Lithium Chloride Exerts Differential Effects on Dentinogenesis and Osteogenesis in Primary Pulp Cultures
- Department of Craniofacial Sciences, University of Connecticut Health Center, Farmington, CT, United States
Wnt/β-catenin signaling is known to play essential roles in odontoblast differentiation and reparative dentin formation. Various Wnt activators including LiCl have been increasingly studied for their effectiveness to induce repair of the dentin-pulp complex. LiCl is a simple salt thought to activate Wnt/β-catenin signaling by inhibiting GSK3β. Previous in vitro and in vivo studies showed that LiCl increased odontoblast differentiation and enhanced reparative dentin formation. However, the underlying molecular and cellular mechanisms by which LiCl regulates odontoblast and osteoblast differentiation during reparative dentinogenesis are not well-understood. Our in vitro studies show that exposure of early dental pulp progenitors to LiCl increased the survival and the pool of αSMA+ progenitors, leading to enhanced odontoblast and osteoblast differentiation. The positive effects of LiCl in the differentiation of osteoblasts and odontoblasts from αSMA+ progenitors are mediated by Wnt/β-catenin signaling. Our results also showed that continuous and late exposure of dental pulp cells to LiCl increased the expression of odontoblast markers through Wnt/β-catenin signaling, and the number of odontoblasts expressing DMP1-Cherry and DSPP-Cerulean transgenes. However, unlike the early treatment, both continuous and late treatments decreased the expression of Bsp and the expression of BSP-GFPtpz transgene. These observations suggest that prolonged treatment with LiCl in more mature cells of the dental pulp has an inhibitory effect on osteoblast differentiation. The inhibitory effects of LiCl on osteogenesis and Bsp were not mediated through Wnt/β-catenin signaling. These observations suggest that the effects of LiCl, and GSK3β antagonists on reparative dentinogenesis involve multiple pathways and are not specific to Wnt/β-catenin signaling.
Introduction
The canonical Wnt signaling cascade (Wnt/β-catenin) begins with the binding of Wnt proteins to a heterodimeric receptor complex, consisting of frizzled (FZD) and low-density lipoprotein receptor-related protein (LRP) (1–3). Regulation of the Wnt/β-catenin signaling pathway depends on the abundance and subcellular localization of β-catenin. In the absence of Wnt proteins, β-catenin in the cytoplasm is degraded by the destruction complex consisting of adenoma polyposis coli (APC), axin, glycogen synthase kinase 3 (GSK-3), and casein kinase I (CKI) (1–3). Binding of Wnt proteins to these receptors, results in the activation of disheveled (DVL) leading to the inactivation of the degradation complex and accumulation of β-catenin in cytoplasm. After translocation into the nucleus, β-catenin associates with T cell factor/lymphoid enhancer factor (TCF/LEF) transcription factors and promotes the transcription of its target genes (1–3).
By controlling the expression of a broad spectrum of target genes, Wnt/β-catenin signaling regulates and coordinates a wide range of cell functions including stemness, proliferation, differentiation, inflammatory and immune responses, and the repair and regeneration of many tissues (1–4).
Several studies have implicated roles of Wnt/β-catenin signaling in reparative dentinogenesis (5–11). Wnt/β-catenin signaling is activated by injury to the dental pulp and participates in the dentin-pulp complex's stepwise healing process (5). Changes in the levels of Wnt/β-catenin signaling affect the rate and extent of reparative dentin formation (5, 8, 12–14).
Lithium chloride (LiCl) is a simple salt that is widely used in the treatment of various disorders (15–17). LiCl is thought to activate Wnt/β-catenin-mediated signaling through the inhibition of GSK3β (18) and is widely used in studies examining roles of Wnt/β-catenin signaling on multiple processes, including the repair and regeneration of the dentin-pulp complex (7, 19–22).
Studies in the pulp showed that the application of LiCl to injured and exposed pulp tissue induced reparative dentinogenesis (7, 19–22). In vitro studies showed that LiCl increased cell migration and mineralization of human dental pulp stem cells, and increased Dspp expression in human dental pulp cells (DPCs) (hDPSCs) (19).
Although the effects of LiCl on reparative dentin and odontoblast differentiation has been established, much remains to be understood about the underlying cellular events leading to increased reparative dentinogenesis. Therefore, to gain a better understanding of the roles of LiCl on odontoblast and osteoblast differentiation, we used well-characterized dental pulp cultures from a series of previously characterized reporter mice (23–25) and examined the response of cells at various stages of differentiation to LiCl.
Materials and Methods
Animal Models
All animal experimental protocols were approved by the Institutional Animal Care and use Committee of UConn Health Center. DSPP-Cerulean/DMP1-Cherry, BSP-GFPtpz, pOBCol2.3FP (referred to as 2.3-GFP), αSMA-GFP and non-transgenic mice have been previously described (23–25). All mice were maintained in the CD1 or C57BL/6 backgrounds. In all experiments, pulp cells from non-transgenic littermates served as negative control.
Cell Cultures, Digital Imaging, and Epifluorescence Analysis
Primary pulp cultures were prepared from the coronal pulp of first and second molars from 5- to 7-d-old mice and grown as described in previous publications (26, 27). The cultures were grown in the presence of vehicle (VH; 0.1% bovine serum albumin), various concentrations of LiCl (Sigma), and Dickkopf1 (DKK1) (R&D Systems), with or without simultaneous LiCl between days 3 and 21 (referred to as continuous exposure), days 7 and 21 (referred to as late exposure), and days 3 and 7 (referred to as early exposure).
Mineralization in live primary cultures was examined by Xylenol orange (XO) staining as described before (24). The mean fluorescence intensity of XO staining, DMP1-Cherry, and BSP-GFPtpz was measured as described before (24). Background fluorescence for XO, DMP1-Cherry and BSP-GFPtpz was measured using dental pulp cultures from non-transgenic littermates, and these values were subtracted from the respective XO and transgene measurements. Live or fixed cultures from reporter mice were imaged as described before (24) using Zeiss Axio Observer Z1 inverted microscope and appropriate filter cubes optimized for the detection of various fluorescent protein variants. The exposure times remained the same throughout the each set of experiments.
Immunocytochemistry
Pulp cultures were processed for immunocytochemistry with a 1:1,000 dilution of anti-GFP Alexa Fluor 488–conjugated antibody (Molecular Probes, Invitrogen) (25). DSPP-Cerulean+ cells were calculated as the ratio of cells stained with anti-GFP antibody (DSPP-Cerulean+ cells) to the total number of Hoechst+ cells (26, 28). Primary dental pulp cultures derived from the transgenic littermates without the addition of anti-GFP antibody served as controls.
RNA Extraction and Analysis
Total RNA was prepared with TRIzol Reagent (Invitrogen) according to the manufacturer's instructions. After DNase treatment, RNA samples were processed for quantitative polymerase chain reaction (qPCR) analysis with specific primers (Supplementary Figure 1) purchased from Applied Biosystems (28). Gene expression was examined by qPCR analysis using 2−DDcT method. For TaqMan qPCR reactions, 9 ng of cDNA was combined with 5 μl of TaqMan universal master Mix (Applied Biosystems, Nranchburg, NJ), 2.5 μl H2O and 0.5 μl TaqMan primers (total 10 μl). All qPCR reactions were run using Biorad PCR System under the following conditions: 50°C for 2 min, 95°C for 10 min, and 40 cycles with denaturation at 95°C for 15 s and extension at 60°C for 1 min. We defined the acceptable range of CT values representing gene expression to be between 10 and 35 cycles, according to manufacturer's recommendations.
Flow Cytometric Analysis
Single-cell suspensions from pulp cultures were processed for fluorescence-activated cell sorting (FACS) analysis at different time points using gating strategies shown in Supplementary Figure 2. Cells growing under proliferation culture conditions were prepared by mild 0.05% trypsin/EDTA (Invitrogen, USA) digestion, while cells growing under mineralization culture conditions were prepared by incubation of cells in enzymatic solution containing 0.05% trypsin/EDTA and 2 U/ml Collagenase P for 10–20 min, followed by centrifugation. Cells were then re-suspended in 300–400 μl of staining medium (1x HBSS, 2% FBS, 10 mM HEPES, in distilled H2O, pH 7.2) containing 1.0 μg/ml FVD eFluor780 (eBiosciences) and strained through a 70 μm strainer to obtain single-cell suspension. Approximately 20,000–100,000 live cells/sample were collected by a BD™ LSR-II FACS cytometer (BD Biosciences, San Jose, CA). Pulp cells from non-transgenic littermates served as a negative control for transgene expression in all experiments.
Proliferation and Apoptosis Assays
The proliferative cells were detected by incubation of cultures with 10 μM EdU (5-ethynyl-2′-deoxyuridine) for 4 h and Click-iT Plus Flow Cytometry Assay kit (Invitrogen) according to the manufacturer's instructions. The apoptotic cells were examined using rabbit cleaved caspase 3 antibody (1:800; Cell Signaling Technology 9661S) for 1 h and goat anti-rabbit Alexa Fluor 594 for 30 min. The number of proliferative and apoptotic cells was calculated by FACS analysis using a BD LSR-II FACS cytometer (BD Biosciences). Pulp cells processed in the absence of EdU/cleaved caspase 3 antibody and cells from non-transgenic littermates served as negative controls in all experiments.
Statistical Analysis
Analysis was performed by GraphPad Prism 8 software (GraphPad Software) using 1-way analysis of variance (ANOVA). Values in all experiments represented mean ± SEM of at least 3 independent experiments, and P ≤ 0.05 was considered statistically significant.
Results
Effects of LiCl on Wnt Responsive Cells
We first examined the effects of LiCl on the Wnt-responsive dental pulp cells by examining the levels of Axin2 expression (29) by quantitative polymerase chain reaction (qPCR) analysis.
qPCR analysis showed marked concentration-dependent increases in Axin2 expression between 4 and 24 h in the LiCl-treated cultures as compared to the VH-treated and NaCl-treated controls (Figure 1A). Conversely, DKK1-treated cultures showed concentration-dependent decreases in Axin2 expression as compared to controls (Figure 1B). The changes with the various concentrations of LiCl and DKK1 were detectable at 4 h. Based on these observations, we selected 10 mM LiCl and 50 ng/ml DKK1 for all the following experiments.
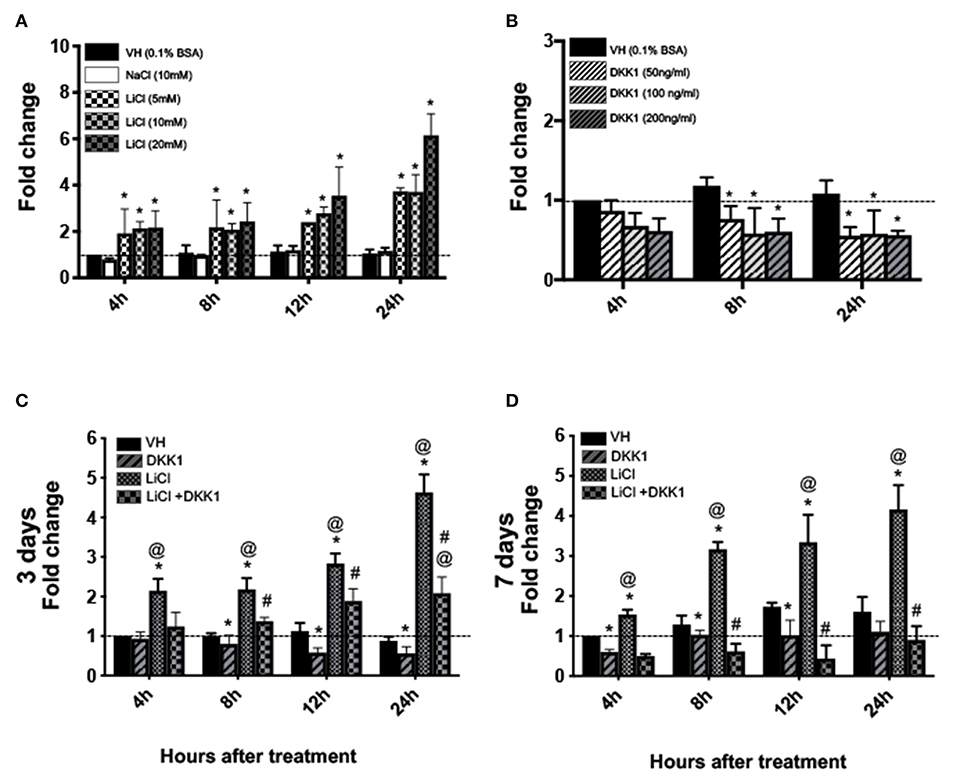
Figure 1. Effects of LiCl on Axin2-expression in primary pulp cultures cultures. (A,B) Graphs showing the dose-dependent changes in the expression of Axin2 in cultures treated with Vehicle (VH, 0.1% BSA), (A) NaCl (10 mM), LiCl (5, 10, and 20 mM), and (B) DKK1 (50, 100, and 200 ng/ml) between 4 and 24 h after treatment at various times at day 3. (C,D) Graphs showing changes in the expression of Axin2 between 4 and 24 h in cultures with various treatments at day 3 (C) and day 7 (D). Expression of Axin2 was normalized to 4 h of the VH-treated cultures, which is arbitrarily set to 1 and is indicated by the dashed line. Results represent mean ± SEM of at least 3 independent experiments. Analysis was performed using 1-way analysis of variance. *P ≤ 0.05 relative to VH at each time point; @P ≤ 0.05 relative to DKK1 at each time point; #P ≤ 0.05 relative to LiCl at each time point.
The addition of 10 mM of LiCl to pulp cultures at days 3 and 7, induced an ~2 to 4.5-fold increase in Axin2 levels (Figures 1C,D). DKK1 decreased these increases (Figures 1C,D). Thus, primary pulp cultures contain Wnt-responsive cells and increased expression of Axin2 by LiCl is mediated by Wnt/β-catenin signaling.
Effects of Continuous Exposure to LiCl on Primary Dental Pulp Cultures
The effects of continuous exposure to LiCl on the mineralization and differentiation of pulp cells in vitro were examined by addition of LiCl and DKK1 with or without LiCl between days 3 and 21 (Figures 2A,B).
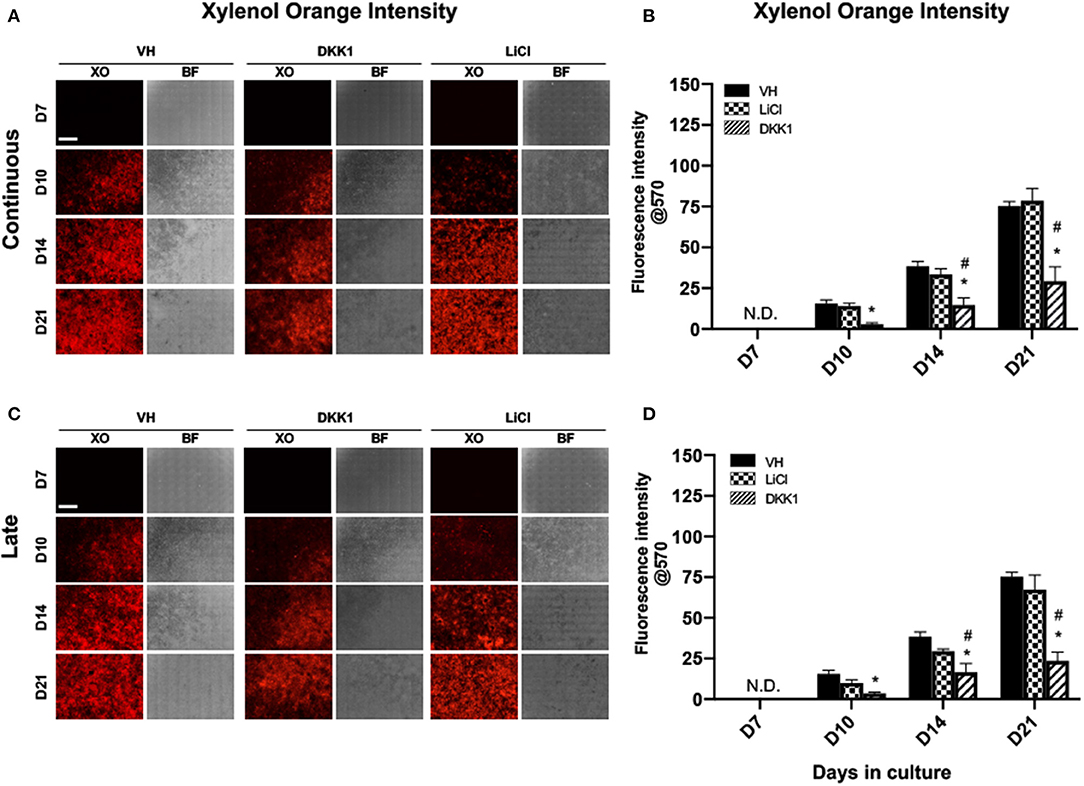
Figure 2. Effects of LiCl on the mineralization of primary dental pulp cultures. Primary pulp cultures were treated with VH (0.1% BSA), DKK1 (50 ng/ml) or LiCl (10 mM) between days 3 and 21 (continuous, A,B), and between days 7 and 21 (late, C,D). (A,C) Representative images of the same areas in cultures treated with VH, DKK1, and LiCl continuously (A) and late (C). Cultures were analyzed under brightfield (BF) and epifluorescent light using TRITC red filter for detection of XO staining. Scale bar = 2 mm. (B,D) Graphs showing changes in the intensity of XO staining (in absolute values) in cultures treated with VH, DKK1, and LiCl continuously (B), and late (D). Continuous and late exposure to LiCl had no significant effect on the intensity of XO. Continuous and late exposure to DKK1 decreased the intensity of XO at days 10, 14, and 21 as compared to VH-treated and LiCl-treated cultures. Results represent mean ± SEM of at least 3 independent experiments. Analysis was performed using 1-way analysis of variance. *P ≤ 0.05 relative to VH at each time point; #P ≤ 0.05 relative to LiCl at each time point. XO, Xylenol orange; N.D, not detected.
The extent of mineralization of LiCl-treated cultures was not different from VH-treated cultures. However, continuous exposure to DKK1 markedly decreased the extent of mineralization compared to VH-treated and LiCl-treated cultures (Figures 2A,B).
Continuous LiCl treatment induced marked increases in the expression of Dmp1 and Dspp between days 10 and 17 that were decreased by DKK1 (Figures 3A,B). However, continuous treatment of the cultures with both LiCl and DKK1 resulted in similarly decreased levels of Bsp (Figure 3C). These findings showed that LiCl increased the expression of Dmp1 and Dspp (markers of odontoblast differentiation) but decreased the expression of Bsp (a marker of osteoblasts) during the mineralization phase of growth in primary pulp cultures. The decreases in the LiCl-induced changes in Dmp1 and Dspp by DKK1 indicated the roles of Wnt/β-catenin signaling in LiCl-induced changes. On the other hand, the LiCl-induced decreases in Bsp were not regulated by Wnt/β-catenin signaling.
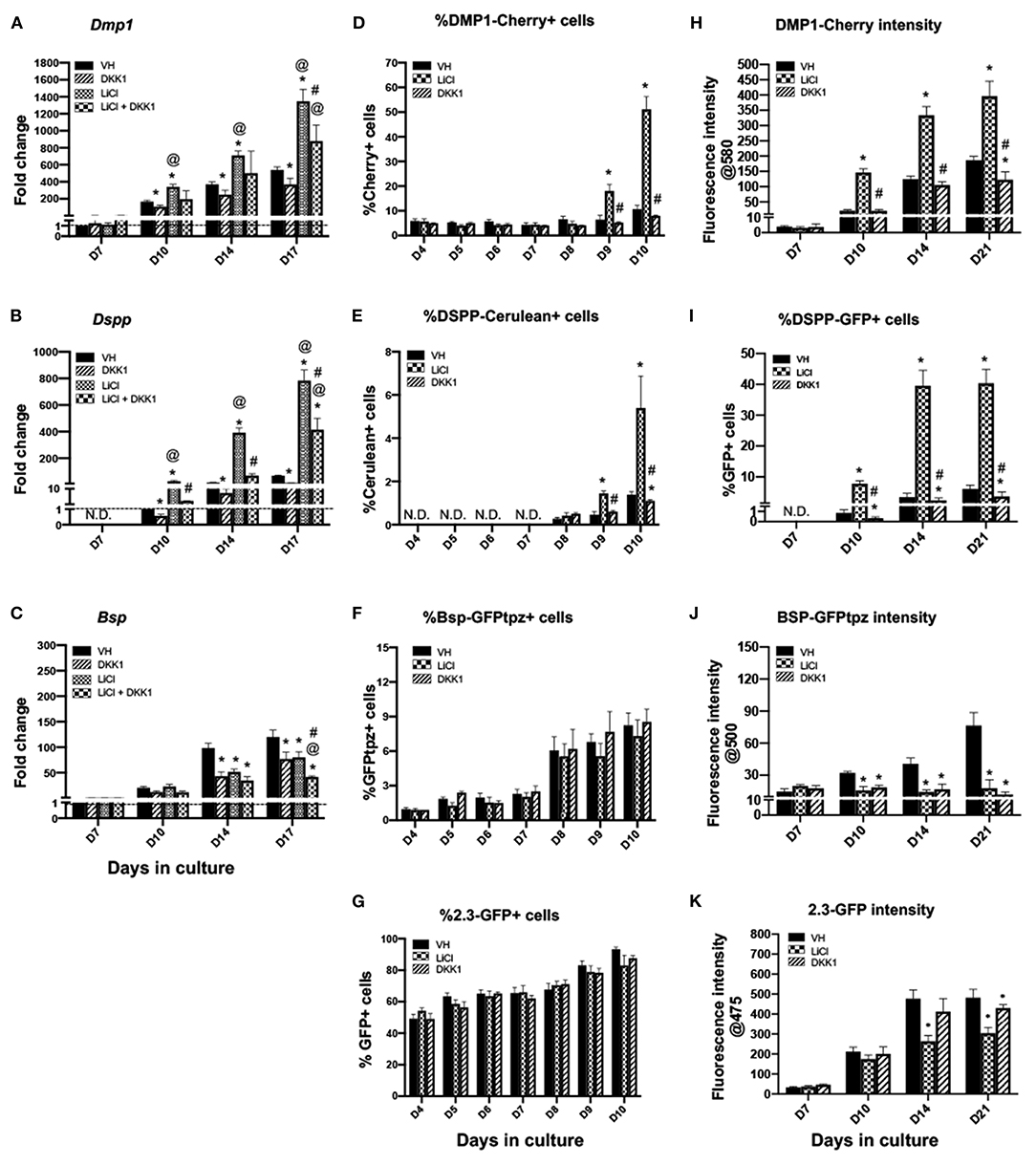
Figure 3. Effects of continuous exposure to LiCl on the expression of differentiation markers and tissue- and stage-specific reporters in primary dental pulp cultures. Primary pulp cultures were treated continuously from days 3 to 21 with VH (0.1% BSA), DKK1 (50 ng/ml), LiCl (10 mM) or simultaneous LiCl (10 mM) and DKK1 (50 ng/ml). (A–C) Graphs showing changes in the expression of Dmp1 (A), Dspp (B) and Bsp (C) in cultures treated continuously with VH, LiCl, and DKK1 alone or with simultaneous LiCl from days 3 to 17. Continuous exposure to LiCl showed significant increases in Dmp1 (A) and Dspp (B) at days 10–17, which were significantly decreased by DKK1 treatment alone. DKK1 treatment decreased the LiCl-induced increases in Dmp1 and Dspp. However, continuous LiCl treatment, as well as DKK1 treatment decreased Bsp expression. The LiCl-induced decreases were not reversed by DKK1 (C). Expression of Dmp1 and Bsp was normalized to day 7 and Dspp was normalized to day 10 of the VH-treated cultures, which is arbitrarily set to 1 and is indicated by the dashed line. (D–G) Graphs showing the changes in the percentage of cells expressing DMP1-Cherry (D), DSPP-Cerulean (E), BSP-GFPtpz (F), and 2.3-GFP (G) transgenes by FACS analysis between days 4 and 10 (every 24 h following the start of continuous treatment). LiCl-treated cultures show significantly increased percentages in DMP1-Cherry+ cells and DSPP-Cerulean+ cells at days 9 and 10. DKK1 treated cultures show decreased percentages of DMP1-Cherry+ and DSPP-Cerulean+ cells that were significant compared to VH treated controls, as well as LiCl-treated cultures (D,E). LiCl treatment decreased the percentage of BSP-GFPtpz+ cells and 2.3-GFP+ cells while DKK1 treatment showed no effects (F,G). (H–K) Graphs showing the changes in the fluorescence intensity of DMP1-Cherry (H), BSP-GFPtpz (J), and 2.3-GFP (K) transgenes by fluorometric analysis, as well as the percentage of DSPP-Cerulean+ cells (I), detected by immunocytochemistry following continuous treatment. Continuous exposure to LiCl showed significant increases in the fluorescence intensity of DMP1-Cherry (H) and the percentage of DSPP-GFP+ cells (I) at days 10–21. LiCl treatment significantly decreased the intensity of BSP-GFPtpz (J) and 2.3-GFP (K) at days 14–21. DKK1 treatment significantly decreased the intensity of DMP1-Cherry and BSP-GFPtpz, as well as the percentage of DSPP-GFP+ cells, at days 10–21. Results in all graphs represent mean ± SEM of at least 3 independent experiments. Analysis was performed using 1-way analysis of variance. *P ≤ 0.05 relative to VH at each time point; @P ≤ 0.05 relative to DKK1 at each time point; #P ≤ 0.05 relative to LiCl at each time point. N.D, not detected.
Primary pulp cultures from 2.3-GFP, DSPP-Cerulean/DMP1-Cherry and BSP-GFPtpz transgenic reporter mice were used to examine if the effects of LiCl on gene expression were related to changes in their levels of transcription and/or changes in the number of cells expressing these markers (Figures 3D–K). In these experiments, FACS analysis was used to quantify the percentages of cells expressing these transgenes 1–7 days after treatment (days 4–10 of culture) (Figures 3D–G) using gating strategies shown in Supplementary Figure 2.
No significant changes were observed in the percentage of cells expressing the various transgenes between days 4 and 7 in the LiCl- and DKK1-treated cultures compared to VH-treated cultures (Figures 3D–G). On the other hand, following the induction of mineralization, there were marked increases in the percentages of DMP1-Cherry+ and DSPP-Cerulean+ cells at days 9 and 10 in the LiCl-treated cultures. DKK1-treated cultures demonstrated significant decreases (Figures 3D,E). LiCl- and DKK1-treated cultures showed no significant changes in the percentages of BSP-GFPtpz+ cells (Figure 3F) and 2.3-GFP+ cells (Figure 3G).
The changes in the transgene expression at later time points (days 10–21) were analyzed by fluorometric analysis and immunocytochemistry (Figures 3H–K, 4A) because of the difficulties in obtaining single cells in mineralized cultures. Between days 10 and 21, LiCl-treated cultures showed continuous and marked increases in the intensity of DMP1-Cherry (Figure 3H), and in the percentage of DSPP-GFP+ cells (Figure 3I) compared to VH-treated cultures. DKK1-treated cultures showed continuous and marked decreases in the intensity of DMP1-Cherry (Figure 3H) and in the percentage of DSPP-GFP+ cells (Figure 3I). LiCl-and DKK1-treated cultures showed continuous decreases in the intensity of BSP-GFPtpz (Figure 3J) and 2.3-GFP (Figure 3K) compared to VH-treated cultures.
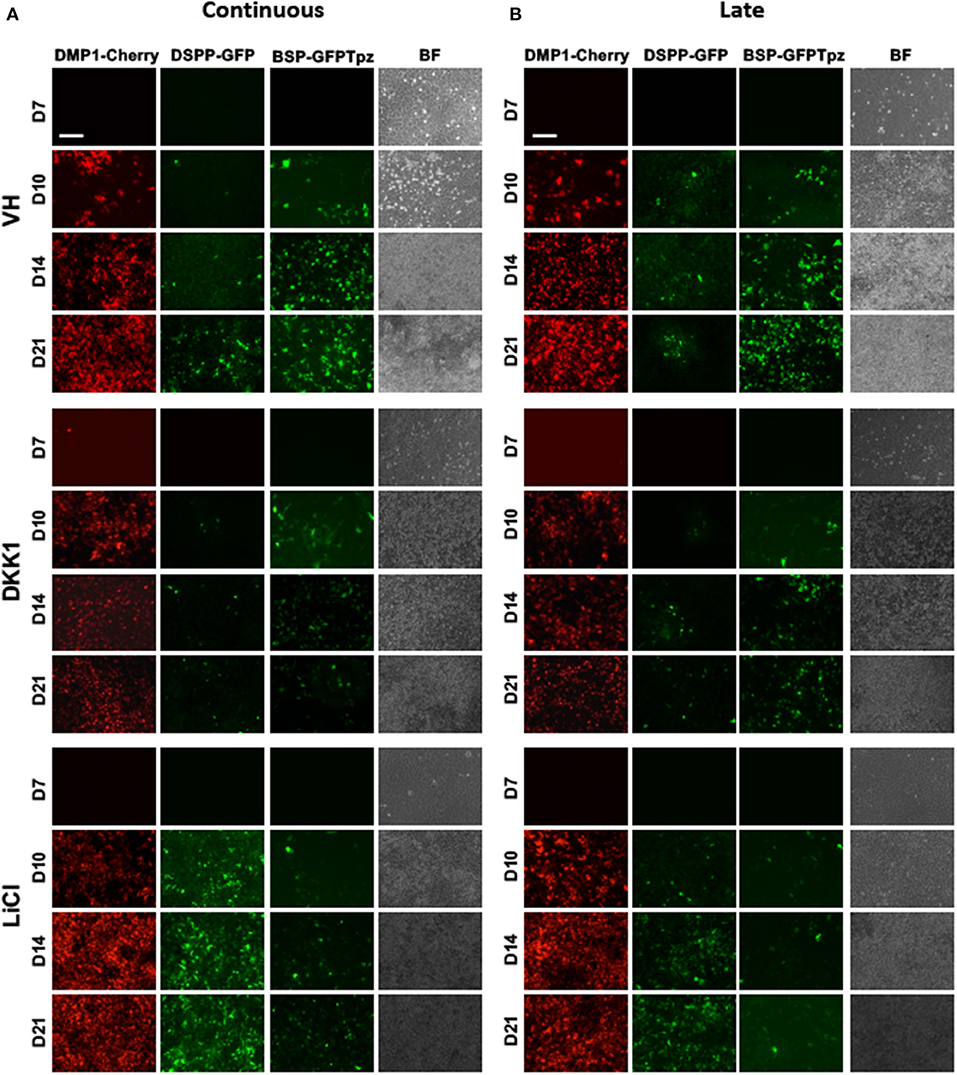
Figure 4. Effects of continuous and late exposure of LiCl and DKK1 on tissue- and stage-specific reporters by epifluorescence imaging. Primary pulp cultures were treated continuously between days 3 and 21 (A) and late between days 7 and 21 (B) with VH (0.1% BSA), DKK1 (50 ng/ml) and LiCl (10 mM). (A,B) Representative images of the cultures from DMP1-Cherry/DSPP-Cerulean and BSP-GFPtpz transgenic mice treated with VH, DKK1, and LiCl continuously (A), and late (B) analyzed under brightfield (BF) and epifluorescent light for the detection of DMP1-Cherry, DSPP-GFP, and BSP-GFPtpz. Continuous and late exposures to LiCl increased DMP1-Cherry and DSPP-GFP expression, which were decreased in DKK1 treated cultures. Continuous and late LiCl and DKK1 treatments decreased BSP-GFPtpz expression. Scale bar = 200 μm.
Effects of Late Exposure to LiCl on Primary Dental Pulp Cultures
Since continuous LiCl treatment demonstrated a lack of significant changes in the various differentiation markers during the first 7 days (proliferation phase), it may suggest that LiCl affects the differentiation of more mature cells. To test this possibility, we examined the effects of LiCl treatment during the mineralization of primary pulp cultures referred to as late treatment (Figures 2, 4, 5).
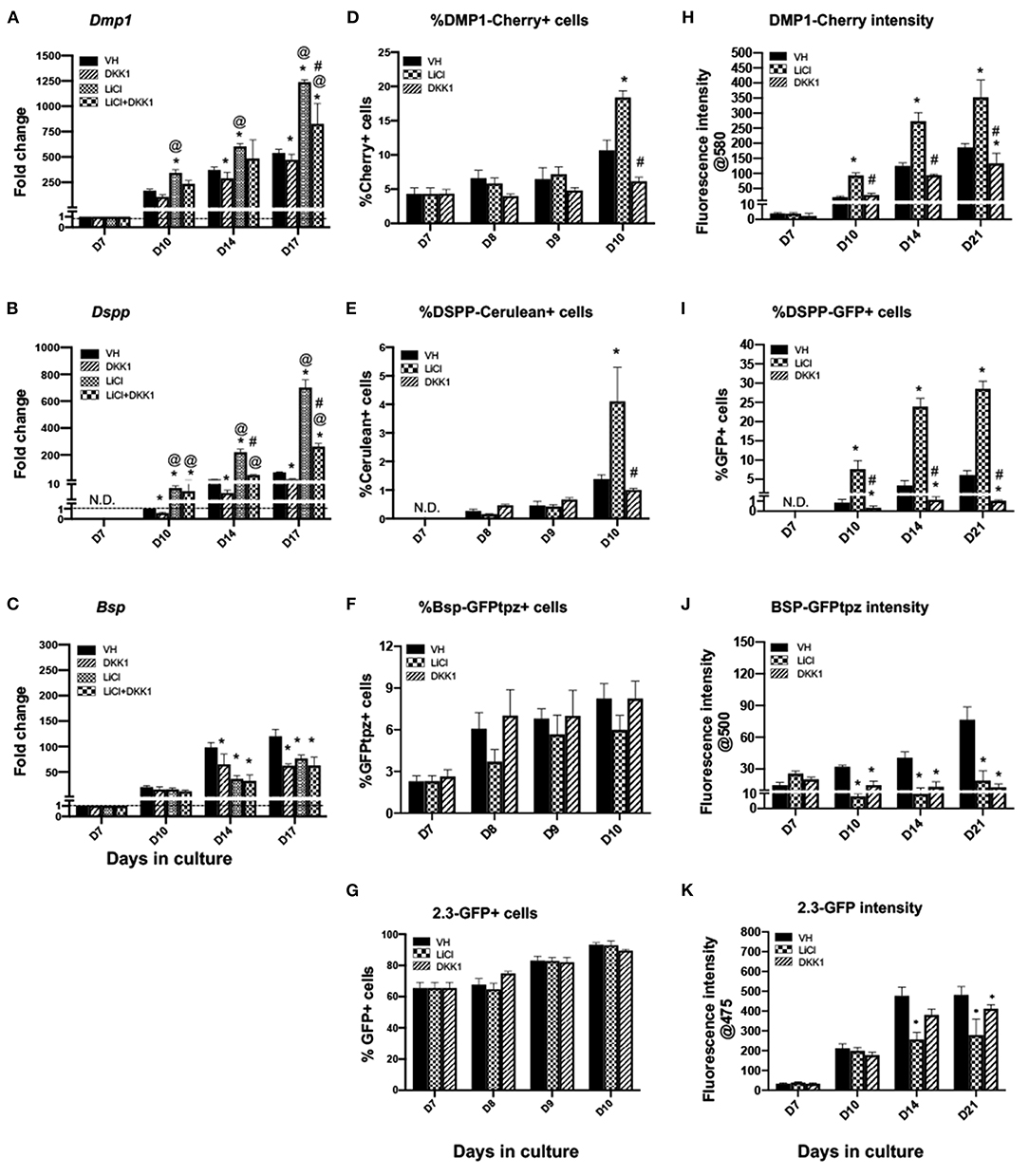
Figure 5. Effects of late exposure to LiCl on the expression of differentiation markers and tissue- and stage-specific reporters in primary dental pulp cultures.Primary pulp cultures were treated late from days 7 to 21 with VH (0.1% BSA), LiCl (10 mM), DKK1 (50 ng/ml) or simultaneous LiCl (10 mM) and DKK1 (50 ng/ml). (A–C) Graphs showing changes in the expression of Dmp1, Dspp, and Bsp in cultures treated with VH, LiCl, and DKK1 alone or with simultaneous LiCl. Late exposure to LiCl showed significant increases in Dmp1 (A) and Dspp (B) at days 10–17, which were significantly decreased by DKK1 treatment alone. DKK1 treatment also significantly decreased the LiCl-induced increases in Dmp1 and Dspp to levels higher than control. However, late LiCl treatment, as well as DKK1 treatment, significantly decreased Bsp expression. The LiCl-induced decreases were not reversed by DKK1 (C). Expression of Dmp1 and Bsp was normalized to day 7 and Dspp was normalized to day 10 of the VH-treated cultures, which is arbitrarily set to 1 and is indicated by the dashed line. (D–G) Graphs showing the changes in the percentage of cells expressing DMP1-Cherry (D), DSPP-Cerulean (E), BSP-GFPtpz (F), and 2.3-GFP (G) transgenes by FACS analysis between days 7 and 10 (every 24 h following the start of late treatment). LiCl-treated cultures show significantly increased the percentages of DMP1-Cherry+ cells and DSPP-Cerulean+ cells at day 10. DKK1 treated cultures show decreased percentages of DMP1-Cherry+ and DSPP-Cerulean+ cells that were significant compared to LiCl-treated cultures (D,E). LiCl and DKK1 treatment showed no statistically significant effects on the percentage of BSP-GFPtpz+ cells and 2.3-GFP+ cells (F,G). (H–K) Graphs showing the changes in the fluorescence intensity of DMP1-Cherry (H), BSP-GFPtpz (J), and 2.3-GFP (K) transgenes, as well as the percentage of DSPP-Cerulean+ cells (I), following late treatments. Late exposure to LiCl showed significant increases in the fluorescence intensity of DMP1-Cherry (H) and the percentage of DSPP-GFP+ cells (I) at days 10–21. DKK1 treatment significantly decreased the intensity of DMP1-Cherry and the percentage of DSPP-GFP+ cells compared to VH-treated controls, as well as LiCl-treated cultures. LiCl and DKK1 treatment significantly decreased the intensity of BSP-GFPtpz (J) and 2.3-GFP (K) at days 10–21 compared to VH-treated controls. Results represent mean ± SEM of at least 3 independent experiments. Analysis was performed using 1-way analysis of variance. *P ≤ 0.05 relative to VH at each time point; @P ≤ 0.05 relative to DKK1 at each time point; #P ≤ 0.05 relative to LiCl at each time point. N.D, not detected.
Late exposure of pulp cultures to LiCl (days 7–21) resulted in changes very similar to continuous treatment, albeit at a lower magnitude. LiCl did not have significant effects on the extent of mineralization (Figures 2C,D), increased the expression levels of Dmp1 and Dspp, but decreased the levels of Bsp (Figures 5A–C). Similar to continuous treatment, late treatment increased in the percentages of DMP1-Cherry+ cells (Figure 5D) and the intensity of DMP1-Cherry expression (Figure 5H) and increased the number of DSPP-Cerulean+ cells (Figures 5E,I).
Late treatment did not have significant effects in the percentage of BSP-GFPtpz+ cells and 2.3-GFP+ cells (Figures 5F,G) between days 7 and 10 and decreased the intensity of BSP-GFPtpz and 2.3-GFP expression (Figures 5J,K).
Effects of LiCl on Early Progenitors' Primary Dental Pulp Cultures
Our previous observations showed that αSMA+ perivascular cells in dental pulp are progenitors capable of giving rise to odontoblasts and osteoblasts during reparative dentinogenesis in vivo (30). Therefore, we examined the response αSMA+ perivascular cells to LiCl and DKK1 treatment.
In these experiments, the cultures from αSMA-GFP+ transgenic mice were treated with LiCl and DKK1 between days 3 and 7. The effects on the percentage of αSMA-GFP+ cells, their proliferation and apoptosis were examined by FACS analysis using gating strategies shown in Supplementary Figure 2 (Tables 1A–E).
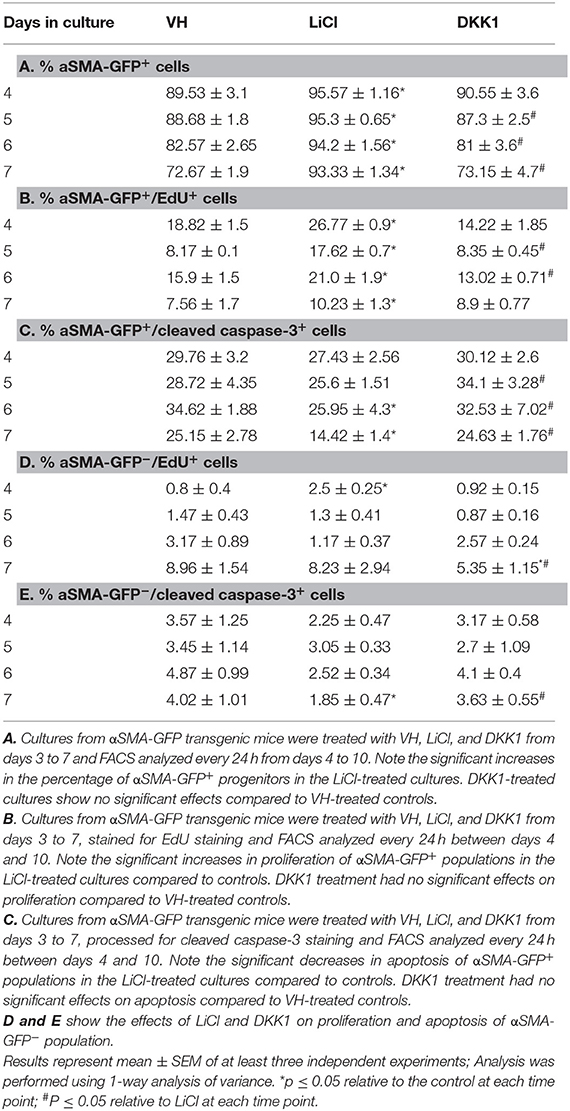
Table 1. Effects of early exposure to LiCl on the percentage of αSMA-GFP+ cells and their survival in primary dental pulp cultures.
LiCl-treated cultures showed markedly increased percentage of αSMA-GFP+ population as compared to VH-and DKK1-treated cultures (Table 1A). Furthermore, LiCl increased proliferation (Table 1B) and decreased apoptosis (Table 1C) in the αSMA-GFP+ population. These changes were not detected in the αSMA-GFP− population (Tables 1D,E)
Early and limited treatment with LiCl induced changes during differentiation similar to continuous and late treatment with a few important differences (Figures 6, 7). Unlike the continuous and late treatment, early treatment increased the extent of mineralization (Figures 6A,B). Early treatment also transiently increased the percentage of 2.3-GFP+ cells at day 8 (Figure 7G), unlike continuous and late treatments, which had no significant effects. The effects of early treatment with LiCl on the expression of Dmp1 (Figure 7A), Dspp (Figure 7B), percentages and intensities of DMP1-Cherry+ and DSPP-GFP+ cells (Figures 7D,E,H,I) were similar to the other treatments, but at lower levels. However, early treatment induced increases in the expression of Bsp and BSP-GFPtpz (Figures 7C,F,J).
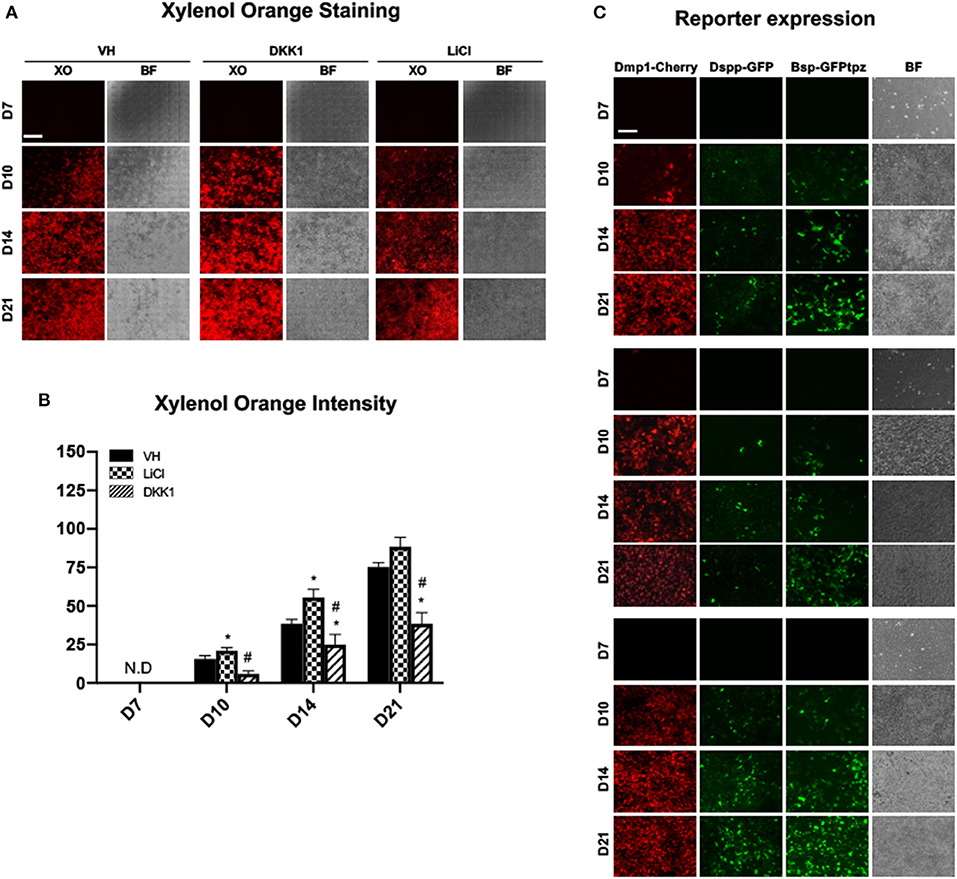
Figure 6. Effects of early exposure to LiCl on mineralization and tissue- and stage-specific reporters in primary dental pulp cultures. Primary pulp cultures were treated with VH (0.1% BSA), DKK1 (50 ng/ml) or LiCl (10 mM) during the proliferation phase of in vitro growth between days 3 and 7. (A) Representative images of the same areas in cultures treated with VH, DKK1, and LiCl analyzed under brightfield (BF) and epifluorescent light using TRITC red filter for detection of XO staining. Scale bar = 2 mm. (B) Graphs showing changes in the intensity of XO staining (in absolute values) in cultures treated with VH, DKK1, and LiCl. Early exposure increased XO staining at days 10 and 14. The intensity of XO staining was measured at 570/610-nm wavelength (excitation/emission) and at a gain of 80. Background fluorescence for XO was measured using cultures without the addition of ascorbic acid and β-glycerophosphate, which lack mineralization potential. The background fluorescence values were subtracted from respective XO measurements. Results represents mean ± SEM of at least 3 independent experiments. Analysis was performed using 1-way analysis of variance. *P ≤ 0.05 relative to VH at each time point; #P ≤ 0.05 relative to LiCl at each time point. XO, Xylenol orange; N.D, not detected. (C) Representative images of the cultures from DMP1-Cherry/DSPP-Cerulean and BSP-GFPtpz transgenic mice treated with VH, DKK1, and LiCl and analyzed under brightfield (BF) and epifluorescent light for the detection of DMP1-Cherry, DSPP-GFP, and BSP-GFPtpz. Early exposures to LiCl increased DMP1-Cherry and DSPP-GFP expression, which were decreased in DKK1 treated cultures. Early LiCl and DKK1 treatments increased and decreased BSP-GFPtpz expression, respectively. Scale bar = 200 μm. XO, Xylenol orange; N.D, not detected; VH, vehicle; GFP, green fluorescent; GFPtpz, topaz variant of GFP.
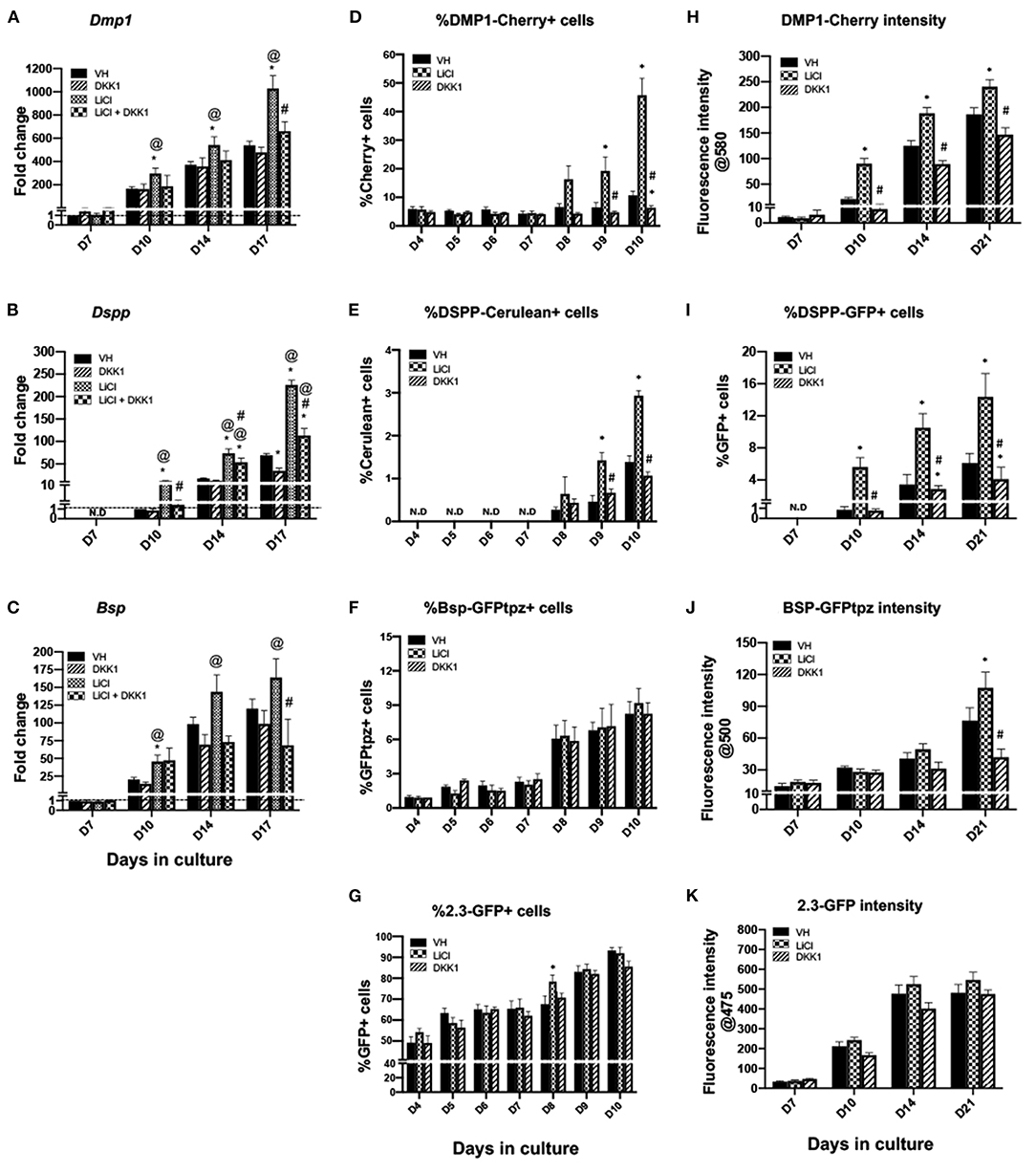
Figure 7. Effects of early exposure to LiCl on the expression of differentiation markers in primary dental pulp cultures. Primary pulp cultures were treated early from days 3 to 7 with VH (0.1% BSA), LiCl (10 mM), DKK1 (50 ng/ml) or simultaneous LiCl (10 mM) and DKK1 (50 ng/ml). (A–C) Graphs showing changes in the expression of Dmp1, Dspp, and Bsp in cultures. Early exposure to LiCl showed significant increases in Dmp1 (A), Dspp (B), and Bsp (C) at days 10–17, which were decreased by DKK1 treatment alone. DKK1 treatment also significantly decreased the LiCl-induced increases in Dmp1, Dspp, and Bsp. Expression of Dmp1 and Bsp was normalized to day 7 and Dspp was normalized to day 10 of the VH-treated cultures, which is arbitrarily set to 1 and is indicated by the dashed line. (D–G) Graphs showing the changes in the percentage of cells expressing DMP1-Cherry (D), DSPP-Cerulean (E), BSP-GFPtpz (F), and 2.3-GFP (G) transgenes by FACS analysis. LiCl treatment significantly increased percentages in DMP1-Cherry+ cells and DSPP-Cerulean+ cells at days 9–10, while DKK1-treated cultures show significantly decreased percentages of DMP1-Cherry+ and DSPP-Cerulean+ cells (D,E). LiCl and DKK1 treatment showed no effects on the percentage of BSP-GFPtpz+ cells and 2.3-GFP+ cells (F,G). (H–K) Graphs showing the changes in the fluorescence intensity of DMP1-Cherry (H), BSP-GFPtpz (J), and 2.3-GFP (K) transgenes, as well as the percentage of DSPP-Cerulean+ cells (I) following early treatment with VH, LiCl, and DKK1 from days 3 to 7. Early exposure to LiCl showed significant increases in the fluorescence intensity of DMP1-Cherry (H), BSP-GFPtpz (J), 2.3-GFP (K) and the percentage of DSPP-GFP+ cells (I) at days 10–21. DKK1 treatment significantly decreased the intensity of DMP1-Cherry, BSP-GFPtpz, and 2.3-GFP as well as the percentage of DSPP-GFP+ cells, at days 10–21. Results represent mean ± SEM of at least 3 independent experiments; Analysis was performed using 1-way analysis of variance. *P ≤ 0.05 relative to VH at each time point; @P ≤ 0.05 relative to DKK1 at each time point; #P ≤ 0.05 relative to LiCl at each time point. N.D, not detected.
Discussion
Using a well-characterized primary pulp culture from various reporter mice, we investigated the underlying mechanisms regulating the stimulatory effects of LiCl on odontoblast and osteoblast differentiation. Our results showed that exposure of pulp cultures to LiCl during the proliferation phase of in vitro growth and before induction of differentiation increased the pool of αSMA+ progenitors by regulating their survival. This limited and early treatment also transiently increased the number of pre-odontoblasts expressing 2.3-GFP that rapidly differentiated into odontoblasts expressing DMP1-Cherry and DSPP-Cerulean transgenes and osteoblasts expressing BSP-GFPtpz. Our results also showed that the LiCl-induced changes in the expression of Dspp, Dmp1, and Bsp in these early progenitors were reversed by DKK1. DKK1 is a secreted protein that, by binding to LRP5/6 and Kremen receptors, functions as a negative regulator of Wnt/β-catenin signaling (31). These observations indicated that the positive effects of LiCl in the differentiation of osteoblasts and odontoblasts from αSMA+ progenitors are mediated by Wnt/β-catenin signaling. The effects of LiCl on early progenitors is very similar to the effects of WNT3a (27).
Our results also showed that continuous and late exposure of dental pulp cells to LiCl increased the expression of odontoblast markers through Wnt/β-catenin signaling and the number of odontoblasts expressing DMP1-Cherry and DSPP-Cerulean transgenes. However, unlike the early treatment, both continuous and late treatments decreased the expression of Bsp and the expression of BSP-GFPtpz transgene. These observations suggest that prolonged treatment by LiCl in more mature cells in the dental pulp has an inhibitory effect on osteoblast differentiation. The LiCl-induced decreases in the levels of Bsp during the mineralization phase of in vitro growth were not reversed by DKK1, indicating that the inhibitory effects of LiCl on osteogenesis and Bsp are not mediated through Wnt/β-catenin signaling. The inhibitory effects of prolonged exposure of pulp cells to LiCl are different from the stimulatory effects of WNT3a on pulp cells (27).
Although the stimulatory effects of Wnt/β-catenin signaling on osteoblast differentiation has previously been well-established (32), the effects of LiCl on osteogenesis remains controversial. Several studies have shown the positive effects of LiCl on osteogenic differentiation. LiCl enhanced osteogenic differentiation by bone marrow-derived mesenchymal stem (33), human periodontal ligament fibroblasts (34), and stem cells from the apical papilla (17).
In contrast, several studies reported inhibitory effects of lithium on osteogenic differentiation. LiCl inhibited the osteogenic differentiation of MC3T3-E1 pre-osteoblasts and a pluripotent mesenchymal cell line C2C12 (35, 36), bone marrow-derived mesenchymal stem cells (37), cementoblasts (38), SHED (39) and osteogenesis in the cultured embryonic palatal shelves (40). In both MC3T3-E1 and C2C12 cell lines, LiCl inhibition of osteogenesis was mediated through BMP2/SMAD signaling pathway (35). Additionally, in MC3T3-E1 cells, the negative effects of LiCl osteogenesis were mediated through GSK3b, whereas in C2C12 cells, these effects were independent of GSK3-mediated signaling (35).
Furthermore, LiCl modulates the activity of several other pathways, including inhibiting inositol monophosphates (IMPase) in the phosphatidylinositol (PI) signaling pathways, with essential positive roles in osteoblast differentiation and bone formation (41, 42). These observations suggest that the inhibitory effects of LiCl on osteogenesis is complex and may involve more than one pathway.
Considering the effects of LiCl on multiple signaling pathways, the results of the studies in which LiCl has been used to block the GSK3 activity should be interpreted carefully. GSK3β, a serine/threonine kinase, modulates many signaling pathways and transcription factors (43), and the effects of LiCl on reparative dentinogenesis cannot be considered specific to Wnt/β-catenin signaling.
A few studies have shown that reparative dentinogenesis induced by LiCl and small-molecule GSK3 inhibitor drugs following pulp exposure resulted in the formation of reparative dentin that was more similar to physiological dentin in tubularity and chemical composition (7, 8). Our observations suggest that the improved reparative dentin structure in these studies as compared to osteodentin is related to LiCl-mediated inhibition of osteogenesis.
Data Availability Statement
The raw data supporting the conclusions of this article will be made available by the authors, without undue reservation.
Ethics Statement
The animal study was reviewed and approved by Institutional Animal Care and use Committee of UConn Health Center.
Author Contributions
AV and MM contributed to conception, design, data acquisition, analysis, or interpretation, drafted and revised the manuscript. All authors gave final approval and agree to be accountable for all aspects of the work.
Funding
This work was supported by grants R01-DE016689 and R90-DE022526 from the National Institutes of Health (National Institute of Dental and Craniofacial Research).
Conflict of Interest
The authors declare that the research was conducted in the absence of any commercial or financial relationships that could be construed as a potential conflict of interest.
Acknowledgments
The authors thank all individuals who provided reagents, valuable input, and technical assistance in various aspects of this study, including Barbara Rodgers and members of the Molecular Core Facility and Flow Cytometry Facility at the University of Connecticut Health Center.
Supplementary Material
The Supplementary Material for this article can be found online at: https://www.frontiersin.org/articles/10.3389/fdmed.2021.649500/full#supplementary-material
References
1. Clevers H, and Nusse R. Wnt/beta-catenin signaling and disease. Cell. (2012) 149:1192–205. doi: 10.1016/j.cell.2012.05.012
2. Ma B, and Hottiger MO. Crosstalk between wnt/beta-catenin and nf-kappab signaling pathway during inflammation. Front Immunol. (2016) 7:378. doi: 10.3389/fimmu.2016.00378
3. Whyte JL, Smith AA, and Helms JA. Wnt signaling and injury repair. Cold Spring Harb Perspect Biol. (2012) 4:a008078. doi: 10.1101/cshperspect.a008078
4. Baron R, and Kneissel M. Wnt signaling in bone homeostasis and disease: from human mutations to treatments. Nat Med. (2013) 19:179–92. doi: 10.1038/nm.3074
5. Babb R, Chandrasekaran D, Carvalho Moreno Neves V, and Sharpe PT. Axin2-expressing cells differentiate into reparative odontoblasts via autocrine wnt/beta-catenin signaling in response to tooth damage. Sci Rep. (2017) 7:3102. doi: 10.1038/s41598-017-03145-6
6. Hunter DJ, Bardet C, Mouraret S, Liu B, Singh G, Sadoine J, et al. Wnt acts as a prosurvival signal to enhance dentin regeneration. J Bone Miner Res. (2015) 30:1150–9. doi: 10.1002/jbmr.2444
7. Ishimoto K, Hayano S, Yanagita T, Kurosaka H, Kawanabe N, Itoh S, et al. Topical application of lithium chloride on the pulp induces dentin regeneration. PLoS ONE. (2015) 10:e0121938. doi: 10.1371/journal.pone.0121938
8. Neves VC, Babb R, Chandrasekaran D, and Sharpe PT. Promotion of natural tooth repair by small molecule gsk3 antagonists. Sci Rep. (2017) 7:39654. doi: 10.1038/srep39654
9. Zaugg LK, Banu A, Walther AR, Chandrasekaran D, Babb RC, Salzlechner C, et al. Translation approach for dentine regeneration using gsk-3 antagonists. J Dental Res. (2020) 99:544–51. doi: 10.1177/0022034520908593
10. Zhao Y, Yuan X, Bellido T, and Helms JA. A correlation between wnt/beta-catenin signaling and the rate of dentin secretion. J Endod. (2019) 45:1357–64.e1351. doi: 10.1016/j.joen.2019.07.014
11. Zhao Y, Yuan X, Liu B, Tulu US, and Helms JA. Wnt-responsive odontoblasts secrete new dentin after superficial tooth injury. J Dental Res. (2018) 97:1047–54. doi: 10.1177/0022034518763151
12. Han N, Zheng Y, Li R, Li X, Zhou M, Niu Y, et al. Beta-catenin enhances odontoblastic differentiation of dental pulp cells through activation of runx2. PLoS ONE. (2014) 9:e88890. doi: 10.1371/journal.pone.0088890
13. Volponi AA, Pang Y, and Sharpe PT. Stem cell-based biological tooth repair and regeneration. Trends Cell Biol. (2010) 20:715–22. doi: 10.1016/j.tcb.2010.09.012
14. Yokose S, and Naka T. Lymphocyte enhancer-binding factor 1: an essential factor in odontoblastic differentiation of dental pulp cells enzymatically isolated from rat incisors. J Bone Miner Metab. (2010) 28:650–8. doi: 10.1007/s00774-010-0185-0
15. Apostu D, Lucaciu O, Mester A, Oltean-Dan D, Baciut M, Baciut G, et al. Systemic drugs with impact on osteoarthritis. Drug Metab Rev. (2019) 51:498–523. doi: 10.1080/03602532.2019.1687511
16. Jakobsson E, Arguello-Miranda O, Chiu SW, Fazal Z, Kruczek J, Nunez-Corrales S, et al. Towards a unified understanding of lithium action in basic biology and its significance for applied biology. J Membr Biol. (2017) 250:587–604. doi: 10.1007/s00232-017-9998-2
17. Wang J, Liu B, Gu S, and Liang J. Effects of wnt/beta-catenin signalling on proliferation and differentiation of apical papilla stem cells. Cell Prolif. (2012) 45:121–31. doi: 10.1111/j.1365-2184.2012.00806.x
18. Klein PS, and Melton DA. A molecular mechanism for the effect of lithium on development. Proc Natl Acad Sci USA. (1996) 93:8455–9. doi: 10.1073/pnas.93.16.8455
19. Ali M, Okamoto M, Komichi S, Watanabe M, Huang H, Takahashi Y, et al. Lithium-containing surface pre-reacted glass fillers enhance hdpsc functions and induce reparative dentin formation in a rat pulp capping model through activation of wnt/β-catenin signaling. Acta Biomater. (2019) 96:594–604. doi: 10.1016/j.actbio.2019.06.016
20. Fathke C, Wilson L, Shah K, Kim B, Hocking A, Moon R, et al. Wnt signaling induces epithelial differentiation during cutaneous wound healing. BMC Cell Biol. (2006) 7:4. doi: 10.1186/1471-2121-7-4
21. Li J, Yao Q, Xu Y, Zhang H, Li L.-L., et al. Lithium chloride-releasing 3d printed scaffold for enhanced cartilage regeneration. Med Sci Monit. (2019) 25:4041–50. doi: 10.12659/MSM.916918
22. Zheng C, Chen J, Liu S, and Jin Y. Stem cell-based bone and dental regeneration: a view of microenvironmental modulation. Int J Oral Sci. (2019) 11:23. doi: 10.1038/s41368-019-0060-3
23. Balic A, Aguila HL, and Mina M. Identification of cells at early and late stages of polarization during odontoblast differentiation using pobcol3.6gfp and pobcol2.3gfp transgenic mice. Bone. (2010) 47:948–58. doi: 10.1016/j.bone.2010.08.009
24. Vijaykumar A, Dyrkacz P, Vidovic-Zdrilic I, Maye P, and Mina M. Expression of bsp-gfptpz transgene during osteogenesis and reparative dentinogenesis. J Dental Res. (2020) 99:89–97. doi: 10.1177/0022034519885089
25. Vijaykumar A, Ghassem-Zadeh S, Vidovic-Zdrilic I, Komitas K, Adameyko I, Krivanek J, et al. Generation and characterization of dspp-cerulean/dmp1-cherry reporter mice. Genesis. (2019) 57:e23324. doi: 10.1002/dvg.23324
26. Sagomonyants K, Kalajzic I, Maye P, and Mina M. Enhanced dentinogenesis of pulp progenitors by early exposure to fgf2. J Dental Res. (2015) 94:1582–90. doi: 10.1177/0022034515599768
27. Vijaykumar A, Root SH, and Mina M. Wnt/beta-catenin signaling promotes the formation of preodontoblasts in vitro. J Dental Res. (2021) 100:387–96. doi: 10.1177/0022034520967353
28. Sagomonyants K, and Mina M. Biphasic effects of fgf2 on odontoblast differentiation involve changes in the bmp and wnt signaling pathways. Connect Tissue Res. (2014) 55 (Suppl. 1):53–6. doi: 10.3109/03008207.2014.923867
29. Jho EH, Zhang T, Domon C, Joo CK, Freund JN, and Costantini F. Wnt/beta-catenin/tcf signaling induces the transcription of axin2, a negative regulator of the signaling pathway. Mol Cell Biol. (2002) 22:1172–83. doi: 10.1128/MCB.22.4.1172-1183.2002
30. Vidovic I, Banerjee A, Fatahi R, Matthews BG, Dyment NA, Kalajzic I, et al. Alphasma-expressing perivascular cells represent dental pulp progenitors in vivo. J Dental Res. (2017) 96:323–30. doi: 10.1177/0022034516678208
31. Cruciat CM, and Niehrs C. Secreted and transmembrane wnt inhibitors and activators. Cold Spring Harb Perspect Biol. (2013) 5:a015081. doi: 10.1101/cshperspect.a015081
32. Wong SK, Chin KY, and Ima-Nirwana S. The skeletal-protecting action and mechanisms of action for mood-stabilizing drug lithium chloride: current evidence and future potential research areas. Front Pharmacol. (2020) 11:430. doi: 10.3389/fphar.2020.00430
33. Yu Z, Fan L, Li J, Ge Z, Dang X, and Wang K. Lithium chloride attenuates the abnormal osteogenic/adipogenic differentiation of bone marrow-derived mesenchymal stem cells obtained from rats with steroid-related osteonecrosis by activating the beta-catenin pathway. Int J Mol Med. (2015) 36:1264–72. doi: 10.3892/ijmm.2015.2340
34. Heo JS, Lee SY, and Lee JC. Wnt/beta-catenin signaling enhances osteoblastogenic differentiation from human periodontal ligament fibroblasts. Mol Cells. (2010) 30:449–54. doi: 10.1007/s10059-010-0139-3
35. Li J, Khavandgar Z, Lin SH, and Murshed M. Lithium chloride attenuates bmp-2 signaling and inhibits osteogenic differentiation through a novel wnt/gsk3- independent mechanism. Bone. (2011) 48:321–31. doi: 10.1016/j.bone.2010.09.033
36. Shi YC, Worton L, Esteban L, Baldock P, Fong C, Eisman JA, et al. Effects of continuous activation of vitamin d and wnt response pathways on osteoblastic proliferation and differentiation. Bone. (2007) 41:87–96. doi: 10.1016/j.bone.2007.04.174
37. de Boer J, Siddappa R, Gaspar C, van Apeldoorn A, Fodde R, and van Blitterswijk C. Wnt signaling inhibits osteogenic differentiation of human mesenchymal stem cells. Bone. (2004) 34:818–26. doi: 10.1016/j.bone.2004.01.016
38. Nemoto E, Koshikawa Y, Kanaya S, Tsuchiya M, Tamura M, Somerman MJ, et al. Wnt signaling inhibits cementoblast differentiation and promotes proliferation. Bone. (2009) 44:805–12. doi: 10.1016/j.bone.2008.12.029
39. Rattanawarawipa P, Pavasant P, Osathanon T, and Sukarawan W. Effect of lithium chloride on cell proliferation and osteogenic differentiation in stem cells from human exfoliated deciduous teeth. Tissue Cell. (2016) 48:425–31. doi: 10.1016/j.tice.2016.08.005
40. Meng L, Wang X, Torensma R, Von den Hoff JW, and Bian Z. Lithium inhibits palatal fusion and osteogenic differentiation in palatal shelves in vitro. Arch Oral Biol. (2015) 60:501–7. doi: 10.1016/j.archoralbio.2014.12.011
41. Brown KM, and Tracy DK. Lithium: the pharmacodynamic actions of the amazing ion. Ther Adv Psychopharmacol. (2013) 3:163–76. doi: 10.1177/2045125312471963
42. Gámez B, Rodríguez-Carballo E, Graupera M, Rosa JL, and Ventura F. Class i pi-3-kinase signaling is critical for bone formation through regulation of smad1 activity in osteoblasts. J Bone Mineral Res. (2016) 31:1617–30. doi: 10.1002/jbmr.2819
Keywords: LiCl, dentinogenesis, osteogenesis, pulp culture, reporter mice
Citation: Vijaykumar A and Mina M (2021) Lithium Chloride Exerts Differential Effects on Dentinogenesis and Osteogenesis in Primary Pulp Cultures. Front. Dent. Med. 2:649500. doi: 10.3389/fdmed.2021.649500
Received: 04 January 2021; Accepted: 28 April 2021;
Published: 16 June 2021.
Edited by:
Emi Shimizu, Rutgers, The State University of New Jersey, United StatesReviewed by:
Naoto Ohkura, Niigata University, JapanTakahiko Morotomi, Kyushu Dental University, Japan
Copyright © 2021 Vijaykumar and Mina. This is an open-access article distributed under the terms of the Creative Commons Attribution License (CC BY). The use, distribution or reproduction in other forums is permitted, provided the original author(s) and the copyright owner(s) are credited and that the original publication in this journal is cited, in accordance with accepted academic practice. No use, distribution or reproduction is permitted which does not comply with these terms.
*Correspondence: Mina Mina, Mina@uchc.edu