their pharmaceutical applications. Int J Pharm. (2007) 329:1–11. doi: 10.1016/j.ijpharm.2006.10.044
116. Davis ME, and Brewster ME. Cyclodextrin-based pharmaceutics: past, present and future. Nat Rev Drug Discov. (2004) 3:1023–35. doi: 10.1038/nrd1576
117. Ueda H, and Endo T. Large-Ring Cyclodextrins. Cyclodextrins and Their Complexes. Weinheim: WILEY-VCH (2006). p. 370–80.
118. Stella VJ, Rao VM, Zannou EA, and Zia VV. Mechanisms of drug release from cyclodextrin complexes. Adv Drug Del Rev. (1999) 36:3–16. doi: 10.1016/S0169-409X(98)00052-0
119. Connors KA. Population characteristics of cyclodextrin complex stabilities in aqueous solution. J Pharm Sci. (1995) 84:843–8. doi: 10.1002/jps.2600840712
120. Connors KA. The stability of cyclodextrin complexes in solution. Chem Rev. (1997) 97:1325–58. doi: 10.1021/cr960371r
121. Qi ZH, and Sikorski CT. Controlled delivery using cyclodextrin technology. In: Intelligent Materials for Controlled Release. Washington, DC: American Chemical Society (1999). p. 113–30.
122. Amidon GL, Lennernas H, Shah VP, and Crison JR. A theoretical basis for a biopharmaceutic drug classification: the correlation of in vitro drug product dissolution and in vivo bioavailability. Pharm Res. (1995) 12:413–20. doi: 10.1023/A:1016212804288
123. Ahr G, Voith B, and Kuhlmann J. Guidances related to bioavailability and bioequivalence: European industry perspective. Eur J Drug Metab Pharmacokinet. (2000) 25:25–7. doi: 10.1007/BF03190052
124. Loftsson T. Cyclodextrins and the biopharmaceutics classification system of drugs. J Incl Phenom Macrocycl Chem. (2002) 44:63–7. doi: 10.1023/A:1023088423667
125. Strickley RG. Solubilizing excipients in oral and injectable formulations. Pharm Res. (2004) 21:201–30. doi: 10.1023/B:PHAM.0000016235.32639.23
126. Braithwaite MC, Tyagi C, Tomar LK, Kumar P, Choonara YE, and Pillay V. Nutraceutical-based therapeutics and formulation strategies augmenting their efficiency to complement modern medicine: an overview. J Funct Foods. (2014) 6:82–99. doi: 10.1016/j.jff.2013.09.022
127. Lim YRI, Preshaw PM, Lim LP, Ong MMA, Lin HS, and Tan KS. Pterostilbene complexed with cyclodextrin exerts antimicrobial and anti-inflammatory effects. Sci Rep. (2020) 10:9072. doi: 10.1038/s41598-020-66031-8
128. Kolenbrander PE, Palmer RJJr, Periasamy S, and Jakubovics NS. Oral multispecies biofilm development and the key role of cell-cell distance. Nat Rev Microbiol. (2010) 8:471–80. doi: 10.1038/nrmicro2381
129. Han YW, Shi W, Huang GT, Kinder Haake S, Park NH, Kuramitsu H, et al. Interactions between periodontal bacteria and human oral epithelial cells: Fusobacterium nucleatum adheres to and invades epithelial cells. Infect Immun. (2000) 68:3140–6. doi: 10.1128/IAI.68.6.3140-3146.2000
130. Milward MR, Chapple IL, Wright HJ, Millard JL, Matthews JB, and Cooper PR. Differential activation of NF-kappaB and gene expression in oral epithelial cells by periodontal pathogens. Clin Exp Immunol. (2007) 148:307–24. doi: 10.1111/j.1365-2249.2007.03342.x
131. Lee P, and Tan KS. Fusobacterium nucleatum activates the immune response through retinoic acid-inducible gene I. J Dent Res. (2014) 93:162–8. doi: 10.1177/0022034513516346
Keywords: resveratrol, pterostilbene, periodontitis, cyclodextrins, antimicrobial, anti-inflammatory
Citation: Lim YRI, Preshaw PM, Lin H and Tan KS (2021) Resveratrol and Its Analogs as Functional Foods in Periodontal Disease Management. Front. Dent. Med. 2:636423. doi: 10.3389/fdmed.2021.636423
Received: 01 December 2020; Accepted: 02 February 2021;
Published: 24 February 2021.
Edited by:
George Grant, University of Aberdeen, United KingdomReviewed by:
Fabio Sczepanik, Pontifical Catholic University of Rio Grande do Sul, BrazilVijay Kumar Chava, Narayana Dental College and Hospital, India
Copyright © 2021 Lim, Preshaw, Lin and Tan. This is an open-access article distributed under the terms of the Creative Commons Attribution License (CC BY). The use, distribution or reproduction in other forums is permitted, provided the original author(s) and the copyright owner(s) are credited and that the original publication in this journal is cited, in accordance with accepted academic practice. No use, distribution or reproduction is permitted which does not comply with these terms.
*Correspondence: Kai Soo Tan, ZGVua3N0QG51cy5lZHUuc2c=