- CRPG, CNRS, Université de Lorraine, UMR 7358, Vandœuvre-lès-Nancy, France
Rare earth elements (REEs) are considered emerging anthropogenic pollutants. Anthropogenic lanthanum, cerium, samarium, and gadolinium alone, or excess of all the REEs have already been reported in the environment. In addition, it is only a matter of time for neodymium (Nd) of anthropogenic origin to be reported disseminated in the environment, given its growing demand for new technologies and its use in permanent magnets of wind turbine. So far, only in a few cases was the addition of anthropogenic Nd detected in soils and sediments by the measurements of REE concentrations. For this reason, we propose to use the Nd isotopic composition to help the distinction of pollution. The isotopic tracing of Nd using variations in the abundance of 143Nd from the radioactive decay of 147Sm (Nd-radiogenic composition) is one option. Here, we expand the Nd isotopic fingerprinting by the investigation of the stable Nd isotopic composition expressed as δxNd, the relative permil (%0) deviation from the isotopic composition of the pure Nd JNdi-1 reference standard. The measurement of δxNd used a MC-ICPMS (multi-collector inductively coupled plasma mass spectrometry) with sample-standard bracketing technique, allowing the determination of precise and accurate Nd isotopic variations. Our results show that Nd-magnets (Neo) and man-made purified Nd materials are not significantly different on average (respectively, δ148Nd of −0.105 ± 0.023 and −0.120 ± 0.141%0). More importantly, they are different from terrestrial rocks (δ148Nd of −0.051 ± 0.031%0). Moreover, the Nd-radiogenic composition of Neo can be highly variable, even when they come from a single supplier. In addition, the study of all Nd stable isotopic compositions demonstrates that irrespective of their natural origin (witnessed by their Nd-radiogenic composition), all Nd from rocks and man-made materials are related by mass-dependent isotopic fractionation laws. We also have defined a parameter, the Δ148−150Nd′, allowing the distinction of thermodynamic isotopic fractionation (the Δ148−150Nd′ is invariant) from kinetic isotopic fractionation (the Δ148−150Nd′ is negatively correlated with the δ148Nd). Such covariation is observed for anthropogenic materials that could be seen as small deficit in 150Nd (around 5 ppm/%0/amu), but too small to be consistent with nuclear field effect. On the other hand, the anthropogenic material defines a covariation in the Δ148−150Nd'–δ148Nd space in full agreement with the theoretical expectation from mass-dependent kinetic isotopic fractionation. The mass-dependent fractionation of Nd by chromatographic separation is also consistent with a kinetic isotopic fractionation. The purification of Nd from other light REEs by industrial processes involves chromatographic separation and, therefore, is likely to produce anthropogenic Nd with low values for δ148Nd associated with high values for Δ148−150Nd′. Both are resolvable with current MC-ICPMS technology and could be useful to trace incoming anthropogenic pollution in the environment. In soils, the combination of low values for δ148Nd with high values for Δ148−150Nd′ is likely to be an unambiguous pollution signal from the degradation in the environment of Neo or other industrial products, especially if this is associated with an Nd-radiogenic composition inconsistent with the surrounding rocks and soils. In contrast, the industrial residue of Nd purification could be characterized by high δ148Nd with low values for Δ148−150Nd′, and the leak or the discharge of such residue could also be unambiguously distinguished.
Introduction
The neodymium (Nd) is one of the rare earth elements (REEs) used in industry for various applications (e.g., metallurgy, battery alloys, and ceramics) but overwhelmingly (70%) in permanent magnets (Dutta et al., 2016). Nd-magnets (Neo) contain 20–35% of Nd and are present in new technologies (CD, DVD, or computer hard drive). By far, the increasing use of Neo is linked to the current energy transition. The wind turbine requires 40–200 kg of Nd per MW (Moss et al., 2011; Shaw and Constantinides, 2012), while the electric car and electric bike can even have a greater share in Neo consumption in the future (Brown, 2016). By 2,035, neodymium demand may be almost 40% of the total demand of REEs compared with the 20% in 2010 (Alonso et al., 2012). In addition, Neo is not currently recycled and might not be in the near future (Yang et al., 2017; Diehl et al., 2018).
Anthropogenic contaminations by REEs have already been reported in continental waters, for gadolinium (Gd), lanthanum (La), samarium (Sm), and cerium (Ce) (e.g., Kulaksiz and Bau, 2013 and reference therein; Hissler et al., 2015). In the environment, anthropogenic Nd has been reported in sediment near industrial activity of REE ore deposits (e.g., Elbaz-Poulichet and Dupuy, 1999; Shynu et al., 2011) and reprocessing (e.g., Oliveira et al., 2007; Sanders et al., 2013; Wen et al., 2013; Cánovas et al., 2018). The increasing industrial processing and exponential rise of REE mine production (Haxel et al., 2002) will create a more preeminent source of Nd pollution. In addition, the fragility and susceptibility to corrosion for Neo (e.g., Sagawa et al., 1987) will likely generate another, although more widespread, source of anthropogenic Nd in the environment. The ecotoxicology of REEs is debated, but high REEs levels in the environment have been linked to negative consequences in plants, on animals, and microorganisms (e.g., Gwenzi et al., 2018). Thus, the growing demand associated to environmental vulnerability of some REE industrial products strongly suggest that anthropogenic Nd can be considered as an emerging pollutant in the environment (Gwenzi et al., 2018). The use of radiogenic isotopic composition of Nd (the variable excess of 143Nd relative to all the other stable isotopes of Nd that is related to the decay of the long-life radioactive 147Sm) has revealed the transfers of Nd from pollution induced by industrial activity to animals (Bosco-Santos et al., 2018). In natural waters, Nd, like all the other REEs, is present at ppt level, and by analogy with Gd (Tepe et al., 2014; Merschel et al., 2015), the use of the concentration difference can be a sufficient tool to identify anthropogenic Nd contamination. So far, all REEs (e.g., Hao et al., 2016) or specific REEs, but not Nd, have been detected in continental waters. In soils and sediments, Nd concentrations are, in most cases, above the ppm level (Freslon et al., 2014 and references therein), making anthropogenic Nd contamination more difficult to detect by the use of the REEs concentration only (e.g., Olmez et al., 1991). Recent studies have focused on polluted river sediments and soils, and only the presence of anthropogenic Gd has been demonstrated by the measurement of REE concentrations alone (da Silva et al., 2018; Altomare et al., 2020).
Here, we propose to expand the isotopic tracing of Nd using variations in the abundance of 143Nd from the radioactive decay of 147Sm (e.g., Piepgras et al., 1979; Biscaye et al., 1997; Frank, 2002; Lahd Geagea et al., 2008; Li et al., 2009; Bosco-Santos et al., 2018) by the investigation of the isotopic composition of Neo. However, the radiogenic isotopic composition of Nd in soils can be highly variable, even in one location (e.g., Viers and Wasserburg, 2004), and so far, only industrial pollution has been traced with variations in the abundance of 143Nd in soils (e.g., Steinmann and Stille, 1997). Therefore, we also propose the measurement of the mass-dependent Nd isotopic signatures as a new tool to better distinguish anthropogenic from natural Nd.
The study of stable Nd isotope variations is recent. Analysis of commercial Nd oxide reagents of high purity (Wakaki and Tanaka, 2012) shows that Nd stable isotopic variations could be inherited from the source materials or be caused by isotope fractionation during the industrial purification of Nd from REEs ores. The latter is further suggested since the stable Nd isotopic compositions of various geological reference materials and terrestrial samples showed only very small natural variations (Ma et al., 2013; Ohno and Hirata, 2013; Saji et al., 2016; McCoy-West et al., 2017, 2020a,b). In this study, we report the stable isotopic variations of Nd affected by anthropogenic processes and investigate the use of the mass-dependent Nd isotopic analysis as a new tool to trace Nd pollution in the environment.
Materials and Methods
Samples
Six man-made purified Nd samples and seven Nd-magnets (Neo) were selected for this study; in addition to the JNdi-1, a pure Nd solution (Tanaka et al., 2000) used as the reference standard. Man-made purified samples (commercially available) are pure Nd in a solution of dilute HNO3 that mainly correspond from the dissolution of Nd oxides. Four correspond to Nd-concentration standard solutions: NNB, JMC (Luais et al., 1997), JMC′, and NIST 3135a (Table 1). The other two are solutions used for their 143Nd/144Nd ratio: JM-Nancy [J&M in Henry et al. (1997)] and AR [Ames Rennes Nd in Chauvel and Blichert-Toft (2001)]. Also, we used a multi-REEs concentration standard (Nancy-16rees; Table 1) containing the 14 naturally occurring lanthanides, Y and Sc. The seven Neo are all made of Nd2Fe14B by the Magnet Expert Ltd Company but differ by their specific pull force (Table 2). Their fragility and susceptibility to corrosion (Sagawa et al., 1987) is diminished by Ni–Cu–Ni coating and by increasing the temperature resistivity (Tmax). We choose magnets with distinct pull force and distinct Tmax (Table 2).
Degree of Purification
We used a Thermo Scientific X-series 2 quadrupole inductively coupled plasma mass spectrometer (ICP-MS) to measure concentrations of La, Ce, praseodymium (Pr), Nd, and Sm in Neo and purified Nd samples. Since all our samples have a high purity of Nd, they were introduced with an Nd concentration of at least 1 ppm to allow the measurement of trace amounts of other REEs. Samples were diluted in 0.5 M HCl with 10 ppb of indium as internal standard. Wash time of 7 min between each measurement allowed full decontamination. At 2σ level, the external reproducibility, calculated from five repeated measurements of the NNB solution is 0.3, 1.5, 1.8, and 5.7 ppt/ppm for La/Nd, Ce/Nd, Pr/Nd, and Sm/Nd ratios, respectively.
Mass Spectrometry
The variations of the Nd isotopic composition were obtained using a Thermo Scientific Neptune Plus multi-collector inductively coupled plasma mass spectrometer (MC-ICPMS) coupled to an Apex HF desolvating nebulizer with an aspiration rate of 50 μl/min. The nine Faraday cups of the multi-collector were used to measure, in static low-resolution mode, the seven Nd isotopes (142Nd, 143Nd, 144Nd, 145Nd, 146Nd, 148Nd, and 150Nd) together with two isotopes of Sm (147Sm and 149Sm), to confirm the lack of Sm isobaric interferences on the 144Nd, 148Nd, and 150Nd.
The standard-sample-standard bracketing technique (e.g., Halicz et al., 1999) has been applied to Nd isotopes. Solutions were introduced at a concentration of 50 ppb of Nd in 2% (by volume) HNO3, generating an average 146Nd beam of 1.3 × 10−11 A on the central collector. A negligible cross-contamination (<0.8 × 10−14 A) between the sample and the standard was achieved by aspirating 2% HNO3 wash solution for 12 min between analyses. Our protocol consists of the measurement of standard and sample isotopes values four and three times, respectively, for 200 s each, allowing the calculation of five brackets, and each value corresponds to their average. Each 200-s measurement consists of 100 s of acquisition of the signal (8.389 s × 12 cycles), repeated twice. A single measurement requires almost 2 h and consumes up to 40 ng of Nd.
Nd stable isotopic compositions are expressed as the permil deviation from the isotopic composition of the JNdi-1 as follows:
where m = 145, 146, 148, or 150. Since the intensities of samples and standards were matched within 10% of each other, the delta notation and the bracketing technique reduce any contribution from the cross-contamination and isobaric interferences (e.g., Galy et al., 2001). At 2σ level, the external reproducibility, calculated from 48 repeated measurements of the JNdi-1 standard, is 0.031%0, 0.040%0, 0.071%0, and 0.107%0 for δ145Nd, δ146Nd, δ148Nd, and δ150Nd, respectively (Table 3). Previous studies have established that terrestrial Nd stable isotopic compositions tend to follow mass-dependent laws (e.g., Wakaki and Tanaka, 2012; Ma et al., 2013; Ohno and Hirata, 2013; Saji et al., 2016; McCoy-West et al., 2017), implying that the largest variations should be displayed by δ150Nd followed by δ148Nd. However, some of the literature data have been acquired using a double-spike method or MC-ICPMS that prevented the study of δ150Nd. Thus, in the following, we will discuss the δ148Nd. In addition, we also introduce the Δ′ notation to calculate the deviation from the thermodynamic mass-dependent behavior of two stable isotope ratios (Young and Galy, 2004). Here, it is applied to 148Nd/144Nd and 150Nd/144Nd with:
where β is the exponent relating the thermodynamic fractionation factors for two isotope ratios (Young et al., 2002) and has a maximum value of 0.6756 for 148Nd/144Nd 150Nd/144Nd using the latest accepted atomic masses of Nd isotopes (Audi et al., 2017) and their reduced masses including molecular masses tending toward infinity (Hayles et al., 2017). At 2σ level, the external reproducibility of Δ148−150Nd′, calculated from 48 repeated measurements of the JNdi-1 standard, is 0.042%0 (Table 3).
We also measured the radiogenic signature of 143Nd, expressed as ε143Nd with the CHUR (chondritic uniform reservoir) for reference (DePaolo and Wasserburg, 1976) such as:
using 143Nd/144Nd ratios normalized to 146Nd/144Nd = 0.7219 (Wasserburg et al., 1981) by the exponential law, and to the JNdi-1 ratio 143Nd/144Nd = 0.512115 (Tanaka et al., 2000), and using a true bracketing linear correction following Wilson et al. (2012).
Chemical Treatment
Neo was dissolved at ambient temperature in 4 ml of concentrated distilled HNO3 under extractor hood. After dilution with de-ionized water and evaporation, an aliquot containing 2 μg of Nd in 2 M HNO3 was taken for chemical separation of Nd from all the other elements contained in Neo. Indeed, their structural formula is Nd2Fe14B, and they often contain some Dy and other elements as traces (e.g., Sagawa et al., 1987). Nd purification is required since the accurate measurement of stable isotopic variations by MC-ICPMS can be disturbed by isobaric interferences and matrix effects (e.g., Galy et al., 2001). Our chemical protocol adapted the chromatographic separation used for the measurement of ε143Nd (Pin and Zalduegui, 1997). Since the Ln-resin produced mass-dependent fractionation of Nd (Wakaki and Tanaka, 2012; Ohno and Hirata, 2013; Saji et al., 2016), we calibrated the volume corresponding to the collection of Nd in order to recover 100% of Nd, and avoid any isotopic bias. A 100% recovery was achieved in three steps. First is the use of a column filled with 1.6 ml of Tru-resin 50–100 μm that removed all major and trace elements from REEs in 2 M HNO3. The addition of 4.5 ml of ultrapure water (>18 MΩ.cm, MilliQ Plus system) released all the REEs from the Tru-resin directly into a second column composed of a bed of 0.6 ml of AG1 X8 resin 200–400 mesh above 3.2 ml of Ln-resin 50–100 μm. The second step consisted of collecting all Nd and some other REEs by the addition of 2.5 ml of 6 M HCl. After evaporation and dilution in 0.2 ml of 0.27 M HCl, the third separation consisted of loading onto another column, with an internal diameter of 4.0 mm, composed of a bed of 0.6 ml of AG1 X8 resin 200–400 mesh above 3.2 ml of Ln-resin 50–100 μm that separated Nd from La, Sm, Eu, and all the heavy REEs. The full elution of Nd required 4 ml of 0.27 M HCl. Regularly, the JNdi-1 standard followed the same chemistry procedure to validate the lack of stable isotopic fractionation by this protocol.
Unfortunately, the purification of Nd from light REEs (Ce and Pr) would have required a different chemical protocol (e.g., Rehkamper et al., 1996), and the occurrence of up to 40% of the Ce and Pr initially present in the sample and still present after the purification of Nd on Ln-resin (e.g., McCoy-West et al., 2020a) might induce inaccuracy in the δmNd due to matrix effects. Since the average Ce/Nd and Pr/Nd mass ratios of supracrustal rocks are 2.46 and 0.27, respectively (e.g., McLennan, 2001), and that soils can see relative enrichment by a factor of 0.5–5 of these ratios (e.g., Braun et al., 1998; Ramos et al., 2016), the incomplete purification of Nd could lead to Ce/Nd ≤ 5 and Pr/Nd ≤ 0.6 in the Nd fraction. Fortunately, using our protocol, the addition of Pr (up to 0.7 Pr/Nd mass ratio) or Ce (up to 5 Ce/Nd mass ratio) to the NNB solution had no effect on the δmNd measurement during the MC-ICPMS analysis (Supplementary Figure 1 and Supplementary Table 1).
The mass-dependent fractionation of Nd by chromatographic separation (Wakaki and Tanaka, 2012; Ohno and Hirata, 2013; Saji et al., 2016) has been further tested using pure Nd solutions (NNB and JMC) and the multi-REE solution (Nancy-16rees). Pure Nd solution JMC (3 μg) and of the multi-REEs solution Nancy-16Rees and 1 μg of pure Nd solution of NNB were evaporated and dissolved in 0.2 ml of 0.27 M HCl. Then, each solution was loaded onto a column, with an internal diameter of 4.0 mm, composed of a bed of 0.6 ml of AG1 X8 resin 200–400 mesh above 3.2 ml of Ln-resin 50–100 μm. An eluate of 0.27 M HCl was added to the column, and the total volume of eluate and corresponding amount of collected Nd are reported in Supplementary Table 2. For the test with 1 μg of Nd of NNB, only four fractions were separated. For the two tests with 3 μg of Nd, 10 fractions were collected (Supplementary Table 2).
Results
Accuracy of the Data
The accuracy of radiogenic data can be validated for two solutions already studied (AR and JM-Nancy) since their ε143Nd of −12.98 ± 0.33 (2σ, N = 19) and −29.40 ± 0.29 (2σ, N = 10), respectively (Table 3), are consistent with literature values of −13.21 ± 0.25 and −29.53 ± 0.47, respectively (Henry et al., 1997; Chauvel and Blichert-Toft, 2001). The ε143Nd of the JM-Nancy (Henry et al., 1997; this study) is more than 21 ε units lower than the JM Nd used by Wakaki and Tanaka (2012). Such solutions were produced by the dissolution of pure Nd metal, and this huge difference likely reflects the use of different source materials by the Johnson-Mattey Company to produce Nd metal. Thus, these two JMs can be considered as two distinct samples.
The accuracy of the stable isotopic compositions presented in this study has been validated by the standard addition method. JM-Nancy and JMC solutions were mixed in known proportions. The variable mixing proportion, calculated by weight, allowed the calculation of an estimated ε143Nd. When the propagation of mixing uncertainties is taken into account, the estimated ε143Nd agrees with the measured ε143Nd of the mix solutions (Figure 1). Such agreement and the small uncertainties associated with the measured ε143Nd suggested the use of the measured ε143Nd as a more precise measurement of the mixing proportion. The linear correlation between the measured δ148Nd and ε143Nd of the mixing is not offset from values of the pure JM-Nancy and JMC solutions (Figure 1) and supports the accurate determination of the stable isotopic variation of Nd with our protocol. Only one of our studied solutions was already measured against the JNdi-1 in the literature, the NIST3135a, with a δ148Nd of −0.04 ± 0.11 (2σ, N = 11, Ma et al., 2013). At 2σmean level, this value is different from the δ148Nd of −0.20 ± 0.13 (2σ, N = 14, Table 3) of NIST3135a reported in this study. However, the accuracy of our measurements has been validated by the standard addition method, and thus, a δ148Nd of −0.20%0 for NIST3135a is more accurate.
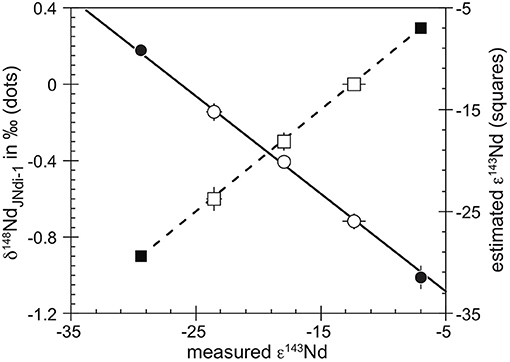
Figure 1. Stable Nd isotopic composition (δ148Nd, left axis, dots) and estimated radiogenic Nd isotopic composition (ε143Nd, right axis, squares) as a function of the measured ε143Nd of the mixture of two purified Nd solutions (JM-Nancy and JMC, filled symbols). For the expected and measured ε143Nd data, the dashed line corresponds to the theoretical mixing of the JM-Nancy and JMC solutions. The plain line corresponds to the least squares linear regression of the mixed solutions (Supplementary Table 3). The extrapolation of this regression goes through the measured isotopic compositions of both pure solutions, validating the accuracy of the δ148Nd measured in this study.
Stable and Radiogenic Nd Isotopic Variations
For the radiogenic compositions, except N35 and N50, all Neo have different ε143Nd, with a total range of around 10 ε units (Table 3). The purified Nd samples have an even greater range (almost 26 ε units) in ε143Nd. The purified Nd samples present a range of 1.19%0 in δ148Nd associated with a Δ148−150Nd′ very close to 0 (Table 3), in agreement with the previous findings of variable stable isotopic compositions of pure Nd metal and solutions (Wakaki and Tanaka, 2012; Ma et al., 2013; Ohno and Hirata, 2013; Saji et al., 2016; McCoy-West et al., 2020a). On the contrary, the δ148Nd of the Neo is rather constant, with an average value of −0.105 ± 0.023%0, (2σmean, N = 7, Table 3) despite variable sources of Nd exhibited by variable ε143Nd (Table 3). The average Δ148−150Nd′ of anthropogenic materials (purified Nd and Neo) is −0.008 ± 0.009%0 (2σmean, N = 13). So, industrially purified Nd have 1) distinct stable isotopic composition from JNdi-1, 2) stable isotopic compositions in agreement with mass-dependent fractionations, and 3) a significant spread in their δ148Nd (Table 3). However, there is no correlation between the δ148Nd and the degree of purification (relative abundance of La, Ce, Pr, and Sm to Nd) or the ε143Nd of the purified Nd samples (Table 3 and Supplementary Table 3). In addition, the Neo has a greater level (~3 orders of magnitude, Supplementary Table 3) of other REEs than the purified Nd samples (Supplementary Table 3).
A detailed description of the mass-dependent fractionation of Nd by chromatographic separation is provided in the Supplementary Material. In short, the purified Nd fractions obtained by chromatographic separation present a range of 0.72%0, 1.11%0, and 1.49%0 in δ148Nd for NNB, JMC, and Nancy-16rees, respectively (Supplementary Table 2). The first fractions are enriched in heavy isotopes, while the last fractions are enriched in light isotopes, but all fractions are characterized by a Δ148−150Nd′ very close to 0, implying a mass-dependent isotopic fractionation between the (Nd3+)–(Cl−)3 complex in solution and Nd bound to the di(2-ethylhexyl) orthophosphoric acid (HDEHP) of the Ln-resin. So, for anthropogenic materials and chromatographic isotopic fractionation (Table 3 and Supplementary Table 2), the Δ148−150Nd′ is always close to 0. In detail, however, a significant correlation is found between the Δ148−150Nd′ and the δ148Nd of purified Nd samples (Figure 2). Such correlation becomes significant when the number of repeat analyses is > 10 (Table 3), allowing reduced uncertainties. It suggests that Neo is on the same trend, while JNdi-1 is outside the trend. In addition, the three different tests of chromatographic isotopic fractionation (Supplementary Table 2) are also consistent with a negative covariation between Δ148−150Nd′ and δ148Nd, although with poorer precision related to a smaller number of repeat analyses.
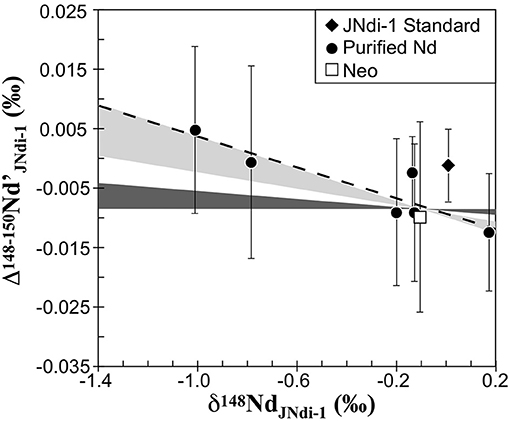
Figure 2. The Δ148−150Nd′ as a function of the δ148Nd for anthropogenic material. The error bar corresponds to 2σmean. The dashed line corresponds to the least squares linear regression of the purified Nd. The shaded areas represent the theoretical domain for a kinetic mass-dependent isotopic fractionation (light gray) and thermodynamic mass-dependent isotopic fractionation (dark gray). The least squares linear regression of the purified Nd is in close agreement with a kinetic mass-dependent isotopic fractionation. The JNdi-1, also a purified Nd, has been excluded from the relationship (see text).
Discussion
Anthropogenic Signature
The significant difference in the radiogenic isotopic compositions of Neo (10 ε units) and purified Nd samples (~26 ε units) suggests that their Nd originate from different batches if not from different REE ores. Therefore, the natural source of REEs is diverse for the set of studied industrially purified Nd materials (Table 3). The ε143Nd has proven to be a useful tool to infer anthropogenic pollution from industrial sites to soils (e.g., Steinmann and Stille, 1997) and even to animals (Bosco-Santos et al., 2018) because the industrial process or storage of REEs was efficient enough to mix variable ore sources or used only one source. Here, we show that Neo from one single supplier can have large variation in their ε143Nd, and increasing the range of supplier would likely widen the range of the radiogenic signature of Neo. Moreover, soils can have a heterogeneous ε143Nd (>8 ε units; Viers and Wasserburg, 2004). So, in the case of an anthropogenic pollution of soils by Neo, the ε143Nd alone could be inconclusive.
There is no relationship between the radiogenic and stable isotopic compositions of anthropogenic materials. Such lack of relationship has already been reported for terrestrial rocks (McCoy-West et al., 2017, 2020b). So, variations in the natural source of Nd cannot explain the range of 1.19%0 in δ148Nd of purified Nd samples. Moreover, when the literature data are included (Table 4 and Figure 3), the δ148Nd of purified Nd samples has a range and an average of 1.45%0 and −0.120 ± 0.141%0 (2σmean, N = 22), respectively. This is not greatly different from the range and average of the subset of anthropogenic Nd samples (purified Nd and Neo taken together) studied here. In addition, the average stable isotopic composition for both the Neo and the purified Nd materials are similar, with a δ148Nd around −0.1%0 (Table 4).
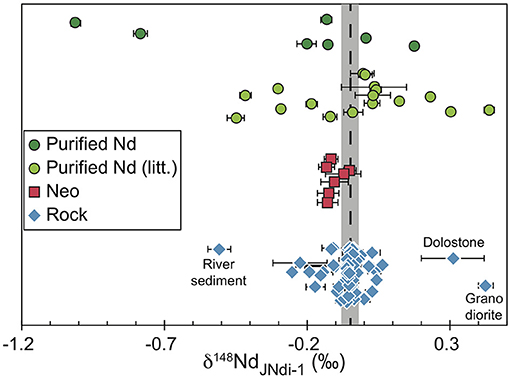
Figure 3. Compilation of stable Nd isotope composition measured in purified Nd (green circles), Neo (red square), and terrestrial rocks (blue diamonds). The nature of the three outlier rocks have been indicated. The references correspond to those in Table 4. The dashed line and the gray area correspond to the mean and 95% confidence level of the mean of the terrestrial rocks, respectively.
The range and average value of the δ148Nd for natural terrestrial samples is more difficult to determine since the large majority of the data reported δ146Nd (e.g., McCoy-West et al., 2017) or δ145Nd (Saji et al., 2016), with only few reporting δ148Nd (Ma et al., 2013; Ohno and Hirata, 2013). However, in some cases, the δ148Nd has been measured simultaneously to the δ146Nd and those two are related by the linear relationship of δ148Nd = 2.00037 × δ146Nd (McCoy-West et al., 2020a). Using this, the δ148Nd of terrestrial rocks has an average of −0.051 ± 0.031%0 (2σmean, N = 58, Ohno and Hirata, 2013; McCoy-West et al., 2017, 2020a,b; Ma et al., 2013; Table 4). Some small differences between data produced in different laboratories have been reported (e.g., McCoy-West et al., 2020a), but the average δ148Nd of rock samples, estimated here, is not different from a smaller but self-consistent dataset (Table 4, McCoy-West et al., 2020a,b; McCoy-West et al., 2017). In addition, three samples, one granodiorite, one river sediment (Ma et al., 2013), and one dolostone (Ohno and Hirata, 2013) are outliers (excluding those returns 2σmean reduced to 0.017%0 with N = 55) on the terrestrial dataset (Figure 3). Consequently, on average, there is a difference of ~0.05%0 between the δ148Nd of anthropogenic materials and terrestrial rocks, with the average anthropogenic materials enriched in light isotopes (Table 4). In addition, anthropogenic materials are characterized by a far greater spread in the δ148Nd than terrestrial rocks (Figure 3).
Nature of the Stable Nd Isotopic Variations in Anthropogenic Materials
All terrestrial materials, despite a diverse origin exhibited by their variable ε143Nd, are related by mass-dependent partitioning of isotopes (e.g., Wakaki and Tanaka, 2012; Ma et al., 2013; Ohno and Hirata, 2013; Saji et al., 2016; McCoy-West et al., 2017). So, the enrichment in light isotopes in anthropogenic materials by comparison with terrestrial rocks is likely to be related to an isotopic fractionation during the purification of Nd from the other REEs. Cation exchange is used to obtain individual lanthanides because it is one of the most successful tools to obtain these elements with a high degree of purity (e.g., Spedding et al., 1956; Powell, 1964; Kolodynska and Hubicki, 2012). Several cation exchange resins with various chemical protocols exist to separate the REEs that might be different from one Nd man-made sample to another. The industrial protocols are likely to be not unique and also to include several steps. This might explain the lack of correlation between the degree of purification and the δ148Nd of the purified Nd samples (Table 3 and Supplementary Table 3). In addition, the degree of impurities in the Neo are far greater (~3 orders of magnitude, Supplementary Table 3) than for the purified Nd samples, but the impurities could be added like Gd (e.g., Yang et al., 2017) or could be the result of a lower degree of purification of the Nd used in the Neo. So the amount of impurities in our studied man-made samples is likely to be not a straightforward proxy for the method of purification of Nd on a first instance.
However, Ln-resin produces mass-dependent fractionation of Nd (Ohno and Hirata, 2013; Saji et al., 2016), and a detailed study of such fractionation gives a general sense of mass-dependent fractionation of Nd by most industrial processes. For anthropogenic materials and chromatographic isotopic fractionation, the Δ148−150Nd′ is correlated with the δ148Nd (Figure 2 and Supplementary Table 2). By definition of Δ148−150Nd′, its variation implies that the mass-dependent fractionation of Nd has not been achieved by a thermodynamic equilibrium process, if the studied material has a common origin (in our case, all materials have been made from terrestrial Nd). Instead, the mass-dependent fractionation of Nd by kinetic processes would induce a linear negative correlation between Δ148−150Nd′ and δ148Nd (Figure 2). Only JNdi-1 is outside the trend and using the y-intercept of the Δ148−150Nd′-δ148Nd linear relationship (Figure 2), the JNdi-1 data would be consistent with an excess of 9 or 29 ppm of 148Nd or 144Nd, respectively, or a deficit of 14 ppm of 150Nd with respect to all the other anthropogenic materials studied here. Such offset of the standard can have different origin (purification, mixing, …) and has already been reported for other isotopic systems (e.g., Mg; Tipper et al., 2008). The negative covariation (Figure 2) implies a small excess of 150Nd associated with the enrichment in heavy isotopes by kinetic mass-dependent fractionation. Using the slope of Δ148−150Nd′-δ148Nd (Figure 2), we can estimate that the excess of 150Nd is around 20 ppm for a rise in δ148Nd of 1%0, so ~5 ppm/%0/amu. This is almost 2 orders of magnitude smaller than the enrichment in 150Nd of 395 ppm/%0/amu that could be related to nuclear field effect (Saji et al., 2016). However, the Δ148−150Nd′ corresponds to the deviation from a thermodynamic mass-dependent fractionation (hence, the maximum value of 0.6756 for β). Also, other values for β are possible. For instance, the minimum value for thermodynamic equilibrium is 0.6734 using molecular masses of 148Nd-M 150Nd-M and 144Nd-M with M a macromolecule with a mass tending toward infinity (Hayles et al., 2017). The absolute minimum for β (0.6666) is reached when kinetic isotopic fractionation is considered (Young et al., 2002). In that case, it would generate a negative relationship between Δ148−150Nd′ and δ148Nd. The field defined in such space by all possible β (0.6666–0.6711) characterizing kinetic isotopic fractionation (Figure 2) is consistent with the slope defined by anthropogenic materials (excluding JNdi-1). A common kinetic isotopic fractionation for the purified metal, solutions, and Neo (Figure 2) suggests that, irrespective of the industrial source of anthropogenic materials (Tables 1, 2), a common process is likely to fractionate Nd isotopes during its industrial purification. The effect of kinetic isotopic fractionation is, however, too small to explain the excess of 150Nd reported previously (Saji et al., 2016). The discrepancy between the two studies is likely to result from the different treatments of the data collected by MC-ICPMS. Nuclear field effect appears in purified Nd samples and chromatographic isotopic fractionation after several corrections of the data, including some parameterization (Saji et al., 2016), or this could be an analytical artifact, already reported in MC-ICPMS (e.g., Newman et al., 2009; Xu et al., 2015). In this study, no correction or parameterization of the data has been applied, suggesting that previous report of nuclear field effect on purified Nd samples might be the result of a mathematical artifact.
The three different tests of chromatographic isotopic fractionation (Supplementary Table 2) are also consistent with a negative covariation of Δ148−150Nd′ and δ148Nd, although with poorer precision related to a smaller number of repeat analyses. However, the δ148Nd of the fractions of chromatographic separation correlate well with the accumulated eluted Nd on a probability scale (Figure 4) and consistent with previous findings (Wakaki and Tanaka, 2012). So, chromatographic tests are consistent with the concept of theoretical plates (e.g., Glueckauf, 1955, 1958, Supplementary Material). This implies that chemical equilibrium is reached at each theoretical plate along the column and is associated with isotopic enrichment by liquid chromatography. The size of the Ln-resin column in our experimental technique, and the interpretation of the elution curves, led to a number of theoretical plates in the column around 100 (Supplementary Material). Such small number associated to the time of the elution—typically a few hours—is enough to reach a chemical equilibrium between the Nd bound to the di(2-ethylhexyl) orthophosphoric acid (HDEHP) of the Ln-resin and dissolved Nd in HCl solution. However, this is not enough to reach an isotopic equilibrium since the isotopic data are more consistent with kinetic isotopic fractionation. In such case, and by analogy with the observations on these tests of chromatographic isotopic fractionation, the common industrial process leading to a kinetic isotopic fractionation of anthropogenic materials studied here (Figure 2) could be the chromatographic purification of REEs. For the 148Nd/144Nd ratio, the isotopic fractionation factor between the (Nd3+) (Cl−)3 complex in aqueous solution and the complex Nd-HDEHP is 0.999969 ± 0.000004 for the 148Nd/144Nd ratio (Supporting Material). Per atomic mass unit, this is roughly half of the isotopic fractionation factor between the two complexes of Nd with α-hydroxyisobutyric acid and sulfonic acid (value of 0.999964 ± 0.000003 for the 146Nd/144Nd ratio, Wakaki and Tanaka, 2012). Consequently, the separation of Nd from other REEs by cation-exchange resins is consistent with an equilibration with the resin at each theoretical plate, but a kinetic effect is likely to dominate the isotopic shift. In addition, we can see that with different kinds of REE complexes, we obtain the same systematic of mass-dependent Nd isotopic fractionation: the heavier isotopes fall at the beginning of the elution, together with the tail of the lighter-than-Nd REEs (Ce and Pr, Figure 4). The industrial use of Nd generally requires high purity of Nd (>99%), and a full separation of Nd from light REEs is difficult to achieve (e.g., Rehkamper et al., 1996; Pin and Zalduegui, 1997; Ohno and Hirata, 2013). An easy way to obtain high-purity Nd is to collect only the eluted fraction where other LREEs are not present. In that case, it seems that high-purity Nd will be enriched in light isotopes (Figure 4) by a kinetic mass-dependent isotopic fractionation. In particular, this might explain the enrichment in light Nd isotopes for the Neo compared with the average of terrestrial rocks (Figure 3) associated with the significant presence of Pr (Supplementary Table 3), the REEs that can be found in higher abundance in the Nd fraction after a chromatographic separation (e.g., Pin and Zalduegui, 1997). Also, this hypothesis could explain the enrichment of Nd in light isotopes of many purified Nd samples by comparison with terrestrial rocks (Table 4). The far greater spread in the δ148Nd of anthropogenic materials than in the δ148Nd of terrestrial rocks could correspond to the variability in the industrial process in terms of (1) purity achieved and (2) isotopic fractionation factor dependent on temperature and ligands. Moreover, the industrial purification of Nd might correspond to several steps of purification, enhancing the isotopic fractionation by a Rayleigh-type distillation. In consequence, we propose that an anthropogenic Nd pollution in the environment could be identified by the shift toward more negative δ148Nd values than the range of natural terrestrial samples. More detailed investigations are needed to define precisely the Δ148−150Nd′ of natural terrestrial samples, but geological processes are likely to be characterized by near-equilibrium isotopic fractionation implying that the Δ148−150Nd′ of anthropogenic materials would be higher than the Δ148−150Nd′ of natural terrestrial samples. In that case, the combination of δ148Nd and Δ148−150Nd′ values would be a very distinctive fingerprint of anthropogenic Nd pollution. In particular, soils with the combination of low values for δ148Nd with high values for Δ148−150Nd′ are likely to be polluted by the degradation in the environment of Neo or other industrial products, especially if this is associated with an ε143Nd inconsistent with the ε143Nd of surrounding rocks and soils. In addition, the industrial residue of Nd purification is likely to be characterized by high δ148Nd with low values for Δ148−150Nd′, and the leak or discharge of such residue could also be unambiguously distinguished.
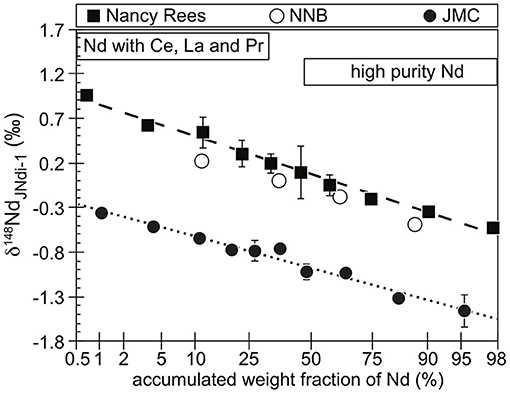
Figure 4. The δ148Nd of the column chromatography experiments plotted against the accumulated weight fraction of Nd in a probability scale. Each Nd solution used in the experiments [JMC, NNB, and Nancy rare earth elements (REEs)] has their own stable Nd isotopic composition. On the experimental purification of Nd in these chromatography experiments, the first Nd to come out of the purification system still bears other REEs (Ce, La, and Pr). If high-purity Nd is required for the industrial purpose, these fractions should not be kept. However, these fraction are also enriched in heavy Nd. As a consequence, high-purity Nd, produced by chromatographic purification, will have a lower δ148Nd than its initial raw material.
Data Availability Statement
The original contributions presented in the study are included in the article/Supplementary Material, further inquiries can be directed to the corresponding author/s.
Author Contributions
AG conceived the study. NB carried out the lab analysis, data processing, and initial interpretation. AG wrote the paper with significant input from NB. All authors contributed to the article and approved the submitted version.
Funding
This research has been funded by the Région Lorraine (Chaîre d'excellence to GA) and the French National Research Agency through the national program Investissements d'Avenir (ANR-10-LABX-21–01/LABEX RESSOURCES21).
Conflict of Interest
The authors declare that the research was conducted in the absence of any commercial or financial relationships that could be construed as a potential conflict of interest.
Acknowledgments
We gratefully acknowledge Damien Cividini and Aimeryc Schumacher for the help during clean lab work and Nd isotope measurement at CRPG, and the three reviewers and the associate editor for their useful suggestions.
Supplementary Material
The Supplementary Material for this article can be found online at: https://www.frontiersin.org/articles/10.3389/fenvc.2021.596928/full#supplementary-material
References
Alonso, E., Sherman, A. M., Wallington, T. J., Everson, M. P., Field, F. R., Roth, R., et al. (2012). Evaluating rare earth element availability: a case with revolutionary demand from clean technologies. Environ. Sci. Technol. 46, 3406–3414. doi: 10.1021/es203518d
Altomare, A. J., Young, N. A., and Beazley, M. J. (2020). A preliminary survey of anthropogenic gadolinium in water and sediment of a constructed wetland. J. Environ. Manage. 255:109897. doi: 10.1016/j.jenvman.2019.109897
Audi, G., Kondev, F. G., Wang, M., Huang, W. J., and Naimi, S. (2017). The NUBASE2016 evaluation of nuclear properties. Chin. Phys. C 41:030001. doi: 10.1088/1674-1137/41/3/030001
Biscaye, P. E., Grousset, F. E., Revel, M., Van der Gaast, S., Zielinski, G. A., Vaars, A., et al. (1997). Asian provenance of glacial dust (stage 2) in the Greenland Ice Sheet Project 2 Ice Core, Summit, Greenland. J. Geophys. Res. Oceans 102, 26765–26781. doi: 10.1029/97JC01249
Bosco-Santos, A., Luiz-Silva, W., and Dantas, E. L. (2018). Tracing rare earth element sources in ucides cordatus crabs by means of 147Sm/144Nd and 143Nd/144Nd isotopic systematics. Water Air Soil. Pollut. 229:365. doi: 10.1007/s11270-018-3990-z
Braun, J.-J., Viers, J., Dupré, B., Polve, M., Ndam, J., and Muller, J.-P. (1998). Solid/liquid REE fractionation in the lateritic system of Goyoum, East Cameroon: the implication for the present dynamics of the soil covers of the humid tropical regions. Geochim. Cosmochim. Acta 62, 273–299. doi: 10.1016/S0016-7037(97)00344-X
Brown, D. N. (2016). Fabrication, processing technologies, and new advances for RE-Fe-B magnets. IEEE Trans. Mag. 52, 1–9. doi: 10.1109/TMAG.2016.2535482
Cánovas, C. R., Macías, F., López, R. P., and Nieto, J. M. (2018). Mobility of rare earth elements, yttrium and scandium from a phosphogypsum stack: environmental and economic implications. Sci. Total Environ. 618, 847–857 doi: 10.1016/j.scitotenv.2017.08.220
Chauvel, C., and Blichert-Toft, J. (2001). A hafnium isotope and trace element perspective on melting of the depleted mantle. Earth Planet. Sci. Lett. 190, 137–151. doi: 10.1016/S0012-821X(01)00379-X
da Silva, Y. J. A. B., do Nascimento, C. W. A., da Silva, Y. J. A. B., Amorim, F. F., Cantalice, J. R. B., Singh, V. P., et al. (2018). Bed and suspended sediment-associated rare earth element concentrations and fluxes in a polluted Brazilian river system. Environ. Sci. Pollut. R. 25, 34426–34437. doi: 10.1007/s11356-018-3357-4
DePaolo, D. J., and Wasserburg, G. J. (1976). Nd isotopic variations and petrogenetic models. Geophys. Res. Lett. 3, 249–252. doi: 10.1029/GL003i005p00249
Diehl, O., Schönfeldt, M., Brouwer, E., Dirks, A., Rachut, K., Gassmann, J., et al. (2018). Towards an alloy recycling of Nd–Fe–B permanent magnets in a circular economy. J. Sustain. Metall. 4, 163–175. doi: 10.1007/s40831-018-0171-7
Dutta, T., Kim, K. H., Uchimiya, M., Kwon, E. E., Jeon, B. H., Deep, A., et al. (2016). Global demand for rare earth resources and strategies for green mining. Environ. Res. 150, 182–190. doi: 10.1016/j.envres.2016.05.052
Elbaz-Poulichet, F., and Dupuy, C. (1999). Behaviour of rare earth elements at the freshwater–seawater interface of two acid mine rivers: the Tinto and Odiel (Andalucia, Spain). Appl. Geochem. 14, 1063–1072. doi: 10.1016/S0883-2927(99)00007-4
Frank, M. (2002). Radiogenic isotopes: tracers of past ocean circulation and erosional input. Rev. Geophys. 40, 1-1-1-38. doi: 10.1029/2000RG000094
Freslon, N., Bayon, G., Toucanne, S., Bermell, S., Bollinger, C., Chéron, S., et al. (2014). Rare earth elements and neodymium isotopes in sedimentary organic matter. Geochim. Cosmochim. Acta 140, 177–198. doi: 10.1016/j.gca.2014.05.016
Galy, A., Belshaw, N. S., Halicz, L., and O'Nions, R. K. (2001). High-precision measurement of magnesium isotopes by multiple-collector inductively coupled plasma mass spectrometry. Int. J. Mass Spectrom. 208, 89–98. doi: 10.1016/S1387-3806(01)00380-3
Glueckauf, E. (1955). Theory of chromatography, part 9, the “theoretical plate” concept in column separations. Trans. Faraday Soc. 51, 34–44. doi: 10.1039/TF9555100034
Glueckauf, E. (1958). Theory of chromatography, Part 11, enrichment of isotopes by chromatography. Trans. Faraday Soc. 54, 1203–1205. doi: 10.1039/TF9585401203
Gwenzi, W., Mangori, L., Danha, C., Chaukura, N., Dunjana, N., and Sanganyado, E. (2018). Sources, behaviour, and environmental and human health risks of high-technology rare earth elements as emerging contaminants. Sci. Total Environ. 636, 299–313. doi: 10.1016/j.scitotenv.2018.04.235
Halicz, L., Galy, A., Belshaw, N. S., and O'Nions, R. K. (1999). High-precision measurement of calcium isotopes in carbonates and related materials by multiple collector inductively coupled plasma mass spectrometry (MC-ICP-MS). J. Anal. At. Spectrom. 14, 1835–1838. doi: 10.1039/a906422b
Hao, X. Z., Wang, D. J., Wang, P. R., Wang, Y. X., and Zhou, D. M. (2016). Evaluation of water quality in surface water and shallow groundwater: a case study of a rare earth mining area in southern Jiangxi Province, China. Environ. Monit. Assess. 188:24. doi: 10.1007/s10661-015-5025-1
Haxel, G. B., Hedrick, J. B., Orris, G. J., Stauffer, P. H., and Hendley, J. W. (2002). Rare Earth Elements: Critical Resources for High Technology. Available online at: https://pubs.usgs.gov/fs/2002/fs087-02 (accessed August 18, 2020). doi: 10.3133/fs08702
Hayles, J. A., Cao, X., and Bao, H. (2017). The statistical mechanical basis of the triple isotope fractionation relationship. Geochem. Perspect. Lett. 3, 1–11. doi: 10.7185/geochemlet.1701
Henry, P., Deloule, E., and Michard, A. (1997). The erosion of the Alps: Nd isotopic and geochemical constraints on the sources of the peri-Alpine molasse sediments. Earth Planet. Sci. Lett. 146, 627–644. doi: 10.1016/S0012-821X(96)00252-X
Hissler, C., Hostache, R., Iffly, J. F., Pfister, L., and Stille, P. (2015). Anthropogenic rare earth element fluxes into floodplains: coupling between geochemical monitoring and hydrodynamic sediment transport modeling. CR Geosci. 347, 294–303. doi: 10.1016/j.crte.2015.01.003
Kolodynska, D., and Hubicki, Z. (2012). “Investigation of sorption and separation of lanthanides on the ion exchangers of various types”, in Ion Exchange Technologies, ed A. Kilislioglu (London: InTech, Publishers), 101–154. doi: 10.5772/50857
Kulaksiz, S., and Bau, M. (2013). Anthropogenic dissolved and colloid/nanoparticle-bound samarium, lanthanum and gadolinium in the Rhine River and the impending destruction of the natural rare earth element distribution in rivers. Earth Planet. Sci. Lett. 362, 43–50. doi: 10.1016/j.epsl.2012.11.033
Lahd Geagea, M., Stille, P., Gauthier-Lafaye, F., and Millet, M. (2008). Tracing of industrial aerosol sources in an urban environment using Pb, Sr, and Nd isotopes. Environ. Sci. Technol. 42, 692–698. doi: 10.1021/es071704c
Li, G., Chen, J., Ji, J., Yang, J., and Conway, T. M. (2009). Natural and anthropogenic sources of East Asian dust. Geology 37, 727–730. doi: 10.1130/G30031A.1
Luais, B., Telouk, P., and Albarède, F. (1997). Precise and accurate neodymium isotopic measurements by plasma-source mass spectrometry. Geochim. Cosmochim. Acta 61, 4847–4854. doi: 10.1016/S0016-7037(97)00293-7
Ma, J., Wei, G., Liu, Y., Ren, Z., Xu, Y., and Yang, Y. (2013). Precise measurement of stable neodymium isotopes of geological materials by using MC-ICP-MS. J. Anal. At Spectrom. 28, 1926–1931. doi: 10.1039/c3ja50229e
McCoy-West, A. J., Millet, M.-A., and Burton, K. W. (2020b). The neodymium stable isotope composition of the oceanic crust: reconciling the mismatch between erupted Mid-Ocean Ridge Basalts and Lower Crustal Gabbros. Front. Earth Sci. 8:25. doi: 10.3389/feart.2020.00025
McCoy-West, A. J., Millet, M.-A., Nowell, G. M., and Burton, K. W. (2017). The neodymium stable isotope composition of the silicate earth and chondrites. Earth Planet. Sci. Lett. 480, 121–132. doi: 10.1016/j.epsl.2017.10.004
McCoy-West, A. J., Millet, M.-A., Nowell, G. M., Nebel, O., and Burton, K. W. (2020a). Simultaneous measurement of neodymium stable and radiogenic isotopes from a single aliquot using a double spike. J. Anal. At. Spectrom. 35, 388–402. doi: 10.1039/C9JA00308H
McLennan, S. M. (2001). Relationships between the trace element composition of sedimentary rocks and upper continental crust. Geochem. Geophys. Geosyst. 2:4. doi: 10.1029/2000GC000109
Merschel, G., Bau, M., Baldewein, L., Dantas, E. L., Walde, D., and Bühn, B. (2015). Tracing and tracking wastewater-derived substances in freshwater lakes and reservoirs: Anthropogenic gadolinium and geogenic REEs in Lake Paranoá, Brasilia. CR Geosci. 347, 284–293. doi: 10.1016/j.crte.2015.01.004
Moss, R. L., Tzimas, E., Kara, H., Willis, P., and Kooroshy, J. (2011). Critical Metals in Strategic Energy Technologies Assessing Rare Metals as Supply-Chain Bottlenecks in Low-Carbon Energy Technologies. Available online at: http://publications.jrc.ec.europa.eu/repository/handle/JRC65592 (accessed August 18, 2020).
Newman, K., Freedman, P. A., Williams, J., Belshaw, N. S., and Halliday, A. N. (2009). High sensitivity skimmers and non-linear mass dependent fractionation in ICP-MS. J. Anal. At Spectrom. 24, 742–751. doi: 10.1039/b819065h
Ohno, T., and Hirata, T. (2013). Determination of mass-dependent isotopic fractionation of cerium and neodymium in geochemical samples by MC-ICPMS. Anal. Sci. 29, 47–53. doi: 10.2116/analsci.29.47
Oliveira, S. M. B., Silva, P. S. C., Mazzilli, B. P., Favaro, D. I. T., and Saueia, C. H. (2007). Rare earth elements as tracers of sediment contamination by phosphogypsum in the Santos estuary, southern Brazil. Appl. Geochem. 22, 837–850 doi: 10.1016/j.apgeochem.2006.12.017
Olmez, I., Sholkovitz, E. R., Hermann, D., and Eganhouse, R. P. (1991). Rare earth elements in sediments off Southern California: a new anthropogenic indicator. Environ. Sci. Technol. 25, 310–316. doi: 10.1021/es00014a015
Piepgras, D. J., Wasserburg, G. J., and Dasch, E. J. (1979). The isotopic composition of Nd in different ocean masses. Earth Planet. Sci. Lett. 45, 223–236. doi: 10.1016/0012-821X(79)90125-0
Pin, C., and Zalduegui, J. F. S. (1997). Sequential separation of light rare-earth elements, thorium and uranium by miniaturized extraction chromatography: Application to isotopic analyses of silicate rocks. Anal. Chim. Acta. 339, 79–89. doi: 10.1016/S0003-2670(96)00499-0
Powell, J. E. (1964). “The separation of rare earths by ion exchange”, in Progress in the Science and Technology of the Rare Earths, ed L. Eyring (Oxford: Pergamon), 62–84.
Ramos, S. J., Dinali, G. S., Oliveira, C., Martins, G. C., Moreira, C. G., Siqueira, J. O., et al. (2016). Rare earth elements in the soil environment. Curr. Pollut. Rep. 2, 28–50. doi: 10.1007/s40726-016-0026-4
Rehkamper, M., Gartner, M., Galer, S. J. G., and Goldstein, S. L. (1996). Separation of Ce from other rare-earth elements with application to Sm–Nd and La–Ce chronometry. Chem. Geol.129, 201–208. doi: 10.1016/0009-2541(95)00143-3
Sagawa, M., Yamamoto, H., Fujimura, S., and Matsuura, Y. (1987). Nd–Fe–B permanent magnet materials. Jpn J. Appl. Phys. 26, 785–800. doi: 10.1143/JJAP.26.785
Saji, N. S., Wielandt, D., Paton, C., and Bizzarro, M. (2016). Ultra-high-precision Nd-isotope measurements of geological materials by MC-ICPMS. J. Anal. At Spectrom. 31, 1490–1504. doi: 10.1039/C6JA00064A
Sanders, L. M., Luiz-Silva, W., Machado, W., Sanders, C. J., Marotta, H., Enrich-Prast, A., et al. (2013). Rare earth element and radionuclide distribution in surface sediments along an estuarine system affected by fertilizer industry contamination. Water Air Soil Pollut. 224:1742. doi: 10.1007/s11270-013-1742-7
Shaw, S., and Constantinides, S. (2012). “Permanent magnets: the demand for rare earths,” in: 8th International Rare Earths Conference (Hong Kong: Arnold Magnetic Technologies).
Shynu, R., Rao, V. P., Kessarkar, P. M., and Rao, T. G. (2011). Rare earth elements in suspended and bottom sediments of the Mandovi estuary, central west coast of India: influence of mining. Estuar. Coast. Shelf Sci. 94, 355–368. doi: 10.1016/j.ecss.2011.07.013
Spedding, F. H., Powell, J. E., and Wheelwright, E. J. (1956). The stability of the rare earth complexes with N-hydroxyethylethylenediaminetriacetic acid. J. Am. Chem. Soc. 78, 34–37. doi: 10.1021/ja01582a007
Steinmann, M., and Stille, P. (1997). Rare earth element behavior and Pb, Sr, Nd isotope systematics in a heavy metal contaminated soil. Appl. Geochem. 12, 607–623. doi: 10.1016/S0883-2927(97)00017-6
Tanaka, T., Ogashi, S., Kamioka, H., Amakawa, H., Kagami, H., Hamamoto, T., et al. (2000). JNdi-1: a neodymium isotopic reference in consistency with LaJolla neodymium. Chem. Geol. 168, 279–281. doi: 10.1016/S0009-2541(00)00198-4
Tepe, N., Romero, M., and Bau, M. (2014). High-technology metals as emerging contaminants: strong increase of anthropogenic gadolinium levels in tap water of Berlin, Germany, from 2009 to 2012. Appl. Geochem. 45, 191–197. doi: 10.1016/j.apgeochem.2014.04.006
Tipper, E. T., Louvat, P., Capmas, F., Galy, A., and Gaillardet, J. (2008). Accuracy of stable Mg and Ca isotope data obtained by MC-ICP-MS using the standard addition method. Chem. Geol. 257, 65–75. doi: 10.1016/j.chemgeo.2008.08.016
Viers, J., and Wasserburg, G. J. (2004). Behavior of Sm and Nd in a lateritic soil profile. Geochim. Cosmochim. Acta 68, 2043–2054. doi: 10.1016/j.gca.2003.10.034
Wakaki, S., and Tanaka, T. (2012). Stable isotope analysis of Nd by double spike thermal ionization mass spectrometry. Int. J. Mass Spectrom. 323–324, 45–54. doi: 10.1016/j.ijms.2012.06.019
Wasserburg, G. J., Jacobsen, S. B., DePaolo, D. J., McCulloch, M. T., and Wen, T. (1981). Precise determination of Sm/Nd ratios, Sm and Nd isotopic abundances in standard solutions. Geochim. Cosmochim. Acta 45, 2311–2323. doi: 10.1016/0016-7037(81)90085-5
Wen, X. J., Duan, C. Q., and Zhang, D. C. (2013). Effect of simulated acid rain on soil acidification and rare earth elements leaching loss in soils of rare earth mining area in southern Jiangxi Province of China. Environ. Earth Sci. 69, 843–853. doi: 10.1007/s12665-012-1969-4
Wilson, D. J., Piotrowski, A. M., Galy, A., and McCave, I. N. (2012). A boundary exchange influence on deglacial neodymium isotope records from the deep western Indian Ocean. Earth Planet. Sci. Lett. 341–344, 35–47. doi: 10.1016/j.epsl.2012.06.009
Xu, L., Hu, Z., Zhang, W., Yang, L., Liu, Y., Gao, S., et al. (2015). In situ Nd isotope analyses in geological materials with signal enhancement and non-linear mass dependent fractionation reduction using laser ablation MC-ICP-MS. J. Anal. At Spectrom. 30, 232–244. doi: 10.1039/C4JA00243A
Yang, Y., Walton, A., Sheridan, R., Guth, K., Gauß, R., Gutfleisch, O., et al. (2017). REE recovery from end-of-life NdFeB permanent magnet scrap: a critical review. J. Sustain. Metall. 3, 122–149. doi: 10.1007/s40831-016-0090-4
Young, E. D., and Galy, A. (2004). The isotope geochemistry and cosmochemistry of magnesium. Rev. Mineral. Geochem. 55, 197–230. doi: 10.2138/gsrmg.55.1.197
Keywords: pollutant, rare earth elements, neodymium, isotopes, permanent magnets, mass dependent fractionation
Citation: Bothamy N and Galy A (2021) Industrially Purified Nd Materials Identified by Distinct Mass-Dependent Isotopic Composition. Front. Environ. Chem. 2:596928. doi: 10.3389/fenvc.2021.596928
Received: 20 August 2020; Accepted: 26 February 2021;
Published: 23 April 2021.
Edited by:
Raoul-Marie Couture, Laval University, CanadaReviewed by:
Wilson Thadeu Valle Machado, Fluminense Federal University, BrazilR. Blaine McCleskey, United States Geological Survey (USGS), United States
Copyright © 2021 Bothamy and Galy. This is an open-access article distributed under the terms of the Creative Commons Attribution License (CC BY). The use, distribution or reproduction in other forums is permitted, provided the original author(s) and the copyright owner(s) are credited and that the original publication in this journal is cited, in accordance with accepted academic practice. No use, distribution or reproduction is permitted which does not comply with these terms.
*Correspondence: Albert Galy, YWdhbHlAY3JwZy5jbnJzLW5hbmN5LmZy