- 1Drug Development Service (SDM), Faculty of Pharmacy, University of Barcelona, Barcelona, Spain
- 2Institute of Research in Microbiology and Medical Parasitology (IMPaM), Faculty of Medicine, University of Buenos Aires-CONICET, Buenos Aires, Argentina
- 3Department of Molecular Biology, Institute of Parasitology and Biomedicine “López-Neyra” (IPBLN-CSIC), Granada, Spain
- 4Department of Cell Biology and Immunology, Institute of Parasitology and Biomedicine “López-Neyra” (IPBLN-CSIC), Granada, Spain
Alternative splicing of pre-mRNA contributes strongly to the diversity of cell- and tissue-specific protein expression patterns. Global transcriptome analyses have suggested that >90% of human multiexon genes are alternatively spliced. Alterations in the splicing process cause missplicing events that lead to genetic diseases and pathologies, including various neurological disorders, cancers, and muscular dystrophies. In recent decades, research has helped to elucidate the mechanisms regulating alternative splicing and, in some cases, to reveal how dysregulation of these mechanisms leads to disease. The resulting knowledge has enabled the design of novel therapeutic strategies for correction of splicing-derived pathologies. In this review, we focus primarily on therapeutic approaches targeting splicing, and we highlight nanotechnology-based gene delivery applications that address the challenges and barriers facing nucleic acid-based therapeutics.
Introduction
The genome is the complete set of DNA that contains all the information necessary for the development and survival of an organism. In humans, the genome is contained in 23 chromosome pairs, comprising approximately 21,000 protein-coding genes and slightly over 3 billion DNA base pairs in total. Although the human genome was sequenced approximately fifteen years ago, there is still disagreement regarding the number of genes due to inconsistencies in the available databases (Willyard, 2018). Regardless of the final count, the numbers of protein-coding genes are similar between humans and worms (∼21,000 and ∼19,000, respectively), and the number found in flies is not drastically lower (∼14,000); however, humans are more complex than these other organisms. The explanation for this apparent paradox may be related to the large predicted number of proteins encoded by the human genome (possibly more than 100,000) as a result of regulatory processes such as alternative transcription, splicing, 3′-end formation, translation and posttranslational modifications. To date, there is no solid evidence (based on, for example, mass spectrometry) to support the existence of this level of complexity in the human proteome. Thus, ambitious projects are being designed to identify and characterize protein variant isoforms for each protein-coding gene (Baker et al., 2017).
Alternative splicing (AS), the phenomenon by which a single precursor (pre-) messenger RNA (mRNA) can generate alternative mRNAs to yield proteins with related or different functions, expands the protein information encoded by the genome. Global transcriptome analyses have estimated that 95–100% of multiexon genes undergo AS (Pan et al., 2008; Wang et al., 2008). The best-known example of the considerable transcriptome diversity resulting from this process is the Drosophila Down syndrome cell adhesion molecule (Dscam) gene, which may give rise to 38,016 cell-surface proteins through AS (Wojtowicz et al., 2007). The functional genome-wide consequences of AS are exemplified by the finding that distinct alternative isoforms encoded by a single gene exhibit distinct protein interaction profiles (Yang et al., 2016). Each of these protein isoforms can be further processed through posttranslational modifications to yield many more distinct proteoforms (Smith et al., 2013) harboring new functions. Recent technological and bioinformatics advances will help to unambiguously decipher the specific sequence and amount of each RNA molecule synthesized by a given cell (Hardwick et al., 2019). In addition, AS approaches specifically designed for single-cell RNA sequencing (scRNAseq) data are emerging, and these approaches may greatly improve our understanding of isoform usage at the single-cell level (Chen et al., 2019).
Alternative splicing in eukaryotes has considerable impacts on a variety of biological pathways; therefore, it is not surprising that AS is a highly orchestrated process involving multiple protein–protein and protein–RNA interactions. The spliceosome, the multiprotein complex that performs the splicing reaction, is composed of a core of five uridine-rich small nuclear RNAs (snRNAs; termed U1, U2, U4, U5, and U6) and 200 other proteins. The spliceosome assembles on pre-mRNA to remove noncoding introns through two sequential transesterification reactions: the branching and exon ligation steps. Detailing all the steps of the AS process is beyond the scope of this review, and comprehensive reviews on the molecular choreography of pre-mRNA splicing have been published elsewhere (Wahl et al., 2009; Matera and Wang, 2014; Wan et al., 2019). In this review, we briefly summarize some aspects of splicing regulation before turning toward advances in therapies and nanodelivery systems targeting splicing for the treatment of human disease.
The major forms of AS include exon skipping, alternative 3′ and 5′ splice site (SS) usage, intron retention, and mutual exon exclusion (Figure 1). Other events that generate different transcript isoforms include alternation of initial exons due to alternative promoter usage and alternation of terminal exons due to alternative polyadenylation. Recognition of exon/intron boundaries for correct intron removal by the splicing machinery requires the presence of several sequence elements on pre-mRNA, including the 5′ and 3′ SSs, the branch point sequence (BPS), and the polypyrimidine (Py) tract. In addition to these core SS motifs, other cis-regulatory elements that recruit specific RNA-binding proteins that either activate or repress the use of adjacent SSs contribute to the fine-tuning and specificity of this pre-mRNA processing event. These sites, known as exonic splicing enhancers (ESEs) or silencers (ESSs) and intronic splicing enhancers (ISEs) or silencers (ISSs), recruit specific trans-acting proteins such as heterogeneous nuclear ribonucleoproteins (hnRNPs) and serine/arginine (SR) proteins (Wu and Maniatis, 1993). AS can also be regulated at the levels of transcription and chromatin structure, adding complexity to the molecular mechanisms that govern splicing control. Two models have been proposed to explain the link between transcription and splicing. The first model, known as the recruitment model, involves the recruitment of splicing factors to pre-mRNA through RNA polymerase II (RNA Pol II) (McCracken et al., 1997). In the second model, the kinetic model, the transcript elongation rate influences AS (de la Mata et al., 2003; Montes et al., 2012; Dujardin et al., 2014; Fong et al., 2014). Chromatin structure, DNA methylation, histone marks, and nucleosome positioning also impact AS by affecting transcription and/or cotranscriptional splicing. An excellent review covering the different levels at which AS is regulated has been published previously (Naftelberg et al., 2015).
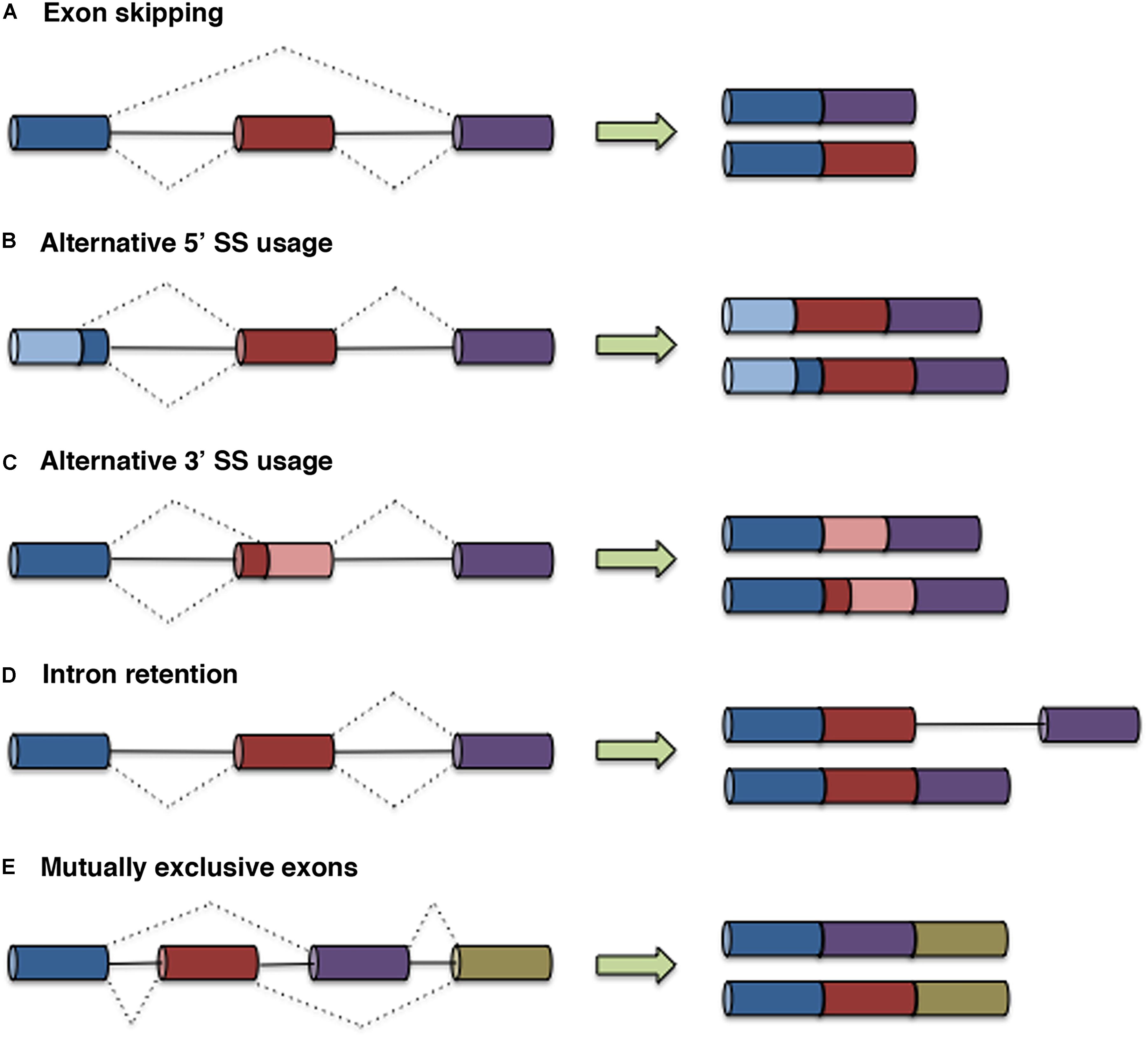
Figure 1. Types of AS. In the graphs, exons are represented by boxes, and introns are represented by lines. Dashed lines indicate AS events. The five main types of AS are illustrated: exon skipping (A), alternative 5′ SS usage (B), alternative 3′ SS usage (C), intron retention (D), and mutually exclusive exons (E).
AS and Disease
Defects in core spliceosome components, trans-acting splicing regulatory factors, cis-regulatory signals, and the transcription rate or changes in chromatin structure or marks can cause multiple pathologies as a result of misprocessing of pre-mRNA, highlighting the importance of RNA processing (Figure 2). To date, 23,868 mutations responsible for human inherited disease that have been reported in the Human Gene Mutation Database (HGMD, accessed in February 2020) have consequences for mRNA splicing (accounting for 8.7% of heritable disease-causing mutations) (Stenson et al., 2012). This number is likely an underestimate, since most of the reported mutations have been identified by genomic DNA sequencing without consideration that missense, nonsense and synonymous changes can affect splicing, as reported previously for coagulation factor IX exon 5 (Tajnik et al., 2016). It has been estimated that up to 50% of mutations that lead to heritable disease occur as a result of errors in the RNA splicing process or its regulation (Lopez-Bigas et al., 2005; Taylor and Lee, 2019). While we wait for functional testing of predicted mutations, the development of new and effective predictive algorithms for splicing effect analysis is critical (Anna and Monika, 2018).
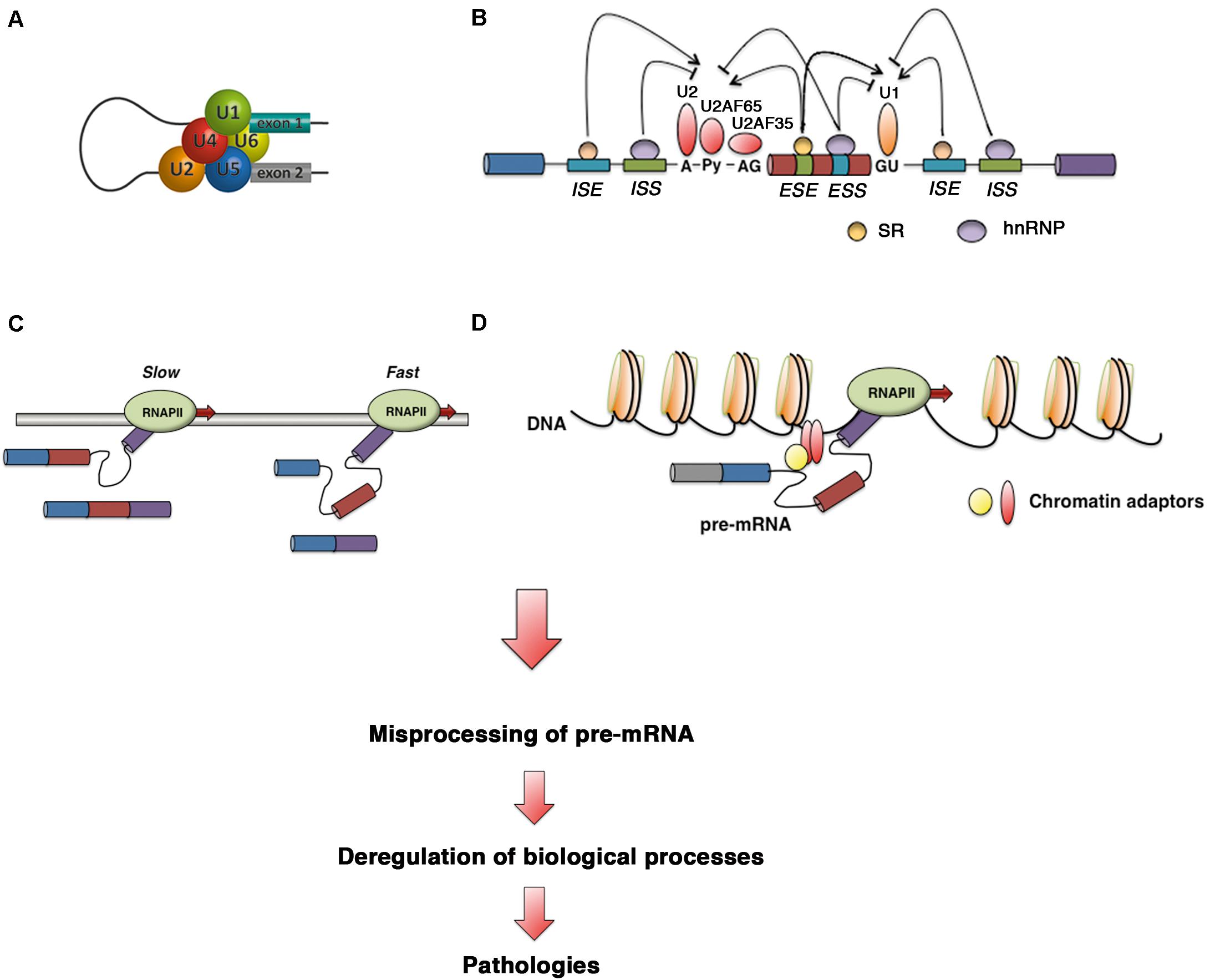
Figure 2. Defective control in pre-mRNA splicing leads to human diseases. Diagram showing how defects in (A) core spliceosome components, (B) trans-acting splicing regulatory factors and cis-regulatory signals, (C) the transcription rate, and (D) chromatin structure or marks can disrupt splicing regulation to cause human disease via misprocessing of pre-mRNA.
Recent advances in the development of novel technologies and tools, such as next-generation sequencing and clustered regularly interspaced short palindromic repeats (CRISPR)/CRISPR-associated protein-9 nuclease (Cas9) genome editing technology, have greatly expanded the available information regarding RNA missplicing events and their association with diseases. These technologies have demonstrated the presence of many naturally occurring genetic variants that affect AS and lead to phenotypic variability and disease susceptibility among humans (Park et al., 2018). For example, 371 splicing quantitative trait loci (sQTLs), including sQTLs in known type 2 diabetes-associated genes or in genes associated with beta cell function and glucose metabolism in human pancreatic islets, have been identified; these sQTLs may aid in elucidation of individual susceptibility to type 2 diabetes (Fadista et al., 2014). Recently, Takata et al., analyzed RNA-seq data on human brain tissues from more than 200 individuals in combination with genotype data and identified approximately 1,500 sQTLs throughout the genome. Interestingly, these researchers observed significant enrichment of epigenetic mark variants that may influence transcriptional activation and AS. In a comparative analysis of genome-wide association study (GWAS) data, many of the observed variants were found to be associated with various human diseases, particularly schizophrenia (Takata et al., 2017).
Mutations that occur in genes encoding fundamental components of the splicing machinery have been described in many splicing-related diseases. However, the frequency of these mutations is low, probably because their effects are incompatible with life. Disease-associated mutations that occur within introns lead to intron retention or to exon skipping upstream or downstream of the mutated SSs without affecting the coding sequence. In contrast, exonic mutations may or may not affect the coding sequence depending on the type of mutation (silent versus missense or nonsense) and can also alter the splicing pattern. Thus, mutations in introns or exons may disrupt RNA secondary structure or disrupt or create de novo cryptic SSs or de novo splicing silencers and enhancers, leading to dysregulation of AS. Mutations or quantitative changes in proteins with regulatory functions during the splicing process can also lead to aberrant splicing, affecting many RNA transcripts at the same time (Havens et al., 2013).
With regard to cancer, genomic studies have identified frequent and recurrent mutations in genes that code for pre-mRNA splicing factors in both hematological malignancies (e.g., myelodysplastic syndrome [MDS], acute myeloid leukemia, and chronic lymphocytic leukemia) (Yoshida et al., 2011) and solid malignancies (e.g., breast cancer, lung cancer, pancreatic cancer and uveal melanoma) (Imielinski et al., 2012; Harbour et al., 2013; Bailey et al., 2016; Nik-Zainal et al., 2016). These findings suggest a potential relationship between certain spliceosome gene mutations and carcinogenesis. For MDS, SF3B1, SRSF2, U2AF1, and ZRSR2 are the four most commonly mutated splicing factor genes, although mutations in other splicing factor genes have also been observed (Taylor and Lee, 2019). Although the underlying mechanisms and contributions of splicing factors in cancer pathogenesis have not been elucidated, and although more work is needed to understand the splicing alterations observed in cancer cells, these data identify novel opportunities for development of splicing-based cancer therapies.
Recent advances in the treatment of some diseases have led to improvements in patient prognosis and life expectancy. For example, spinal muscular atrophy (SMA) type 1, which is considered to be most serious at an early age, can currently be treated with Zolgensma®. Zolgensma® is a new gene therapy-based drug approved by the United States Food and Drug Administration (FDA) that improves the quality of life and life expectancy of infants with SMA type 1. Although this treatment can cure this deadly inherited disease, the Swiss multinational corporation Novartis AG has established a sale price of 2.1 million dollars for a single intravenous administration. This drug is by far the most expensive pharmacological treatment in existence today.
Identification of splicing mutations has significantly advanced our understanding of how splicing dysregulation contributes to disease pathogenesis and of how splicing, a key pre-mRNA processing event, can be targeted for therapeutic applications. In Supplementary Table 1, we provide a list of the most frequent splicing-related human diseases that could be targeted for gene therapy. To learn more, readers are directed to several comprehensive reviews covering human diseases caused by RNA missplicing that have been published elsewhere (Cieply and Carstens, 2015; Daguenet et al., 2015; Chabot and Shkreta, 2016; Scotti and Swanson, 2016).
Therapeutic Approaches
Designing effective therapeutic strategies to overcome the consequences of aberrant splicing events on disease states remains a major challenge. Gene therapy has emerged as a promising pharmacotherapeutic option for patients with diseases of genetic origin. Hence, targeting of aberrant RNA splicing is a logical approach for directly correcting disease-associated splicing alterations without affecting the genome. Other approaches, such as targeting splicing reactions to disrupt the expression of disease-related proteins or targeting exon junctions mutated mRNA to disrupt protein coding, can be used to reframe and rescue protein expression (Havens et al., 2013).
Several strategies have been designed to manipulate the splicing process, including spliceosome-mediated RNA trans-splicing (SMaRT) and the use of antisense oligonucleotides (ASOs), bifunctional oligonucleotides, small-molecule compounds, and modified snRNAs (Figure 3). All of these approaches have been used to correct the effects of RNA misprocessing. In recent years, genome editing techniques involving zinc finger (ZF) proteins (ZFPs), transcription activator-like nucleases (TALENs) and CRISPR/Cas9 systems have become new treatment avenues for correction of splicing defects (Hsu et al., 2014; Fernandez et al., 2017; Knott and Doudna, 2018).
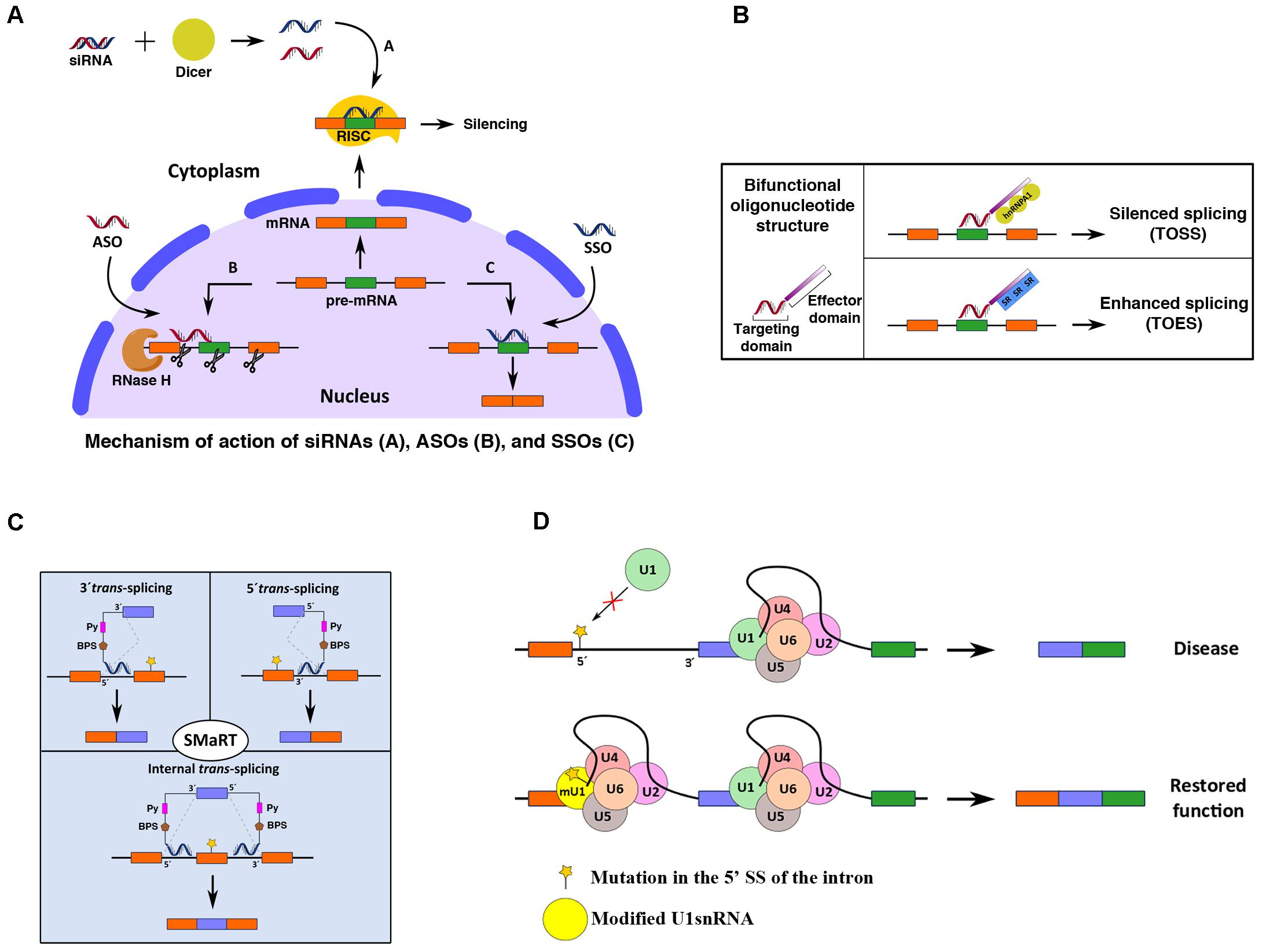
Figure 3. Schematic representation of different splicing-targeting strategies for gene modification. (A) siRNA-, ASO-, and SSO-based strategies (a, b, and c in the figure, respectively) for pre-mRNA editing. (B) Bifunctional oligonucleotides contain one small sequence complementary to the pre-mRNA (targeting domain) and another region (effector domain) that recruits specific regulatory factors to modulate the splicing outcome (TOSS and TOES). (C) Three types of SMaRT approaches are depicted: 5′ trans-splicing, 3′ trans-splicing, and IER, which target the 5′ end, 3′ end, or internal portion of a mutated target pre-mRNA, respectively. See the text for further details on these RNA splicing-editing mechanisms. (D) Schematic representation of the general strategy for correction of splicing defects using modified snRNAs. The cartoon shows the restoration of a mutated 5′ SS of an intron using a modified U1 snRNA.
RNA Editing Approaches
Antisense Oligonucleotides (ASOs)
Antisense oligonucleotides strategies use short synthetic single-stranded DNA molecules that are complementary to a specific pre-mRNA sequence to alter the splicing process. ASO binding to a target pre-mRNA in the nucleus sterically blocks the recruitment of trans-acting proteins to the pre-mRNA sequence at the target site (Figure 3A).
ASOs can be designed to target (i) the SS, thereby redirecting splicing to an adjacent site; (ii) auxiliary sequences (enhancers or silencer elements) within the immature transcript, thereby modifying the outcome of the splicing reaction (by blocking or promoting splicing); and (iii) RNA bases, thereby stabilizing/destabilizing regulatory structures and modifying the splicing outcome (Havens et al., 2013; Sune-Pou et al., 2017).
Splicing-related ASOs that act according to the first two mechanisms mentioned above by promoting or redirecting splicing are also called splice-switching oligonucleotides (SSOs). These short, 15- to 30-nucleotide-long sequences sterically block important motifs in pre-mRNA (i.e., SSs and/or regulatory sequences) to prevent RNA–RNA base-pairing or protein–RNA binding interactions between spliceosome components and pre-mRNA without promoting degradation of the RNA transcript while altering splicing outcomes (Havens and Hastings, 2016). The nucleotides of an SSO are chemically modified (e.g., into morpholino antisense oligomers) such that the RNA-cleaving enzyme RNase H is not recruited to degrade the pre-mRNA–SSO complex (Summerton, 1999). This property differs from the RNase H activity exerted by conventional ASOs, which inhibits gene expression by degrading the target pre-mRNA. Chemical modifications of ASOs are also crucial because they stabilize the ASOs in vivo and improve their cellular uptake, release and binding affinity for their targeted RNA sequences; unmodified oligonucleotides are highly susceptible to degradation by circulating nucleases and are excreted by the kidneys. Examples of these chemical modifications include changes to the phosphate backbones and/or sugar components of the oligonucleotides, such as the use of a phosphorothioate backbone (first-generation ASOs) (Eckstein, 2014), the use of locked nucleic acid chemistry for bridging of the sugar furanose ring (Campbell and Wengel, 2011), alterations at 2′ positions of the ribose sugar ring (2′-O-methylation [2′-OMe] and 2′-O-methoxyethylation [2′-MOE]) (second-generation ASOs) (Geary et al., 2001), and the addition of phosphorodiamidate morpholinos (third-generation ASOs) (Summerton, 1999).
The clinical application of this technology has resulted in the commercialization of VitraveneTM (fomivirsen), which, in 1998, became the first ASO approved by the FDA for the treatment of AIDS-related cytomegalovirus retinitis; MacugenTM (pegaptanib), approved by the FDA in 2004 for the treatment of neovascular age-related macular degeneration; and KynamroTM (mipomersen), approved by the FDA in 2013 for the treatment of homozygous familial hypercholesterolemia. These products have been withdrawn from the market for commercial reasons owing to an overall small patient population and competing alternative drugs, such as statins in the case of familial hypercholesterolemia (Sharma and Watts, 2015). The first published report on the use of ASOs as a splicing-targeting therapeutic tool was published by Dominski and Kole in 1993. These authors restored correct splicing in thalassemic pre-mRNA by using a 2′-OMe ASO (Dominski and Kole, 1993). Since then, many ASO strategies have been designed to modify splicing for the treatment of several diseases, and some of them are currently in clinical trials. Only two ASOs are already FDA approved (Supplementary Table 2). Exondys 51TM (eteplirsen) was the first drug in its class to be approved by the FDA (in September 2016) under the Accelerated Approval Program to treat patients with Duchenne muscular dystrophy (DMD). Exondys 51TM belongs to the third generation of phosphorodiamidate morpholino ASOs and is specifically indicated for patients who have a confirmed mutation of the dystrophin gene amenable to exon 51 skipping. This mutation affects approximately 13% of the population with DMD. Recently, Vyondys 53TM (golodirsen), a DMD drug that is highly similar to eteplirsen except that involves exon 53 skipping rather than exon 51 skipping, was denied by the FDA in August 2019 because of the risk of infections related to intravenous infusion ports and renal toxicity seen in preclinical studies. A similar decision was reached in January 2016 for the promising drug KyndrisaTM (drisapersen). This drug was intended for the treatment of patients with DMD amenable to exon 51 skipping but failed to demonstrate substantial effectiveness. The pharmaceutical company BioMarin invested over $66 million in the development of drisapersen; as a consequence of the denial, BioMarin also discontinued the development of three follow-on products of drisapersen, BMN 044, BMN 045, and BMN 053. These products were in mid-stage trials for specific forms of the muscle-wasting disease.
In December 2016, the FDA approved SpinrazaTM (nusinersen), the first drug approved for the treatment of SMA the first drug approved for the treatment of SMA (Lorson et al., 1999; Monani et al., 1999). SpinrazaTM is a 2′-OMe phosphorothioate ASO that targets an intron 7 internal SS within SMN2 pre-mRNA, inducing exon 7 inclusion and producing a functional SMN protein. Notably, because ASOs generally do not cross the blood–brain barrier, repeated intrathecal nusinersen delivery is required. This requirement is highly disadvantageous and makes administration challenging, especially for infants (Verma, 2018).
Investigational ASOs for Huntington’s disease (HD), amyotrophic lateral sclerosis (ALS) and transthyretin (TTR) amyloidosis, such as RG6042, tofersen and inotersen, respectively, are currently in phase III clinical trials. RG6042 (previously known as IONIS-HTTRx) reduces the concentration of mutant huntingtin (HTT) levels in the cerebrospinal fluid of patients with HD without causing serious adverse events (Tabrizi et al., 2019). Tofersen (previously known as BIIB067) targets superoxide dismutase (SOD1) in ALS patients, reducing SOD1 concentrations in spinal fluid to preserve motor neurons and slow the progression of the disease. Inotersen is a 2′-MOE-modified ASO that reduces the production of TTR and improves disease course and quality of life in early hereditary TTR amyloidosis polyneuropathy (ATTR) (Mathew and Wang, 2019).
The development of the ASO milasen is an example of how cutting-edge medicine can be used with great speed for patient-customized treatment of a rare and fatal neurodegenerative disease (ceroid lipofuscinosis 7, CLN7, a form of Batten’s disease). Researchers at Boston Children’s Hospital identified a novel mutation in a 6-year-old girl, designed and produced an ASO, and obtained FDA approval for its clinical deployment in less than one year (Kim et al., 2019).
ASOs are also applicable to cancer treatment. For instance, Dewaele et al., used ASO-mediated exon skipping to decrease the expression of MDM4, a splice isoform produced in cancer cells (Dewaele et al., 2016). Similarly, Hong et al. preclinically and clinically evaluated a chemically modified ASO termed AZD9150 that targets the STAT3 gene, a transcriptional activator and oncogenic mediator of the JAK-STAT signaling pathway (Hong et al., 2015). Ross et al. described the use of an ethyl-containing ASO (AZD4785) to downregulate KRAS mRNA, which is mutated in approximately 20% of human cancers, and demonstrated its efficacy in preclinical KRAS mutant lung cancer models (Ross et al., 2017).
Antisense oligonucleotides have been validated as therapeutic agents; however, because of the high cost associated with these products, improvements must be made to prevent health insurance companies from denying patients access. For instance, Exondys 51TM is priced at $300,000 per patient per year even though the efficacy of this drug is controversial.
Bifunctional Oligonucleotides
Bifunctional oligonucleotides are ectopic modulators of AS used to control the patterns of splicing of specific genes. In brief, these oligonucleotides contain two parts: (i) an antisense portion targeting a specific sequence and (ii) a nonhybridizing tail or effector domain that recruits acting factors (targeted oligonucleotide enhancer of splicing [TOES] or targeted oligonucleotide silencer of splicing [TOSS]) (Brosseau et al., 2014) (Figure 3B). Oligonucleotides containing binding site sequences for the splicing repressor hnRNP A1/A2 have been used to reprogram AS via TOSS. The oligonucleotides were positioned upstream of a 5′ SS to interfere with U1 snRNP binding and repress SS use (Villemaire et al., 2003). Dikson et al. used a TOSS with hnRNPA1 tails to block the inclusion of exon 8 in SMN2, thereby favoring exon 7 inclusion and restoring the functionality of the protein (Dickson et al., 2008). Similarly, bifunctional TOES, whose tail of enhancer sequences recruits activating proteins such as positively acting SR proteins, has been used to increase the splicing of refractory exon 7 in SMN2 in fibroblasts derived from patients with SMA (Skordis et al., 2003; Owen et al., 2011). This approach is mechanistically different than ASO approaches, although both can be used for stimulating the inclusion of exon 7 in SMA. Whereas ASOs such as SpinrazaTM block the binding of splicing factors to intron 7, causing exon 7 inclusion, bifunctional TOSS/TOES is intended to direct exon 7 inclusion and thus restore protein expression.
Small Interfering RNAs (siRNAs)
In 1998, another related technology emerged with the discovery of the siRNA pathway, which can be used to silence the expression of genes (Fire et al., 1998). The discovery of RNA interference (RNAi), in which double-stranded RNA (dsRNA) is hybridized with a specific mRNA sequence to induce its silencing or degradation, was a major scientific breakthrough for which Craig Mello and Andrew Fire were recognized with the Nobel Prize in Physiology and Medicine in 2006. This discovery revolutionized the way scientists study gene function and offered an innovative strategy for the treatment of diseases, particularly those of genetic origin, as demonstrated for the first time by Elbashir et al. (2001). Through this strategy, administration of synthetic 21- to 25-nucleotide duplexes with overhanging 3′ ends (siRNAs) can be used to suppress the expression of endogenous and heterologous genes (Figure 3A). Although many classes of small RNAs have emerged, three main categories are widely recognized: siRNAs; microRNAs, or miRNAs; and piwi-interacting RNAs, or piRNAs. These RNA types differ in structure, biological roles, associated effector proteins and origins (Dana et al., 2017). Physiologically, in cells, siRNAs help to maintain genomic integrity by preventing the action of foreign nucleic acids, including those of viruses, transposons and retrotransposons and transgenes, while miRNAs act as posttranscriptional endogenous gene regulators (Meister and Tuschl, 2004). piRNAs have been implicated in the silencing of retrotransposons at both the posttranscriptional and epigenetic levels as well as other genetic elements in germlines, particularly those activated during spermatogenesis (Siomi et al., 2011). Upon delivery into cells, siRNAs are bound by a multiprotein component complex, known as the RNA-induced silencing complex (RISC), in the cytoplasm; the two strands are then separated, and the strand with the RISC hybridizes with the target mRNA. After that step, Argonaute-2 (Ago2), a catalytic component of the RISC, drives mRNA cleavage (Dana et al., 2017). Since siRNA-mediated targeting of aberrant splicing isoforms is widely used as an RNAi technology, only siRNA approaches will be discussed in detail in this section (Figure 3A).
These siRNA approaches can be used to target aberrant splicing isoforms for therapeutic applications (Sune-Pou et al., 2017). Indeed, siRNAs targeting exonic/intronic sequences close to alternative exon or exonic/intronic junction sequences can induce degradation of alternatively spliced and aberrant mRNAs without affecting the expression of normal mRNAs. Such targeting approaches have been used for diseases such as Ullrich congenital muscular dystrophy (UCMD) (Bolduc et al., 2014), growth hormone deficiency (GHD) type II diseases (Ryther et al., 2004) and several cancers. In the context of cancer, it has been observed that the occurrence of specific splice variants is increased during tumorigenesis and that the splicing regulatory machinery is abnormal in many malignant cells (Hayes et al., 2006). Bolduc et al. designed different siRNAs targeting the most frequent mutation that causes exon 16 skipping in the COL6A3 gene and tested the siRNAs in vitro in UCMD-derived dermal fibroblasts. These siRNAs resulted in specific knockdown of the mutant allele and increased the abundance and quality of collagen VI matrix production (Bolduc et al., 2014). Similarly, Ryther et al. used siRNAs to specifically degrade exon 3-skipped transcripts in a GHD type II disease (Ryther et al., 2004). Finally, Hayes et al. have shown that siRNA-mediated downregulation of SR protein kinase 1 (SRPK1), which is significantly upregulated in tumors of the pancreas, breast and colon, decreases cell proliferation and increases apoptosis. Moreover, the sensitivity of tumor cells to chemotherapeutic agents such as gemcitabine and cisplatin increases upon treatment with this siRNA (Hayes et al., 2006).
In October 2016, the development of revusiran, an siRNA designed for the treatment of ATTR amyloidosis, was suspended after a randomized double-blind placebo-controlled phase III trial of its efficacy and safety, ENDEAVOUR, demonstrated that the siRNA caused greater mortality than a placebo (18 of 206 enrolled patients). However, in August 2018, the FDA approved OnpattroTM (patisiran), the first siRNA-based drug for the treatment of hereditary TTR-mediated amyloidosis (hATTR) (Supplementary Table 2). Hence, siRNA-based technology has shown promising therapeutic results and the possibility of translation into clinical use.
Small-Molecule Compounds
Small-molecule compounds can also be used to modulate RNA expression. Some molecules are capable of binding specific three-dimensional RNA structures, thereby preventing their translation or function. Furthermore, these compounds can also modify splicing factor activity (by affecting posttranslational modifications of splicing factors) or directly alter splicing events. Compared with oligonucleotide-based therapeutics, these compounds are easier to deliver to target sites and normally have lower toxicity profiles. However, small-molecule compounds frequently act through unknown mechanisms, resulting in a lack of information, and have less target specificity than other therapeutic formulations, thus potentially exhibiting more nonspecific and off-target effects. While oligonucleotides (ASOs, TOSS, TOES and siRNAs) can very specifically and efficiently modulate particular RNA targets upon complementary recognition of the RNA sequences, small molecules can recognize specific three-dimensional structures and overcome known oligonucleotide drawbacks, e.g., poor pharmacological properties.
Some small molecules have already been approved for use in clinical practice for applications other than splicing defect correction. For example, digoxin and other prescribed cardiotonic steroids, routinely used in the treatment of heart failure, have been described as modulators of AS (Stoilov et al., 2008). Pentamidine and Hoechst 33258 also modulate AS in myotonic dystrophy (DM) by disrupting MBNL1 binding to CUG in vitro and in vivo (Warf et al., 2009; Parkesh et al., 2012). The histone deacetylase sodium butyrate, which is known to upregulate the expression of splicing factors, has been demonstrated to increase CFTR transcript levels, leading to activation of CFTR channels and restoration of their function in CFTR-derived epithelial cells (Nissim-Rafinia and Kerem, 2006). It has also been reported that the plant cytokinin kinetin improves IKBKAP mRNA splicing in patients with familial dysautonomia (FD) (Axelrod et al., 2011).
Some small-molecule splicing modulators have been evaluated in clinical trials for the treatment of solid tumors and leukemia. For instance, E7107 (pladienolide B) is a splicing modulator whose preferential cytotoxicity is positively influenced by some antiapoptotic BCL2 family genes, such as BCL2A1, BCL2L2 and MCL1. Furthermore, Aird et al. have reported that combinations of E7107 and BCLxL (BCL2L1-encoded) inhibitors enhance cytotoxicity in cancer cells (Aird et al., 2019). Notably, E7107 was the first compound of a new class of anticancer agents targeting the spliceosome. Specifically, E7107 interacts with subunit 1 of SF3b to block the normal splicing of oncogenes. Unfortunately, the development of E7107 was suspended after phase I clinical trials due to an unacceptable profile of adverse events. Herboxidienes, spliceostatin A, meayamycin B and sudemycin D6/K have also been shown to exhibit antitumoral activity in vivo by targeting the SF3b subunit of the spliceosome (Lin, 2017). Recently, Carabet et al. reported VPC-80051 as the first small-molecule inhibitor of hnRNPA1, which plays an important role in cancer by controlling the transcriptional levels of the oncoprotein c-Myc (Carabet et al., 2019). Thus, small molecules that selectively inhibit hnRNP A1-RNA interactions can be designed for the treatment of tumors expressing cancer-specific alternatively spliced proteins. Similarly, highly specific inhibitors of the RNA helicase Brr2, which is an essential component of the spliceosome, have been designed for therapeutic purposes (Iwatani-Yoshihara et al., 2017).
Recently, the Massachusetts-based pharmaceutical company Skyhawk Therapeutics invested $100 million in the development of small-molecule compounds capable of correcting misspliced exon skipping related to cancer and neurological diseases. Novartis and Roche have also independently developed two different splicing-modulating compounds for the treatment of SMA: branaplam and risdiplam, respectively. Both molecules enhance exon 7 inclusion to increase the levels of functional SMN protein. Branaplam, also known as LMI070, is an orally available drug that was designed by Novartis using a high-throughput phenotypic screening approach with approximately 1.4 million compounds. Currently, this molecule is in a phase II clinical trial that is expected to be completed in July 2020.
Risdiplam (Ratni et al., 2018) is a brain-penetrant orally administered drug that is currently being evaluated in patients with SMA in four multicenter clinical trials (NCT02913482, NCT02908685, NCT03032172, and NCT03779334). This drug is a splicing modulator that increases exon 7 inclusion in the SMN2 gene, thereby increasing the levels of SMN protein throughout the organism. Furthermore, this drug is being studied for use in patients of all age ranges with SMA types 1, 2, and 3.
All these examples demonstrate the applicability of small molecules for splicing event modulation and suggest that these molecules are useful as complements and alternatives to oligonucleotides.
SMaRT
Spliceosome-mediated RNA trans-splicing is a gene-reprogramming system based on the trans-splicing process that can be used for therapeutic applications. The trans-splicing methodology is designed to correct aberrant mRNAs by replacing the entire coding sequence upstream or downstream of a target SS. Three different components are involved: the target mRNA, the spliceosome machinery and the pre-trans-splicing molecule (PTM), also known as the RNA trans-splicing molecule (RTM). The first two components are present in cells, while the third must be provided exogenously. Trans-splicing is induced between the exogenous RNA and the endogenous pre-mRNA, producing a chimeric RNA with the wild-type sequence (without mutation/s). To achieve successful correction, the PTM must be correctly designed with the following regions: a binding domain (complementary to the pre-mRNA), a splicing domain (incorporating 5′ and 3′ SS, intronic BPS and Py sequences) and a coding domain (containing the wild-type coding region) (Wally et al., 2012; Berger et al., 2016; Figure 3C).
Depending on the targeted region of the pre-mRNA, SMaRT can be divided into (i) 5′-trans-splicing, which targets the 5′ portion; (ii) 3′-trans-splicing, which targets the 3′ portion; and (iii) internal exon replacement (IER), which targets an internal portion of the pre-mRNA (Figure 3C). SMaRT approaches have been used in models of cystic fibrosis (CF) (Song et al., 2009), hemophilia A (Chao et al., 2003), SMA (Coady and Lorson, 2010), retinitis pigmentosa (RP) (Berger et al., 2015), frontotemporal dementia with parkinsonism-17 (FTDP-17) and tauopathies (Rodriguez-Martin et al., 2005, 2009). The results have shown that this technology can successfully reprogram gene expression and offers promising gene therapy applications. However, trans-splicing approaches require the use of vectors for the delivery of the PTM into cells. Therefore, selection of a good delivery vector is critical for future treatment approaches based on this technology (Wally et al., 2012). SMaRT offers multiple advantages as a gene therapy tool; however, it needs to be better understood and optimized in order to increase its overall efficiency.
Modified snRNAs
Exon-specific U1 snRNAs (ExSpe U1s) are modified U1 snRNAs complementary to intronic regions downstream of the 5′ SS that can be used to eliminate the skipping of some exons caused by different mutations (Figure 3D). ExSpe U1s have been tested in different models and have shown potential for use in therapeutic applications (Dal Mas et al., 2015). In one study, different ExSpe U1s were tested for the treatment of SMA, CD and hemophilia B in SMN2 exon 7, CFTR exon 12 and F9 exon 5 models, respectively (Fernandez Alanis et al., 2012). Similar approaches have been reported for ATP8B1 deficiency (van der Woerd et al., 2015), FD (Donadon et al., 2018b), Sanfilippo syndrome type C (Matos et al., 2014), Fanconi anemia (Mattioli et al., 2014), RP (Tanner et al., 2009), thalassemia (Gorman et al., 2000), severe coagulation factor VII (FVII) deficiency (hemophilia A, HA) (Pinotti et al., 2008; Donadon et al., 2018a; Balestra et al., 2019b), CDKL5-deficiency disorder (CDD) (Balestra et al., 2019a), Seckel syndrome (Scalet et al., 2017), and hereditary tyrosinemia type I (HT1) (Scalet et al., 2018).
Other modified versions of spliceosomal snRNAs have also been tested for their usefulness in restoration of base pairing to the mutated SS. For example, combined treatment with mutation-adapted U1 and U6 snRNAs has been used to correct mutation-induced splice defects in exon 5 of the BBS1 gene (Schmid et al., 2013); this gene is implicated in Bardet-Biedl syndrome, which causes retinal degeneration and developmental disabilities. Another example of a modified oligonucleotide is U7 snRNA, which participates in histone pre-mRNA maturation by recognizing the sequence of the histone 3′ untranslated region. Changes in the target sequence can be introduced to convert this snRNA into an antisense tool capable of blocking splicing signals and inducing exon skipping or inclusion (Brun et al., 2003). This strategy has been used to design artificial U7 snRNAs to enhance exon 23 skipping of mutated dystrophin in DMD (Brun et al., 2003; Goyenvalle et al., 2004).
The modified snRNA approach is based on engineered variants of small coding genes and has various advantages. Its main advantages are the possible exploitation of virtually any viral vector and the fact that, based on its molecular mechanisms, it does not alter the physiological expression of the target gene.
Genome Editing Approaches
ZFPs
Zinc finger proteins are powerful and widely studied tools for efficient establishment of targeted genetic modifications (Cristea et al., 2011). ZFPs designed for therapeutic use consist of ZF arrays in which every ZF element recognizes three bases of a DNA sequence through an α-helix structure (Figure 4A). Examples include zinc finger nucleases (ZFNs) that cleave DNA and zinc finger transcription factors (ZFP-TF) that modulate gene expression (Wild and Tabrizi, 2017).
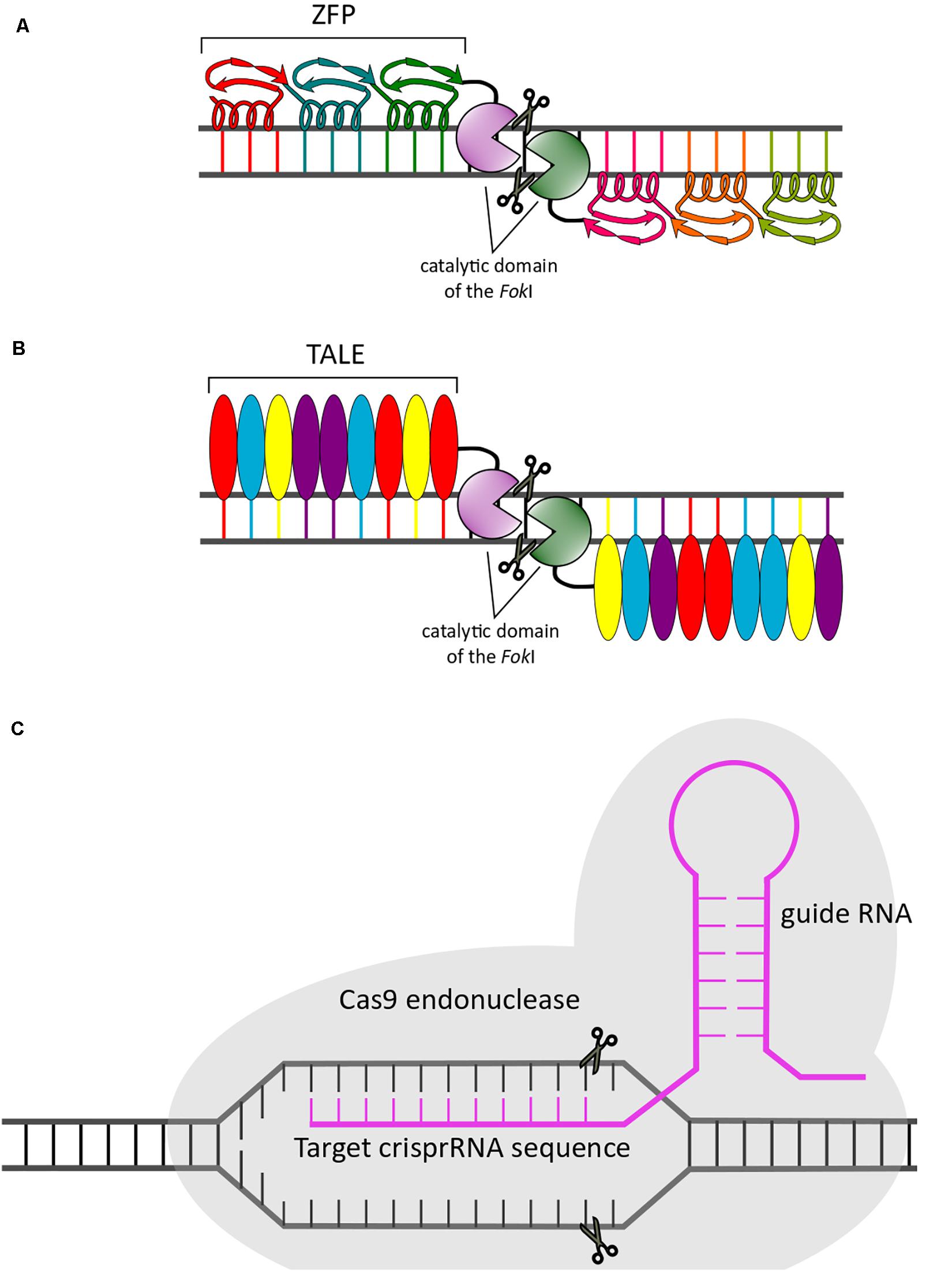
Figure 4. Schematic representation of genome editing approaches. The three gene editing systems described in the main text are illustrated: ZFPs (A), TALENs (B), and CRISPR/Cas9 (C).
Zinc finger nucleases were the first endonucleases designed for genome editing. In one such application, the association of ZF domains with the DNA cleavage domain of the restriction protein FokI leads to breakage of a specific region in the DNA sequence (Porteus and Baltimore, 2003). To this end, two different ZFNs must recognize adjacent sequences separated by a spacer sequence where the break will be located. After this step, DNA repair pathways such as nonhomologous end joining (NHEJ) or homologous recombination (HR) with a codelivered exogenous DNA template lead to the establishment of a modified sequence in which the targeted mutation is corrected (Cristea et al., 2011). Unfortunately, the use of ZFNs has some limitations, including high cost, off-target effects due to low specificity, and inappropriate interaction between domains.
Zinc finger nucleases can be used as therapeutic tools to correct genetic mutations associated with splicing-related diseases. The main advantage of ZFNs is that the correction of mutations is permanent; thus, continuous administration is not needed. For instance, Ousterout et al., applied ZFNs for permanent deletion of exon 51 of the dystrophin gene for the treatment of DMD (Ousterout et al., 2015). Other authors have designed ZFPs targeting CAG repeats to decrease the levels of mutant HTT for therapeutic purposes in HD (Garriga-Canut et al., 2012; Zeitler et al., 2019).
The current and completed clinical trials of ZFN therapies (13 in total1, accessed in February 2020) focus on three major areas: cancer (1 clinical trial), blood disorders (thalassemia, hemophilia B and sickle-cell disease: 3 clinical trials), infectious diseases (human immunodeficiency virus [HIV]: 7 clinical trials) and orphan diseases (mucopolysaccharidosis: 2 clinical trials).
TALENs
These genome editing tools are chimeric nucleases engineered by fusion of the DNA-binding domain of the bacterial protein TALE with the catalytic domain of the restriction endonuclease FokI (Schornack et al., 2006; Figure 4B). Recognition of a specific DNA sequence is performed by the binding domain, which is composed of monomeric tandem repeats of 33–35 conserved amino acids; within this domain is a variable region known as the repeat variable diresidue (RVD) located at residues 12 and 13. The RVD is responsible for binding to a specific nucleotide. Similar to ZFNs, TALENs work as pairs, recognizing sequences separated by 12–25 bp to promote cleavage of the DNA by the FokI endonuclease. The advantage of TALENs compared to ZFNs is decreased cytotoxicity due to reduced off-target effects (Sun and Zhao, 2013).
Fang et al. used TALENs in vivo for the treatment of β654-thalassemia in a mouse model. The TALENs targeted the mutation site, corrected aberrant β-globin RNA splicing, and ameliorated the β-thalassemia phenotype in β654 mice (Fang et al., 2018). TALENs have also been designed for the treatment of HD (Fink et al., 2016) and DMD (Li et al., 2015). The current trials on TALEN therapies (5 in total1, accessed in February 2020) are focused entirely on cancer.
CRISPR/Cas9
The development of CRISPR/Cas9 technology is considered one of the most important breakthroughs of the last decade. This nucleic acid immune system was first discovered in bacteria and archaea more than thirty years ago (Ishino et al., 1987; Hermans et al., 1991; Mojica et al., 1993). In 2012, Jennifer Doudna and Emmanuelle Charpentier suggested that this system could be used for RNA-programmable genome editing (Jinek et al., 2012). After that study, Feng Zhang and George Church performed the first in vitro studies in eukaryotes demonstrating the genome editing capacity of the CRISPR/Cas9 system in mouse and human cells (Cong et al., 2013; Mali et al., 2013). Since these seminal reports, many researchers have contributed to the molecular understanding, technological development and medical applications of this gene editing system (Lander, 2016).
The CRISPR/Cas9 gene editing system requires administration of two main elements into a cell: (i) a small molecule of RNA (guide RNA [gRNA]) with a sequence complementary to that of the target mutation intended for editing and (ii) an endonuclease CRISPR-associated system (Cas) that allows the cleavage of the specific sequence under the guidance of gRNA binding (Figure 4C). Although many Cas9 orthologs have been investigated, the most widely used is Cas9 from Streptococcus pyogenes. In most eukaryotic cells and after cleavage, two repair pathways, NHEJ and homology-directed repair (HDR), are used to correct CRISPR-mediated breaks. The application areas of CRISPR/Cas9 technology go beyond genome editing, and many comprehensive reviews discussing the potential and specific applications of this system in science and medicine have been published (Adli, 2018 and references therein).
Several studies have used CRISPR/Cas9 to correct splicing-related defects. Yuan et al. used a CRISPR-guided cytidine deaminase to successfully correct mutations associated with splicing-related diseases. These researchers used this tool to restore the expression and function of the protein dystrophin in DMD patients (Yuan et al., 2018). Foltz et al. used CRISPR/Cas9 to generate corrected induced pluripotent stem cells (iPSCs) from fibroblasts with a mutation in the PRPF8 splicing factor from patients with RP. These researchers were also able to differentiate each of these clones into retinal pigment epithelial cells with a nearly normal phenotype, highlighting the power and utility of this genome editing tool (Foltz et al., 2018). Dastidar et al. used CRISPR/Cas9 to excise a CTG-repeat expansion of the DMPK gene, abnormal length of which leads to sequestration of muscle blind-like (MBLN) splicing factors, and achieved correction efficiencies of up to 90% in myotonic dystrophy type-1 (DM1) iPSCs. These results support the use of this tool in developing new therapies for the treatment of DM1 (Dastidar et al., 2018). CRISPR/Cas9 has also been used to treat the neurodegenerative disease X-linked dystonia-parkinsonism (XDP) by excising the SINE-VNTR-Alu (SVA) retrotransposition in intron 32 of the TAF1 gene in multiple pluripotent stem cell-derived neuronal lineages. An XDP-specific transcriptional signature with normalized TAF1 expression levels was achieved in these cells (Aneichyk et al., 2018). Kemaladewey et al. demonstrated in vivo systemic delivery of an adeno-associated virus (AAV) carrying CRISPR/Cas9 genome editing components in a mouse model of congenital muscular dystrophy type 1A (MDC1A) to correct a pathogenic SS mutation in LAMA2 pre-mRNA in order to include exon 2. The LAMA2 gene encodes the α2 chain of the most abundant laminin isoform of the basal lamina (Laminin-211), and restoration of full-length LAMA2 expression by CRISPR/Cas9 improves muscle histopathology and function (Kemaladewi et al., 2017). These observations, together with those described in a follow-up report by the same authors, validate the use of this gene editing technology as a therapeutic strategy for MDC1A (Kemaladewi et al., 2019). Thus far, trials using CRISPR therapies (27 in total1, accessed in February 2020) have focused mainly on cancer. Very recently, Stadtmauer et al. reported data from the first phase I clinical trial on cancer immunotherapy combined with CRISPR. The results of the trial demonstrated that it is feasible and safe to apply this technology for cancer immunotherapy (Stadtmauer et al., 2020).
Despite the targeting specificity of Cas9, off-target DNA cleavage activity can occur. In addition, many recent reports have suggested that CRISPR/Cas9 might unintentionally generate alternatively spliced products, large genomic deletions, translocations and inversions; this is a matter of concern that should be further evaluated prior to the clinical application of this technology (Smith et al., 2018).
A modification of the CRISPR/Cas9 system termed the base editing system (BEs) has expanded the arsenal of tools for genome modification. Unlike CRISPR, the BEs can introduce precise base changes without introducing double-strand breaks (DSBs) and, in the case of HDR, without the requirement of a template donor (Hess et al., 2017; Rees and Liu, 2018). Two classes of BEs have been developed: the cytosine BEs (CBEs) (Komor et al., 2016) and the adenine BEs (ABEs) (Gaudelli et al., 2017); these systems include cytidine deaminases and evolved Escherichia coli TadA, respectively. Osborn et al. applied the ABEs to an in vitro model of primary fibroblasts extracted from recessive dystrophic epidermolysis bullosa (RDEB) patients. Compared with HDR using a donor template, the ABEs efficiently corrected two COL7A1 mutations with minimal indels (Osborn et al., 2020). Recently, Song et al. also systemically delivered an ABEs and a single gRNA (sgRNA) to edit a point mutation in the FAH gene in an adult mouse model of HTI. The results showed improvements in liver histology in ABEs-treated mice, and the correction of the point mutation was confirmed by sequencing, indicating restoration from the diseased to the normal phenotype in vivo (Song et al., 2020). The enormous potential of this technique is related to the fact that conversion of A⋅T to G⋅C base pairs in genomic DNA makes it possible to correct almost half of the 32,000 point mutations that cause genetic diseases (Gaudelli et al., 2017).
Some of the strategies presented in this section are feasible treatment options for several diseases. Although the potential of nucleic acids (DNA or RNA) as drugs immediately became obvious decades ago, the actual development of nucleic acid-based medicines has faced major and evident hurdles. For instance, nucleic acids are highly susceptible to degradation by endogenous nucleases. Some of these nucleic acids, such as short oligonucleotides in their native form, have a very short half-life, even before they are filtered out through the kidneys. Large DNA/RNA constructs with highly negative charges cannot cross the vascular endothelium, dense extracellular matrix and cell and nuclear membranes to reach their intracellular DNA, pre-mRNA or mRNA targets. Moreover, off-target effects of many DNA/RNA therapeutic tools can lead to devastating adverse reactions. Finally, some of these tools can be immunogenic. Although chemical modifications can improve pharmacokinetic and pharmacodynamic properties, the ability of these promising therapeutic tools to efficiently deliver DNA/RNA in order to modify sufficient numbers of cells for therapeutic benefits is still the limiting factor for the translation of preclinical models to standard clinical care. This ongoing challenge, which is considered the Achilles heel of gene therapy (Somia and Verma, 2000), is beginning to be overcome through the use of nanotechnologies. These technologies use complexes of nucleic acids or encapsulate the nucleic acids in nonviral vectors, such as liposomes, lipids, and polymeric or inorganic nanoparticles, to enhance safe delivery to the target site. Next, we will focus on the application of nanotechnologies for gene delivery and discuss the advantages and problems associated with nanotechnology-based systems. Addressing the problems will dismantle the barriers facing nucleic acid-based therapeutics.
Nanomedicine
In recent decades, various vectors and tools have been developed for gene therapy. In addition, the advent of new gene editing therapies, such as CRISPR/Cas9, has sparked investigation into appropriate gene delivery systems, including viral vector and transposon-based vector systems. Nanostructures are nanoscale-sized particles capable of transfecting cells and releasing cargoes such as small molecules, DNA, RNA and peptides to exert pharmacological effects. These nonviral vectors have received considerable attention due to their advantages compared to viral systems, which have been the most common choices for gene delivery. Several good reviews have extensively explained the differences between these types of vectors (Chira et al., 2015; Ramamoorth and Narvekar, 2015; Foldvari et al., 2016). The main advantage of nonviral gene delivery systems is their low immunogenicity, as high immunogenicity can impair viral transduction efficacy. Insertional mutagenesis is also a recognized safety concern associated with viral vectors intended for use in gene therapy (Hacein-Bey-Abina et al., 2003; David and Doherty, 2017), and viral integration is recognized as a common outcome of applications that utilize AAVs for genome editing (Hanlon et al., 2019). The major advantages of viral vectors include strong and prolonged transgene expression, broad cell tropism, and thorough understanding of viral gene function. Compared with viral systems, nanoparticle-mediated nucleic acid delivery systems have the advantages of weak immunogenicity, lack of integration and absence of potential for viral recombination, all of which translate to improved safety (Yin et al., 2014). The development processes and manufacturing capacity for clinical-grade nanoparticles are also advantages of nanoparticle-based methods versus viral methods. Nanotechnologies are applicable to a large cohort of patients (Paliwal et al., 2014)., and several nanoparticle-based formulations are already on the market. For example, the siRNA-based drug Onpattro®, used for the treatment of the polyneuropathy hATTR in adults via inhibition of the production of the disease-causing protein, is encased in lipid nanoparticles for delivery into the body. However, the transfection efficiency of nanoparticle-based systems is comparatively poor, and poor transfection efficiency is the main limitation for this and other nonviral methods. For this reason, AAV vectors are the most commonly used vectors for nucleic-acid delivery. A good overview of the current status of the clinical translation of viral and nonviral systems for gene therapy has recently been published (Kaemmerer, 2018).
Several formulations based on nanoparticles have demonstrated sustained expression of transported cargoes and long-term achievement of biological effects (Cohen et al., 2000; Shi et al., 2014). Nanoparticles can also achieve successful tissue-specific delivery of biomolecules through different strategies. For example, incorporation of specific antibodies into the nanoparticle surface has enabled effective targeting of nanoparticles to the brain and lung endothelium (Kolhar et al., 2013) for the treatment of many types of cancer, inflammation dysfunction, and infectious disease (Cardoso et al., 2012). Specific chemical components have also been incorporated into nanoparticles to increase delivery of biomolecules to targeted cells. Incorporation of phosphatidylserine (PS), cholesteryl-9-carboxynonanoeate (9-CCN) (Maiseyeu et al., 2010), or folate into targeted nanoparticles (Krzyszton et al., 2017) has been shown to increase uptake by macrophages to help treat atherosclerosis and rheumatoid arthritis.
Nanoparticles exist in different forms and can be divided into different classes based on their compositions and properties: polymeric nanoparticles, liposomes, lipid nanoparticles, and inorganic nanoparticles (Figure 5).
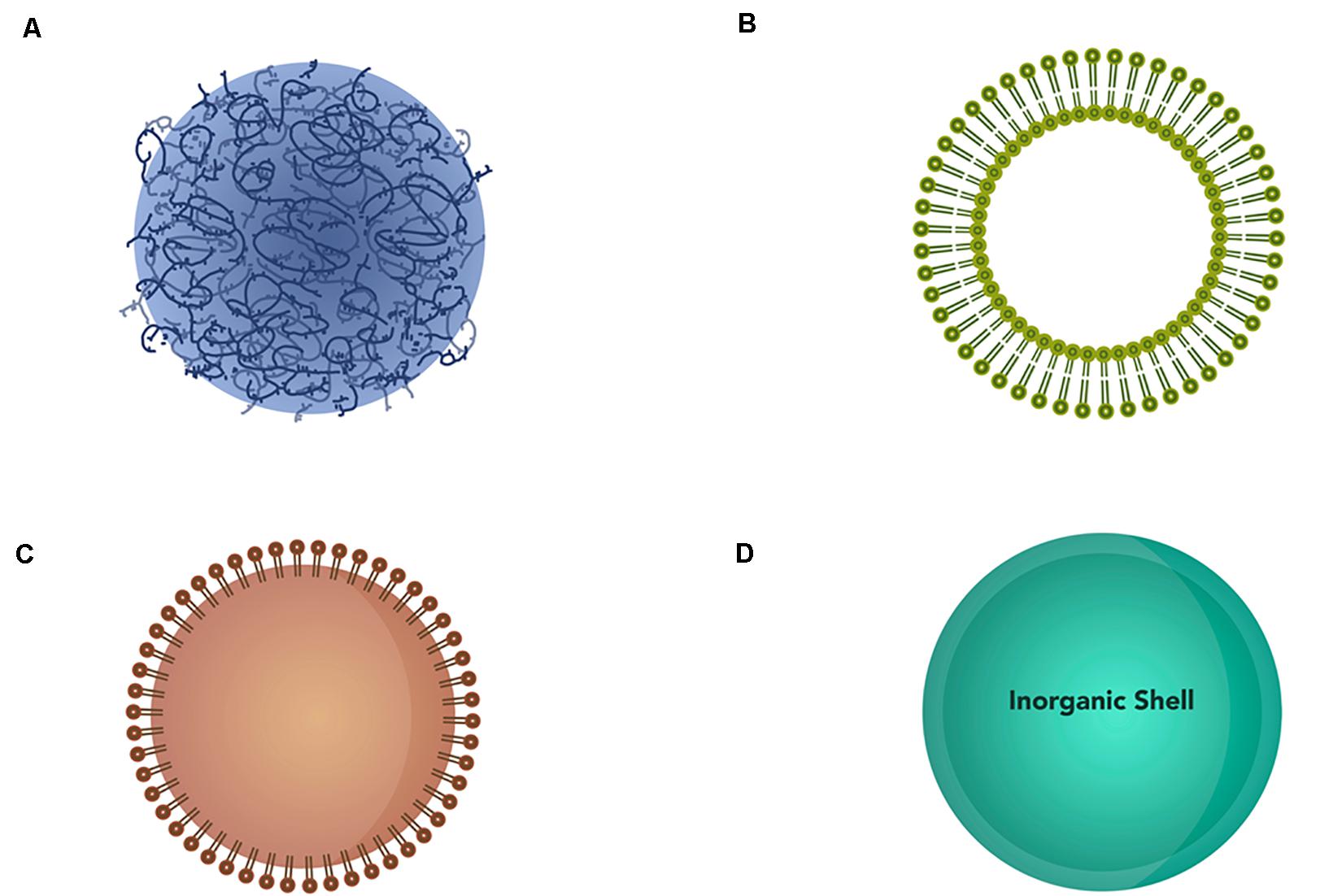
Figure 5. Schematic representation of different types of nanoparticles. (A) Polymeric nanoparticles, (B) liposomes, (C) lipid nanoparticles, and (D) inorganic nanoparticles.
Polymeric Nanoparticles
Polymeric nanoparticles are based on a polymeric matrix. The most frequently used polymers are poly-lactide-coglycolide (PLGA), polyhydroxyalkanoates (PHAs) and CDs (CDs) (Zhang et al., 2018). PLGA is a biodegradable and biocompatible polymer formed by units of lactic acid and glycolic acid. This excipient is approved by the FDA and has been extensively used to develop nanoparticles (Zakharova et al., 2017). The grade of the polymer depends on the ratio between lactic acid and polyglycolic acid (PGA), which can affect the final characteristics of the nanoparticle. For example, polymers with higher glycolide contents have shorter deterioration times due to their more hydrophilic and amorphous characteristics. On the other hand, polymers with higher lactic content are more hydrophobic, thus exhibiting longer deterioration times (Schliecker et al., 2003). Frequently, PLGA is mixed with other polymers to improve the characteristics of the resulting nanoparticles. For example, polyethylenimine (PEI) is commonly incorporated to improve the transfection efficiency of nanostructures (Xie et al., 2016; Wang et al., 2018). PEI is a polycationic polymer capable of condensing DNA and RNA into stable nanostructures, primarily via electrostatic interactions. However, several studies have revealed that this polymer is cytotoxic, as elegantly discussed by Hunter more than a decade ago (Hunter, 2006). Thus, the translation of PEI-based nanoparticles to clinical applications is limited. Another polymer frequently mixed with PLGA is polyethylene glycol (PEG) (Zhang et al., 2014). PEG is a nonionic biocompatible polymer coated onto the surfaces of nanoparticles that prevents recognition and destruction of these carriers by the mononuclear phagocyte system (MPS), thereby increasing the plasma half-lives of the nanoparticles (Mustafa et al., 2017). Furthermore, PEGylation of nanoparticles improves their stability by reducing intermolecular aggregation and the accessibility of the target site (Gref et al., 2000). PLGA-based nanoparticles can also be functionalized with ligands such as antibodies and Fab fragments to improve cellular targeting (Kennedy et al., 2018).
Polyhydroxyalkanoates are polyesters produced by microorganisms through, for example, bacterial fermentation of sugars or lipids. Because they are biodegradable and biocompatible polymers, PHAs have been used as bioimplant materials for medical and therapeutic applications for more than thirty years (reviewed in Zhang et al., 2018). Different types of PHAs can be used for nanoparticle formulation. There are 2 types of PHAs with different chain lengths: short-chain-length PHAs (scl-PHAs), which are composed of 3 to 5 carbon atoms, and medium-chain-length PHAs (mcl-PHAs), which are composed of 6 to 16 carbon atoms. There is also a subtype of PHAs that includes copolymers of scl-mcl PHAs of 4 to 12 carbon atoms (Hazer and Steinbuchel, 2007). PHA-based nanoparticles have been used to deliver biomolecules for anticancer (Lu et al., 2011; Fan et al., 2018; Radu et al., 2019) and antibacterial applications (Castro-Mayorga et al., 2014, 2016; Mukheem et al., 2018). Nanocarriers fabricated from PHA−grafted copolymers have also been developed for efficient siRNA delivery (Zhou et al., 2012); these formulations are safe siRNA carriers for gene therapy. A review discussing the use of PHA-based nanovehicles as therapeutic delivery carriers has been recently published (Lin, 2017).
CDs are cyclic oligosaccharides extensively used in pharmaceutical and biomedical applications. These molecules can be divided into 3 groups: α-CDs (6 subunits of glucose), β-CDs (7 subunits of glucose) and γ-CDs (8 subunits of glucose). CDs are biocompatible products approved by the FDA that are currently present in marketed formulations (Jambhekar and Breen, 2016). The cyclic structure of CDs results in a hydrophobic lumen and a hydrophilic surface. This characteristic allows the use of CDs for multiple purposes, such as the vectorization of lipophilic drugs (Fine-Shamir et al., 2017). Furthermore, CDs can penetrate cells and release their cargoes through, for example, pH-dependent mechanisms (Tardy et al., 2016). CD-based nanoparticles have also been used for gene delivery. For example, Zuckerman et al. developed CD nanoparticles containing siRNAs for the treatment of chronic kidney diseases. Additionally, these researchers reported the functionalization of these nanoparticles with mannose or transferrin for enhanced nanoparticle uptake (Zuckerman et al., 2015).
Liposomes
Liposomes were some of the first nanostructures to be developed for drug delivery. Liposomes were developed in the 1960s and are currently present in marketed formulations such as Doxyl®, Myocet® and Caelix®. At present, no liposome-based marketed formulations for gene delivery exist. Liposomes are nanoscale particles with a lipid bilayer composition that forms a spherical structure inside an aqueous compartment. In aqueous solution, liposomes form colloidal dispersions. The main components of liposomes are phospholipids, such as phosphatidylcholine (PC), phosphatidylethanolamine (PE), PS, phosphatidylinositol (PI) and phosphatidyl glycerol (PG), and cholesterol, which can be incorporated into the phospholipid membrane to increase liposome stability (Bozzuto and Molinari, 2015). Other excipients can be used to improve the properties of liposomes or to endow them with new characteristics suitable for gene delivery. For example, dioleoylphosphatidylethanolamine (DOPE) is used to produce pH-sensitive liposomes, and the cationic lipids N-[1-(2,3-dioleyloxy)propyl]-N,N,N-trimethylammonium chloride (DOTMA) and N-[1-(2,3-dioleoyloxy)propyl]-N,N,N-trimethylammonium chloride (DOTAP) are used to formulate liposomes with cationic charges to facilitate the incorporation of DNA and RNA. In addition, polymers and carbohydrates, such as PEG and monosialoganglioside (GM1), can be incorporated into nanoparticle formulations to improve their in vivo half-lives and stability (Daraee et al., 2016b).
Many published studies have shown the efficacy of liposomes in delivering RNA or DNA into cells. For example, Dorrani et al. developed a liposome formulation with DOTAP and sodium cholate (NaChol) as edge-activators that is capable of efficiently delivering siRNA through skin layers after topical administration, demonstrating that liposomes are good candidates for the treatment of skin diseases such as melanoma (Dorrani et al., 2016). Qiao et al. developed a formulation incorporating mannosylated zwitterionic-based cationic liposomes (man-ZCLs) that increases the endosomal/lysosomal escape of nanostructures to enable administration of a DNA vaccine for HIV, providing a new, safe and effective HIV vaccination method that can be tested in future studies (Qiao et al., 2016). Recently, a four-component liposome formulation with DOTAP, DOPE, PEG, and cholesterol was used to transfect Cas9/sgRNA with high efficacy in order to knock out targeted genes in vivo (Hosseini et al., 2019).
Lipid Nanoparticles
Lipid nanocarriers are some of the most promising nonviral tools for gene therapy. Currently, the only medicine approved by the FDA and European Medicines Agency (EMA) that uses nanostructures to deliver RNA is Onpattro® (mentioned in section “Small Interfering RNAs (siRNAs)”); Onpattro® is a lipid nanoparticle-based drug product that transports patisiran, an siRNA molecule for the treatment of TTR amyloidosis (Rizk and Tuzmen, 2019).
Lipid nanoparticles were developed to address important drawbacks of other lipid-based systems, such as instability and the necessity for use of surfactants and other toxic substances; to increase loading capacity; and to resolve other problems related to manufacturing and scale-up processes (Muller et al., 2000). Lipid nanoparticles can be divided into 2 categories: solid lipid nanoparticles (SLNs) and nanostructured lipid carriers (NLCs) (Gordillo-Galeano and Mora-Huertas, 2018). Both types of nanoparticles use lipid excipients that are biocompatible and biodegradable. These structures are highly attractive for clinical applications due to their simple and inexpensive manufacturing processes that do not require organic solvents and can be easily scaled up; their high stability; and their ability to be administered through different routes, such as the parenteral, pulmonary, oral and topical routes (Uner and Yener, 2007).
Solid lipid nanoparticles have been developed for gene delivery since 2000. The structure of SLNs can help to protect the drug or RNA/DNA against external agents and can enable modified and/or targeted release (Muller et al., 2000). SLNs are manufactured with solid lipid excipients. The most common excipients include stearic acid, cholesterol derivatives (e.g., cholesteryl oleate), glyceryl monostearate, glyceryl behenate, cetylpalmitate, glycolipids, tripalmitine and tristearin. Other essential excipients that are incorporated into these formulations are surfactants and cosurfactants, such as Pluronic® compounds (i.e., Pluronic F68), Poloxamer® compounds (i.e., Poloxamer 188), Brij®, Tween 80, and Span 20. Cationic molecules can also be incorporated in formulations to provide a positive surface charge (forming cationic SLNs [cSLNs]) in order to facilitate the formation of SLNplexes with DNA/RNA. The most commonly used cationic excipients are octadecylamine, benzalkonium chloride, cetrimide (DTAB), DOTAP, N,N-di-(β-stearoylethyl)-N,N-dimethyl-ammonium chloride (Esterquat 1 [EQ1]) and stearylamine (de Jesus and Zuhorn, 2015). To improve the efficacy of this type of vector, other excipients, such as protamine (Limeres et al., 2019), can be incorporated into the formulation. There are many examples of the use of SLNs for gene therapy. For example, Apaolaza et al. developed a formulation incorporating hyaluronic acid for transfection of cells with a plasmid containing the RS1 gene. Intravitreal administration of this formulation induced the expression of the protein retinoschisin in photoreceptors of Rs1h-deficient mice, leading to structural improvements in degenerated retinas (Apaolaza et al., 2016). Rassu et al. designed SLNs capable of carrying BACE1 siRNA to the brain after nasal administration for the treatment of Alzheimer’s disease. These researchers formulated nanoparticles with RVG-9R, a type of cell-penetrating peptide (CPP) that facilitated the transcellular pathway in neuronal cells. Furthermore, the researchers coated the nanoparticles with chitosan, which provided extra protection to the siRNA and increased the mucoadhesiveness of the particles, thereby increasing the residence time in the nasal cavity (Rassu et al., 2017).
Nanostructured lipid carriers were first developed several years after SLNs. In contrast to SLNs, which involve solid lipids, NLCs involve liquid lipids; the use of liquid lipids increases the stability and drug loading capacity of the nanoparticles (Uner and Yener, 2007). The liquid lipids most commonly used to formulate NLCs are oleic acid and caprylic/capric triglycerides. Other lipids used are canola stearin and myristyl myristate. The other excipients are the same as those used for SLNs. A study by Taratula et al. has demonstrated the high loading capacity and gene delivery potential of NLCs. The authors developed a multifunctional NLC-based system containing a drug (paclitaxel or doxorubicin), two different types of siRNA, and a modified synthetic analog of luteinizing hormone-releasing hormone (LHRH) to increase specificity for local targeted delivery to lung tumors (Taratula et al., 2013). Similarly, Chen et al. have shown the capability of NLCs to coencapsulate plasmids and temozolomide, an anticancer drug. Those authors tested the system in vitro and in vivo for efficient delivery to malignant glioblastoma cells for the treatment of malignant gliomatosis cerebri (Chen et al., 2016).
Inorganic Nanoparticles
Inorganic nanoparticles include nanostructures that are manufactured using inorganic materials, such as gold, silicon and iron oxide; carbon materials; layered double hydroxide (LDH); or calcium phosphate (Xu et al., 2006). The easy surface functionalization, good target delivery and controlled release of these nanoparticles are their main advantages. Some of the most widely used inorganic nanostructures are gold nanoparticles (AuNPs). This type of nanoparticle is used in the biomedical field for different applications, such as biodetection, biodiagnostics, and bioelectronic or therapeutic agent development. Among the advantages of these nanoparticles are their size- and structure-dependent visual and electronic characteristics and high surface/volume ratios and the capability to functionalize their surfaces due to their high affinities for different functional groups (Giljohann et al., 2010; Daraee et al., 2016a). One example of an AuNP-based gene delivery system was recently developed by Jia et al. (2017). The authors surface-conjugated AuNPs with thiol-modified antago-miR155, an RNA antagonist to a potent promoter of proinflammatory type 1 macrophage polarization (miR155) that plays an important role in diabetic cardiomyopathy. In vivo administration of the AuNP complex resulted in the incorporation of nucleic acids into macrophages via phagocytosis and led to reduced inflammation, reduced apoptosis and restoration of cardiac function. AuNPs have also been used to deliver Cas9 ribonucleoprotein and donor DNA in vitro and in vivo and to correct the DNA mutation in the dystrophin gene that causes DMD (Lee et al., 2017).
Oxide nanoparticles can be classified into two important groups: silicon oxide nanoparticles and iron nanoparticles (Xu et al., 2006). Mesoporous silica nanoparticles (MSNs) have been extensively investigated with regard to gene delivery. Indeed, RNA can be loaded onto the surfaces of small pore-sized MSNs, thereby enabling RNA delivery into cells. Furthermore, RNA and drugs can be loaded onto the same large pore-sized MSNs; thus, large pore-sized MSNs have the capacity to deliver two therapeutic agents at the same time. Sun et al. developed core-shell hierarchical MSNs (H-MSNs); doxorubicin can be loaded into the core mesopores, and siRNAs that downregulate the expression of P-gp to reverse multidrug resistance (MDR) can be loaded into the shell mesopores. The specific release of siRNA into the microtumor environment enables inhibition of MDR, and the subsequent release of doxorubicin enhances this effect (Sun et al., 2017).
Iron nanoparticles can be made of different materials, such as magnetite (Fe3O4) and maghemite (Fe2O3). The intrinsic magnetic properties of these nanoparticles can be used for gene or drug delivery. For example, an external magnetic field can be used to guide the nanoparticles to a specific zone of the body for drug release. Furthermore, a magnetic field can be applied to a cell culture dish to enhance cell transfection by magnetic nanoparticles in a procedure known as magnetofection (Estelrich et al., 2015). Despite the difficulties encountered in translating the magnetofection technique to clinical applications, advanced studies have demonstrated that iron nanoparticles can be applied for ex vivo delivery of chemically modified RNA (cmRNA), opening the door to continuing studies on gene therapy applications (Badieyan et al., 2017).
Concluding Remarks
In 1993, the Nobel Prize in Physiology and Medicine was awarded to Phillip Sharp and Richard Roberts for their discovery of adenoviral RNA splicing (Berget et al., 1977; Chow et al., 1977). This discovery had notable consequences for elucidation of gene expression regulation and the evolution of eukaryotic cells. More than forty years after this seminal discovery, we have a deep understanding of the molecular mechanisms that control this important regulatory process, and we have recently begun to unravel the molecular links that connect faulty splicing with many human disorders. This knowledge has enabled the design of innovative therapeutic strategies intended to correct splicing defects. Many tools based on nucleic acid gene repair have been tested with positive results, and many more tools warrant further development. The field is moving notably quickly, but we have attempted to provide a general overview of the main developments. However, as important as it is to decipher the mechanisms that govern the connections between missplicing and pathologies and to apply these findings in the clinic, research on the safe transport of therapeutic biomolecules into cells and to their targets is equally important. Further development, characterization and testing of engineered technologies for targeted delivery and controlled release of DNA and RNA directly into cells with clinical applications are needed, as the demand for innovative nucleic acid delivery systems continues to grow. Nanoparticles possess considerable potential for use in the controlled delivery of therapeutic agents to specific target sites for splicing-based treatments. Conducting related research is a challenging task, as basic scientists must interact and collaborate with nanotechnology experts. Funding opportunities should emphasize such collaboration as a way forward for grant support.
Author Contributions
All authors contributed to the writing and revision of the manuscript and approved the submitted version.
Funding
This work was supported by grants from the Spanish Ministry of Economy and Competitiveness (grant number BFU2017-89179-R to CS and grant number BFU2016-79699-P to CH-M). Support from the European Region Development Fund (ERDF [FEDER]) is also acknowledged.
Conflict of Interest
The authors declare that the research was conducted in the absence of any commercial or financial relationships that could be construed as a potential conflict of interest.
Supplementary Material
The Supplementary Material for this article can be found online at: https://www.frontiersin.org/articles/10.3389/fgene.2020.00731/full#supplementary-material
Footnotes
References
Aird, D., Teng, T., Huang, C. L., Pazolli, E., Banka, D., Cheung-Ong, K., et al. (2019). Sensitivity to splicing modulation of BCL2 family genes defines cancer therapeutic strategies for splicing modulators. Nat. Commun. 10:137.
Alves, C. A., Benito-Vicente, A., Medeiros, A. M., Reeves, K., Martin, C., and Bourbon, M. (2018). Further evidence of novel APOB mutations as a cause of familial hypercholesterolaemia. Atherosclerosis. 277, 448–456. doi: 10.1016/j.atherosclerosis.2018.06.819
Aneichyk, T., Hendriks, W. T., Yadav, R., Shin, D., Gao, D., Vaine, C. A., et al. (2018). Dissecting the causal mechanism of X-linked dystonia-parkinsonism by integrating genome and transcriptome assembly. Cell. 172, 897.e821–909.e281.
Anna, A., and Monika, G. (2018). Splicing mutations in human genetic disorders: examples, detection, and confirmation. J. Appl. Genet. 59, 253–268. doi: 10.1007/s13353-018-0444-7
Apaolaza, P. S., Del Pozo-Rodriguez, A., Solinis, M. A., Rodriguez, J. M., Friedrich, U., Torrecilla, J., et al. (2016). Structural recovery of the retina in a retinoschisin-deficient mouse after gene replacement therapy by solid lipid nanoparticles. Biomaterials 90, 40–49. doi: 10.1016/j.biomaterials.2016.03.004
Arnold, E. S., Ling, S. C., Huelga, S. C., Lagier-Tourenne, C., Polymenidou, M., Ditsworth, D., et al. (2013). ALS-linked TDP-43 mutations produce aberrant RNA splicing and adult-onset motor neuron disease without aggregation or loss of nuclear TDP-43. Proc. Natl. Acad. Sci. U.S.A. 110, E736–E745.
Axelrod, F. B., Liebes, L., Gold-Von Simson, G., Mendoza, S., Mull, J., Leyne, M., et al. (2011). Kinetin improves IKBKAP mRNA splicing in patients with familial dysautonomia. Pediatr. Res. 70, 480–483. doi: 10.1203/pdr.0b013e31822e1825
Aznarez, I., Chan, E. M., Zielenski, J., Blencowe, B. J., and Tsui, L. C. (2003). Characterization of disease-associated mutations affecting an exonic splicing enhancer and two cryptic splice sites in exon 13 of the cystic fibrosis transmembrane conductance regulator gene. Hum. Mol. Genet. 12, 2031–2040. doi: 10.1093/hmg/ddg215
Badieyan, Z. S., Pasewald, T., Mykhaylyk, O., Rudolph, C., and Plank, C. (2017). Efficient ex vivo delivery of chemically modified messenger RNA using lipofection and magnetofection. Biochem. Biophys. Res. Commun. 482, 796–801. doi: 10.1016/j.bbrc.2016.11.113
Bailey, P., Chang, D. K., Nones, K., Johns, A. L., Patch, A. M., Gingras, M. C., et al. (2016). Genomic analyses identify molecular subtypes of pancreatic cancer. Nature. 531, 47–52.
Baker, M. S., Ahn, S. B., Mohamedali, A., Islam, M. T., Cantor, D., Verhaert, P. D., et al. (2017). Accelerating the search for the missing proteins in the human proteome. Nat. Commun. 8:14271.
Baker, N. L., Mörgelin, M., Peat, R., Goemans, N., North, K. N., Bateman, J. F., et al. (2005). Dominant collagen VI mutations are a common cause of Ullrich congenital muscular dystrophy. Hum. Mol. Genet. 14, 279–293. doi: 10.1093/hmg/ddi025
Balestra, D., Giorgio, D., Bizzotto, M., Fazzari, M., Ben Zeev, B., Pinotti, M., et al. (2019a). Splicing mutations impairing CDKL5 expression and activity can be efficiently rescued by U1snRNA-based therapy. Int. J. Mol. Sci. 20:4130. doi: 10.3390/ijms20174130
Balestra, D., Maestri, I., Branchini, A., Ferrarese, M., Bernardi, F., and Pinotti, M. (2019b). An altered splicing registry explains the differential ExSpeU1-mediated rescue of splicing mutations causing haemophilia A. Front. Genet. 10:974. doi: 10.3389/fgene.2019.00974
Barbaux, S., Niaudet, P., Gubler, M. C., Grünfeld, J. P., Jaubert, F., Kuttenn, F., et al. (1997). Donor splice-site mutations in WT1 are responsible for Frasier syndrome. Nat. Genet. 17, 467–470. doi: 10.1038/ng1297-467
Berger, A., Lorain, S., Josephine, C., Desrosiers, M., Peccate, C., Voit, T., et al. (2015). Repair of rhodopsin mRNA by spliceosome-mediated RNA trans-splicing: a new approach for autosomal dominant retinitis pigmentosa. Mol. Ther. 23, 918–930. doi: 10.1038/mt.2015.11
Berger, A., Maire, S., Gaillard, M. C., Sahel, J. A., Hantraye, P., and Bemelmans, A. P. (2016). mRNA trans-splicing in gene therapy for genetic diseases. Wiley Interdiscipl. Rev. RNA 7, 487–498. doi: 10.1002/wrna.1347
Berget, S. M., Moore, C., and Sharp, P. A. (1977). Spliced segments at the 5’ terminus of adenovirus 2 late mRNA. Proc. Natl. Acad. Sci. U.S.A. 74, 3171–3175. doi: 10.1073/pnas.74.8.3171
Bolduc, V., Zou, Y., Ko, D., and Bonnemann, C. G. (2014). siRNA-mediated allele-specific silencing of a COL6A3 mutation in a cellular model of dominant ullrich muscular dystrophy. Mol. Ther. Nucleic Acids 3:e147. doi: 10.1038/mtna.2013.74
Bourbon, M., Duarte, M. A., Alves, A. C., Medeiros, A. M., Marques, L., and Soutar, A. K. (2009). Genetic diagnosis of familial hypercholesterolaemia: the importance of functional analysis of potential splice-site mutations. J. Med. Genet. 46, 352–357. doi: 10.1136/jmg.2007.057000
Bowen, D. J. (2002). Haemophilia A and haemophilia B: molecular insights. Mol. Pathol. 55, 127–144. doi: 10.1136/mp.55.2.127
Bozzuto, G., and Molinari, A. (2015). Liposomes as nanomedical devices. Int. J. Nanomed. 10, 975–999.
Brooks, A. N., Choi, P. S., de Waal, L., Sharifnia, T., Imielinski, M., Saksena, G., et al. (2014). A pan-cancer analysis of transcriptome changes associated with somatic mutations in U2AF1 reveals commonly altered splicing events. PLoS ONE 9:e87361. doi: 10.1371/journal.pone.0087361
Brosseau, J. P., Lucier, J. F., Lamarche, A. A., Shkreta, L., Gendron, D., Lapointe, E., et al. (2014). Redirecting splicing with bifunctional oligonucleotides. Nucleic Acids Res. 42, e40. doi: 10.1093/nar/gkt1287
Brun, C., Suter, D., Pauli, C., Dunant, P., Lochmuller, H., Burgunder, J. M., et al. (2003). U7 snRNAs induce correction of mutated dystrophin pre-mRNA by exon skipping. Cell Mol. Life Sci. 60, 557–566. doi: 10.1007/s000180300047
Cacace, R., Sleegers, K., and Van Broeckhoven, C. (2016). Molecular genetics of early-onset Alzheimer disease revisited. Alzheimers Dement. 12, 733–748. doi: 10.1016/j.jalz.2016.01.012
Camacho Vanegas, O., Bertini, E., Zhang, R. Z., Petrini, S., Minosse, C., Sabatelli, P., et al. (2001). Ullrich scleroatonic muscular dystrophy is caused by recessive mutations in collagen type VI. Proc. Natl. Acad. Sci. U.S.A. 98, 7516–7521. doi: 10.1073/pnas.121027598
Campbell, M. A., and Wengel, J. (2011). Locked vs. unlocked nucleic acids (LNA vs. UNA): contrasting structures work towards common therapeutic goals. Chem. Soc. Rev. 40, 5680–5689.
Carabet, L. A., Leblanc, E., Lallous, N., Morin, H., Ghaidi, F., Lee, J., et al. (2019). Computer-aided discovery of small molecules targeting the RNA splicing activity of hnRNP A1 in castration-resistant prostate cancer. Molecules 24, 763. doi: 10.3390/molecules24040763
Cardoso, M. M., Peca, I. N., and Roque, A. C. (2012). Antibody-conjugated nanoparticles for therapeutic applications. Curr. Med. Chem 19, 3103–3127. doi: 10.2174/092986712800784667
Castro-Mayorga, J. L., Fabra, M. J., Cabedo, L., and Lagaron, J. M. (2016). On the Use of the electrospinning coating technique to produce antimicrobial polyhydroxyalkanoate materials containing in situ-stabilized silver nanoparticles. Nanomaterials (Basel) 7:4. doi: 10.3390/nano7010004
Castro-Mayorga, J. L., Martinez-Abad, A., Fabra, M. J., Olivera, C., Reis, M., and Lagaron, J. M. (2014). Stabilization of antimicrobial silver nanoparticles by a polyhydroxyalkanoate obtained from mixed bacterial culture. Int. J. Biol. Macromol. 71, 103–110. doi: 10.1016/j.ijbiomac.2014.06.059
Chabot, B., and Shkreta, L. (2016). Defective control of pre-messenger RNA splicing in human disease. J. Cell. Biol. 212, 13–27. doi: 10.1083/jcb.201510032
Chao, H., Mansfield, S. G., Bartel, R. C., Hiriyanna, S., Mitchell, L. G., Garcia-Blanco, M. A., et al. (2003). Phenotype correction of hemophilia A mice by spliceosome-mediated RNA trans-splicing. Nat. Med. 9, 1015–1019. doi: 10.1038/nm900
Chen, G., Ning, B., and Shi, T. (2019). Single-cell RNA-Seq technologies and related computational data analysis. Front. Genet. 10:317. doi: 10.3389/fgene.2019.00317
Chen, Z., Lai, X., Song, S., Zhu, X., and Zhu, J. (2016). Nanostructured lipid carriers based temozolomide and gene co-encapsulated nanomedicine for gliomatosis cerebri combination therapy. Drug Deliv. 23, 1369–1373.
Chira, S., Jackson, C. S., Oprea, I., Ozturk, F., Pepper, M. S., Diaconu, I., et al. (2015). Progresses towards safe and efficient gene therapy vectors. Oncotarget. 6, 30675–30703. doi: 10.18632/oncotarget.5169
Chow, L. T., Gelinas, R. E., Broker, T. R., and Roberts, R. J. (1977). An amazing sequence arrangement at the 5’ ends of adenovirus 2 messenger RNA. Cell. Sep 12, 1–8. doi: 10.1016/0092-8674(77)90180-5
Cieply, B., and Carstens, R. P. (2015). Functional roles of alternative splicing factors in human disease. Wiley Interdiscipl. Rev. RNA 311–326. doi: 10.1002/wrna.1276
Cladaras, C., Hadzopoulou-Cladaras, M., Felberg, B. K., Pavlakis, G., and Zannis, V. I. (1987). The molecular basis of a familial ApoE deficiency: an acceptor splice site mutation in the third intron of the deficient apoE gene. J. Biol. Chem. 262, 2310–2315.
Coady, T. H., and Lorson, C. L. (2010). Trans-splicing-mediated improvement in a severe mouse model of spinal muscular atrophy. J. Neurosci. 30, 126–130. doi: 10.1523/jneurosci.4489-09.2010
Cohen, H., Levy, R. J., Gao, J., Fishbein, I., Kousaev, V., Sosnowski, S., et al. (2000). Sustained delivery and expression of DNA encapsulated in polymeric nanoparticles. Gene Ther. 7, 1896–1905. doi: 10.1038/sj.gt.3301318
Cong, L., Ran, F. A., Cox, D., Lin, S., Barretto, R., Habib, N., et al. (2013). Multiplex genome engineering using CRISPR/Cas systems. Science 339, 819–823.
Coutinho, M. F., Lacerda, L., Prata, M. J., Ribeiro, H., Lopes, L., Ferreira, C., et al. (2008). Molecular characterization of portuguese patients with mucopolysaccharidosis IIIC: two novel mutations in the HGSNAT gene. Clin. Genet. 74, 194–195. doi: 10.1111/j.1399-0004.2008.01040.x
Cristea, S., Gregory, P. D., Urnov, F. D., and Cost, G. J. (2011). Dissection of splicing regulation at an endogenous locus by zinc-finger nuclease-mediated gene editing. PLoS ONE 6:e16961. doi: 10.1371/journal.pone.0016961
Cuyvers, E., and Sleegers, K. (2016). Genetic variations underlying Alzheimer’s disease: evidence from genome-wide association studies and beyond. Lancet Neurol. 15, 857–868. doi: 10.1016/s1474-4422(16)00127-7
Daguenet, E., Dujardin, G., and Valcarcel, J. (2015). The pathogenicity of splicing defects: mechanistic insights into pre-mRNA processing inform novel therapeutic approaches. EMBO Rep. 16, 1640–1655. doi: 10.15252/embr.201541116
Daiger, S. P., Bowne, S. J., and Sullivan, L. S. (2014). Genes and mutations causing autosomal dominant retinitis pigmentosa. Cold Spring Harb. Perpect Med. 5:a017129. doi: 10.1101/cshperspect.a017129
Dal Mas, A., Rogalska, M. E., Bussani, E., and Pagani, F. (2015). Improvement of SMN2 pre-mRNA processing mediated by exon-specific U1 small nuclear RNA. Am. J. Hum. Genet. 96, 93–103. doi: 10.1016/j.ajhg.2014.12.009
Dana, H., Chalbatani, G. M., Mahmoodzadeh, H., Karimloo, R., Rezaiean, O., Moradzadeh, A., et al. (2017). Molecular mechanisms and biological functions of siRNA. Int. J. Biomed. Sci. 13, 48–57.
Daraee, H., Eatemadi, A., Abbasi, E., Fekri Aval, S., Kouhi, M., and Akbarzadeh, A. (2016a). Application of gold nanoparticles in biomedical and drug delivery. Artif. Cells Nanomed. Biotechnol. 44, 410–422.
Daraee, H., Eatemadi, A., Kouhi, M., Alimirzalu, S., and Akbarzadeh, A. (2016b). Application of liposomes in medicine and drug delivery. Artif Cells Nanomed. Biotechnol. 44, 381–391.
Dastidar, S., Ardui, S., Singh, K., Majumdar, D., Nair, N., Fu, Y., et al. (2018). Efficient CRISPR/Cas9-mediated editing of trinucleotide repeat expansion in myotonic dystrophy patient-derived iPS and myogenic cells. Nucleic Acids Res. 46, 8275–8298. doi: 10.1093/nar/gky548
David, R. M., and Doherty, A. T. (2017). Viral vectors: the road to reducing genotoxicity. Toxicol Sci. 155, 315–325. doi: 10.1093/toxsci/kfw220
de Jesus, M. B., and Zuhorn, I. S. (2015). Solid lipid nanoparticles as nucleic acid delivery system: properties and molecular mechanisms. J. Control. Release 201, 1–13. doi: 10.1016/j.jconrel.2015.01.010
de la Mata, M., Alonso, C. R., Kadener, S., Fededa, J. P., Blaustein, M., Pelisch, F., et al. (2003). A slow RNA polymerase II affects alternative splicing in vivo. Mol Cell. 12, 525–532. doi: 10.1016/j.molcel.2003.08.001
den Hollander, A. I., Koenekoop, R. K., Yzer, S., Lopez, I., Arends, M. L., Voesenek, K. E. J., et al. (2006). Mutations in the CEP290 (NPHP6) gene are a frequent cause of leber congenital amaurosis. Am. J. Hum. Genet. 79, 556–561. doi: 10.1086/507318
Dessein, A. F., Fontaine, M., Andresen, B. S., Gregersen, N., Brivet, M., Rabier, D., et al. (2010). A novel mutation of the ACADM gene (c.145C> G) associated with the common c.985A > G mutation on the other ACADM allele causes mild MCAD deficiency: a case report. Orphanet J. Rare Dis. 5, 26. doi: 10.1186/1750-1172-5-26
Dewaele, M., Tabaglio, T., Willekens, K., Bezzi, M., Teo, S. X., Low, D. H., et al. (2016). Antisense oligonucleotide-mediated MDM4 exon 6 skipping impairs tumor growth. J. Clin. Investig. 126, 68–84.
Dickson, A., Osman, E., and Lorson, C. L. (2008). A negatively acting bifunctional RNA increases survival motor neuron both in vitro and in vivo. Hum. Gene Ther. 19, 1307–1315. doi: 10.1089/hum.2008.067
Disset, A., Bourgeois, C. F., Benmalek, N., Claustres, M., Stevenin, J., and Tuffery-Giraud, S. (2006). An exon skipping-associated nonsense mutation in the dystrophin gene uncovers a complex interplay between multiple antagonistic splicing elements. Hum. Mol. Genet. 15, 999–1013. doi: 10.1093/hmg/ddl015
Dominski, Z., and Kole, R. (1993). Restoration of correct splicing in thalassemic pre-mRNA by antisense oligonucleotides. Proc. Natl. Acad. Sci. U.S.A. 90, 8673–8677. doi: 10.1073/pnas.90.18.8673
Donadon, I., McVey, J. H., Garagiola, I., Branchini, A., Mortarino, M., Peyvandi, F., et al. (2018a). Clustered F8 missense mutations cause hemophilia A by combined alteration of splicing and protein biosynthesis and activity. Haematologica 103, 344–350. doi: 10.3324/haematol.2017.178327
Donadon, I., Pinotti, M., Rajkowska, K., Pianigiani, G., Barbon, E., Morini, E., et al. (2018b). Exon-specific U1 snRNAs improve ELP1 exon 20 definition and rescue ELP1 protein expression in a familial dysautonomia mouse model. Hum. Mol. Genet. 27, 2466–2476. doi: 10.1093/hmg/ddy151
Dorrani, M., Garbuzenko, O. B., Minko, T., and Michniak-Kohn, B. (2016). Development of edge-activated liposomes for siRNA delivery to human basal epidermis for melanoma therapy. J. Control. Release 228, 150–158. doi: 10.1016/j.jconrel.2016.03.010
Dujardin, G., Lafaille, C., de la Mata, M., Marasco, L. E., Munoz, M. J., Le Jossic-Corcos, C., et al. (2014). How slow RNA polymerase II elongation favors alternative exon skipping. Mol. Cell 54, 683–690. doi: 10.1016/j.molcel.2014.03.044
Eckstein, F. (2014). Phosphorothioates, essential components of therapeutic oligonucleotides. Nucleic Therap. 24, 374–387. doi: 10.1089/nat.2014.0506
Elbashir, S. M., Harborth, J., Lendeckel, W., Yalcin, A., Weber, K., and Tuschl, T. (2001). Duplexes of 21-nucleotide RNAs mediate RNA interference in cultured mammalian cells. Nature 411, 494–498. doi: 10.1038/35078107
Estelrich, J., Escribano, E., Queralt, J., and Busquets, M. A. (2015). Iron oxide nanoparticles for magnetically-guided and magnetically-responsive drug delivery. Int. J. Mol. Sci. 16, 8070–8101. doi: 10.3390/ijms16048070
Fadista, J., Vikman, P., Laakso, E. O., Mollet, I. G., Esguerra, J. L., Taneera, J., et al. (2014). Global genomic and transcriptomic analysis of human pancreatic islets reveals novel genes influencing glucose metabolism. Proc. Natl. Acad. Sci. U.S.A. 111, 13924–13929. doi: 10.1073/pnas.1402665111
Fan, F., Wang, L., Ouyang, Z., Wen, Y., and Lu, X. (2018). Development and optimization of a tumor targeting system based on microbial synthesized PHA biopolymers and PhaP mediated functional modification. Appl. Microbiol. Biotechnol. 102, 3229–3241. doi: 10.1007/s00253-018-8790-2
Fang, Y., Cheng, Y., Lu, D., Gong, X., Yang, G., Gong, Z., et al. (2018). Treatment of beta(654) -thalassaemia by TALENs in a mouse model. Cell Prolif. 51, e12491. doi: 10.1111/cpr.12491
Fernandez, A., Josa, S., and Montoliu, L. (2017). A history of genome editing in mammals. Mamm Genome 28, 237–246. doi: 10.1007/s00335-017-9699-2
Fernandez Alanis, E., Pinotti, M., Dal Mas, A., Balestra, D., Cavallari, N., Rogalska, M. E., et al. (2012). An exon-specific U1 small nuclear RNA (snRNA) strategy to correct splicing defects. Hum. Mol. Genet. 21, 2389–2398. doi: 10.1093/hmg/dds045
Ferrari, S., Di Iorio, E., Barbaro, V., Ponzin, D., Sorrentino, F. S., and Parmeggiani, F. (2011). Retinitis pigmentosa: genes and disease mechanisms. Curr. Genomics 12, 238–249. doi: 10.2174/138920211795860107
Fine, J. D., and Hintner, H. (2009). Life with Epidermolysis Bullosa (EB): Etiology, Diagnosis, Multidisciplinary Care and Therapy. Berlin: Springer.
Fine-Shamir, N., Beig, A., Zur, M., Lindley, D., Miller, J. M., and Dahan, A. (2017). Toward successful cyclodextrin based solubility-enabling formulations for oral delivery of lipophilic drugs: solubility-permeability trade-off, biorelevant dissolution, and the unstirred water layer. Mol. Pharm. 14, 2138–2146. doi: 10.1021/acs.molpharmaceut.7b00275
Fink, K. D., Deng, P., Gutierrez, J., Anderson, J. S., Torrest, A., Komarla, A., et al. (2016). Allele-specific reduction of the mutant huntingtin allele using transcription activator-like effectors in human huntington’s disease fibroblasts. Cell Transplant. 25, 677–686. doi: 10.3727/096368916x690863
Fire, A., Xu, S., Montgomery, M. K., Kostas, S. A., Driver, S. E., and Mello, C. C. (1998). Potent and specific genetic interference by double-stranded RNA in Caenorhabditis elegans. Nature 391, 806–811. doi: 10.1038/35888
Foldvari, M., Chen, D. W., Nafissi, N., Calderon, D., Narsineni, L., and Rafiee, A. (2016). Non-viral gene therapy: gains and challenges of non-invasive administration methods. J. Control. Release 240, 165–190. doi: 10.1016/j.jconrel.2015.12.012
Foltz, L. P., Howden, S. E., Thomson, J. A., and Clegg, D. O. (2018). Functional assessment of patient-derived retinal pigment epithelial cells edited by CRISPR/Cas9. Int. J. Mol. Sci. 19:4127. doi: 10.3390/ijms19124127
Fong, N., Kim, H., Zhou, Y., Ji, X., Qiu, J., Saldi, T., et al. (2014). Pre-mRNA splicing is facilitated by an optimal RNA polymerase II elongation rate. Genes Dev. 28, 2663–2676. doi: 10.1101/gad.252106.114
Furney, S. J., Pedersen, M., Gentien, D., Dumont, A. G., Rapinat, A., Desjardins, L., et al. (2013). SF3B1 mutations are associated with alternative splicing in uveal melanoma. Cancer Discov. 3, 1122–1129. doi: 10.1158/2159-8290.cd-13-0330
Garriga-Canut, M., Agustin-Pavon, C., Herrmann, F., Sanchez, A., Dierssen, M., Fillat, C., et al. (2012). Synthetic zinc finger repressors reduce mutant huntingtin expression in the brain of R6/2 mice. Proc. Natl. Acad. Sci. U.S.A. 109, E3136–E3145.
Gaudelli, N. M., Komor, A. C., Rees, H. A., Packer, M. S., Badran, A. H., Bryson, D. I., et al. (2017). Programmable base editing of A∗T to G∗C in genomic DNA without DNA cleavage. Nature 551, 464–471. doi: 10.1038/nature24644
Geary, R. S., Watanabe, T. A., Truong, L., Freier, S., Lesnik, E. A., Sioufi, N. B., et al. (2001). Pharmacokinetic properties of 2’-O-(2-methoxyethyl)-modified oligonucleotide analogs in rats. J. Pharmacol. Exp. Therap. 296, 890–897.
Giardine, B., van Baal, S., Kaimakis, P., Riemer, C., Miller, W., Samara, M., et al. (2007). HbVar database of human hemoglobin variants and thalassemia mutations: 2007 update. Hum. Mutat. 28, 206. doi: 10.1002/humu.9479
Giljohann, D. A., Seferos, D. S., Daniel, W. L., Massich, M. D., Patel, P. C., and Mirkin, C. A. (2010). Gold nanoparticles for biology and medicine. Angew. Chem. Int. Ed. Engl. 49, 3280–3294.
Gordillo-Galeano, A., and Mora-Huertas, C. E. (2018). Solid lipid nanoparticles and nanostructured lipid carriers: a review emphasizing on particle structure and drug release. Eur. J. Pharm. Biopharm. 133, 285–308. doi: 10.1016/j.ejpb.2018.10.017
Gorman, L., Mercatante, D. R., and Kole, R. (2000). Restoration of correct splicing of thalassemic beta-globin pre-mRNA by modified U1 snRNAs. J. Biol. Chem. 275, 35914–35919. doi: 10.1074/jbc.m006259200
Goyenvalle, A., Vulin, A., Fougerousse, F., Leturcq, F., Kaplan, J. C., Garcia, L., et al. (2004). Rescue of dystrophic muscle through U7 snRNA-mediated exon skipping. Science 306, 1796–1799. doi: 10.1126/science.1104297
Gref, R., Luck, M., Quellec, P., Marchand, M., Dellacherie, E., Harnisch, S., et al. (2000). ‘Stealth’ corona-core nanoparticles surface modified by polyethylene glycol (PEG): influences of the corona (PEG chain length and surface density) and of the core composition on phagocytic uptake and plasma protein adsorption. Colloids Surf. B Biointerfaces 18, 301–313. doi: 10.1016/s0927-7765(99)00156-3
Habara, Y., Takeshima, Y., Awano, H., Okizuka, Y., Zhang, Z., Saiki, K., et al. (2009). In vitro splicing analysis showed that availability of a cryptic splice site is not a determinant for alternative splicing patterns caused by (1G–A mutations in introns of the dystrophin gene. J. Med. Genet. 46, 542–547. doi: 10.1136/jmg.2008.061259
Hacein-Bey-Abina, S., Von Kalle, C., Schmidt, M., McCormack, M. P., Wulffraat, N., Leboulch, P., et al. (2003). LMO2-associated clonal T cell proliferation in two patients after gene therapy for SCID-X1. Science 302, 415–419. doi: 10.1126/science.1088547
Hanlon, K. S., Kleinstiver, B. P., Garcia, S. P., Zaborowski, M. P., Volak, A., Spirig, S. E., et al. (2019). High levels of AAV vector integration into CRISPR-induced DNA breaks. Nat. Commun. 10:4439.
Harbour, J. W., Roberson, Anbunathan, H., Onken, M. D., Worley, L. A., and Bowcock, A. M. (2013). Recurrent mutations at codon 625 of the splicing factor SF3B1 in uveal melanoma. Nat. Genet. 45, 133–135. doi: 10.1038/ng.2523
Hardwick, S. A., Joglekar, A., Flicek, P., Frankish, A., and Tilgner, H. U. (2019). Getting the entire message: progress in isoform sequencing. Front. Genet. 10:709. doi: 10.3389/fgene.2019.00709
Havens, M. A., Duelli, D. M., and Hastings, M. L. (2013). Targeting RNA splicing for disease therapy. Wiley Interdiscipl. Rev. RNA 4, 247–266. doi: 10.1002/wrna.1158
Havens, M. A., and Hastings, M. L. (2016). Splice-switching antisense oligonucleotides as therapeutic drugs. Nucleic Acids Res. 44, 6549–6563. doi: 10.1093/nar/gkw533
Hayes, G. M., Carrigan, P. E., Beck, A. M., and Miller, L. J. (2006). Targeting the RNA splicing machinery as a novel treatment strategy for pancreatic carcinoma. Cancer Res. 66, 3819–3827. doi: 10.1158/0008-5472.can-05-4065
Hazer, B., and Steinbuchel, A. (2007). Increased diversification of polyhydroxyalkanoates by modification reactions for industrial and medical applications. Appl. Microbiol. Biotechnol. 74, 1–12. doi: 10.1007/s00253-006-0732-8
He, H., Liyanarachchi, S., Akagi, K., Nagy, R., Li, J., Dietrich, R. C., et al. (2011). Mutations in U4atac snRNA, a component of the minor spliceosome, in the developmental disorder MOPD I. Science 332, 238–240. doi: 10.1126/science.1200587
Hermans, P. W., van Soolingen, D., Bik, E. M., de Haas, P. E., Dale, J. W., and van Embden, J. D. (1991). Insertion element IS987 from Mycobacterium bovis BCG is located in a hot-spot integration region for insertion elements in Mycobacterium tuberculosis complex strains. Infect. Immun. 59, 2695–2705. doi: 10.1128/iai.59.8.2695-2705.1991
Hess, G. T., Tycko, J., Yao, D., and Bassik, M. C. (2017). Methods and applications of CRISPR-mediated base editing in eukaryotic genomes. Mol. Cell. 68, 26–43. doi: 10.1016/j.molcel.2017.09.029
Ho, C., Musa, F., Bell, C., and Walker, S. (2015). LDLR gene synonymous mutation c.1813C> T results in mRNA splicing variation in a kindred with familial hypercholesterolaemia. Ann. Clin. Biochem. 52, 680–684. doi: 10.1177/0004563215572702
Hong, D., Kurzrock, R., Kim, Y., Woessner, R., Younes, A., Nemunaitis, J., et al. (2015). AZD9150, a next-generation antisense oligonucleotide inhibitor of STAT3 with early evidence of clinical activity in lymphoma and lung cancer. Sci. Transl. Med. 7:314ra185. doi: 10.1126/scitranslmed.aac5272
Hosseini, E. S., Nikkhah, M., and Hosseinkhani, S. (2019). Cholesterol-rich lipid-mediated nanoparticles boost of transfection efficiency, utilized for gene editing by CRISPR-Cas9. Int. J. Nanomed. 14, 4353–4366. doi: 10.2147/ijn.s199104
Hsu, P. D., Lander, E. S., and Zhang, F. (2014). Development and applications of CRISPR-Cas9 for genome engineering. Cell 157, 1262–1278. doi: 10.1016/j.cell.2014.05.010
Hunter, A. C. (2006). Molecular hurdles in polyfectin design and mechanistic background to polycation induced cytotoxicity. Adv. Drug Deliv. Rev. 58, 1523–1531. doi: 10.1016/j.addr.2006.09.008
Hutton, M., Lendon, C. L., Rizzu, P., Baker, M., Froelich, S., Houlden, H., et al. (1998). Association of missense and 5’-splice-site mutations in tau with the inherited dementia FTDP-17. Nature 393, 702–705.
Imielinski, M., Berger, A. H., Hammerman, P. S., Hernandez, B., Pugh, T. J., Hodis, E., et al. (2012). Mapping the hallmarks of lung adenocarcinoma with massively parallel sequencing. Cell 150, 1107–1120.
Ishino, Y., Shinagawa, H., Makino, K., Amemura, M., and Nakata, A. (1987). Nucleotide sequence of the iap gene, responsible for alkaline phosphatase isozyme conversion in Escherichia coli, and identification of the gene product. J. Bacteriol. 169, 5429–5433. doi: 10.1128/jb.169.12.5429-5433.1987
Iwatani-Yoshihara, M., Ito, M., Klein, M. G., Yamamoto, T., Yonemori, K., Tanaka, T., et al. (2017). Discovery of allosteric inhibitors targeting the spliceosomal RNA helicase Brr2. J. Med. Chem. 60, 5759–5771. doi: 10.1021/acs.jmedchem.7b00461
Jambhekar, S. S., and Breen, P. (2016). CDs in pharmaceutical formulations I: structure and physicochemical properties, formation of complexes, and types of complex. Drug Discov. Today 21, 356–362. doi: 10.1016/j.drudis.2015.11.017
Jia, C., Chen, H., Wei, M., Chen, X., Zhang, Y., Cao, L., et al. (2017). Gold nanoparticle-based miR155 antagonist macrophage delivery restores the cardiac function in ovariectomized diabetic mouse model. Int. J. Nanomed. 12, 4963–4979. doi: 10.2147/ijn.s138400
Jinek, M., Chylinski, K., Fonfara, I., Hauer, M., Doudna, J. A., and Charpentier, E. (2012). A programmable dual-RNA-guided DNA endonuclease in adaptive bacterial immunity. Science 337, 816–821. doi: 10.1126/science.1225829
Kaemmerer, W. F. (2018). How will the field of gene therapy survive its success? Bioeng. Transl. Med. 3, 166–177. doi: 10.1002/btm2.10090
Kemaladewi, D. U., Bassi, P. S., Erwood, S., Al-Basha, D., Gawlik, K. I., Lindsay, K., et al. (2019). A mutation-independent approach for muscular dystrophy via upregulation of a modifier gene. Nature 572, 125–130. doi: 10.1038/s41586-019-1430-x
Kemaladewi, D. U., Maino, E., Hyatt, E., Hou, H., Ding, M., Place, K. M., et al. (2017). Correction of a splicing defect in a mouse model of congenital muscular dystrophy type 1A using a homology-directed-repair-independent mechanism. Nat. Med. 23, 984–989. doi: 10.1038/nm.4367
Kennedy, P. J., Sousa, F., Ferreira, D., Pereira, C., Nestor, M., Oliveira, C., et al. (2018). Fab-conjugated PLGA nanoparticles effectively target cancer cells expressing human CD44v6. Acta Biomater. 81, 208–218. doi: 10.1016/j.actbio.2018.09.043
Kim, J., Hu, C., Moufawad, El Achkar, C., Black, L. E., Douville, J., et al. (2019). Patient-customized oligonucleotide therapy for a rare genetic disease. N. Engl. J. Med. 381, 1644–1652.
Knott, G. J., and Doudna, J. A. (2018). CRISPR-Cas guides the future of genetic engineering. Science 361, 866–869. doi: 10.1126/science.aat5011
Kolhar, P., Anselmo, A. C., Gupta, V., Pant, K., Prabhakarpandian, B., Ruoslahti, E., et al. (2013). Using shape effects to target antibody-coated nanoparticles to lung and brain endothelium. Proc. Natl. Acad. Sci. U.S.A. 110, 10753–10758. doi: 10.1073/pnas.1308345110
Komor, A. C., Kim, Y. B., Packer, M. S., Zuris, J. A., and Liu, D. R. (2016). Programmable editing of a target base in genomic DNA without double-stranded DNA cleavage. Nature 533, 420–424. doi: 10.1038/nature17946
Krzyszton, R., Salem, B., Lee, D. J., Schwake, G., Wagner, E., and Radler, J. O. (2017). Microfluidic self-assembly of folate-targeted monomolecular siRNA-lipid nanoparticles. Nanoscale 9, 7442–7453. doi: 10.1039/c7nr01593c
Lapuk, A., Marr, H., Jakkula, L., Pedro, H., Bhattacharya, S., Purdom, E., et al. (2010). Exon-level microarray analyses identify alternative splicing programs in breast cancer. Mol. Cancer Res. 8, 961–974. doi: 10.1158/1541-7786.mcr-09-0528
Lee, K., Conboy, M., Park, H. M., Jiang, F., Kim, H. J., Dewitt, M. A., et al. (2017). Nanoparticle delivery of Cas9 ribonucleoprotein and donor DNA in vivo induces homology-directed DNA repair. Nat. Biomed. Eng. 1, 889–901. doi: 10.1038/s41551-017-0137-2
Lee, S. C., Dvinge, H., Kim, E., Cho, H., Micol, J. B., Chung, Y. R., et al. (2016). Modulation of splicing catalysis for therapeutic targeting of leukemia with mutations in genes enconding spliceosomal proteins. Nat. Med. 22, 672–678. doi: 10.1038/nm.4097
Lelli, N., Garuti, R., Ghisellini, M., Tiozzo, R., Rolleri, M., Aimale, V., et al. (1995). Ocurrence of multiple aberrantly spliced mRNAs of the LDL-receptor gene upon a donor splice site mutation that causes familial hypercholesterolemia (FHBenevento). J. Lipid Res. 36, 1315–1324.
Li, H. L., Fujimoto, N., Sasakawa, N., Shirai, S., Ohkame, T., Sakuma, T., et al. (2015). Precise correction of the dystrophin gene in duchenne muscular dystrophy patient induced pluripotent stem cells by TALEN and CRISPR-Cas9. Stem Cell Rep. 4, 143–154. doi: 10.1016/j.stemcr.2014.10.013
Limeres, M. J., Sune-Pou, M., Prieto-Sanchez, S., Moreno-Castro, C., Nusblat, A. D., Hernandez-Munain, C., et al. (2019). Development and characterization of an improved formulation of cholesteryl oleate-loaded cationic solid-lipid nanoparticles as an efficient non-viral gene delivery system. Colloids Surf. B Biointerfaces 184:110533. doi: 10.1016/j.colsurfb.2019.110533
Lin, J. C. (2017). Therapeutic applications of targeted alternative splicing to cancer treatment. Int. J. Mol. Sci. 19:75. doi: 10.3390/ijms19010075
Lopez-Bigas, N., Audit, B., Ouzounis, C., Parra, G., and Guigo, R. (2005). Are splicing mutations the most frequent cause of hereditary disease? FEBS Lett. 579, 1900–1903. doi: 10.1016/j.febslet.2005.02.047
Lorson, C. L., Hahnen, E., Androphy, E. J., and Wirth, B. (1999). A single nucleotide in the SMN gene regulates splicing and is responsible for spinal muscular atrophy. Proc. Natl. Acad. Sci. U.SA. 96, 6307–6311. doi: 10.1073/pnas.96.11.6307
Lu, X. Y., Ciraolo, E., Stefenia, R., Chen, G. Q., Zhang, Y., and Hirsch, E. (2011). Sustained release of PI3K inhibitor from PHA nanoparticles and in vitro growth inhibition of cancer cell lines. Appl. Microbiol. Biotechnol. 89, 1423–1433. doi: 10.1007/s00253-011-3101-1
Maiseyeu, A., Mihai, G., Roy, S., Kherada, N., Simonetti, O. P., Sen, C. K., et al. (2010). Detection of macrophages via paramagnetic vesicles incorporating oxidatively tailored cholesterol ester: an approach for atherosclerosis imaging. Nanomedicine (Lond) 5, 1341–1356. doi: 10.2217/nnm.10.87
Mali, P., Yang, L., Esvelt, K. M., Aach, J., Guell, M., DiCarlo, J. E., et al. (2013). RNA-guided human genome engineering via Cas9. Science 339, 823–826. doi: 10.1126/science.1232033
Matera, A. G., and Wang, Z. (2014). A day in the life of the spliceosome. Nat. Rev. Mol. Cell Biol. 15, 108–121. doi: 10.1038/nrm3742
Mathew, V., and Wang, A. K. (2019). Inotersen: new promise for the treatment of hereditary transthyretin amyloidosis. Drug Design Dev. Ther. 13, 1515–1525. doi: 10.2147/dddt.s162913
Matos, L., Canals, I., Dridi, L., Choi, Y., Prata, M. J., Jordan, P., et al. (2014). Therapeutic strategies based on modified U1 snRNAs and chaperones for Sanfilippo C splicing mutations. Orphanet. J. Rare Dis. 9:180.
Mattioli, C., Pianigiani, G., De Rocco, D., Bianco, A. M., Cappelli, E., Savoia, A., et al. (2014). Unusual splice site mutations disrupt FANCA exon 8 definition. Biochim. Biophys. Acta 1842, 1052–1058. doi: 10.1016/j.bbadis.2014.03.014
McCracken, S., Fong, N., Yankulov, K., Ballantyne, S., Pan, G., Greenblatt, J., et al. (1997). The C-terminal domain of RNA polymerase II couples mRNA processing to transcription. Nature 385, 357–361. doi: 10.1038/385357a0
Meister, G., and Tuschl, T. (2004). Mechanisms of gene silencing by double-stranded RNA. Nature 431, 343–349. doi: 10.1038/nature02873
Mojica, F. J., Juez, G., and Rodriguez-Valera, F. (1993). Transcription at different salinities of Haloferax mediterranei sequences adjacent to partially modified PstI sites. Mol. Microbiol. 9, 613–621. doi: 10.1111/j.1365-2958.1993.tb01721.x
Monani, U. R., Lorson, C. L., Parsons, D. W., Prior, T. W., Androphy, E. J., Burghes, A. H., et al. (1999). A single nucleotide difference that alters splicing patterns distinguishes the SMA gene SMN1 from the copy gene SMN2. Hum. Mol. Genet. 8, 1177–1183. doi: 10.1093/hmg/8.7.1177
Montes, M., Cloutier, A., Sánchez-Hernández, N., Michelle, L., Lemieux, B., Blanchette, M., et al. (2012). TCERG1 regulates alternative splicing of Bcl-x gene by modulating the rate of RNAPII transcription. Mol. Cell. Biol. 32, 751–762. doi: 10.1128/mcb.06255-11
Mukheem, A., Muthoosamy, K., Manickam, S., Sudesh, K., Shahabuddin, S., Saidur, R., et al. (2018). Fabrication and characterization of an electrospun PHA/graphene silver nanocomposite scaffold for antibacterial applications. Materials (Basel) 11:1673. doi: 10.3390/ma11091673
Muller, R. H., Mader, K., and Gohla, S. (2000). Solid lipid nanoparticles (SLN) for controlled drug delivery – a review of the state of the art. Eur. J. Pharm. Biopharm. 50, 161–177. doi: 10.1016/s0939-6411(00)00087-4
Mustafa, S., Devi, V. K., and Pai, R. S. (2017). Effect of PEG and water-soluble chitosan coating on moxifloxacin-loaded PLGA long-circulating nanoparticles. Drug Deliv. Transl. Res. 7, 27–36. doi: 10.1007/s13346-016-0326-7
Naftelberg, S., Schor, I. E., Ast, G., and Kornblihtt, A. R. (2015). Regulation of alternative splicing through coupling with transcription and chromatin structure. Annu. Rev. Biochem. 84, 165–198. doi: 10.1146/annurev-biochem-060614-034242
Nielsen, K. B., Sorensen, S., Cartegni, L., Corydon, T. J., Doktor, T. K., Schroeder, L. D., et al. (2007). Seemingly neutral polymorphic variants may confer immunity to splicing-inactivating mutations: a synonymous snp in exon 5 of MCAD protects from deleterious mutations in a flanking exonic splicing enhancer. Am. J. Hum. Genet. 80, 416–432. doi: 10.1086/511992
Nielsen, L. R., and Schwartz Scheibel, E. (1992). Screening for mutations in the gene encoding factor IX. J. Inher Metab. Dis. 15, 339–341. doi: 10.1007/bf02435971
Nik-Zainal, S., Davies, H., Staaf, J., Ramakrishna, M., Glodzik, D., Zou, X., et al. (2016). Landscape of somatic mutations in 560 breast cancer whole-genome sequences. Nature 534, 47–54.
Nissim-Rafinia, M., and Kerem, B. (2006). Splicing modulation as a modifier of the CFTR function. Prog. Mol. Subcell Biol. 44, 233–254. doi: 10.1007/978-3-540-34449-0_10
Norcliffe-Kaufmann, L., and Kaufmann, H. (2012). Familial dysautonomia (Riley-Day syndrome): when baroreceptor feedback fails. Auton Neurosci. 172, 26–30. doi: 10.1016/j.autneu.2012.10.012
Osborn, M. J., Newby, G. A., McElroy, A. N., Knipping, F., Nielsen, S. C., Riddle, M. J., et al. (2020). Base editor correction of COL7A1 in recessive dystrophic epidermolysis bullosa patient-derived fibroblasts and iPSCs. J. Invest. Dermatol. 140:e335.
Ousterout, D. G., Kabadi, A. M., Thakore, P. I., Perez-Pinera, P., Brown, M. T., Majoros, W. H., et al. (2015). Correction of dystrophin expression in cells from Duchenne muscular dystrophy patients through genomic excision of exon 51 by zinc finger nucleases. Mol. Ther. 23, 523–532. doi: 10.1038/mt.2014.234
Owen, N., Zhou, H., Malygin, A. A., Sangha, J., Smith, L. D., Muntoni, F., et al. (2011). Design principles for bifunctional targeted oligonucleotide enhancers of splicing. Nucleic Acids Res. 39, 7194–7208. doi: 10.1093/nar/gkr152
Paliwal, R., Babu, R. J., and Palakurthi, S. (2014). Nanomedicine scale-up technologies: feasibilities and challenges. AAPS PharmSciTech. 15, 1527–1534. doi: 10.1208/s12249-014-0177-9
Pan, Q., Shai, O., Lee, L. J., Frey, B. J., and Blencowe, B. J. (2008). Deep surveying of alternative splicing complexity in the human transcriptome by high-throughput sequencing. Nat. Genet. 40, 1413–1415. doi: 10.1038/ng.259
Park, E., Pan, Z., Zhang, Z., Lin, L., and Xing, Y. (2018). The expanding landscape of alternative splicing variation in human populations. Am. J. Hum. Genet. 102, 11–26. doi: 10.1016/j.ajhg.2017.11.002
Parkesh, R., Childs-Disney, J. L., Nakamori, M., Kumar, A., Wang, E., Wang, T., et al. (2012). Design of a bioactive small molecule that targets the myotonic dystrophy type 1 RNA via an RNA motif-ligand database and chemical similarity searching. J. Am. Chem. Soc. 134, 4731–4742. doi: 10.1021/ja210088v
Pinotti, M., Balestra, D., Rizzotto, L., Maestri, I., Pagani, F., and Bernardi, F. (2009). Rescue of coagulation factor VII function by the U1+5A snRNA. Blood 113, 6461–6464. doi: 10.1182/blood-2009-03-207613
Pinotti, M., Rizzotto, L., Balestra, D., Lewandowska, M. A., Cavallari, N., Marchetti, G., et al. (2008). U1-snRNA-mediated rescue of mRNA processing in severe factor VII deficiency. Blood 111, 2681–2684. doi: 10.1182/blood-2007-10-117440
Planté-Bordeneuve, V., and Said, G. (2011). Familial amyloid polyneuropathy. Lancet Neurol. 10, 1086–1097.
Porteus, M. H., and Baltimore, D. (2003). Chimeric nucleases stimulate gene targeting in human cells. Science 300:763. doi: 10.1126/science.1078395
Procter, A. M., Phillips, J. A., and Cooper, D. N. (1998). The molecular genetics of growth hormone deficiency. Hum. Genet. 103, 255–272.
Qiao, C., Liu, J., Yang, J., Li, Y., Weng, J., Shao, Y., et al. (2016). Enhanced non-inflammasome mediated immune responses by mannosylated zwitterionic-based cationic liposomes for HIV DNA vaccines. Biomaterials 85, 1–17. doi: 10.1016/j.biomaterials.2016.01.054
Radu, I. C., Hudita, A., Zaharia, C., Galateanu, B., Iovu, H., Tanasa, E. V., et al. (2019). Poly(3-hydroxybutyrate-CO-3-hydroxyvalerate) PHBHV biocompatible nanocarriers for 5-FU delivery targeting colorectal cancer. Drug Deliv. 26, 318–327. doi: 10.1080/10717544.2019.1582729
Ramamoorth, M., and Narvekar, A. (2015). Non viral vectors in gene therapy- an overview. J. Clin. Diagn Res. 9, GE01–GE06.
Rassu, G., Soddu, E., Posadino, A. M., Pintus, G., Sarmento, B., Giunchedi, P., et al. (2017). Nose-to-brain delivery of BACE1 siRNA loaded in solid lipid nanoparticles for Alzheimer’s therapy. Colloids Surf. B Biointerfaces 152, 296–301. doi: 10.1016/j.colsurfb.2017.01.031
Ratni, H., Ebeling, M., Baird, J., Bendels, S., Bylund, J., Chen, K. S., et al. (2018). Discovery of risdiplam, a selective survival of motor neuron-2 (SMN2) gene splicing modifier for the treatment of spinal muscular atrophy (SMA). J. Med. Chem. 61, 6501–6517.
Rees, H. A., and Liu, D. R. (2018). Base editing: precision chemistry on the genome and transcriptome of living cells. Nat. Rev. Genet. 19, 770–788. doi: 10.1038/s41576-018-0059-1
Rizk, M., and Tuzmen, S. (2019). Patisiran for the treatment of patients with familial amyloid polyneuropathy. Drugs Today (Barc) 55, 315–327.
Rodriguez-Martin, T., Anthony, K., Garcia-Blanco, M. A., Mansfield, S. G., Anderton, B. H., and Gallo, J. M. (2009). Correction of tau mis-splicing caused by FTDP-17 MAPT mutations by spliceosome-mediated RNA trans-splicing. Hum. Mol. Genet. 18, 3266–3273. doi: 10.1093/hmg/ddp264
Rodriguez-Martin, T., Garcia-Blanco, M. A., Mansfield, S. G., Grover, A. C., Hutton, M., Yu, Q., et al. (2005). Reprogramming of tau alternative splicing by spliceosome-mediated RNA trans-splicing: implications for tauopathies. Proc. Natl. Acad. Sci. U.S.A. 102, 15659–15664. doi: 10.1073/pnas.0503150102
Ross, S. J., Revenko, A. S., Hanson, L. L., Ellston, R., Staniszewska, A., Whalley, N., et al. (2017). Targeting KRAS-dependent tumors with AZD4785, a high-affinity therapeutic antisense oligonucleotide inhibitor of KRAS. Sci. Transl. Med. 9:eaal5253. doi: 10.1126/scitranslmed.aal5253
Ryther, R. C., Flynt, A. S., Harris, B. D., Phillips, J. A. III, and Patton, J. G. (2004). GH1 splicing is regulated by multiple enhancers whose mutation produces a dominant-negative GH isoform that can be degraded by allele-specific small interfering RNA (siRNA). Endocrinology 145, 2988–2996. doi: 10.1210/en.2003-1724
Sathasivam, K., Neueder, A., Gipson, T. A., Landles, C., Benjamin, A. C., Bondulich, M. K., et al. (2013). Aberrant splicing of HTT generates the pathogenic exon 1 protein in Huntington disease. Proc. Natl. Acad. Sci. U.S.A. 110, 2366–2370. doi: 10.1073/pnas.1221891110
Scalet, D., Balestra, D., Rohban, S., Bovolenta, M., Perrone, D., Bernardi, F., et al. (2017). Exploring splicing-switching molecules for seckel syndrome therapy. Biochim. Biophys. Acta Mol. Basis Dis. 1863, 15–20. doi: 10.1016/j.bbadis.2016.09.011
Scalet, D., Sacchetto, C., Bernardi, F., Pinotti, M., van de Graaf, S. F. J., and Balestra, D. (2018). The somatic FAH C.1061C> A change counteracts the frequent FAH c.1062+5G > A mutation and permits U1snRNA-based splicing correction. J. Hum. Genet. 63, 683–686. doi: 10.1038/s10038-018-0427-x
Schliecker, G., Schmidt, C., Fuchs, S., and Kissel, T. (2003). Characterization of a homologous series of D,L-lactic acid oligomers; a mechanistic study on the degradation kinetics in vitro. Biomaterials 24, 3835–3844. doi: 10.1016/s0142-9612(03)00243-6
Schmid, F., Hiller, T., Korner, G., Glaus, E., Berger, W., and Neidhardt, J. (2013). A gene therapeutic approach to correct splice defects with modified U1 and U6 snRNPs. Hum. Gene Ther. 24, 97–104. doi: 10.1089/hum.2012.110
Schornack, S., Meyer, A., Romer, P., Jordan, T., and Lahaye, T. (2006). Gene-for-gene-mediated recognition of nuclear-targeted AvrBs3-like bacterial effector proteins. J. Plant Physiol. 163, 256–272. doi: 10.1016/j.jplph.2005.12.001
Scotti, M. M., and Swanson, M. S. (2016). RNA mis-splicing in disease. Nat. Rev. Genet. 17, 19–32. doi: 10.1038/nrg.2015.3
Sharma, V. K., and Watts, J. K. (2015). Oligonucleotide therapeutics: chemistry, delivery and clinical progress. Future Med. Chem. 7, 2221–2242. doi: 10.4155/fmc.15.144
Shi, J., Xu, Y., Xu, X., Zhu, X., Pridgen, E., Wu, J., et al. (2014). Hybrid lipid-polymer nanoparticles for sustained siRNA delivery and gene silencing. Nanomedicine 10, 897–900.
Siomi, M. C., Sato, K., Pezic, D., and Aravin, A. A. (2011). PIWI-interacting small RNAs: the vanguard of genome defence. Nat. Rev. Mol. Cell Biol. 12, 246–258. doi: 10.1038/nrm3089
Skordis, L. A., Dunckley, M. G., Yue, B., Eperon, I. C., and Muntoni, F. (2003). Bifunctional antisense oligonucleotides provide a trans-acting splicing enhancer that stimulates SMN2 gene expression in patient fibroblasts. Proc. Natl. Acad. Sci. U.S.A. 100, 4114–4119. doi: 10.1073/pnas.0633863100
Smith, J. L., Mou, H., and Xue, W. (2018). Understanding and repurposing CRISPR-mediated alternative splicing. Genome Biol. 19:184.
Smith, L. M., Kelleher, N. L., and Consortium for Top Down, P. (2013). Proteoform: a single term describing protein complexity. Nat. Methods 10, 186–187. doi: 10.1038/nmeth.2369
Somia, N., and Verma, I. M. (2000). Gene therapy: trials and tribulations. Nat. Rev. Genet. 1, 91–99. doi: 10.1038/35038533
Song, C. Q., Jiang, T., Richter, M., Rhym, L. H., Koblan, L. W., Zafra, M. P., et al. (2020). Adenine base editing in an adult mouse model of tyrosinaemia. Nat. Biomed. Eng. 4, 125–130. doi: 10.1038/s41551-019-0357-8
Song, Y., Lou, H. H., Boyer, J. L., Limberis, M. P., Vandenberghe, L. H., Hackett, N. R., et al. (2009). Functional cystic fibrosis transmembrane conductance regulator expression in cystic fibrosis airway epithelial cells by AAV6.2-mediated segmental trans-splicing. Hum. Gene Ther. 20, 267–281. doi: 10.1089/hum.2008.173
Sreedharan, J., Blair, I. P., Tripathi, V. B., Hu, X., Vance, C., Rogelj, B., et al. (2008). TDP-43 mutations in familial and sporadic amyotrophic lateral sclerosis. Science 319, 1668–1672.
Stadtmauer, E. A., Fraietta, J. A., Davis, M. M., Cohen, A. D., Weber, K. L., Lancaster, E., et al. (2020). CRISPR-engineered T cells in patients with refractory cancer. Science 367:eaba7365.
Stenson, P. D., Ball, E. V., Mort, M., Phillips, A. D., Shaw, K., and Cooper, D. N. (2012). The human gene mutation database (HGMD) and its exploitation in the fields of personalized genomics and molecular evolution. Curr. Protoc. Bioinform. Chapter 1:Unit113.
Stoilov, P., Lin, C. H., Damoiseaux, R., Nikolic, J., and Black, D. L. (2008). A high-throughput screening strategy identifies cardiotonic steroids as alternative splicing modulators. Proc. Natl. Acad. Sci. U.S.A. 105, 11218–11223. doi: 10.1073/pnas.0801661105
Strong, T. V., Wilkinson, D. J., Mansoura, M. K., Devor, D. C., Henze, K., Yang, Y., et al. (1993). Expression of an abundant alternatively spliced form of the cystic fibrosis transmembrane conductance regulator (CFTR) gene is not associated with a cAMP-activated chloride conductance. Hum. Mol. Genet. 2, 225–230. doi: 10.1093/hmg/2.3.225
Summerton, J. (1999). Morpholino antisense oligomers: the case for an RNase H-independent structural type. Biochim. Biophys. Acta 1489, 141–158. doi: 10.1016/s0167-4781(99)00150-5
Sun, L., Wang, D., Chen, Y., Wang, L., Huang, P., Li, Y., et al. (2017). Core-shell hierarchical mesostructured silica nanoparticles for gene/chemo-synergetic stepwise therapy of multidrug-resistant cancer. Biomaterials 133, 219–228. doi: 10.1016/j.biomaterials.2017.04.028
Sun, N., and Zhao, H. (2013). Transcription activator-like effector nucleases (TALENs): a highly efficient and versatile tool for genome editing. Biotechnol. Bioeng. 110, 1811–1821. doi: 10.1002/bit.24890
Sune-Pou, M., Prieto-Sanchez, S., Boyero-Corral, S., Moreno-Castro, C., El Yousfi, Y., Sune-Negre, J. M., et al. (2017). Targeting splicing in the treatment of human disease. Genes (Basel) 8:87. doi: 10.3390/genes8030087
Tabrizi, S. J., Leavitt, B. R., Landwehrmeyer, G. B., Wild, E. J., Saft, C., Barker, R. A., et al. (2019). Targeting huntingtin expression in patients with huntington’s disease. N. Engl. J. Med. 380, 2307–2316.
Tajnik, M., Rogalska, M. E., Bussani, E., Barbon, E., Balestra, D., Pinotti, M., et al. (2016). Molecular basis and therapeutic strategies to rescue factor IX variants that affect splicing and protein function. PLoS Genet. 12:e1006082. doi: 10.1371/journal.pgen.1006082
Takata, A., Matsumoto, N., and Kato, T. (2017). Genome-wide identification of splicing QTLs in the human brain and their enrichment among schizophrenia-associated loci. Nat. Commun. 8:14519.
Taniguchi-Ikeda, M., Kobayashi, K., Kanagawa, M., Yu, C., Mori, K., Oda, T., et al. (2011). Pathogenic exon-trapping by SVA retrotransposon and rescue in Fukuyama muscular dystrophy. Nature 478, 127–131. doi: 10.1038/nature10456
Tanner, G., Glaus, E., Barthelmes, D., Ader, M., Fleischhauer, J., Pagani, F., et al. (2009). Therapeutic strategy to rescue mutation-induced exon skipping in rhodopsin by adaptation of U1 snRNA. Hum. Mutat. 30, 255–263. doi: 10.1002/humu.20861
Taratula, O., Kuzmov, A., Shah, M., Garbuzenko, O. B., and Minko, T. (2013). Nanostructured lipid carriers as multifunctional nanomedicine platform for pulmonary co-delivery of anticancer drugs and siRNA. J. Control. Release 171, 349–357. doi: 10.1016/j.jconrel.2013.04.018
Tardy, B. L., Tan, S., Dam, H. H., Ejima, H., Blencowe, A., Qiao, G. G., et al. (2016). Nanoparticles assembled via pH-responsive reversible segregation of CDs in polyrotaxanes. Nanoscale 8, 15589–15596. doi: 10.1039/c6nr04841b
Taylor, J., and Lee, S. C. (2019). Mutations in spliceosome genes and therapeutic opportunities in myeloid malignancies. Genes Chromosomes Cancer. 58, 889–902. doi: 10.1002/gcc.22784
Tumer, Z. (2013). An overview and update of ATP7A mutations leading to menkes disease and occipital horn syndrome. Hum. Mutat. 34, 417–429. doi: 10.1002/humu.22266
Ullrich, N. J., and Gordon, L. B. (2015). Hutchinson-gilford progeria syndrome. Handb. Clin. Neurol. 132, 249–264.
Uner, M., and Yener, G. (2007). Importance of solid lipid nanoparticles (SLN) in various administration routes and future perspectives. Int. J. Nanomed. 2, 289–300.
van der Woerd, W. L., Mulder, J., Pagani, F., Beuers, U., Houwen, R. H., and van de Graaf, S. F. (2015). Analysis of aberrant pre-messenger RNA splicing resulting from mutations in ATP8B1 and efficient in vitro rescue by adapted U1 small nuclear RNA. Hepatology 61, 1382–1391. doi: 10.1002/hep.27620
Verma, A. (2018). Recent advances in antisense oligonucleotide therapy in genetic neuromuscular diseases. Ann. Indian Acad. Neurol. 21, 3–8.
Villemaire, J., Dion, I., Elela, S. A., and Chabot, B. (2003). Reprogramming alternative pre-messenger RNA splicing through the use of protein-binding antisense oligonucleotides. J. Biol. Chem. 278, 50031–50039. doi: 10.1074/jbc.m308897200
Wahl, M. C., Will, C. L., and Luhrmann, R. (2009). The spliceosome: design principles of a dynamic RNP machine. Cell 136, 701–718. doi: 10.1016/j.cell.2009.02.009
Wally, V., Murauer, E. M., and Bauer, J. W. (2012). Spliceosome-mediated trans-splicing: the therapeutic cut and paste. J. Invest. Dermatol. 132, 1959–1966. doi: 10.1038/jid.2012.101
Wan, R., Bai, R., and Shi, Y. (2019). Molecular choreography of pre-mRNA splicing by the spliceosome. Curr. Opin. Struct. Biol. 59, 124–133. doi: 10.1016/j.sbi.2019.07.010
Wang, E. T., Sandberg, R., Luo, S., Khrebtukova, I., Zhang, L., Mayr, C., et al. (2008). Alternative isoform regulation in human tissue transcriptomes. Nature 456, 470–476. doi: 10.1038/nature07509
Wang, X., Yang, L., Zhang, H., Tian, B., Li, R., Hou, X., et al. (2018). Fluorescent magnetic PEI-PLGA nanoparticles loaded with paclitaxel for concurrent cell imaging, enhanced apoptosis and autophagy in human brain cancer. Colloids Surf. B Biointerf. 172, 708–717. doi: 10.1016/j.colsurfb.2018.09.033
Warf, M. B., Nakamori, M., Matthys, C. M., Thornton, C. A., and Berglund, J. A. (2009). Pentamidine reverses the splicing defects associated with myotonic dystrophy. Proc. Natl. Acad. Sci. U.S.A. 106, 18551–18556. doi: 10.1073/pnas.0903234106
Wild, E. J., and Tabrizi, S. J. (2017). Therapies targeting DNA and RNA in Huntington’s disease. Lancet Neurol. 16, 837–847. doi: 10.1016/s1474-4422(17)30280-6
Willyard, C. (2018). New human gene tally reignites debate. Nature 558, 354–355. doi: 10.1038/d41586-018-05462-w
Wojtowicz, W. M., Wu, W., Andre, I., Qian, B., Baker, D., and Zipursky, S. L. (2007). A vast repertoire of Dscam binding specificities arises from modular interactions of variable Ig domains. Cell 130, 1134–1145. doi: 10.1016/j.cell.2007.08.026
Wu, J. Y., and Maniatis, T. (1993). Specific interactions between proteins implicated in splice site selection and regulated alternative splicing. Cell 75, 1061–1070. doi: 10.1016/0092-8674(93)90316-i
Xie, Q., Jia, L. N., Xu, H. Y., Hu, X. G., Wang, W., and Jia, J. (2016). Fabrication of core-shell PEI/pBMP2-PLGA electrospun scaffold for gene delivery to periodontal ligament stem cells. Stem Cells Int. 2016:5385137.
Xu, Z. P., Zeng, Q., Lu, G.-Q. M., and Yu, A. (2006). Inorganic nanoparticles as carriers for efficient cellular delivery. Chem. Eng. Sci. 61, 1027–1040. doi: 10.1016/j.ces.2005.06.019
Yanaizu, M., Sakai, K., Tosaki, Y., Kino, Y., and Satoh, J. (2018). Small nuclear RNA-mediated modulation of splicing reveals a therapeutic strategy for a TREM2 mutation and its post-transcriptional regulation. Sci. Rep. 8:6937.
Yang, X., Coulombe-Huntington, J., Kang, S., Sheynkman, G. M., Hao, T., Richardson, A., et al. (2016). Widespread expansion of protein interaction capabilities by alternative splicing. Cell 164, 805–817.
Yin, H., Kanasty, R. L., Eltoukhy, A. A., Vegas, A. J., Dorkin, J. R., and Anderson, D. G. (2014). Non-viral vectors for gene-based therapy. Nat. Rev. Genet. 15, 541–555.
Yoshida, K., Sanada, M., Shiraishi, Y., Nowak, D., Nagata, Y., Yamamoto, R., et al. (2011). Frequent pathway mutations of splicing machinery in myelodysplasia. Nature 478, 64–69.
Yuan, J., Ma, Y., Huang, T., Chen, Y., Peng, Y., Li, B., et al. (2018). Genetic modulation of RNA splicing with a CRISPR-guided cytidine deaminase. Mol. Cell 72:e387.
Zakharova, L., Pashirova, T., Kashapov, R., Gabdrakhmanov, D., and Sinyashin, O. (2017). Drug Delivery Mediated by Confined Nanosystems: Structure-Activity Relations and Factors Responsible for the Efficacy of Formulations, eds E.A.A.M. Grumezescu. Amsterdam: Elsevier.
Zeitler, B., Froelich, S., Marlen, K., Shivak, D. A., Yu, Q., Li, D., et al. (2019). Allele-selective transcriptional repression of mutant HTT for the treatment of Huntington’s disease. Nat. Med. 25, 1131–1142.
Zhang, J., Shishatskaya, E. I., Volova, T. G., da Silva, L. F., and Chen, G. Q. (2018). Polyhydroxyalkanoates (PHA) for therapeutic applications. Mater. Sci. Eng. C Mater. Biol. Appl. 86, 144–150. doi: 10.1016/j.msec.2017.12.035
Zhang, K., Tang, X., Zhang, J., Lu, W., Lin, X., Zhang, Y., et al. (2014). PEG-PLGA copolymers: their structure and structure-influenced drug delivery applications. J. Control. Release 183, 77–86. doi: 10.1016/j.jconrel.2014.03.026
Zhou, L., Chen, Z., Chi, W., Yang, X., Wang, W., and Zhang, B. (2012). Mono-methoxy-poly(3-hydroxybutyrate-co-4-hydroxybutyrate)-graft-hyper-branched polyethylenimine copolymers for siRNA delivery. Biomaterials 33, 2334–2344. doi: 10.1016/j.biomaterials.2011.11.060
Zhu, H., Tucker, H. M., Grear, K. E., Simpson, J. F., Manning, A. K., Cupples, L. A., et al. (2007). A common polymorphism decreases low-density lipoprotein receptor exon 12 splicing efficiency and associates with increased cholesterol. Hum. Mol. Genet. 16, 1765–1772. doi: 10.1093/hmg/ddm124
Keywords: splicing, RNA, gene therapy and therapeutic delivery, siRNAs, ASOs, SMaRT, gene editing, nanoparticle
Citation: Suñé-Pou M, Limeres MJ, Moreno-Castro C, Hernández-Munain C, Suñé-Negre JM, Cuestas ML and Suñé C (2020) Innovative Therapeutic and Delivery Approaches Using Nanotechnology to Correct Splicing Defects Underlying Disease. Front. Genet. 11:731. doi: 10.3389/fgene.2020.00731
Received: 17 February 2020; Accepted: 16 June 2020;
Published: 14 July 2020.
Edited by:
Rosanna Asselta, Humanitas University, ItalyReviewed by:
Dario Balestra, University of Ferrara, ItalyMauricio Fernando Budini, University of Chile, Chile
Copyright © 2020 Suñé-Pou, Limeres, Moreno-Castro, Hernández-Munain, Suñé-Negre, Cuestas and Suñé. This is an open-access article distributed under the terms of the Creative Commons Attribution License (CC BY). The use, distribution or reproduction in other forums is permitted, provided the original author(s) and the copyright owner(s) are credited and that the original publication in this journal is cited, in accordance with accepted academic practice. No use, distribution or reproduction is permitted which does not comply with these terms.
*Correspondence: Carlos Suñé, Y3N1bmVAaXBiLmNzaWMuZXM=
†These authors have contributed equally to this work