- 1School of Sciences and Centre for Marine Ecosystems Research, Edith Cowan University, Joondalup, WA, Australia
- 2The UWA Oceans Institute, University of Western Australia, Crawley, WA, Australia
- 3Centre d'Estudis Avançats de Blanes – Consejo Superior de Investigaciones Científicas, Blanes, Spain
- 4Institute of Environment, Health and Societies, Brunel University London, Uxbridge, UK
Seagrasses of the genus Posidonia can form an irregular seascape due to erosional processes exposing thick walls of organic matter-rich soils. However, little is known about the location and characteristics of these particular formations. Here we provide comprehensive estimates of organic carbon (Corg) storage in Posidonia oceanica and Posidonia australis meadows, while providing insight into their location and mechanisms of formation, and highlighting future research directions. Erosional reef escarpments are restricted to shallow highly productive P. oceanica meadows from the Mediterranean Sea and P. australis meadows from the Indian Ocean, and sustain the existence of Corg-rich deposits in surrounding meadows. The thickness of the mat escarpments can reach up to 3 m and their length can vary from few to hundreds of meters. Mechanisms of formation appear to differ among sites, from naturally-induced escarpments by wave action and/or tidal flow to human-induced escarpments by dredging activities. The inter-twined remains of seagrass shoots within the sediment matrix consolidate the sandy substrate and hold the exposed Posidonia mat escarpments together, maintaining a semi-rigid structure. This phenomenon is unusual but of exceptional importance in marine biogeochemical cycles, revealing the largest Corg sinks among seagrasses worldwide (ranging from 15 to 176 kg Corg m−2 in 2 m-thick mats accumulated at 2–249 g Corg m−2 yr−1 over 300–3000 yr).
Introduction
Seagrasses form dense and extensive coastal meadows extending from intertidal areas down to 40 m depth worldwide except in the Antarctica (Green and Short, 2003). The meadows are ecologically important as they support coastal communities essential for maintaining high biodiversity levels (Hemminga and Duarte, 2000). Seagrasses also provide other key ecosystem services such as shoreline protection against erosion by substrate stabilization and hydrodynamic energy dissipation (Green and Short, 2003; Boudouresque et al., 2014), and by providing a source of carbonate sand for beach formation (Canals and Ballesteros, 1997; Tigny et al., 2007).
Additionally, and noteworthy, seagrasses capacity to sequester and store organic carbon (Corg) contributes to the mitigation of anthropogenic CO2 emissions (Fourqurean et al., 2012; Duarte et al., 2013). In this sense, the leaf sheaths, rhizomes and roots detritus of the Mediterranean endemic Posidonia oceanica form a highly organic structure known as mat (Pérès and Picard, 1964; Boudouresque and Meinesz, 1982). The organic-rich deposits beneath the P. oceanica canopy can reach up to 13 m-thick and 6000 years of age, and contain massive carbon storage ranging from 40 to 770 kg Corg m−2 (Mateo et al., 1997; Lo Iacono et al., 2008; Serrano et al., 2014); little is known about the Corg storage and thickness of Posidonia australis mats (Paling and McComb, 2000). The mats of P. oceanica have started to be studied from palaeoecological viewpoints only recently (Lo Iacono et al., 2008; López-Sáez et al., 2009; Serrano et al., 2012, 2013; López-Merino et al., 2015), while the study of P. australis mats is at its onset (Rozaimi et al., 2013; Marbà et al., 2015; Serrano et al., 2016).
Several factors are involved in the accumulation of organic-rich material in the P. oceanica mat, resulting from the millenarian balance between material accretion (detritus and sediment), decomposition and erosion (Duarte and Cebrian, 1996; Mateo et al., 1997, 2006; Pergent et al., 1997; Gacia et al., 2002; Boudouresque et al., 2006). On the one hand, the rhizomes can reach lengths over 1 m, with both plagiotropic (horizontal) and orthotropic (vertical) growth (Pergent and Pergent-Martini, 1990), forming an extensive plant detritus network embedded within an inorganic sediment matrix (Pérès and Picard, 1964). On the other hand, plant detritus show a reduced decay inside the mat (Serrano et al., 2012) due to the refractory nature of the plant tissue (Kuo, 1978; Kuo and Cambridge, 1978; Harrison, 1989; Klap et al., 2000) and the anoxic environment inside the mat (Mateo et al., 1997, 2006). Although other seagrasses accumulate Corg (Lavery et al., 2013), no records for the storage of massive Corg have been reported so far for any other seagrass species but P. oceanica (Fourqurean et al., 2012). However, large quantities of decay-resistant organic matter have been reported for P. australis and Thalassodendron ciliatum (Mateo et al., 2006).
Seagrass meadows typically form a relatively homogeneous but highly diverse habitat in the near-shore environment, with the upper and lower depth limits determined, generally, by hydrodynamic conditions and light limitation (Duarte, 1991; Collier et al., 2008). Under some circumstances, the erosion of seagrass meadows can expose the mats creating erosional escarpments (Pérès and Picard, 1964; Mateo et al., 1997). The existence of exposed mat walls in P. oceanica meadows in the Mediterranean Sea and their erosive mechanism of formation were first described by Pérès and Picard (1964). However, the existence of escarpments of remarkable dimensions (up to 3 min height), and their process of formation and evolution remains largely unknown (Boudouresque et al., 2014). In this study, we want to start filling this gap by providing new information on P. australis (Indian Ocean) and P. oceanica (Mediterranean Sea) mat escarpments. Aiming to identify and compare the reef structures they form, we have collected literature data and complemented it with the study of new mats in order to unravel potential mechanisms of formation and carbon storage capacity, providing our viewpoints based on existing knowledge, field observations and expert judgment, discussing current advances and future research directions.
Materials and Methods
P. australis mats were sampled at Oyster Harbor, Big Lagoon, Waychinicup Inlet, and Port Pirie in Australia (Image 1 and Data Sheet 1). The Big Lagoon (Shark Bay) is a sheltered marine embayment consisting in a deep central channel surrounded by shallow seagrass meadows, while the Oyster Harbor and Waychinicup Inlet are estuaries, and the Port Pirie is a large marine embayment. The coring of the mats was carried out at a water depth of 2–3 m on continuous meadows, within 10 m of mat escarpments (vertical coring).
Up to 3 m-long mat cores were collected by manual percussion and rotation using 65 mm-diameter PVC pipes. Compression of sediments during coring was corrected by distributing the spatial discordances proportionally between the expected and the observed sediment layers (Glew et al., 2001). The overall degree of core shortening was < 30%. All results reported refer to the decompressed depths.
P. oceanica mats were sampled at Mellieha Bay and Salina Bay in Malta (Mediterranean Sea; Image 1 and Data Sheet 1). The sites consist of relatively sheltered sub-tidal basins. The mat cores were taken horizontally from exposed vertical mat walls (2–3 m water depth on top of the mat wall), using hand-operated PVC corers (100 cm long; 80 mm diameter). Three horizontal cores were sampled down the mat escarpment at 16, 73, and 145 cm depth at Salina Bay, and at 10, 40, 80, 120, 160, 200, and 230 cm depth at Mellieha Bay.
Cores were sealed and stored at 5°C before processing. The P. australis cores (vertical coring) were sub-sampled at 1 cm intervals. The outermost 10 cm of the horizontal P. oceanica cores were discarded to eliminate possible contamination with recent material; the remainder material from each level was homogenized and sub-sampled for analysis.
Samples were weighed before and after oven drying to constant weight at 70°C, and ground in a ball mill grinder. For organic carbon (Corg) analysis, 1 g of ground sample was acidified with 4% HCl, centrifuged (5 min at 3400 rpm), and the supernatant removed by pipette. The sample was then washed with Milli-Q water, centrifuged, and the supernatant removed again. The residual samples were re-dried and encapsulated for Corg analysis using a Micro Cube elemental analyzer (Elementar Analysensysteme GmbH) at the UC Davis Facilities. The content of Corg (in %) was calculated for the bulk (pre-acidified) sediment.
Fourteen samples of Posidonia sheath macro-remains, and eight samples of shells were radiocarbon dated following standard procedures (Stuiver and Pollack, 1977, Data Sheet 2). The sheath fibers and shells were rinsed in Milli-Q water, sonicated for 5 min to remove inorganic particles, and inspected for attached contaminants. The samples were dried at 60°C before radiocarbon dating. The dates were calibrated using CALIB 7.1 software with the MARINE13.14C curve (Stuiver and Reimer, 1993; Reimer et al., 2013) and corrected for the local DeltaR by subtracting 30–91 years at Australia (Bowman, 1985; Squire et al., 2013) and 70 years at Malta (Siani et al., 2000). Dates are expressed as calibrated years BP. The calibrated ages were used to produce age–depth models (linear regression).
Because the thickness of sampled mat varied among sites, we normalized the substrate thickness over which the Corg stocks and accumulation rates were calculated to allow comparisons. The Corg inventories per unit area (kg m−2) were estimated by multiplying the sediment dry bulk density (g cm−3) by the Corg concentration, and then normalized to g Corg m−2 (i.e., cumulative mass in a soil thickness of 2 m). The long-term accumulation rates (g m−2 yr−1) of Corg were calculated by multiplying the average Corg concentration by the sediment accumulation rates. Previously reported Corg stocks and accumulation rates in other P. oceanica mat sediment cores were also standardized to 2 m-thick deposits and compiled in Table 1. ANOVA was applied to test for any significant effect of species composition (P. oceanica and P. australis) on average Corg stocks and accumulation rates.
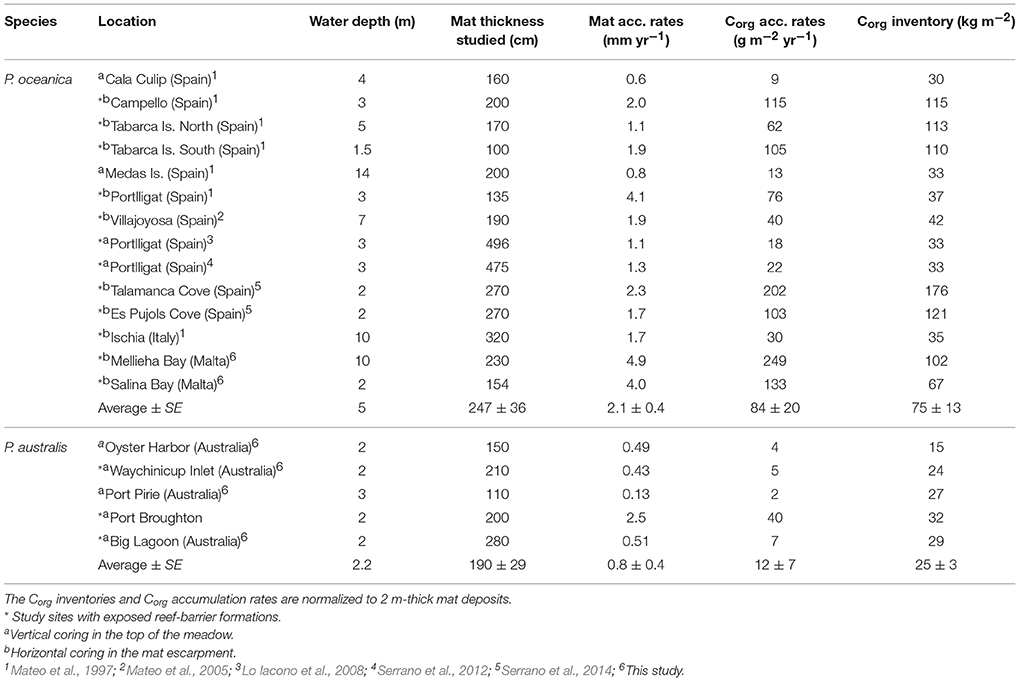
Table 1. Compilation of organic carbon (Corg) stocks and accumulation rates in P. oceanica and P. australis meadows.
Lifetime observations of mat escarpments in Posidonia meadows made by the authors of this manuscript are described. The maximum height of the reef escarpments surveyed was measured in situ and the length was estimated from combining in situ observations and aerial imagery.
Results and Discussion
Exposed reef escarpments are found in both P. australis and P. oceanica meadows (Figure 1). However, reef escarpments are not always present in seagrass and are restricted to highly productive Posidonia meadows that have likely been located in shallow (i.e., < 5 m depth) and relatively protected areas for the last 1000–4000 years (Data Sheet 2), which supported seagrass productivity and stability. The formation of these erosional structures appears to be related to hydrodynamic energy over present or centennial time scales (as either continuous or pulse events; Pérès and Picard (1964) or dredging activities, which can erode the edge of the meadow exposing the organic-rich deposits. The exposed face of seagrass mat is held together by the inter-twined remains of seagrass tissues, avoiding collapse and maintaining a semi-rigid structure, which is susceptible to erosion (i.e., in consolidated sediments) and can lead to the formation of vertical escarpments ranging from 1 to 3 m in thickness, and from < 100 m in length in P. oceanica to >500 m in P. australis meadows. Reports on escarpment of this size are not common and have only previously been described in the Mediterranean Sea, in particular in France (Molinier and Picard, 1952; Picard, 1953; Boudouresque et al., 1980, 1985, 1990; Boudouresque and Meinesz, 1982; Belsher et al., 2005), Spain (Mateo et al., 1997; Ribera et al., 1997; Serrano et al., 2014), and Italy (Mateo et al., 1997). Based on our observations we describe the existence of P. oceanica mat escarpments in several additional locations around the Mediterranean Sea. Additionally, we have described for the first time escarpments in P. australis in Australia: Big Lagoon, Waychinicup Inlet, Cheynes Bay, and Port Broughton (Image 1 and Data Sheet 1). Although, the dataset compiled provides a comprehensive summary, further studies are required to identify and describe erosional escarpments in seagrass meadows.
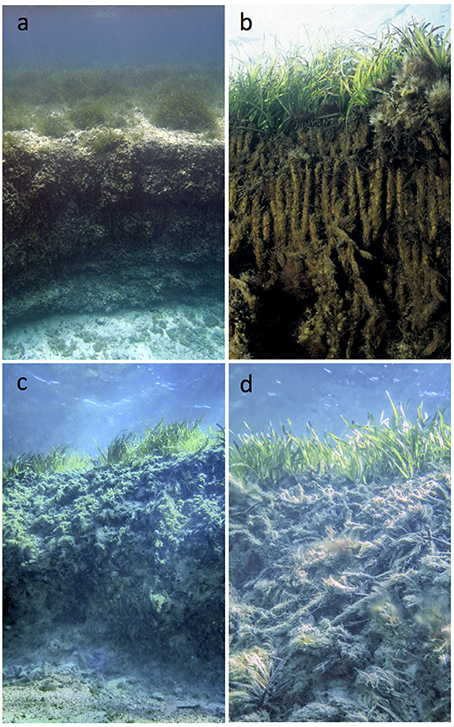
Figure 1. Erosional escarpment in a P. oceanica meadow in Es Pujols Cove (Formentera, Balearic Islands, Spain) illustrating the organic-rich soils (A,B), Erosional escarpment in a P. australis meadow in Big Lagoon (Shark Bay, Western Australia; C,D). The water depth at the top of the formation is 3 m, the exposed face of the mat has a thickness of 2.7 m and the age of the base of the exposure is 1200 cal. yr BP. The water depth at the top of the formation is 2 m, the exposed face has a thickness of 2.5 m and the age at the base of the exposure is 4000 cal. yr BP. Note the vertical rhizomal growth of P. oceanica (B) compared to the horizontal rhizomal growth of P. australis (D). Photo credit: M. A. Mateo (A), E. Ballesteros (B), P. Lavery (C), and O. Serrano (D).
The mechanisms of formation of erosional escarpments appear to differ among sites. In P. australis meadows at Big Lagoon (Shark Bay), tidal currents (tides up to 2 m) have created a deep channel allowing water exchange between the lagoon and the ocean. The channel passes through the shallow bank of seagrass, with water flow eroding and exposing the seagrass mats. At Cheynes Bay and Waychinicup Inlet (Albany), current and wave action could also lead to the erosion of P. australis meadows and the formation of reef escarpments up to 3 m-thick. However, severe river discharges could also explain the formation of mat escarpments at Waychinicup Inlet. In P. oceanica meadows of the Mediterranean Sea, where tides are < 0.5 m, escarpment formation is most likely related to waves and associated currents, in particular during extreme storm events. Human activities (e.g., dredging) that erode the base of the meadow, can also led to the formation of mat escarpments. At Port Broughton (Adelaide), and Sanitja Cove (Balearic Islands) dredging to clear shipping channel and boating activities led to the formation of mat escarpments.
Although, the mechanisms of formation of the Posidonia escarpments could differ among places, their dynamics could share some similarities (i.e., collapses due to erosion of its base by hydrodynamic action) with the formation of cliffs in rocky shores (Stephenson, 2000) or wrack banquettes along the beaches (Mateo et al., 2003). It seems probable that the hydrodynamic energy gradually erodes the base of the escarpment until the top edge of the mat collapses and, consequently, the meadow retreats (Pérès and Picard, 1964; Boudouresque et al., 1980). The curve shapes present along the base of the escarpments and the occasional presence of loose fragments of mat with living seagrass at the base of the escarpments supports this hypothesis. Further research aiming to unravel the mechanisms of formation, dynamics and processes within mat escarpments is required to test the hypotheses described above.
According to the age-depth models, accumulation rates in Posidonia meadows (i.e., mats close to the upper limit of seagrass distribution) ranged from 0.13 to 4.9 mm yr−1 (Table 1). The P. australis meadows sampled in this study contained in average 25 ± 3 kg Corg m−2 (in 2 m-thick deposits) accumulated at a rate of 12 ± 7 g Corg m−2 yr−1 over 1000–3500 years, the largest stocks recorded in seagrasses other than P. oceanica meadows (averaged 75 ± 13 kg Corg m−2 and 84 ± 20 g Corg m−2 yr−1; Table 1). The comparison of Corg storage capacity and accumulation rates by P. australis and P. oceanica meadows provide new insights into the significant differences in Corg storage potential among Posidonia seagrasses (P < 0.001 for both Corg storage and accumulation rates). The Corg storage in P. oceanica and P. australis meadows is exceptional compared to other seagrass species (ranging from 0.6 to 12 kg Corg m−2 in 1 m-thick deposits; Lavery et al., 2013; Campbell et al., 2015; Miyajima et al., 2015), and the presence of an exposed mat escarpment provides an early indication of rich Corg deposits beneath the surrounding meadows. Although, in this study we only surveyed directly mat escarpments (i.e., by horizontal coring) in Malta and Balearic Islands, we assumed that the Corg contents measured in the mats near the escarpments (i.e., by vertical coring) are representative of the Corg content in meadows close to the upper limit of seagrass distribution where escarpments can be found. The erosive nature of escarpments implies that their edges have retracted over time, and the exposure of the escarpments to oxic conditions and irradiance may lead to a shift in the Corg composition and accumulation in these areas due to the growth of e.g. algae and microbes compared to intact Corg stores underneath the meadows, but scientific evidence is lacking to support this hypothesis.
The three-fold higher Corg stores in P. oceanica compared to P. australis could be attributed to the higher sediment accumulation rates in P. oceanica meadows (2.1 ± 0.4 mm yr−1), enhanced by their vertical rhizomal growth (i.e., orthotropic) compared to the horizontal rhizomal growth (i.e., plagiotropic) in P. australis meadows (0.8 ± 0.4 mm yr−1; Table 1; Gobert et al., 2006). In Figure 1, we highlight the differences in the rhizomal growth between the two Posidonia species. The higher mat accumulation rate of P. oceanica meadows could also contribute to a greater preservation of Corg stores after burial, as a result of more rapid burial of Corg into anoxic conditions (i.e., typically occurring at about 5 cm depth in the mat; Mateo et al., 2006). In addition, the higher belowground biomass of P. oceanica (1610 g m−2; Duarte and Chiscano, 1999) compared to P. australis (658 g m−2; Paling and McComb, 2000) may contribute to the higher Corg stores in the Mediterranean species. The geomorphological setting (e.g., run-off, hydrodynamic energy and water depth) in which seagrass meadows are found can influence the Corg storage capacity of seagrasses (Serrano et al., 2015). Although, the number of cores and species studied in coastal and estuarine ecosystems was unbalanced (i.e., P. oceanica dominate in coastal habitats and P. australis dominate in estuarine habitats) and therefore we were not able to test the effects of coastal geomorphology on Corg storage, further studies are required to address its implications. According to the curve of the Holocene sea level change inferred along the Mediterranean (Lambeck and Bard, 2000) and Australian (Lambeck and Nakada, 1990) coasts, the sea level was constant since 6000–4000 years ago. Thus, assuming that seagrasses have been present at the same locations since the Mid-Holocene, the maximum potential thickness of seagrass mats is estimated to be 8 to 13 m for P. oceanica and 3 to 5 m for P. australis, based on the mat accumulation rates compiled in our study. At these mat depths, the potential carbon stocks would be 315–473 kg Corg m−2 in P. oceanica and 40–60 kg Corg m−2 in P. australis. Previous studies reported 691–770 kg Corg m−2 in 8–13 m thick deposits in P. oceanica based on the exceptional meadows at Balearic Island (Serrano et al., 2014), and from Rozaimi et al. (2016) it is possible to estimate 22–36 kg Corg m−2 in 3–5 m thick deposits in P. australis. However, the ranges reported in our study encompass several meadows around the Mediterranean Sea and Australia and therefore constitute more comprehensive estimates of the Corg storage potential of P. oceanica and P. australis.
In summary, we conclude that exposed mat escarpments up to 3 m in height in seagrass Posidonia are unusual but of exceptional importance in marine biogeochemical cycles, preluding the largest carbon sinks among seagrasses worldwide. Further surveys are required to identify additional seagrass escarpments along the coasts, and to gain new insights into their ecology and mechanisms of formation.
Author Contributions
Conceived and designed the experiments: OS, PL, and MM; Performed the experiments: OS, PL, LL-M, EB, and MM. Analyzed the data: OS. All authors contributed reagents, materials and analysis tools and wrote the paper.
Funding
This work was supported by the projects ECU Faculty Research Grant Scheme, the ECU Early Career Research Grant Scheme, the CSIRO Flagship Marine and Coastal Carbon Biogeochemical Cluster (Coastal Carbon Cluster), and the project SUMILEN (CTM2013-47728-R, Spanish Ministry of Economy and Competitiveness). LL is funded by The Leverhulme Trust toward an Early Career Fellowship (ECF-2013-530, “Posidonia as environmental archive: long-term ecology and conservation views”).
Conflict of Interest Statement
The authors declare that the research was conducted in the absence of any commercial or financial relationships that could be construed as a potential conflict of interest.
Acknowledgments
Data supporting this study will be available in ACEF Coastal Data Portal (http://acef.tern.org.au/portal/). The authors are grateful to M. Rozaimi, A. Laffrata, J. Bongiovanni and T. De Bettignies for their help in field and/or laboratory tasks. This is a contribution of the Benthic Ecology Group, 2014SGR120.
Supplementary Material
The Supplementary Material for this article can be found online at: http://journal.frontiersin.org/article/10.3389/fmars.2016.00042
References
Belsher, T., Houlgatte, E., and Boudouresque, C. F. (2005). Cartographie de la prairie à Posidonia oceanica et des principaux faciès sédimentaires marins du Parc National de Port-Cros (Var, France, Méditerranée). Sci. Rep. Port Cros Natl. Park 21, 19–28.
Boudouresque, C. F., Bonhomme, D., Astruch, P., Bonhomme, P., Goujard, A., and Thibaut, T. (2014). “Insight into the typology of reef formations of the Mediterranean seagrass Posidonia oceanica,” in Proceedings of the Fifth Mediterranean Symposium on Marine Vegetation, eds C. Bouafif, H. Langar, and A. Ouerghi (Portoroz: RAC/SPA publ.), 58–63.
Boudouresque, C. F., Mayot, N., and Pergent, G. (2006). The outstanding traits of the functioning of the Posidonia oceanica seagrass ecosystem. Biol. Mar. Medit. 13, 109–113
Boudouresque, C. F., and Meinesz, A. (1982). Découverte de l'Herbier de Posidonie. Sci. Rep. Port Cros Natl. Park 4, 79.
Boudouresque, C. F., Meinesz, A., Ballesteros, E., Ben Maiz, N., Boisset, F., Cinelli, F. S., et al. (1990). Livre Rouge “Gérard Vuignier” des Végétaux, Peuplements et Paysages Marins Menacés de Méditerranée. MAP Technical Report Series 43. Athens: UNEP/IUCN/GIS Posidonie. p. 250.
Boudouresque, C. F., Meinesz, A., and Lefevre, J. R. (1985). Cartographie des peuplements benthiques marins de Corse: I. La formation récifale à Posidonia oceanica de Saint Florent. Annales Institut Océanographique N.S. 61, 27–38.
Boudouresque, C. F., Thommeret, J., and Thommeret, Y. (1980). Sur la découverte d'un bioconcrétionnement fossile intercalé dans l'herbier à Posidonia oceanica de la baie de Calvi (Corse). Journées d'Étude de Systématique et Biogéographie méditerranéenne. Monaco: CIESM publ., 139–142.
Bowman, G. M. (1985). Revised radiocarbon oceanic reservoir correction for Southern Australia. Search 16, 164–165.
Campbell, J. E., Lacey, E. A., Decker, R. A., Crooks, S., and Fourqurean, J. W. (2015). Carbon storage in seagrass beds of Abu Dhabi, United Arab Emirates. Estuaries Coasts 38, 242–251. doi: 10.1007/s12237-014-9802-9
Canals, M., and Ballesteros, E. (1997). Production of carbonate particles by phytobenthic communities on the Mallorca-Menorca shelf, northwestern Mediterranean Sea. Deep Sea Res. II 44, 611–629.
Collier, C. J., Lavery, P. S., Ralph, P. J., and Masini, R. J. (2008). Physiological characteristics of the seagrass Posidonia sinuosa along a depth-related gradient of light availability. Mar. Ecol. Prog. Series 353, 65–79. doi: 10.3354/meps07171
Duarte, C. M., and Cebrian, J. (1996). The fate of marine autotrophic production. Limnol. Oceanogr. 41, 1758–1766.
Duarte, C. M., and Chiscano, C. L. (1999). Seagrass biomass and production: a reassessment. Aquat. Bot. 65, 159–174.
Duarte, C. M., Losada, I. J., Hendriks, I. E., Mazarrasa, I., and Marba, N. (2013). The role of coastal plant communities for climate change mitigation and adaptation. Nat. Clim. Change 3, 961–968. doi: 10.1038/nclimate1970
Fourqurean, J. W., Duarte, C. M., Kennedy, H., Marba, N., Holmer, M., Mateo, M. A., et al. (2012). Seagrass ecosystems as a globally significant carbon stock. Nat. Geosci. 5, 505–509. doi: 10.1038/ngeo1477
Gacia, E., Duarte, C. M., and Middelburg, J. J. (2002). Carbon and nutrient deposition in a Mediterranean seagrass (Posidonia oceanica) meadows. Limnol. Oceanogr. 47, 23–32. doi: 10.4319/lo.2002.47.1.0023
Glew, J. R., Smol, J. P., and Last, W. M. (2001). “Sediment core collection and extrusion,” in Tracking Environmental Change Using Lake Sediments, eds W. M. Last and J. P. Smol (Dordrecht: Kluwer Academic Publishers), 73–105.
Gobert, S., Cambridge, M., Velimirov, B., Pergent, G., Lepoint, G., Bouquegneau, J, et al (2006). “Biology of Posidonia,” in Seagrasses: Biology, Ecology and Conservation, eds A. Larkum, R. Orth, and C. Duarte (Amsterdam: Springer Netherlands), 381–408.
Green, E. P., and Short, F. T. (2003). World Atlas of Seagrasses. Berkeley, CA: University of California Press.
Harrison, P. G. (1989). Detrital processing in seagrass systems: a review of factors affecting decay rates, remineralization and detritivory. Aquat. Bot. 23, 263–228.
Hemminga, M., and Duarte, C. M. (2000). Seagrass Ecology. Cambridge, UK: Cambridge University Press.
Klap, V. A., Hemminga, M. A., and Boon, J. J. (2000). Retention of lignin in seagrasses: angiosperms that returned to the sea. Mar. Ecol. Prog. Series 194, 1–11. doi: 10.3354/meps194001
Kuo, J. (1978). Morphology, anatomy and histochemistry of the Australian seagrasses of the genus Posidonia König (Posidoniaceae). I. Leaf blade and leaf sheath of Posidonia australis Hook F. Aquat. Bot. 5, 171–190.
Kuo, J., and Cambridge, M. L. (1978). Morphology, anatomy and histochemistry of the Australian seagrasses of the genus Posidonia König (Posidoniaceae). II Rhizome and root of Posidonia australis Hook. F. Aquat. Bot. 5, 191–206.
Lambeck, K., and Bard, E. (2000). Sea-level change along the French Mediterranean coast for the past 30,000 years. Earth Planet. Sci. Lett. 175, 203–222. doi: 10.1016/S0012-821X(99)00289-7
Lambeck, K., and Nakada, M. (1990). Late Pleistocene and Holocene sea-level change along the Australian coast. Glob. Planet. Change 3, 143–176.
Lavery, P. S., Mateo, M. A., Serrano, O., and Rozaimi, M. (2013). Variability in the carbon storage of seagrass habitats and its implications for global estimates of Blue Carbon ecosystem service. PLoS ONE 8:e73748. doi: 10.1371/journal.pone.0073748
Lo Iacono, C., Mateo, M. A., Gracia, E., Guasch, L., Carbonell, R., Serrano, L., et al. (2008). Very high-resolution seismo-acoustic imaging of seagrass meadows (Mediterranean Sea): implications for carbon sink estimates. Geophys. Res. Lett. 35, 1–5. doi: 10.1029/2008GL034773
López-Merino, L., Serrano, O., Adame, M. F., Mateo, M. A., and Martinez-Cortizas, A. (2015). Glomalin accumulated in seagrass sediments reveals past alterations in soil quality due to land-use change. Glob. Planet. Change 133, 87–95. doi: 10.1016/j.gloplacha.2015.08.004
López-Sáez, J. A., López-Merino, L., Mateo, M. A., Serrano, O., Pérez-Díaz, S., and Serrano, L. (2009). Palaeoecological potential of the marine organic deposits of Posidonia oceanica: a case study in the NE Iberian Peninsula. Palaeogeogr. Palaeoclimatol. Palaeoecol. 271, 215–224. doi: 10.1016/j.palaeo.2008.10.020
Marbà, N., Arias-Ortiz, A., Masqué, P., Kendrick, G. A., Mazarrasa, I., Bastyan, G. R., et al. (2015). Impact of seagrass loss and subsequent revegetation on carbon sequestration and stocks. J. Ecol. 103, 296–302. doi: 10.1111/1365-2745.12370
Mateo, M. A., Cebrián, J., Dunton, K., and Mutchler, T. (2006). “Carbon flux in seagrass ecosystems,” in Seagrass: Biology, Ecology and Conservation, eds A. W. D. Larkum, R. J. Orth, and C. M. Duarte (New York, NY: Springer), 157–191.
Mateo, M. A., Renom, P., Guallar, C., and Garrido, D. (2005). “Posidonia oceanica: un archivo orgánico milenario,” in Evolucion Paleoambiental de los Puertos y Fondeaderos Antiguos en el Mediterraneo Occidental, eds L. De Maria and R. Turcheti (Roma: Rubettino Editore), 219–229.
Mateo, M. A., Romero, J., Pérez, M., Littler, M. M., and Littler, D. S. (1997). Dynamics of millenary organic deposits resulting from the growth of the Mediterranean seagrass Posidonia oceanica. Estuar. Coastal Shelf Sci. 44, 103–110.
Mateo, M. A., Sánchez-Lizaso, J. L., and Romero, J. (2003). Posidonia oceanica ‘banquettes’: a preliminary assessment of the relevance for meadow carbon and nutrients budget. Estuar. Coastal Shelf Sci. 56, 85–90. doi: 10.1016/S0272-7714(02)00123-3
Miyajima, T., Hori, M., Hamaguchi, M., Shimabukuro, H., Adachi, H. Yamano, H., et al. (2015). Geographic variability in organic carbon stock and accumulation rate in sediments of East and Southeast Asian seagrass meadows. Glob. Biogeochem. Cycles 29, 397–415. doi: 10.1002/2014GB004979
Molinier, R., and Picard, J. (1952). Recherches sur les herbiers de Phanerogames marines du littoral mediterranéen francais. Annales de l'Institut Océanographique 27, 157–234.
Paling, E. I., and McComb, A. J. (2000). Autumn biomass, below-ground productivity, rhizome growth at bed edge and nitrogen content in seagrasses from Western Australia. Aquat. Bot. 67, 207–219. doi: 10.1016/s0304-3770(00)00092-9
Pérès, J. M., and Picard, J. (1964). Nouveau manuel de bionomie benthique de la Mediterranee. Recueil des Travaux de la Station Marine d'Endoume 31, 1–37.
Pergent, G., and Pergent-Martini, C. (1990). Some applications of lepidochronological analysis in the seagrass Posidonia oceanica. Bot. Mar. 33, 299–310.
Pergent, G., Rico-Raimondino, V., and Pergent-Martini, C. (1997). Fate of primary production in Posidonia oceanica meadows of the Mediterranean. Aquat. Bot. 59, 307–321.
Picard, J. (1953). Les herbiers de posidonies important facteur de l'élévation des fonds littoraux. Revue Gémorphodynamique 2, 83–84.
Reimer, P. J., Bard, E., Bayliss, A., Beck, J. W., Blackwell, P. G., Bronk Ramsey, C, et al (2013). IntCal13 and MARINE13 radiocarbon age calibration curves 0-50000 years calBP. Radiocarbon 55, 1869–1887. doi: 10.2458/azu_js_rc.55.16947
Ribera, G., Coloreu, M., Rodriguez-Prieto, C., and Ballesteros, E. (1997). Phytobenthic assemblages of Addaia Bay (Menorca, Western Mediterranean): composition and distribution. Bot. Mar. 40, 523–532.
Rozaimi, M., Serrano, O., and Lavery, P. S. (2013). Comparison of carbon stores by two morphologically different seagrasses. J. R. Soc. West. Aust. 96, 81–83.
Rozaimi, M., Lavery, P. S., Serrano, O., and Kyrwood, D. (2016). Long-term carbon storage and its recent loss in an estuarine Posidonia australis meadow (Albany, Western Australia). Estuar. Coast. Shelf Sci. 171, 58–65. doi: 10.1016/j.ecss.2016.01.001
Serrano, O., Davis, G., Lavery, P. S., Duarte, C. M., Martínez-Cortizas, A., Mateo, M. A., et al. (2016). Reconstruction of millennial-scale fluxes of metals in the Australian coastal environment using seagrass archives. Sci. Total Env. 541, 883–894. doi: 10.1016/j.scitotenv.2015.09.017
Serrano, O., Lavery, P. S, Rozaimi, M., and Mateo, M. A. (2014). Influence of water depth on the carbon sequestration capacity of seagrasses. Global Biogeochem. Cycles 28, 950–961. doi: 10.1002/2014GB004872
Serrano, O., Martínez-Cortizas, A., Mateo, M. A., Biester, H., and Bindler, R. (2013). Millennial scale impact on the marine biogeochemical cycle of mercury from early mining on the Iberian Peninsula. Global Biogeochem. Cycles 27, 1–10. doi: 10.1029/2012GB004296
Serrano, O., Mateo, M. A., Renom, P., and Julia, R. (2012). Characterization of soils beneath a Posidonia oceanica meadow. Geoderma 185, 26–36. doi: 10.1016/j.geoderma.2012.03.020
Serrano, O., Ricart, A. M., Lavery, P. S., Mateo, M. A., Arias-Ortiz, A., Masque, P, et al (2015). Key biogeochemical factors affecting soil carbon storage in Posidonia meadows. Biogeosci. Discussions 12, 18913–18944. doi: 10.5194/bgd-12-18913-2015
Siani, G. M., Pateme, M., Amold, M., Bard, E., Metivier, B., Tisnerat, N, et al (2000). Radiocarbon reservoir ages in the Mediterranean Sea and Black Sea. Radiocarbon 42, 271–280.
Squire, P., Joannes-Boyau, R., Scheffers, A. M., Nothdurft, L. D., Hua, Q., Collins, L. B, et al (2013). A marine reservoir correction for the Houtman-Abrolhos archipelago, east Indian Ocean, Western Australia. Radiocarbon 55, 103–114. doi: 10.1017/S0033822200047834
Stephenson, W. J. (2000). Shore platforms: a neglected coastal feature? Prog. Phys. Geogr. 24, 311–327. doi: 10.1177/030913330002400301
Stuiver, M., and Reimer, P. J. (1993). Extended 14C data base and revised CALIB 3.0 14C age calibration. Radiocarbon 35, 215–230.
Keywords: ecosystem services, biogeochemical cycles, blue carbon, Posidonia oceanica, Posidonia australis, Mediterranean Sea, Indian Ocean
Citation: Serrano O, Lavery PS, López-Merino L, Ballesteros E and Mateo MA (2016) Location and Associated Carbon Storage of Erosional Escarpments of Seagrass Posidonia Mats. Front. Mar. Sci. 3:42. doi: 10.3389/fmars.2016.00042
Received: 12 December 2015; Accepted: 16 March 2016;
Published: 31 March 2016.
Edited by:
Paul Snelgrove, Memorial University of Newfoundland, CanadaReviewed by:
Stefano Aliani, National Research Council of Italy - Institute Marine Science, ItalyRaquel Vaquer-Sunyer, Universitat de les Illes Balears, Spain
Copyright © 2016 Serrano, Lavery, López-Merino, Ballesteros and Mateo. This is an open-access article distributed under the terms of the Creative Commons Attribution License (CC BY). The use, distribution or reproduction in other forums is permitted, provided the original author(s) or licensor are credited and that the original publication in this journal is cited, in accordance with accepted academic practice. No use, distribution or reproduction is permitted which does not comply with these terms.
*Correspondence: Oscar Serrano, by5zZXJyYW5vZ3Jhc0BlY3UuZWR1LmF1