- 1Ministry of Education Key Laboratory of Pollution Processes and Environmental Criteria, Tianjin Key Laboratory of Environmental Remediation and Pollution Control, College of Environmental Science and Engineering, Nankai University, Tianjin, China
- 2Tianjin Key Laboratory of Molecular Optoelectronic Sciences, Department of Chemistry, Collaborative Innovation Center of Chemical Science and Engineering, School of Science, Tianjin University, Tianjin, China
Exploring a unique structure with superior catalytic performance has remained a severe challenge in many important catalytic reactions. Here, we reported a phenomenon that CeO2-based catalysts loaded with different Pt precursors showed a significant difference in the performance of the reduction of NO with H2. The supported platinum nitrate [PtCe(N)] exhibited a superior low-temperature catalytic performance than the supported chloroplatinic acid [PtCe(C)]. In a wide operating temperature (125–200°C), more than 80% NOx conversion was achieved over PtCe(N) as well as excellent thermal stability. Various characterizations were used to study the microstructure and chemical electronic states. Results showed the introduction of a low valence state of Pt species into the CeO2 resulted in the rearrangement of charges on the surface of CeO2, accompanied by increasing contents of oxygen vacancies and Ce3+ sites. Furthermore, the X-ray photoelectron spectroscopy (XPS) and Raman spectra confirmed that the divalent Pt atom could substitute Ce atom to form the Pt-O-Ce3+ structure, which was the base unit in the high-performance PtCe(N) catalyst. The tunable catalytic system of the Pt-O-Ce3+ structure provides a strategy for the design of supported metal catalysts and may as a model unit for future studies of many other reactions.
Introduction
The use and application of diesel engines in industrial processes became widespread due to their good power performance and economy (Pronk et al., 2009). Excessive emission of nitrogen oxides (NOx) can cause serious environmental pollution and severe health issues (Anenberg et al., 2017; Oberschelp et al., 2019). For example, photochemical smog and acid rain were caused by the high toxicity of NOx. Meanwhile, NOx can also damage the lungs and cause respiratory diseases (Han et al., 2019b). Because of the series of hazards, several measures were used to eliminate the effects of NOx emission. Periodic operation in dilute and concentrated combustion phases was applied in NOx storage and reduction (NSR) technology to prevent pollution (Yoshida et al., 2016). A non-negligible amount of reductive gas (H2, HC, and CO) was generated during the rich regeneration period of the diesel lean NOx trap. In addition, H2 molecules still tended toward high activity even at low temperatures and after the catalysts were aged, so that the reduction of NO with H2 as the model was mentioned by researchers (Abdulhamid et al., 2003; Jozsa et al., 2004). Compared to traditional NH3-SCR reaction, H2 as the reductant has advantages of lower temperature activity, cleanliness, and is pollution-free. In view of this situation, great effort has been made to reduce NOx. Nakatsuji et al. achieved the regeneration of Rh-based catalysts with H2 produced during the strong combustion phase (Nakatsuji and Komppa, 2002). Otherwise, Crocker reported that ceria has a good capacity to store oxygen, which could improve the storage capacity of NSR catalysts and thus reduce NOx easily (Ji et al., 2008).
The strategies to improve the catalytic activity of noble metals have been widely investigated (Jiang et al., 2020; Kuai et al., 2020). The unique chemical structure always played an important role in influencing reactions. The recent examples include the following: (1) a Pt1/FeOx single-atom catalyst showed a higher catalytic performance, which greatly improved the utilization of a single atom (Qiao et al., 2011); (2) single Pt atoms were fixed on the surface of defective TiO2 in which way the increased concentration of oxygen vacancy enhanced photocatalytic performance (Chen et al., 2020); and (3) the in-situ formation of a metal bond M-Co (M = Pt, Rh, and Pd) structure during the reaction promoted the reduction of NO (Wang et al., 2013; Nguyen et al., 2016). Based on the above research, the strategy of how to construct a new structure with high performance was of great significance to the development of highly efficient catalysts.
In our work, we proposed and validated a new structure of Pt-O-Ce3+ to remove NOx effectively. The triggering mechanism was confirmed by replacing Ce atoms with Pt atoms of low valence states, described as PtCe(N) catalyst whose Pt atoms were derived from platinum nitrate solution. Then the introduction of such Pt atoms would cause a redistribution of charge on the surface of CeO2, resulting in the increase of oxygen vacancies and Ce3+ sites. When served as a catalyst for reducing NOx, the PtCe(N) catalyst exhibited a superior low-temperature capability of removal of NOx. The results would help to provide a deep understanding of the relationship between structure and efficiency, which was regarded as a valuable strategy for the design and synthesis of new de-NOx catalysts.
Experimental
Catalyst Preparation
The ceria nanorods were synthesized according to the hydrothermal method (Fu et al., 2019). In total, 1.74 g Ce(NO3)3·6H2O was added into 5 ml deionized water with vigorous stirring for 30 min, and 19.2 g NaOH was dissolved in 65 ml deionized water. After Ce(NO3)3·6H2O and NaOH were fully dissolved in water, two solutions were mixed up and stirred for another 30 min. Then, the mixed solution was transferred to a Teflon bottle and further tightly sealed in a stainless-steel autoclave. The thermal procedure of catalysts was performed to heat at 100° for 24 h in an oven. When cooling to room temperature, the solution was washed with deionized water and anhydrous ethanol several times. The solid powder was obtained by drying in air at 80° overnight, and ceria nanorods were obtained by calcining in air at 500° for 4 h with the rate of 5°·min−1.
Ceria-supported different platinum precursors were synthesized by the wet mpregnation method (Spezzati et al., 2017; DeRita et al., 2019). In total, 0.5 g CeO2 was dispersed in 40 ml deionized water, and 2.5 ml (1 mg·ml−1) platinum nitrate [Pt(NO3)2] solution was dropped into the above suspension under vigorously stirring at 70° for 4 h. The precipitate was washed with deionized water and selected via centrifugal mode. After drying overnight at 80°, the sample was calcined at 300° for 4 h in muffle oven. Then the catalysts were obtained, which were described as PtCe(N). Uniformly, the preparation method of PtCe(C) catalysts was the same as that of PtCe(N) catalysts, except for replacing the platinum nitrate with chloroplatinic acid (H2PtCl6). By means of heat treatment and calcination, [PtCl6]2− species were dechlorinated and little Cl ion was detected (Zhang et al., 2019; Ye et al., 2020). Meanwhile, the Pt content was the same on the PtCe(N) and PtCe(C) catalyst (0.204% vs. 0.209%), as determined by ICP-MS. Noteworthily, the Pt atoms from platinum nitrate solution and chloroplatinic acid solution were divalent [Pt(II)] and tetravalent [Pt(IV)], respectively.
Catalyst Characterizations
For the structural analyses of the catalyst, transmission electron microscopy (TEM) and field-emission high-resolution transmission electron microscopy (HRTEM) measurements were carried out on a JEOL JEM 2100F microscope operating at an accelerating voltage of 200 kV. The powder X-ray diffraction (XRD) was carried out on a Rigaku D/MAX 2500v/PC X-ray diffractometer with Cu-Ka radiation (λ = 0.15418 nm). The nitrogen adsorption–desorption isotherms were measured using a Quantachrome Autosorb IQ instrument at −196°C. The specific surface area was calculated by the Brunauer-Emmett-Teller (BET) equation and the pore size distribution was determined using a cylindrical pore model (BJH method). The X-ray photoelectron spectroscopy (XPS) analysis was recorded on a surface analysis system (Thermal ESCALAB 250 spectrometer) equipped with Al Ka monochromatized radiation. The C 1s peak at 284.6 eV was used as a reference, and these kinds of peaks were calibrated by C 1s peak standardized at 284.6 eV. Raman spectra were carried out on a TEO SR-500I-A Raman spectrometer with the excitation laser wavelength of 532 nm. Detailed information on the surface defects was obtained using electron paramagnetic resonance (EPR) performed using an electron paramagnetic resonance spectrometer (Bruker E500) at room temperature. Hydrogen temperature-programmed reduction (H2-TPR) was carried out on a Micromerities Autochem 2920II instrument in order to obtain the reducibility of the catalysts. Firstly, the catalyst (100 mg) was pretreated in Ar (25 ml·min−1) at 100°C for 1 h, and then cooled to ambient temperature. After then, the sample was heated in 10% H2/Ar from room temperature to 1000°C at a heating rate of 10°C·min−1.
Catalytic Tests
The catalytic activity tests were performed at atmospheric with a fixed-bed continuous flow quartz microreactor (inner diameter = 10 mm). In total, 0.1 g catalyst (40~60 mesh) was used to reduce NOx with H2. The flow rate of the reaction gas was 100 ml·min−1 accompanied with 1000 ppm NO, 4000 ppm H2 and balanced with argon, and the corresponding gas hourly space velocity (GHSV) was about 60,000 ml·g−1·h−1. The component and concentration of outlet gas can be obtained by KM-940 flue gas analyzer (Kane International Limited, UK). The NOx conversion rate was calculated by the following equations:
The [NOx]in and [NOx]out noted the inlet and outlet concentration at a steady state and separately ([NOx] = [NO] + [NO2]).
Results and Discussion
Catalytic Activity
Figure 1A showed the conversion of NOx at different temperatures (50–200°) on CeO2 based catalysts with different platinum precursors. Across the whole temperature range, CeO2 catalysts had almost no activity for NOx removal. It proved Pt atoms were the active center in the catalytic reaction directly. Lower than 75°, all catalysts showed poor activity. Compared with PtCe(C) catalysts with rising temperatures, PtCe(N) catalysts exhibited much better activity. Especially the NOx conversion of PtCe(N) catalysts reached 90% at 125°, which showed ~70% efficiency higher than PtCe(C) catalysts. When 100% NOx conversion was achieved over PtCe(N) catalysts from 150 to 200°, the activity of PtCe(C) was still lower though the activity curve was going up. These results showed a platinum nitrate precursor supported on CeO2 supports could greatly promote the performance of catalysts at a lower temperature. Moreover, the thermal stability of catalysts was measured to verify materials' availability. The temperature at 175° was chosen for the test because of its good activity (60 vs. 100%) over both PtCe(C) and PtCe(N) catalysts. Specifically, they behaved perfect thermal stability within constant reaction gas after 48 h (Figure 1B). What's more, activation energy could be used to express the minimum energy required for a reaction to occur, and the magnitude of activation energy could reflect the difficulty of the chemical reaction. As shown in Figure 1C, the apparent energy of reaction catalyzed by PtCe(N) (Ea = 28.5 kJ/mol) was smaller compared with PtCe(C) (Ea = 44.5 kJ/mol) based on Arrhenius plots. It was recognized that the lower the activation energy the better the reaction. The results were consistent with the catalytic activity curve (Figure 1A). Superior catalytic performance of PtCe(N) catalysts implied stronger interaction existed between Pt atoms and CeO2 supports in the reaction, which might due to the introduction of different platinum precursors. So, various characterizations were carried out to investigate the relationship between structure and catalytic activities (Macino et al., 2019).
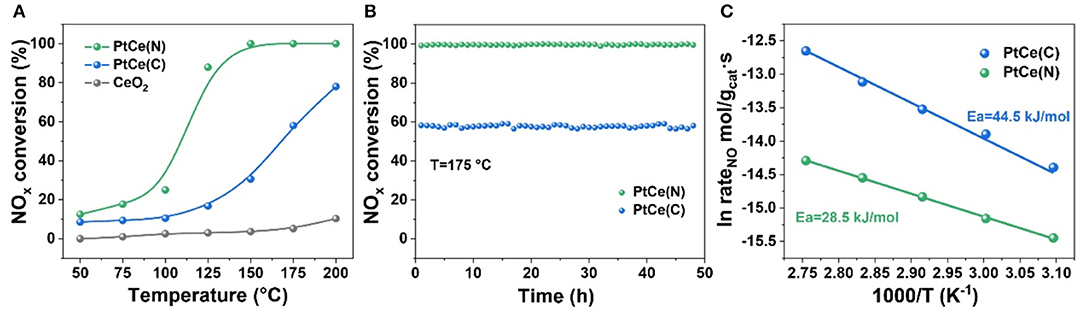
Figure 1. (A) NOx conversion as a function of temperature over CeO2, PtCe(C), and PtCe(N) catalysts. (B) The thermal stability of PtCe(C) and PtCe(N) catalysts. Reaction conditions: 1000 ppm NO, 4000 ppm H2 balanced with Ar, 60 000 ml·g−1·h−1. (C) The arrhenius plot of reaction rate for the PtCe(C) and PtCe(N) catalysts.
Morphology and Structure
To explore reasons for the difference in catalytic performance, the morphology and microstructure were investigated in detail by high-resolution transmission electron microscope (HRTEM) and energy-dispersive X-ray spectroscopy (EDXS) mappings (Figure 2). The images perfectly showed the CeO2 in the shape of nanorods (Figures 2A,B). The lattice fringe was about 3.1 Å, assigned to the interplanar distance of (111) plane of CeO2. Otherwise, EDXS mapping revealed the uniform dispersion of Ce and O elements on CeO2 nanorods (Figure 2C). The Pt atoms were easily loaded at the surface of CeO2 nanorods. The results showed that series of CeO2 based catalysts showed the same phase and similar morphology (Figures 2D–I), indicating that the difference in catalytic performance was not directly related to the micromorphology. Moreover, as shown in Figures 2F,I, EDXS mappings of PtCe(C) and PtCe(N) catalysts showed that Pt atoms were successfully dispersed in all of the CeO2 catalysts (Li et al., 2019). And the content of Cl ion in PtCe(C) was also detected by the TEM-EDXS (Supplementary Figure 1; Supplementary Table 1). The results showed that there was little Cl ion in the sample and far less than the Pt content.
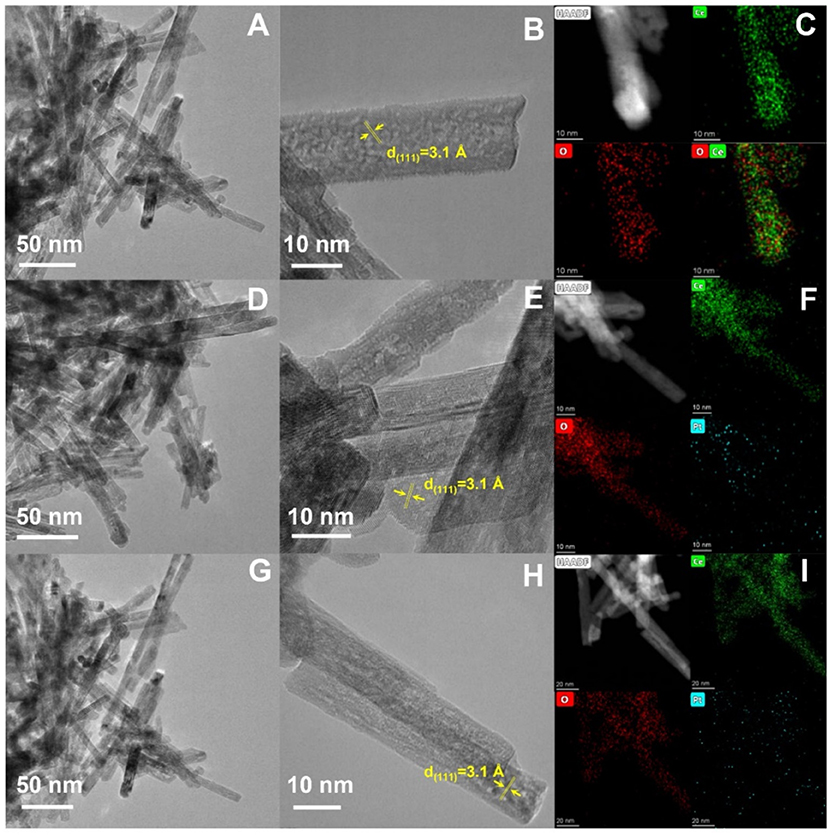
Figure 2. TEM and HRTEM images and corresponding EDXS mappings of CeO2 (A–C), PtCe(C) (D–F), and PtCe(N) (G–I) catalysts.
The effect of Pt atoms incorporation on the structure of CeO2 supports was first verified. The X-ray diffraction (XRD) patterns of catalysts before and after the introduction of Pt atoms were shown in Figure 3A and Supplementary Figure 2. Each sample exhibited a CeO2 spinel phase (PDF NO. 34-0394). It could be seen that CeO2 nanorods had perfect crystallinity with mainly (111) facet exposed, which was extremely consistent with the lattice fringe of 3.1 Å from HRTEM images. In addition, the characteristic peaks at 33.1°, 47.5°, 56.3°, 59.1°, 69.4°, 76.7°, 79.1°, and 88.4° corresponding to the (200), (220), (311), (222), (400), (331), (420), and (422) planes of the CeO2 spinel phase, respectively. Compared with CeO2 catalysts, the crystalline of PtCe(C) and PtCe(N) catalysts tended to increase after the introduction of Pt atoms. The results showed Pt atoms might play an important role to stabilize the crystalline phase of CeO2. In addition, there were no significant changes in the position of characteristic peaks, and no diffraction peaks ascribed to Pt species were detected on catalysts, indicating that Pt species were highly dispersed on catalysts or their grain size were below the detection limit of XRD (Xu et al., 2018, 2019).
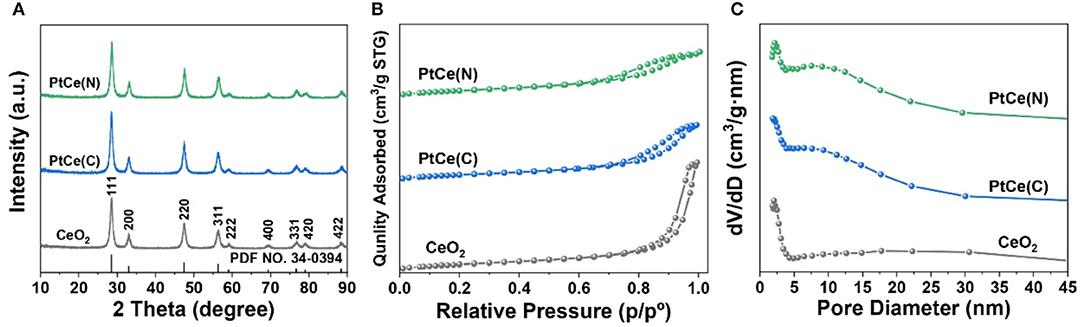
Figure 3. The XRD patterns (A), nitrogen adsorption-desorption isotherm (B) and pore size distribution (C) of CeO2, PtCe(C), and PtCe(N) catalysts.
The N2 adsorption-desorption isotherms on CeO2, PtCe(C), and PtCe(N) catalysts were carried out to explore the specific surface and pore structure after the addition of Pt atoms (Figures 3B,C). All catalysts followed the mesoporous structure curve, and the relevant data were listed in Table 1. The BET-specific surface areas of PtCe(C) (81.16 m2·g−1) and PtCe(N) (87.93 m2·g−1) catalysts were similar to that of CeO2 support (86.35 m2·g−1). But both pore volume and average pore diameter on catalysts loaded with platinum showed a decrease obviously, which might due to blockages caused by Pt atoms in the pores. It was known that Pt atoms are larger in diameter than Ce atoms. It was a possible reason to cause changes of specific surface and pore structure by replacing Ce atoms with Pt atoms.
Surface Element Composition and Status
X-ray photoelectron spectroscopy (XPS) technique was performed to investigate the surface components and valence states of the elements on catalysts (Xu et al., 2020). The normalized band areas of Pt 4f, Ce 3d, and O 1s XPS spectra are shown in Figure 4, and the surface atomic concentrations are listed in Table 2. Figure 4A exhibits the Pt 4f XPS spectrum with two characteristic peaks at 76.3 and 73.0 eV on PtCe(C) catalysts, which showed that Ptδ+ with a high oxidation state (δ was 2~4) was the primary species (Mukri et al., 2013). Different from PtCe(C) catalysts, four peaks of Pt 4f were found at ~75.9, 74.0, 71.9, and 70.6 eV in which the binding energy at 70.6 eV agreed with that of Pt metal and the binding energy at 71.9 eV was about 0.8 eV lower than Pt(II) oxide (Pereira-Hernandez et al., 2019). The results indicated that the Pt atoms over PtCe(N) catalysts tended to a lower valence state between Pt0 and Pt2+. The binding energy of Pt 4f over PtCe(C) and PtCe(N) catalysts was lower than those of Pt(IV) and Pt(II), respectively, suggesting that there was a strong electronic interaction between Pt atoms and CeO2. Another different phenomenon pointed to the O 1s XPS spectrum (Figure 4B). Referring to relevant literature, the peaks at low binding energy from 529.3 to 529.7 eV were ascribed to the lattice oxygen (Olatt), while the peak at high binding energy from 530.9 to 531.1 eV was assigned to the surface-adsorbed oxygen (Oads) (Han et al., 2019a). It was noticed the ratio of Oads/(Oads + Olatt) increased to 27.1% on PtCe(N) catalysts compared to 25.6% for CeO2 supports and 25.5% for PtCe(C) catalysts. The only variable in our system was the introduction of different platinum precursors, suggesting Pt (II) atoms doping could greatly create extra oxygen vacancies. As shown in Figure 4C, the XPS peak of Ce 3d was fitted into 10 overlapping peaks on all catalysts. The peaks assigned to u′ and v′ were the main representatives of the 3d104f1 electronic state of Ce3+, while uo, u, u′′, u′′′, vo, v, v′′, and v′′′ peaks represented the 3d104f0 electronic state of Ce4+ (Wang M. et al., 2019). Results of the Ce3+ content [Ce3+/(Ce3+ + Ce4+)] were shown in Table 2. There were a larger Ce3+ percentage (13.2%) on PtCe(N) catalysts. The Ce3+ rations were higher than those of the PtCe(C) catalysts (10.1%) and CeO2 supports (10.4%). It was known that the formation of Ce3+ was directly related to the presence of oxygen vacancies. Compared with PtCe(C) catalysts, a series of variations proved the substitution of Ce (IV) atoms by Pt (II) atoms on PtCe(N) catalysts generated more oxygen vacancies and Ce3+ sites, forming a structure of Pt-O-Ce3+. The unique Pt-O-Ce3+ structures might enhance the catalytic activity. Otherwise, the used catalysts were also tested with XPS measurement (Figures 4D–I), and no significant changes were found among Pt 4f, Ce 3d, and O 1s XPS spectra, realizing all catalysts were stable before and after the reaction (Wang R. et al., 2019).
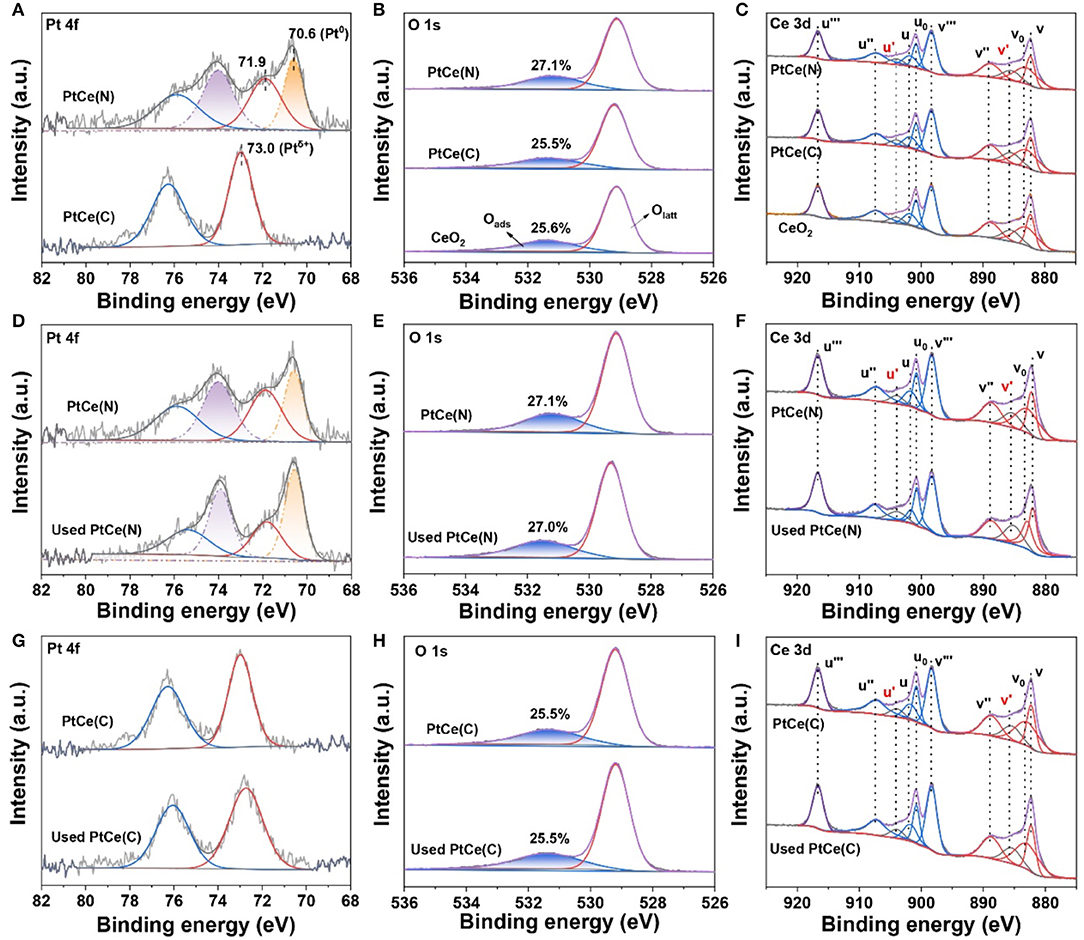
Figure 4. XPS spectra for CeO2, PtCe(N), and PtCe(C) catalysts of Pt 4f, Ti 2p, and O 1s (A–C). XPS spectra for PtCe(N) and used PtCe(N) catalysts of Pt 4f, Ti 2p, and O1s (D–F). XPS spectra for PtCe(C) and used PtCe(C) catalysts of Pt 4f, Ti 2p, and O1s (G–I).
Identification of Pt-O-Ce3+ Structure
The molecular structure and composition of catalysts were further investigated by Raman spectroscopy. As shown in Figure 5A, CeO2 was the crystal possessing the fluorite structure with a space group, and there was only one triply degenerate Raman-active optical phonon with frequency at about 464 cm−1. Except for the characteristic peak at 464 cm−1, there were two other peaks at 598 and 970 cm−1 that appeared after the introduction of Pt atoms. For the Raman spectrum of catalysts at 598 cm−1, it represented the Frenkel-type oxygen vacancies created by the relocation of oxygen anions from tetrahedral sites to octahedral sites, which was caused by the defect sites in the crystal lattice (Guo et al., 2018; Fan et al., 2019). Compared with CeO2 catalysts, the signal at 598 cm−1 was significantly enhanced on the PtCe(C) and PtCe(N) catalysts. And the strongest signals were found over PtCe(N) catalysts, indicating more oxygen vacancies were formed on the surface of CeO2 after introducing Pt atoms. It was consistent with XPS results. Moreover, the peak at 970 cm−1 assigned to the asymmetric structure of Pt-O-Ce was obviously found on PtCe(N) catalysts (Huang et al., 2014; Wang et al., 2015). The formation of the new structure was recognized as the most intuitive evidence that Pt atoms replaced Ce atoms on the surface of CeO2. As a consequence, the charge population around substitution sites was changed after the Pt (II) atoms replaced Ce (IV) atoms. Combined with XPS results, oxygen vacancies were used to balance the reduced valence, accompanied by the increase in Ce3+ content. On the contrary, it was found that a weak signal of Pt-O-Ce structure occurred on PtCe(C) catalysts from the Raman spectrum. Despite the existence of the substitution phenomenon, the introduction of Pt (IV) atoms didn't cause the increase of oxygen vacancies. The difference might directly relate to the activity of the catalytic reaction.
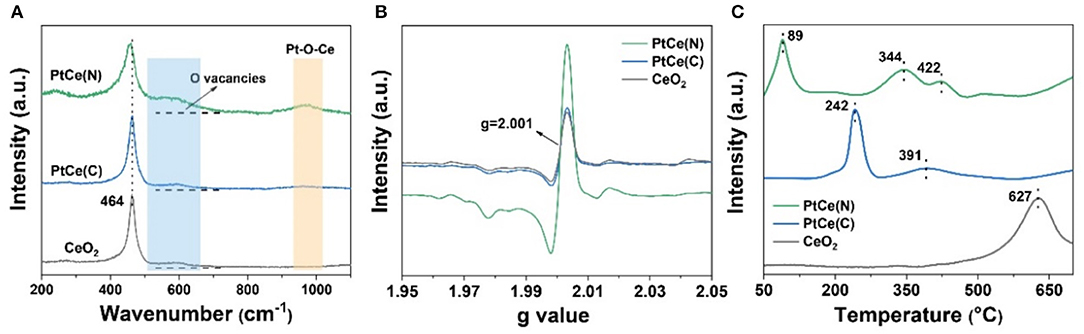
Figure 5. The Raman spectra (A), EPR spectra (B), and H2-TPR profiles (C) of CeO2, PtCe(C), and PtCe(N) catalysts.
The electron paramagnetic resonance (EPR) measurement was further performed to get more information about oxygen vacancies on different catalysts. As shown in Figure 5B, CeO2 had a signal at g = 2.001 at room temperature, which was assigned to the existence of oxygen vacancies (Pan et al., 2013; Li et al., 2018). Similarly, the PtCe(C) catalysts showed the same signal strength as CeO2, indicting constant density of oxygen vacancies after loading with Pt (IV) atoms. It was noted that the EPR signals of PtCe(N) catalysts were remarkably strong at g = 2.001, which was consistent with the results of XPS and Raman. On the basis of the experiment, the defect was attributed to the presence of oxygen vacancies in the lattice, by means of replacing Ce atoms with Pt (II) atoms. As a consequence, the structure of Pt-O-Ce3+ was beneficial to promote the catalytic reaction.
The redox ability of catalysts was confirmed with hydrogen temperature-programmed reduction (H2-TPR) (Figure 5C). For CeO2 supports, there was only one main peak that appeared at 627 cm−1, which was ascribed to the reduction of bulk oxygen of CeO2 (bulk Ce4+ to Ce3+) (Yan et al., 2020). After Pt atoms doped, the peak shifted to a lower temperature range of 340–430° on PtCe(C) (344 and 422°) and PtCe(N) (391°) catalysts, indicating the improvement of redox ability on Pt-based catalysts. Otherwise, the peak loaded at 89° was found on PtCe(N) catalysts, which was attributed to the PtOx species strongly interacting with CeO2 supports. Similarly, a weaker interaction between PtOx species and CeO2 supports was discovered on PtCe(C) catalysts from the peak of 242° (Wang et al., 2015; Duan et al., 2017). The results could well explain the Pt (II) atoms greatly enhanced the interaction between metal and support due to the formation of Pt-O-Ce3+ structures.
Conclusions
In summary, CeO2-based catalysts loading with different Pt precursors were successfully prepared for the reduction of NO with H2. PtCe(N) exhibited excellent de-NOx performance (>80%) over a wide operating temperature (125–200°) as well as excellent thermal stability. According to the results of XPS, Raman, and EPR, Pt (II) atoms of platinum nitrate could replace Ce (IV) atoms on the surface of CeO2 nanorods during the synthetic process, and thus the local charge environment would change accordingly. This might result in an increment of oxygen vacancies and Ce3+ sites, which could balance the negative charge and enhance the electronic metal-support interactions. Furthermore, we proposed a triggering mechanism to form unique structures, and we confirmed the Pt-O-Ce3+ ensemble was the base unit in the high-performance PtCe(N) catalyst. In addition, compared to PtCe(N) catalysts, no obvious changes about surface components and valence states were found on PtCe(C) catalysts, and this was accompanied by a 40% drop in NOx conversion. This work provided a strategy to develop high-efficiency de-NOx catalysts.
Data Availability Statement
The original contributions presented in the study are included in the article/Supplementary Material, further inquiries can be directed to the corresponding author.
Author Contributions
GL, ZH, and SZ designed the research. GL and ZH conducted the fabrication and sample characterizations. XM and NM helped with sample fabrication and processing. GL, ZH, and SZ wrote the manuscript. HZ and YL helped improve the manuscript. All authors listed have made a substantial, direct and intellectual contribution to the work, and approved it for publication and contributed to the discussion of the manuscript.
Funding
This work was supported by the National Natural Science Foundation of China (grant Nos. 22076082 and 21874099), the Tianjin Commission of Science and Technology as key technologies R&D projects (grant Nos. 18YFZCSF00730, 18YFZCSF00770, 18ZXSZSF00230, 19YFZCSF00740, and 20YFZCSN01070).
Conflict of Interest
The authors declare that the research was conducted in the absence of any commercial or financial relationships that could be construed as a potential conflict of interest.
Supplementary Material
The Supplementary Material for this article can be found online at: https://www.frontiersin.org/articles/10.3389/fenvc.2021.672844/full#supplementary-material
References
Abdulhamid, H., Fridell, E., and Skoglundh, M. (2003). Influence of the type of reducing agent (H2, CO, C3H6 and C3H8) on the reduction of stored NOx in a Pt/BaO/Al2O3 model catalyst. Top. Catal. 30, 1–4. doi: 10.1023/B:TOCA.0000029745.87107.b8
Anenberg, S. C., Miller, J., Minjares, R., Du, L., Henze, D. K., Lacey, F., et al. (2017). Impacts and mitigation of excess diesel-related NOx emissions in 11 major vehicle markets. Nature 545, 467–471. doi: 10.1038/nature22086
Chen, Y., Ji, S., Sun, W., Lei, Y., Wang, Q., Li, A., et al. (2020). Engineering the atomic interface with single platinum atoms for enhanced photocatalytic hydrogen production. Angew. Chem. Int. Ed. Engl. 59, 1295–1301. doi: 10.1002/anie.201912439
DeRita, L., Resasco, J., Dai, S., Boubnov, A., Thang, H. V., Hoffman, A. S., et al. (2019). Structural evolution of atomically dispersed Pt catalysts dictates reactivity. Nat. Mater. 18, 746–751. doi: 10.1038/s41563-019-0349-9
Duan, Z., Chi, K., Liu, J., Shi, J., Zhao, Z., Wei, Y., et al. (2017). The catalytic performances and reaction mechanism of nanoparticle Cd/Ce-Ti oxide catalysts for NH3-SCR reaction. RSC Adv. 7, 50127–50134. doi: 10.1039/C7RA06931F
Fan, Z., Wang, Z., Shi, J., Gao, C., Gao, G., Wang, B., et al. (2019). Charge-redistribution-induced new active sites on (001) facets of α-Mn2O3 for significantly enhanced selective catalytic reduction of NO by NH3. J. Catal. 370, 30–37. doi: 10.1016/j.jcat.2018.12.001
Fu, X., Guo, L., Wang, W., Ma, C., Jia, C., Wu, K., et al. (2019). Direct identification of active surface species for the water-gas shift reaction on a gold-ceria catalyst. J. Am. Chem. Soc. 141, 4613–4623. doi: 10.1021/jacs.8b09306
Guo, Y., Mei, S., Yuan, K., Wang, D., Liu, H., Yan, C., et al. (2018). Low-temperature CO2 methanation over CeO2-supported Ru single atoms, nanoclusters, and nanoparticles competitively tuned by strong metal-support interactions and H-spillover effect. ACS Catal. 8, 6203–6215. doi: 10.1021/acscatal.7b04469
Han, L., Gao, M., Feng, C., Shi, L., and Zhang, D. (2019a). Fe2O3-CeO2@Al2O3 nanoarrays on Al-mesh as SO2-tolerant monolith catalysts for NOx reduction by NH3. Environ. Sci. Technol. 53, 5946–5956. doi: 10.1021/acs.est.9b01217
Han, L., Gao, M., Hasegawa, J. Y., Li, S., Shen, Y., Li, H., et al. (2019b). SO2-tolerant selective catalytic reduction of NOx over Meso-TiO2@Fe2O3@Al2O3 metal-based monolith catalysts. Environ. Sci. Technol. 53, 6462–6473. doi: 10.1021/acs.est.9b00435
Huang, H., Dai, Q., and Wang, X. (2014). Morphology effect of Ru/CeO2 catalysts for the catalytic combustion of chlorobenzene. Appl. Catal. B 158–159, 96–105. doi: 10.1016/j.apcatb.2014.01.062
Ji, Y., Choi, J. S., Toops, T. J., Crocker, M., and Naseri, M. (2008). Influence of ceria on the NOx storage/reduction behavior of lean NOx trap catalysts. Catal. Today 136, 146–155. doi: 10.1016/j.cattod.2007.11.059
Jiang, L., Liu, K., Hung, S., Zhou, L., Qin, R., Zhang, Q., et al. (2020). Facet engineering accelerates spillover hydrogenation on highly diluted metal nanocatalysts. Nat. Nanotechnol. 15, 848–853. doi: 10.1038/s41565-020-0746-x
Jozsa, P., Jobson, E., and Larsson, M. (2004). Reduction of NOx stored at low temperatures on a NOx adsorbing catalyst. Top. Catal. 30, 1–4. doi: 10.1023/B:TOCA.0000029837.68739.55
Kuai, L., Chen, Z., Liu, S., Kan, E., Yu, N., Ren, Y., et al. (2020). Titania supported synergistic palladium single atoms and nanoparticles for room temperature ketone and aldehydes hydrogenation. Nat. Commun. 11:48. doi: 10.1038/s41467-019-13941-5
Li, J., Guan, Q., Wu, H., Liu, W., Lin, Y., Sun, Z., et al. (2019). Highly active and stable metal single-atom catalysts achieved by strong electronic metal-support interactions. J. Am. Chem. Soc. 141, 14515–14519. doi: 10.1021/jacs.9b06482
Li, J., Zhou, H., Zhuo, H., Wei, Z., Zhuang, G., Zhong, X., et al. (2018). Oxygen vacancies on TiO2 promoted the activity and stability of supported Pd nanoparticles for the oxygen reduction reaction. J. Mater. Chem. A 6, 2264–2272. doi: 10.1039/C7TA09831F
Macino, M., Barnes, A. J., Althahban, S. M., Qu, R., Gibson, E. K., Morgan, D. J., et al. (2019). Tuning of catalytic sites in Pt/TiO2 catalysts for the chemoselective hydrogenation of 3-nitrostyrene. Nat. Catal. 2, 873–881. doi: 10.1038/s41929-019-0334-3
Mukri, B., Waghmare, U., and Hegde, M. (2013). Platinum ion-doped TiO2: high catalytic activity of Pt2+ with oxide ion vacancy in O2−x compared to Pt4+ without oxide ion vacancy in O2. Chem. Mater. 25, 3822–3833. doi: 10.1021/cm4015404
Nakatsuji, T., and Komppa, V. (2002). A catalytic NOx reduction system using periodic steps, lean, and rich operations. Catal. Today 75, 407–412. doi: 10.1016/S0920-5861(02)00090-1
Nguyen, L., Zhang, S., Wang, L., Li, Y., Yoshida, H., Patlolla, A., et al. (2016). Reduction of nitric oxide with hydrogen on catalysts of singly dispersed bimetallic sites Pt1Com and Pd1Con. ACS Catal. 6, 840–850. doi: 10.1021/acscatal.5b00842
Oberschelp, C., Pfister, S., Raptis, C. E., and Hellweg, S. (2019). Global emission hotspots of coal power generation. Nat. Sustain. 2, 113–121. doi: 10.1038/s41893-019-0221-6
Pan, X., Yang, M., Fu, X., Zhang, N., and Xu, Y. (2013). Defective TiO2 with oxygen vacancies: synthesis, properties, and photocatalytic applications. Nanoscale 5, 3601–3614. doi: 10.1039/c3nr00476g
Pereira-Hernandez, X. I., DeLaRiva, A., Muravev, V., Kunwar, D., Xiong, H., Sudduth, B., et al. (2019). Tuning Pt-CeO2 interactions by high-temperature vapor-phase synthesis for improved reducibility of lattice oxygen. Nat. Commun. 10:1358. doi: 10.1038/s41467-019-09308-5
Pronk, A., Coble, J., and Stewart, P. A. (2009). Occupational exposure to diesel engine exhaust: a literature review. J. Expo. Sci. Environ. Epidemiol. 19, 443–457. doi: 10.1038/jes.2009.21
Qiao, B., Wang, A., Yang, X., Allard, L. F., Jiang, Z., Cui, Y., et al. (2011). Single-atom catalysis of CO oxidation using Pt1/FeOx. Nat. Chem. 3, 634–641. doi: 10.1038/nchem.1095
Spezzati, G., Su, Y., Hofmann, J. P., Benavidez, A. D., DeLaRiva, A. T., McCabe, J., et al. (2017). Atomically dispersed Pd-O species on CeO2(111) as highly active sites for low-temperature CO oxidation. ACS Catal. 7, 6887–6891. doi: 10.1021/acscatal.7b02001
Wang, F., Li, C., Zhang, X., Wei, M., Evans, D., and Duan, X. (2015). Catalytic behavior of supported Ru nanoparticles on the {100}, {110}, and {111} facet of CeO2. J. Catal. 329, 177–186. doi: 10.1016/j.jcat.2015.05.014
Wang, L., Zhang, S., Zhu, Y., Patlolla, A., Shan, J., Yoshida, H., et al. (2013). Catalysis and in situ studies of Rh1/Co3O4 nanorods in reduction of NO with H2. ACS Catal. 3, 1011–1019. doi: 10.1021/cs300816u
Wang, M., Shen, M., Jin, X., Tian, J., Li, M., Zhou, Y., et al. (2019). Oxygen vacancy generation and stabilization in CeO2−x by Cu introduction with improved CO2 photocatalytic reduction activity. ACS Catal. 9, 4573–4581. doi: 10.1021/acscatal.8b03975
Wang, R., Hao, Z., Li, Y., Liu, G., Zhang, H., Wang, H., et al. (2019). Relationship between structure and performance of a novel highly dispersed MnOx on Co-Al layered double oxide for low temperature NH3-SCR. Appl. Catal. B 258:117983. doi: 10.1016/j.apcatb.2019.117983
Xu, H., Liu, J., Zhang, Z., Liu, S., Lin, Q., Wang, Y., et al. (2019). Design and synthesis of highly-dispersed WO3 catalyst with highly effective NH3-SCR activity for NOx abatement. ACS Catal. 9, 11557–11562. doi: 10.1021/acscatal.9b03503
Xu, H., Liu, S., Wang, Y., Lin, Q., Lin, C., Lan, L., et al. (2018). Promotional effect of Al2O3 on WO3/CeO2-ZrO2 monolithic catalyst for selective catalytic reduction of nitrogen oxides with ammonia after hydrothermal aging treatment. Appl. Surf. Sci. 427, 656–669. doi: 10.1016/j.apsusc.2017.08.166
Xu, Q., Fang, Z., Chen, Y., Guo, Y., Guo, Y., Wang, L., et al. (2020). Titania-samarium-manganese composite oxide for the low-temperature selective catalytic reduction of NO with NH3. Environ. Sci. Technol. 54, 2530–2538. doi: 10.1021/acs.est.9b06701
Yan, L., Wang, F., Wang, P., Impeng, S., Liu, X., Han, L., et al. (2020). Unraveling the unexpected offset effects of Cd and SO2 deactivation over CeO2-WO3/TiO2 catalysts for NOx reduction. Environ. Sci. Technol. 54, 7697–7705. doi: 10.1021/acs.est.0c01749
Ye, X., Yang, C., Pan, X., Ma, J., Zhang, Y., Ren, Y., et al. (2020). Highly selective hydrogenation of CO2 to ethanol via designed bifunctional Ir1-In2O3 single-atom catalyst. J. Am. Chem. Soc. 142, 19001–19005. doi: 10.1021/jacs.0c08607
Yoshida, K., Kobayashi, H., Bisaiji, Y., Oikawa, N., and Fukuma, T. (2016). Application and improvement of NOx storage and reduction technology to meet real driving emissions. Top. Catal. 59, 845–853. doi: 10.1007/s11244-016-0558-2
Keywords: NOx removal, oxygen vacancy, Ce3+ site, Pt-O-Ce3+, substitution
Citation: Liu G, Hao Z, Mi X, Ma N, Zhang H, Li Y and Zhan S (2021) The Strategy for Constructing the Structure: Pt-O-Ce3+ Applied in Efficient NOx Removal. Front. Environ. Chem. 2:672844. doi: 10.3389/fenvc.2021.672844
Received: 26 February 2021; Accepted: 01 April 2021;
Published: 13 May 2021.
Edited by:
Dengsong Zhang, Shanghai University, ChinaReviewed by:
Zhiming Liu, Beijing University of Chemical Technology, ChinaJian-Wen Shi, Xi'an Jiaotong University, China
Copyright © 2021 Liu, Hao, Mi, Ma, Zhang, Li and Zhan. This is an open-access article distributed under the terms of the Creative Commons Attribution License (CC BY). The use, distribution or reproduction in other forums is permitted, provided the original author(s) and the copyright owner(s) are credited and that the original publication in this journal is cited, in accordance with accepted academic practice. No use, distribution or reproduction is permitted which does not comply with these terms.
*Correspondence: Sihui Zhan, c2lodWl6aGFuQG5hbmthaS5lZHUuY24=
†These authors have contributed equally to this work