Environmental Drivers of Variation in Bleaching Severity of Acropora Species during an Extreme Thermal Anomaly
- 1Marine Biology and Aquaculture, College of Science and Engineering, James Cook University, Townsville, QLD, Australia
- 2ARC Centre of Excellence for Coral Reef Studies, James Cook University, Townsville, QLD, Australia
- 3AIMS@JCU, Australian Institute of Marine Science, Townsville, QLD, Australia
High sea surface temperatures caused global coral bleaching during 2015–2016. During this thermal stress event, we quantified within- and among-species variability in bleaching severity for critical habitat-forming Acropora corals. The objective of this study was to understand the drivers of spatial and species-specific variation in the bleaching susceptibility of these corals, and to evaluate whether bleaching susceptibility under extreme thermal stress was consistent with that observed during less severe bleaching events. We surveyed and mapped Acropora corals at 10 sites (N = 596) around the Lizard Island group on the northern Great Barrier Reef. For each colony, bleaching severity was quantified using a new image analysis technique, and we assessed whether small-scale environmental variables (depth, microhabitat, competition intensity) and species traits (colony morphology, colony size, known symbiont clade association) explained variation in bleaching. Results showed that during severe thermal stress, bleaching of branching corals was linked to microhabitat features, and was more severe at reef edge compared with lagoonal sites. Bleaching severity worsened over a very short time-frame (~1 week), but did not differ systematically with water depth, competition intensity, or colony size. At our study location, within- and among-species variation in bleaching severity was relatively low compared to the level of variation reported in the literature. More broadly, our results indicate that variability in bleaching susceptibility during extreme thermal stress is not consistent with that observed during previous bleaching events that have ranged in severity among globally dispersed sites, with fewer species escaping bleaching during severe thermal stress. In addition, shaded microhabitats can provide a refuge from bleaching which provides further evidence of the importance of topographic complexity for maintaining the biodiversity and ecosystem functioning of coral reefs.
Introduction
Mass coral bleaching in response to increased sea surface temperature is a major threat to the persistence of coral reefs. Analyses of sea surface temperature data indicate that ocean warming has accelerated in recent decades, and that coral reefs are increasingly being exposed to thermal stress (Heron et al., 2016). Since the 1980s, global mass bleaching events have caused large-scale and significant coral loss. For example, in 1998, increased seawater temperatures caused widespread bleaching and coral mortality in most of the world's coral reef regions, with mortality in excess of 90% on some reefs in the central and western Indian Ocean (Spalding and Brown, 2015). Moreover, between June 2014 and April 2016, bleaching was observed throughout the global oceans during what is now considered to be the longest bleaching event on record (Eakin et al., 2016). In the context of bleaching, temperature stress is often measured in “degree heating weeks” (DHW, °C-weeks), a metric which summarizes the duration of time over which temperatures have been above the average temperature of the warmest summer month at each location (e.g., Eakin et al., 2010). The recent thermal stress event caused severe bleaching on the northern section of the Great Barrier Reef in 2016, where approximately one third of reefs experienced levels of heat stress that were up to two-fold higher than those experienced in the 1998 bleaching event in the same region (Hughes et al., 2017). We here investigate whether species susceptibility to bleaching under extreme heat stress is consistent with species susceptibility reported during previous bleaching events.
Different coral species respond differently to environmental stressors, leading to substantial among-species variability in bleaching susceptibility. In general, the literature documents relatively high bleaching severity for branching corals from the genera Stylophora, Acropora, and Pocillopora, and lower bleaching severity for mound-shaped Porites and Diploastrea (e.g., Marshall and Baird, 2000; Loya et al., 2001; van Woesik et al., 2011; Swain et al., 2016). However, bleaching severity is spatially patchy (e.g., Wooldridge and Done, 2004; Penin et al., 2007). For instance, bleaching severity varies between habitats with some studies reporting bleaching to be less severe in shallow compared with deep lagoons (Grimsdich et al., 2010), while others report the opposite trend (Fisk and Done, 1985; Muhando, 1999). Bleaching severity can also vary with depth (e.g., Penin et al., 2007), although some studies have reported no significant variation in bleaching with depth when values were pooled across genera (Bruno et al., 2001). While temperature stress is recognized to be the primary driver of mass-bleaching (Berkelmans et al., 2004; Hughes et al., 2017), there is no strong consensus about additional environmental drivers of spatial variation in bleaching severity. It is likely that a combination of environmental factors (e.g., local light intensity and water flow) and biological factors (including species-specific responses, and local abundances of susceptible vs. tolerant species) influence spatial patterns of bleaching severity.
In addition to among-species variation in bleaching susceptibility, there is often high variation in the bleaching responses of individuals of the same species. For instance, during the 1998 bleaching event, massive Porites were more susceptible to bleaching in the Palm Islands on the central GBR than they were at nearby Magnetic Island (Marshall and Baird, 2000). Similarly, during a bleaching event in the central Pacific, bleaching was observed at some sites but not others for each of several monitored species (Fagerstrom and Rougerie, 1994). Indeed, numerous studies report within-species variation in bleaching severity across different habitats (e.g., Bruno et al., 2001; Aronson et al., 2002; Hardman et al., 2004). There are numerous potential biotic drivers of this within-species variability. First, different types of Symbiodium are more resistant to increased ocean temperature than others (e.g., Thornhill et al., 2006; Jones et al., 2008; Lesser et al., 2010; Howells et al., 2013), and many coral species can associate with more than one type of Symbiodinium (Baker, 2003; Sampayo et al., 2008). Therefore, we assessed whether species that have the capacity to associate with more than one symbiont type show lower bleaching severity, on average, than other species. Second, bleaching severity is influenced by coral colony size. For example, larger colonies experienced more extensive bleaching than smaller colonies of several species during a major Caribbean bleaching event in 2005 (Brandt, 2009). However, other studies have found contrary results with higher bleaching for smaller colonies for some species (Pratchett et al., 2013), or that colony size only influences bleaching prevalence for certain colony morphologies in certain locations (Wagner et al., 2010). Finally, other benthic organisms that compete for space with corals, such as soft corals and macroalgae, contain secondary metabolites that can lead to the expulsion of Symbiodinium (i.e., bleaching, Aceret et al., 1995). Moreover, competition can influence coral fitness more generally (e.g., by growth suppression, see Horwitz et al., 2017), and such effects might act as an additional stressor that increases bleaching severity. To the best of our knowledge, effects of competition on bleaching severity have not previously been investigated in situ.
The topographic complexity of reefs results in high variability in environmental conditions over small spatial scales. For instance, stable and biologically significant temperature variation occurs at small scales (1–2 m, e.g., Gorospe and Karl, 2011), and also at larger between-habitat scales (hundreds of meters, e.g., Lundgren and Hillis-Starr, 2008). Water flow also varies within- and among-habitats (e.g., Fulton and Bellwood, 2005; Hoogenboom and Connolly, 2009). Therefore, spatial variation in abiotic drivers, such as light intensity, water flow, temperature, and turbidity, influences which corals bleach and where (e.g., West and Salm, 2003). Previous studies report different effects of water flow on bleaching severity, with evidence of increased bleaching severity at exposed sites with high wave activity (McClanahan et al., 2007), as well as evidence of reduced bleaching, along with higher survival of bleached corals, under high water flow conditions (Nakamura and van Woesik, 2001; Nakamura and Yamasaki, 2005). Variability in bleaching among different reef habitats is also associated with site-specific turbidity levels (e.g., Williams et al., 2010). However, observed responses range from a negative effect of turbidity whereby suspended particulates are thought to act as an additional stressor that lowers temperature tolerance (Williams et al., 2010; Hongo and Yamano, 2013), to predictions that turbidity may lessen the severity of bleaching in some shallow habitats by reducing light penetration (West and Salm, 2003; Cacciapaglia and van Woesik, 2016).
Methodological issues associated with quantifying bleaching severity in the field might also lead to variation between studies. While observer differences are unlikely to explain variation in bleaching severity between habitats reported in a single study, observers can differ in color sensitivity or in training (e.g., Siebeck et al., 2006). Many observer methods measure bleaching in simple categories (e.g., “pale,” “partially bleached,” and “bleached”), and this categorization can obscure color gradation. To overcome issues associated with categorization of bleaching, some studies estimate the proportion of each coral colony that is healthy vs. bleached (e.g., Obura, 2001), providing a finer resolution of bleaching severity. Despite these advances, however, a recent review highlighted the relatively high measurement uncertainty for bleaching severity, and noted that standardizing measuring protocols would help to increase the precision of bleaching estimates (Swain et al., 2016). To help standardize bleaching measurements, we developed a new quantification of bleaching severity by measuring coral “whiteness” in individually white-balanced images of healthy, pale and bleached corals.
The objective of this study was to understand the drivers of small-scale variation in the bleaching susceptibility of branching corals, and to evaluate whether bleaching susceptibility under extreme thermal stress is consistent with that observed during previous (less severe) bleaching events. We focused on corals from the genus Acropora due to their high abundance on Indo-Pacific reefs, their importance for the structural complexity of reefs, and their variable bleaching severity within- and among-species (e.g., Marshall and Baird, 2000; Loya et al., 2001; Swain et al., 2016). Specifically, we aimed to understand whether and how variation in bleaching severity was associated with depth, spatial location of colonies relative to the reef edge (a measure of exposure to wave energy and general reef habitat), microhabitat, colony size, colony morphology, and the level of competition and the identity of competitors. We also evaluated whether association with multiple symbiont types could explain among-species variation in bleaching severity using data from the Geosymbio database (Franklin et al., 2012). Finally, we compiled literature data on the response of Acropora species during previous thermal stress events, and assessed whether those species that have been consistently reported to be severely bleached in previous studies were also the most severely bleached during the extreme thermal anomaly which occurred on the Great Barrier Reef during the austral summer of 2016.
Materials and Methods
Field Data Collection
Surveys of coral bleaching were conducted at predominantly shallow, lagoonal sites, and at one additional mid-shelf location, within and around the Lizard Island group (northern Great Barrier Reef, 14°40.140S, 145°27.649E) during early March 2016 ~2 weeks after bleaching was first reported at the location. Thermal stress at this location reached ~10 DHW during this bleaching event (Hughes et al., 2017) and in situ temperature loggers (Onset Hobo) measured an average temperature of 30.3°C (range 27.7–33.2°C) at two reef crest sites during February and March 2016. At the time of the surveys, significant bleaching of susceptible coral species had been observed, but mortality was still negligible (widespread bleaching-related mortality was observed on reefs in the region 1 month later, Hoogenboom unpubl. data). Over a period of 8 days, divers conducted in-water surveys at 10 sites where the bleaching status of ~60 Acropora colonies was monitored per site. Colonies were selected haphazardly as divers swam along a depth contour from a randomly selected starting place, making a conscious effort to observe colonies from different reef microhabitats as far as practicable given the topography of each site. The spatial position of each colony was taken using a towed GPS (Garmin eTRex) that was time-synchronized with a dive watch, and the depth of each colony was recorded using a dive computer (Suunto, D4 and Zoop). Each colony was photographed from directly above (as described below), and additional photographs of colony morphology, local reef topography and colony microhabitat, neighboring competitors, and corallite shape were taken to enable measurement of colony size and competition intensity, and to assist species-level identification. We also kept track of the time and date of observations because ongoing heat stress suggested that bleaching severity would continue to increase during and after the observation period. The full dataset, including coral images and spatial positions, is available in Critchell and Hoogenboom (2017).
Measurement of Bleaching Severity (Response Variable)
Individual coral colonies were photographed from directly overhead, without flash, and from as close as practicable, with a Canon G16 digital camera in an underwater housing. Each photograph contained a color reference chart and scale bar. As differing light conditions of each colony did not allow for identical camera settings to be used in each photograph, individual settings based on the highest image quality (pixel count) and lowest sensitivity (ISO) settings were used. Post-processing was conducted using Adobe Photoshop Creative Cloud (2015) software with images transformed into the device-independent CIEL*a*b* color space which measures color based on lightness (L), along a green-red gradient (a), and along a blue-yellow gradient (b). All images were individually white balanced by identifying true black, true white, and 50% gray thresholds in each photograph. Subsequently, four regions of the colony were selected haphazardly from across the surface area of each coral colony, using the color sample tool. Each sampled region was a constant distance from the branch tip (1–2 cm), and avoided the outer margins of the colonies where branches are often oriented in slightly different directions, and can be shaded by upper branches. The color sample tool in the software was set to capture an 11 × 11 pixel sample for each region of the coral surface, and calculated the average color across each 121 pixels region. The four L*a*b* color samples were averaged for each colony, in order to gain a single numerical measurement of color, the divergence of each L*a*b* average value from black was calculated as ΔE (after Riggs, 1997). This method generated a value for each colony within a range of 0–100, with increasing values representing increasingly bleached (nearest to white) colonies. To determine a reference point for the color of healthy (unbleached) corals, the same technique was used to calculate “whiteness” values for Acropora colonies (n = 12) that showed normal colouration, and that were surveyed and photographed during March 2016 at sites around Orpheus Island. These additional colonies included the same species and colony morphologies as observed at Lizard Island.
Drivers of Bleaching Severity (Explanatory Variables)
Image Analysis
Images of each coral colony (N = 596) were analyzed to determine coral colony morphology after Wallace (1999) as either digitate, corymbose, arborescent, tabular, arborescent table, or hispidose/caespitose. Each coral colony was identified to species level based on Wallace et al. (2012) and Wallace (1999) except for 7 colonies for which species identification could not be reliably determined from the photographs (referred to in our dataset as Acropora spp.). We note that many coral species display morphological plasticity and certain pairs of species have overlapping variation in morphology which poses a challenge for species identification. In our study, some colonies within the following species pairs were difficult to distinguish from each other from photos alone and, therefore, species-level differences between these pairs should be interpreted with caution: A. loripes and A. longicyathus, A. nasuta and A. valida, A. humilis and A. gemmifera.
Colony planar surface area was measured for each colony using image analysis in Image J (version 1.51 h, US National Institute for Health). For each colony we measured the longest diameter and the diameter perpendicular to that and calculated planar area based on the geometric formula for the area of an ellipse. The microhabitat of each colony was also assessed from images of the localized reef topography, and was categorized as; “elevated” (where the topography of the reef meant the coral was >~40 cm above the surrounding corals) “open” (where the colony was on flat reef substratum without any obvious shading by competitors), “crevice” (where the colony grew within a crack in the reef matrix), “overhang” (where the colony was shaded by the reef matrix or other colonies), or “sand” (where the colony grew above a sand patch). Competition intensity was measured by dividing each coral into 8 equal segments centered over the mid-point of the colony, and counting the number of these “octants” in which a benthic competitor was within ~5 cm of the focal colony, after Hoogenboom et al. (2011). These data were subsequently categorized as either: “no competitors,” “low” (competitors present in 1–2 of octants), “medium” (competitors present in 3–4 octants), and “high” (competitors present in >4 octants). In addition, we noted whether competitors included soft corals (categorical variable with soft corals present or absent) and macroalgae (categorical variable with macroalgae present or absent). Only 8 of 596 colonies were in competition with macroalgae so this variable was excluded from subsequent analysis.
Spatial Data
For each colony, depth data measured in the field were converted to depth below lowest astronomical tide based on the known tidal height at the time of sampling. The spatial position data for each colony was used to calculate the position of each colony relative to the open ocean. To do this, the position of the reef edge was defined from reef polygons extracted from Google Earth images (Lizard Island, −14.666777S 145.462971E, image date 10/10/2011 accessed 06/02/2017 with eye altitude of 6.9 km; No Name Reef, −14.641968S 145.653061E, image date 15/09/2016 accessed 07/02/2017 with eye altitude of 4.36 km), and were imported into ArcGIS (ESRI, version 10.2). The spatial position of each coral colony and the reef polygons were transformed to GDA 1994 MGA Zone 55 projection to enable measurement of distances in meters with conversion from decimal degrees. The “near” function was used in ArcGIS to calculate the distance (m) of each point (i.e., each coral colony) from the nearest reef edge.
Coral-Symbiodinium Associations
Given the influence of different Symbiodinium on the thermal tolerance of Acropora corals (e.g., Howells et al., 2013), we determined the total number of Symbiodinium clades reported in the GeoSymbio database for the surveyed Acropora species (Franklin et al., 2012). Only records that identified Symbiodinium using denaturing gradient gel electrophoresis profiles of the internal transcribed spacer 2 region of rDNA were included to avoid confounding effects due to the use of different methods of identifying Symbiodinium. Furthermore, only Acropora species for which there were more than three records in the database were included in this analysis.
Reported Bleaching Severity of Acropora during Previous Bleaching Events
To compare the results from our in-water surveys with observations of Acropora bleaching in previous events, we conducted a comprehensive literature search using Web of Science to conduct cited reference searches for Marshall and Baird (2000) and Loya et al. (2001), and an additional keywords search for “Acropora” and “bleaching.” To capture the gray literature we also scanned all papers listed in the online bleaching database ReefBase (1631 records, as of March 2016, ReefBase, 2017) and extracted data from publications that were publically available. Among this set of publications, data were only used if the study reported field observations during a thermal bleaching event (not laboratory experiments), if corals were identified to species level, and if bleaching was quantified in a way that captured gradation in bleaching severity. We excluded papers where bleaching effects were measured as a change in coral cover between different observation periods due to difficultly ascribing changes in abundances solely to bleaching. In total, 57 publications matched our criteria, yielding 527 records of bleaching for 86 Acropora species. We retained species names as reported in the original publications despite some subsequent synonymization of names (e.g., A. wallaceae was synonymized with A. samoensis by Wallace, 1999), and we recorded colony morphologies of species based on Wallace (1999) and Veron (2000).
To standardize bleaching metrics between studies, data extracted from each publication were re-categorized as follows: “none” means no bleaching of that species was reported in that study; “low” means that the study recorded the species to be partially bleached or with <25% of colonies affected; “moderate” means that 26–50% of colonies were bleached or there was partial bleaching with low levels of recorded mortality; “high” means 50–80% of colonies were bleached and/or mortality was observed; “severe” means that more than 80% of colonies of that species were bleached and/or high levels of bleaching-related mortality were recorded. Data are presented as the percentages of records for each species that fell within each of these categories. In both the data from Lizard Island, and the literature data, the measurement of bleaching severity reflects the short- and long-term thermal history of each colony because measurements were made under natural field conditions. The database we have compiled is accessible in the Supplementary Material.
Data Analysis
To identify the strongest predictors of bleaching severity during the extreme thermal anomaly, we used a linear mixed-effects model that included all main effects (day of observation, colony morphology, depth, microhabitat, competition intensity, presence of soft corals, colony size, and distance to open ocean, where the latter captures variation in environmental conditions between reef-edge and lagoon habitats), and a set of specific interaction terms that were established a-priori based on evidence in the literature. Water flow potentially modulates bleaching severity through effects on gas exchange which are, in turn, affected by both colony morphology and colony size (Hoogenboom and Connolly, 2009). Consequently, we included interaction terms between distance from ocean (a proxy for wave exposure and general reef habitat) and colony morphology, and between distance from ocean and colony size. Colony morphology determines how much light impinges on the coral tissue surface, and light intensity also changes with depth (Hoogenboom et al., 2008). Therefore, we considered that different morphologies might bleach differently at different depths and included a depth by morphology interaction. Different coral morphologies use different competition strategies and the outcome of competition can depend on colony size (Jackson, 1979). Therefore, we included competition by colony size and competition by morphology interaction terms. Finally, we considered that different coral morphologies might bleach at different rates and included the interaction between day of observation and morphology. We had no a priori reason to expect that effects on bleaching severity from the day of observation (duration of exposure to thermal stress), or that effects of the presence of soft corals, should depend on any other environmental factor and therefore omitted those interactions. The dataset includes two random effects; “site” (because corals were observed at a random selection of sites at the location) and “species” (because we observed a random subset of the pool of species based on which species were present at each site rather than observing species selected a priori). We used model selection based on a likelihood ratio test to assess whether the mixed-effects model should include random effects for both “site” and “species within site.” All statistical analyses were performed using the R statistical software (R Development Core Team, 2017).
Our assessment of the Acropora community naturally present at each site meant that we were likely to have different numbers of observations of bleaching severity for different species, and a different composition of species at different sites. To determine whether differences in species composition between sites contributed to among-site variation in bleaching severity we categorized sites as either “exposed” (<420 m from reef edge, 4 sites) or “lagoon” (>510 m from reef edge, 6 sites), and calculated community similarity between pairs of sites using the Bray–Curtis index of dissimilarity. The categorization of “exposed” vs. “lagoon” was based on a natural distance division in our data which yielded approximately equal numbers of sites in each category. This community similarity approach was chosen in place of a multivariate species-by-site ordination because the latter technique is not recommended when there are many more variables (species) than samples (sites). A similar approach was used to assess whether the relative frequency of microhabitats differed between exposed and lagoon sites using a χ2 goodness of fit test.
Data describing Symbiodinium association of Acropora were only available for a subset of the species we observed. These sparse data did not permit quantitative analysis and, therefore, we used graphical analysis to assess whether the capacity to host different symbiont types was related to bleaching severity. Finally, hierarchical cluster analysis was used to group coral species based on their bleaching severity during previous bleaching events as reported in the literature. Subsequently, we applied the same species groupings to the species observed at Lizard Island, and assessed whether mean bleaching severity observed at our study site differed systematically among these predetermined species groups.
Results
Bleaching severity values measured using our new method ranged from 42 (least “white”) to 99 (very close to pure white) across the 10 Lizard Island study sites. In contrast, “whiteness” values for unbleached corals at Orpheus Island (photographed at the same time of year, and including the same coral species and morphologies as at Lizard Island) averaged 43 (±s.e. 3.2, range 21–61). Overall, 97% of coral colonies observed at Lizard Island (N = 596) showed whiteness values outside of the range observed for unbleached corals at Orpheus Island, and 71% of colonies had whiteness values >80 (Figure 1).
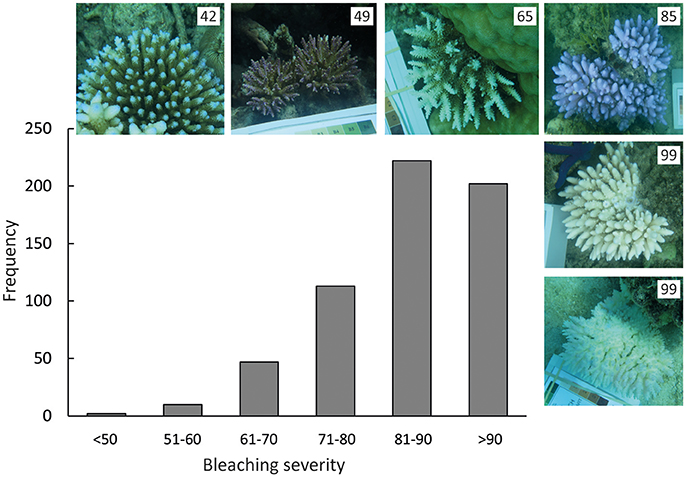
Figure 1. Frequency distribution of bleaching severity measurements for Acropora corals (N = 596) at Lizard Island in March 2016, as measured from white-balanced images of corals in situ. Photos show representatives of coral colonies with different bleaching severity, and numbers in the upper right hand corner of each image show the bleaching severity for each coral.
Among the set of hypothesized correlates of bleaching severity, only day of observation, microhabitat, distance of colonies from the open ocean, and colony morphology explained a significant amount of the variation in bleaching severity. We observed a clear signal of increased bleaching severity over time, despite the relatively short observation period (8 days, Table 1). This temporal variation was equivalent in magnitude to the variation in bleaching severity among microhabitats (average bleaching values were ~79 on day 1 and ~88 on day 8, Figures 2A,C). In addition, hispidose, digitate, and arborescent morphologies were the most severely bleached, whereas tabular morphologies were the least severely bleached (Table 1, Figure 2B). Finally, bleaching severity decreased with distance away from the open ocean, with corals at sites in the lagoon generally showing lower bleaching severity than those at sites close to the reef edge (Table 1, Figure 2D).
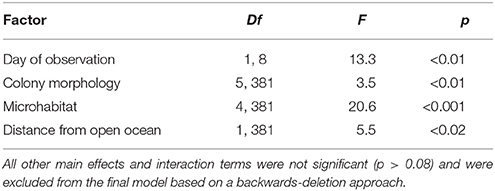
Table 1. Results of general linear mixed effects model of bleaching severity, with site and species included as random effects in the model.
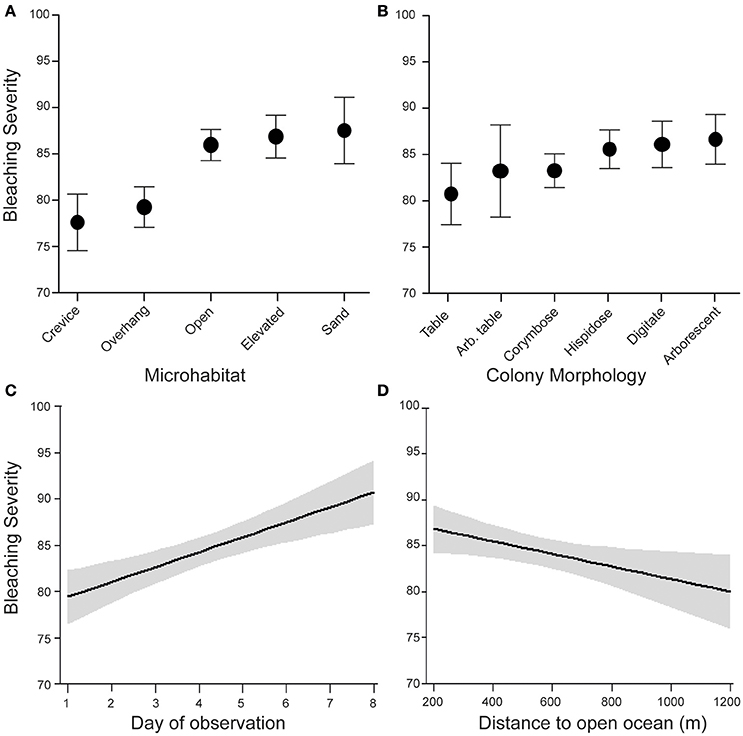
Figure 2. Environmental and morphological correlates of bleaching severity for corals at sites around Lizard Island in March 2016. Data show effects of (A) microhabitat, (B) colony morphology, (C) day of observation and (D) distance to open ocean from linear mixed effects model with N = 596 coral colonies, site included as a random effect, and main effects of the minimal model obtained from backwards deletion of non-significant terms.
Corals growing in crevice and overhang environments showed significantly less severe bleaching than corals in open, elevated, and sand microhabitats (Figure 2, Table 1), supporting the general consensus that bleaching is more severe under conditions of high irradiance. In contrast, depth (range −0.5 to 5 m below LAT) was not significantly associated with bleaching [GLMM, “depth” effect, F(1, 570) = 0.08, p = 0.78]. The relative frequency of different microhabitats occupied by the coral colonies we observed differed between sites that were close to the reef edge and sites that were close to the center of the lagoon (Figure 3). Overall, Acropora colonies were more frequently found in open microhabitats at reef edge sites compared with a higher frequency of elevated and crevice microhabitats at lagoonal sites (Goodness of fit test, χ2 = 19.3, df = 4, p < 0.001). Despite these differences in microhabitat availability, Bray–Curtis similarity of species composition between pairs of sites was approximately equal when reef edge sites were compared with each other (mean dissimilarity 0.54 between 6 pairs of sites), to when lagoonal sites were compared with each other (mean dissimilarity 0.53 between 15 pairs of sites), and to when lagoonal sites were compared with reef edge sites (mean dissimilarity 0.51 between 24 pairs of sites).
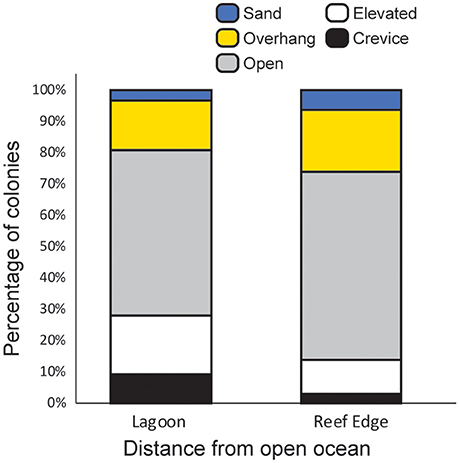
Figure 3. Relative frequency of different microhabitats for Acropora colonies (N = 596) observed at lagoonal sites (>510 m from reef edge, 6 sites) and reef edge sites (<410 m from reef crest, 4 sites).
Corymbose coral species, including A. secale, A. selago, and A. nasuta were among the least severely bleached whereas arborescent species, including A. listeri, A. grandis and A. aspera, were among the most severely bleached (Figures 2B, 4A). Species' mean bleaching severity values ranged from 74 (for A. aculeus) to 95 (for A. carduus) and we observed relatively small within-species variation in bleaching severity with the coefficient of variation of bleaching severity for each species ranging from 1 to 21% (average 10%). However, clear interpretation of among-species variation is hindered by differences in sample sizes; our assessment of the in situ Acropora community meant that we observed only single colonies of some species but >40 colonies of other species (Figure 4A). In addition, formal model selection did not support the inclusion of “species within site” as a random effect in the GLME (likelihood ratio test, model with “species within site” was not superior to a model with only “site” as a random effect, likelihood ratio 0.49, p = 0.48). This result indicates that differences in bleaching intensity among species were generally consistent among sites. Finally, although data documenting symbiont clade diversity for the coral species we observed were too sparse to permit formal analysis, we found no clear indication that species which associated with more than one symbiont clade bleached less severely (Figure 4B).
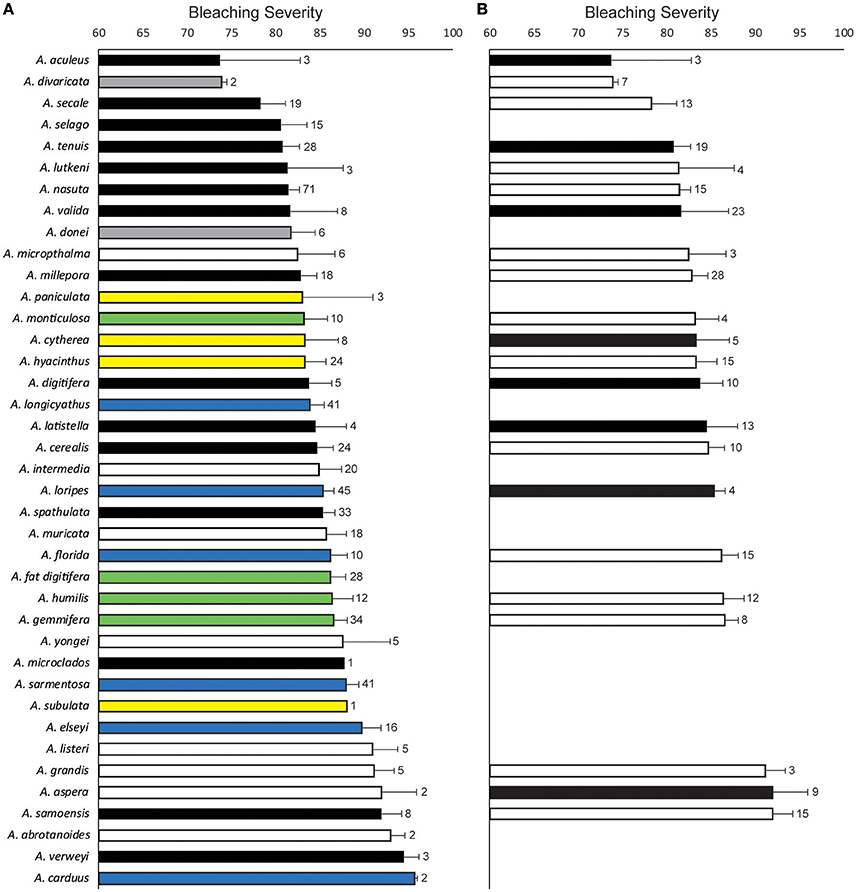
Figure 4. Variation in bleaching severity within- and between-species in the genus Acropora observed at sites around Lizard Island in March 2016. Bars show the mean bleaching severity (colony “whiteness”) measured from white-balanced images of colonies of each species. Error bars show standard error and numbers adjacent to error bars indicate sample sizes. In (A) bars are colored by colony morphology as: black (corymbose), gray (arborescent table), white (arborescent), yellow (table), blue (hispidose), and green (digitate) and numbers indicate colonies observed in the field. In (B) bars are colored by symbiont association as: black (species has been recorded to associate with multiple symbiont types) and white (species has been recorded to only associate with a single symbiont type) and numbers indicate records of Symbiodinium type in the Geosymbio database.
Competition intensity had no effect on bleaching severity [GLME, “competition” effect, F(3, 570) = 2.2, p = 0.09], nor did the presence of soft corals [GLME, “soft corals” effect, F(1, 570) = 0.31, p = 0.58], or the size of the coral colony [GLME, “colony area” effect, F(1, 570) = 0.02, p = 0.90]. We found no evidence that distance from the open ocean, depth, or competition intensity affected bleaching severity differently for different colony morphologies [GLME, “morphology by distance,” F(5, 535) = 1.8, p = 0.12; “morphology by depth,” F(5, 535) = 1.0, p = 0.39; “morphology by competition,” F(15, 535) = 0.9, p = 0.57]. Similarly, the effect of competition intensity on bleaching severity did not depend on colony size [GLME, “competition by colony area,” F(3, 535) = 0.57, p = 0.63], nor did the effect of distance from the open ocean depend on colony size [GLME, “colony area by distance,” F(1, 535) = 1.9, p = 0.16]. Finally, our analysis did not support the hypothesis that different morphologies bleached at different rates [GLME, “day by morphology,” F(5, 535) = 0.63, p = 0.68].
Published records of bleaching severity of Acropora species from previous bleaching events indicate high variability between morphologies (Figure 5), as well as high variability within and among species (Figure 6). Consistent with our observations of Acropora at Lizard Island, the literature demonstrates that arborescent and hispidose Acropora are more frequently observed to be severely bleached, while arborescent tables are among the least severely bleached in both datasets (Figures 2B, 5). However, digitate and tabular morphologies showed contrasting bleaching severity at Lizard Island compared with the literature. When the responses of different coral species are considered, the literature indicates a greater degree of within- and among-species variability in bleaching severity than we observed at Lizard Island, despite having similar number of observations in both cases (N = 596 colonies measured at Lizard Island, N = 532 literature records), and data for a large number of species in both cases (40 species at Lizard Island, 87 species in the literature). In the literature, for the 48 species with at least 5 records of bleaching severity, 27% (13 species) showed high fidelity to a single bleaching category despite inevitable variation in the intensity of thermal stress among locations and bleaching events. In addition, 63% of species had records of bleaching within at least 3 categories, and 23% of species showed the full range of bleaching severity scores (from none to severe, Figure 6). Only one species (A. desalwii) was never recorded to show above “moderate” bleaching, likely due to its restricted geographic distribution and occurrence below 15 m depth (Wallace, 1999). Cluster analysis of the literature data for the subset of Acropora species we observed at Lizard Island revealed 7 clusters based on the bleaching severity categories most often recorded for those species during previous bleaching events (Figure 7). However, we found no evidence of systematic variation in average bleaching scores measured at Lizard Island for these clusters of species (Figure 8).
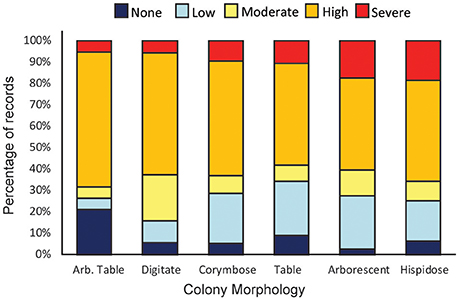
Figure 5. Records of bleaching severity for different Acropora colony morphologies compiled from the literature. Bars show the percentage of records in the literature for each colony morphology in each bleaching severity category (N = 429). Data for elkhorn and encrusting colony morphologies have been excluded to facilitate comparison with data from Lizard Island plotted in Figure 2B. Numbers of records for each morphology are 19 (Arb. Table), 70 (digitate), 95 (corymbose), 64 (table), 149 (Arborescent) and 32 (hispidose/caespitose).
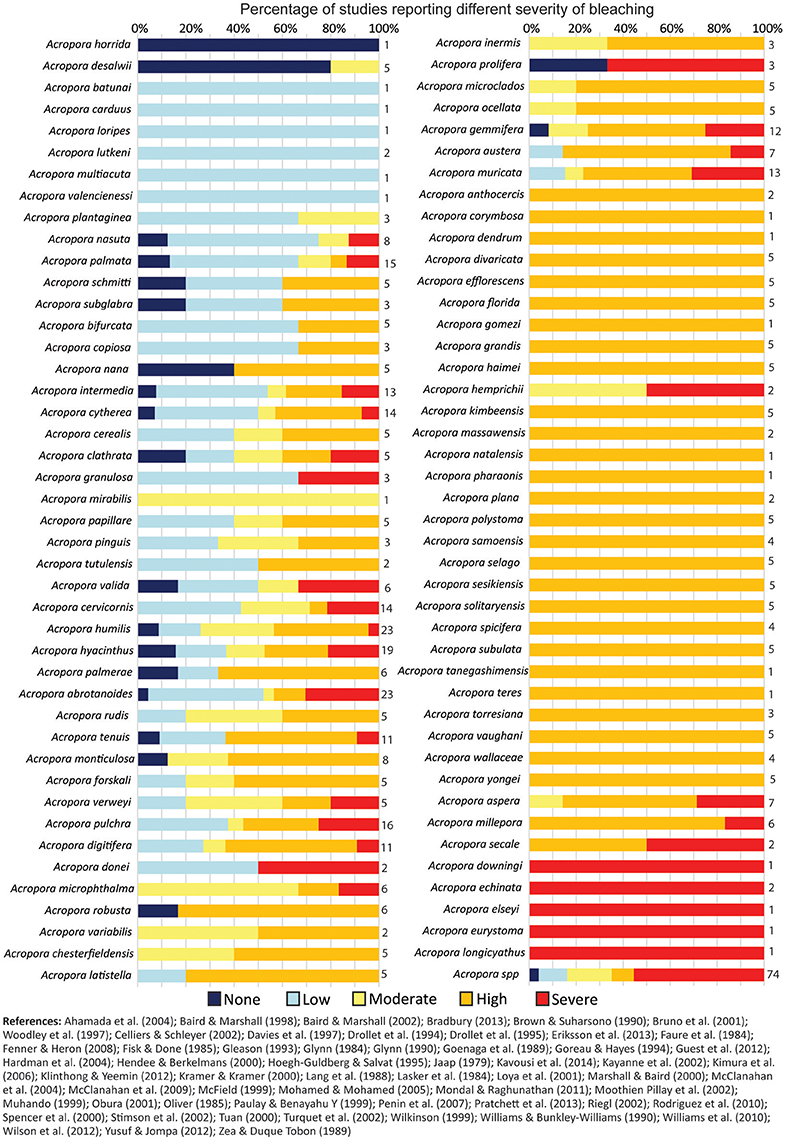
Figure 6. Records of bleaching severity for different Acropora species compiled from the literature. Bars show the percentage of records in the literature for each species in each bleaching severity category (N = 527) and numbers adjacent to bars indicate number of records per species.
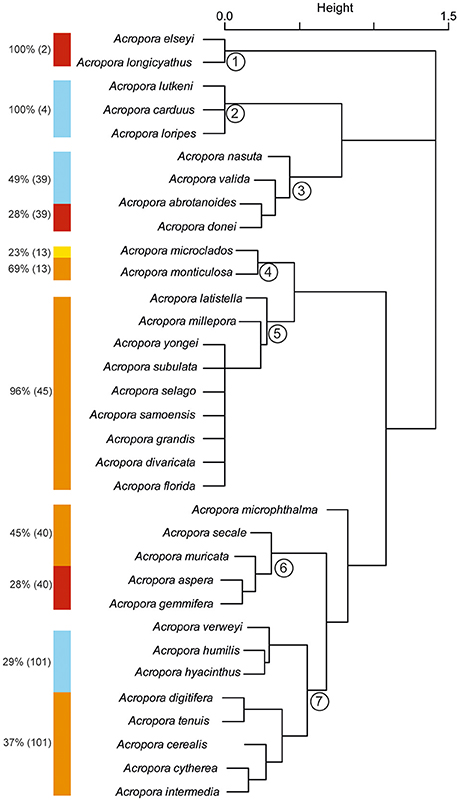
Figure 7. Cluster analysis of Acropora species observed at Lizard Island based on records of occurrence of different bleaching severity in the literature. Color bars adjacent to each cluster show the bleaching severity observed in at least 20% of records for the species (dark blue, no bleaching; pale blue, low bleaching; yellow, moderate bleaching; orange, high bleaching; red, severe bleaching). Percentage values adjacent to color bars show percentage of records in each bleaching category and values in parentheses show number of records.
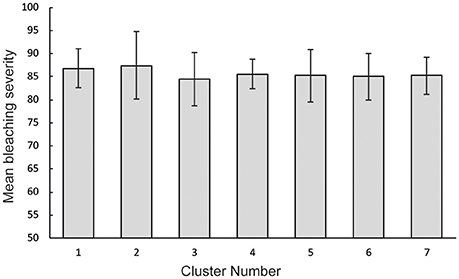
Figure 8. Mean bleaching severity for different groups of Acropora species observed at Lizard Island. Groups were identified using hierarchical cluster analysis and error bars show standard error.
Discussion
The results of this study show that during severe thermal stress, small-scale spatial variation in the bleaching susceptibility of branching corals is linked to microhabitat availability, and the proximity of sites to the open ocean, and that bleaching severity worsens over a very short time-frame (~1 week). We found no evidence that water depth (range −0.5 to 5 m below LAT), competition intensity (range no competition to competitors surrounding entire colony circumference), or colony size (range 5–90 cm diameter) systematically influenced bleaching severity. At our study location, different colony morphologies differed in their bleaching severity under temperature stress, but within- and among-species variation in bleaching severity was low compared with the variation reported in the literature.
Environmental Drivers of Bleaching Severity
Our results generally support the hypothesis that coral bleaching is caused by a combination of high water temperature and high solar radiation (Jokiel and Coles, 1990; Lesser et al., 1990; Brown et al., 1994). Colonies in shaded microhabitats (crevices and overhangs) were less severely bleached than those in microhabitats with higher light exposure (open, elevated, and sand). The structural complexity of reefs causes high variation in irradiance among microhabitats (Brakel, 1979), whereby overhangs and crevices receive ≤40% of the irradiance that reaches open habitats at a similar depth (3–5 m, Anthony and Hoegh-Guldberg, 2003). Consistent with previous studies (e.g., Williams et al., 2010), colonies in sandy patches were the most severely bleached, likely because carbonate sand is highly reflective and amplifies light intensity (Ortiz et al., 2009). While colonies in microhabitats with low irradiance can have low survival (Baird and Hughes, 2000) and growth (Anthony and Hoegh-Guldberg, 2003) under normal conditions, our results support that crevice and overhang habitats may serve as refuges from thermal stress (see also West and Salm, 2003). Consequently, reefs' structural complexity supports ecosystem functioning and biodiversity not only by providing habitat and shelter for mobile reef organisms (Syms and Jones, 2000; Pratchett et al., 2008), but also by providing microhabitats that can increase coral survival during periods of thermal stress. In contrast to the strong effect of microhabitat, competition intensity had no effect on bleaching severity. One explanation for this is that some corals retract their polyps when exposed to high water temperatures (Jones et al., 2000) which might lower the incidence of contact between competitors and/or prevent the release of secondary metabolites. However, other species increase their feeding rates in response to bleaching (Grottoli et al., 2006), which is likely to increase the incidence of tissue contact between adjacent colonies. Further research into species-specific tissue retraction behaviors during thermal stress is required to explain this result.
In our study, water depth did not influence bleaching severity for corals that occur within the upper ~6 m depths of the reef. A likely explanation for this finding is that water temperatures are often similar across this depth range, as the thermocline occurs at depths that are usually well below 20 m on coral reefs (e.g., Grigg, 2006). Moreover, the generally high water clarity at the study sites means there would have been limited attenuation of light over this depth range. Both still water conditions and water clarity increase the penetration depth of solar radiation into seawater, consequently increasing radiant heating throughout the water column and reducing variability in temperature with depth (Glynn, 1993; Brown, 1997). In our dataset, 90% of the surveyed colonies were located at a depth of <2.5 m below LAT (~3–4 m water depth given the tidal range at the location). Based on estimates of light attenuation from other offshore reefs with high water clarity (Cooper et al., 2007), light intensity at this depth would be ~70% of subsurface light intensity. Collectively these results indicate that crevice and overhang microhabitats provide a greater shading effect than light attenuation with depth in clear waters across the surveyed depth range. The absence of a depth effect also demonstrates that abnormally low sea levels were not the cause of coral bleaching at our study location. Although low sea levels due to El Niño Southern Oscillation have been associated with a local coral mortality event in Indonesia (Ampou et al., 2017), a strong depth-dependent pattern of bleaching severity, with higher severity in the shallowest depths (i.e., <1 m depth), would be expected in areas where coral bleaching was caused by tidal emersion.
We used distance from the open ocean as a metric to capture potential spatial variation in wave energy and other environmental conditions between reef-edge and lagoonal sites. Previous studies reveal contrasting effects of water flow on bleaching severity. Thermal bleaching is linked to photoinhibition of photosynthesis (e.g., Jones et al., 2000) and this inhibition can be mitigated by higher water flow (Nakamura et al., 2005). However, contrary to such effects, we found lower bleaching severity in lagoon sites which generally have low wave energy and low flow compared with reef edge locations (Fulton and Bellwood, 2005). This result is consistent with a field study in the Indian Ocean which also found a positive correlation between bleaching intensity and water flow speed (McClanahan et al., 2007). Coral reef lagoons are characterized by shallow water with limited mixing, which facilitates heating until surface waves force cooler waters over the reef crest (Monismith, 2007). Consequently, corals in lagoon environments experience greater variability in their local temperature. Heat stress experiments indicate that corals from habitats with high variability in temperature have lower mortality rates than corals from habitats with moderate thermal variability (Oliver and Palumbi, 2011). While we do not have site-specific temperature data at our survey sites, temperature loggers deployed at the study location indicate that the lagoon had slightly higher and more variable temperatures than the reef edge during December through to March 2016 (reef edge site: average 29.7°C range 27.9–31.7°C; lagoon site: average 30.0°C range 25.6–33.2°C). Overall, our results support the hypothesis that prior exposure to variable temperature regimes can promote thermal tolerance of coral colonies. Nevertheless, the declining bleaching severity with distance from the open ocean might also be related to differences in microhabitat availability across this gradient as we observed a higher frequency of crevice microhabitats, and a lower frequency of open microhabitats, at lagoonal sites.
Among-Species Variation in Bleaching Severity
Bleaching severity differed among the various branching morphologies of Acropora observed at Lizard Island. Previous studies have reported disparate results regarding the effect of colony morphology on bleaching, including: no clear effect of morphology (Williams et al., 2010); higher bleaching susceptibility for branching and tabular corals compared with massive and encrusting colonies (Marshall and Baird, 2000; Loya et al., 2001); and higher bleaching severity of massive corals compared with branching corals (Ortiz et al., 2009). These disparate results might be partially explained by variation in growth rates, both among-species and among-locations due to changes in environmental conditions. Fast-growing branching morphologies are more susceptible to bleaching than morphologies with slower growth rates (e.g., massive corals, Hoegh-Guldberg and Salvat, 1995; Marshall and Baird, 2000; Brandt, 2009). This pattern is thought to be related to metabolic rates: fast-growing colonies have higher metabolic rates and, thus, accumulate more harmful oxygen free radicals which results in oxidative stress that is linked to bleaching susceptibility (e.g., Jokiel and Coles, 1974; Hoegh-Guldberg and Salvat, 1995; Baird and Marshall, 2002). Among Acropora corals specifically, a recent study by Dornelas et al. (2017) showed that digitate and corymbose growth forms have slower growth rates than arborescent and tabular growth forms. These results are broadly consistent with the bleaching severity of these species reported in the literature. However, in our surveys, tabular corals were the least severely bleached despite having rapid growth rates (Dornelas et al., 2017). At present, we do not have a clear explanation for these contrasting results and further studies are required to disentangle the influence of growth rate compared with other environmental variables on coral bleaching susceptibility.
The type of Symbiodinium present within coral tissues can have a significant influence on the bleaching susceptibility of corals (e.g., Glynn, 1993; Baker, 2003; Berkelmans and Van Oppen, 2006; Abrego et al., 2008). In particular, some corals can increase their thermal tolerance if they can change the dominant symbiont clade in their tissues to a more thermally tolerant one (Berkelmans and Van Oppen, 2006). This implies that corals harboring multiple symbiont types potentially have an ecological advantage if they can shuffle their symbionts to “match” their ambient environmental conditions. However, under times of stress, this advantage can only manifest if the symbiont community includes symbionts that are tolerant to a given stressor. Our data showed no clear relation between bleaching severity and the capacity of Acropora species to harbor multiple Symbiodinium types. This result suggests that it is the presence of a specific heat-tolerant symbiont, rather than the ability to host multiple symbiont types, that confers thermal tolerance. We note, however, that while there is an increasing research emphasis on the functional differences between Symbiodinium clades (e.g., Suggett et al., 2015, 2017), the coral species coverage of these data remains relatively sparse and this constrained our analyses. We limited our analysis to the level of Symbiodinium clades, but differences in thermal tolerance exist among Symbiodinium belonging to the same clade (Tchernov et al., 2004; Sampayo et al., 2008; Correa and Baker, 2009; LaJeunesse et al., 2014). Thus, while our results suggest that Acropora species known to associate with one or multiple Symbiodinium clades did not exhibit differences in bleaching resistance, finer-scale resolution of symbiont identities may have explained additional variation in bleaching intensity (Sampayo et al., 2008).
A Standardized Method for Measuring Bleaching Severity
The image analysis technique developed here provides a sensitive measure of bleaching severity that captures gradation within and between species, and that overcomes some of the limitations of survey observation methods (e.g., Siebeck et al., 2006). First, our technique eliminates in situ observer bias and corrects for color variation due to differences in the in situ light environment. Second, the data are continuous which allows a more precise measure of bleaching severity by avoiding the loss of information that occurs with categorical data. Third, photographs provide a permanent photographic record of the state of each individual colony which may be useful for future comparisons. Finally, this technique can be developed further, and extended to other coral groups, by quantifying the “whiteness” of healthy corals to provide a species-specific baseline for coral colony health in the absence of environmental stressors. Despite these advantages, this new technique is more time consuming than in situ observer based techniques. White-balancing and color analysis took ~3–5 min per image, with approximately half of this time spent on white-balancing. In addition, many corals contain fluorescent proteins in their tissues which give colonies a blue or pink colouration that overlays the golden brown color of the Symbiodinium within the coral cells (e.g., Alieva et al., 2008). Our technique likely underestimates bleaching severity of heavily pigmented colonies because these host-pigments make them appear to be less white than a non-pigmented colony with the same level of bleaching (i.e., symbiont loss). However, this issue makes our results conservative as to the differences between morphologies, microhabitats and sampling days because it introduces additional variability in the dataset. We also note that, when colonies are only partially bleached (e.g., where the upper surface of the colony is whiter than the lower surfaces, Harriott, 1985), more than four measurement points may be needed to accurately represent the color distribution of each colony.
Conclusions
During the extreme heat stress that affected the northern GBR in 2016, 97% of Acropora colonies observed at our study location were pale or bleached, and ~70% of colonies had whiteness values consistent with a categorization of “severe” bleaching. In contrast, in previous bleaching events nearly a quarter of Acropora species were reported to show high within-species variability in bleaching severity, with scores ranging from “none” to “severe.” Overall, we consistently observed severe bleaching during the extreme thermal anomaly experienced at our study location, in comparison to more variable bleaching severity reported during a broad range of bleaching events described in the literature. These comparisons highlight the importance of measuring and reporting the magnitude of thermal stress experienced at different sites during bleaching so that species- and/or location-specific temperature thresholds for different levels of bleaching can be quantified. Our results also highlight the importance of monitoring and reporting the timing of bleaching surveys relative to the onset of thermal stress, as our new image analysis technique detected a 10% increase in bleaching severity over a period of 1 week. Microhabitat structure, but not competition intensity, water depth or colony size, also contributed to variation in bleaching severity of Acropora corals. Crevices and overhang microhabitats, which can mitigate bleaching severity, are more prevalent in structurally complex reefs. Such complexity is a product of the successful recruitment and growth of morphologically complex species, such as Acropora species that are important contributors to spatial complexity in Indo-Pacific reefs (Pratchett et al., 2008). Collectively, these results suggest a negative feedback loop whereby bleaching reduces the abundance of branching species, which lowers the occurrence of shaded microhabitats, which then leads to more severe bleaching.
Author Contributions
All authors contributed to the initial conceptualization of this project. Field data were collected by GF, TC, and SJ (at Lizard Island) and by KP, BR, KB, and MH (at Orpheus Island). Color analyses were conducted by GF and AP, and colony size measurements were conducted by MÁ-N and SJ. Coral identification, microhabitat and competition data were compiled by MH, KN, AP, TC, and GF. Spatial analyses were conducted by KC. MH analyzed the data and wrote the first draft of the paper with all authors making a substantial contribution to subsequent drafts (particularly SJ, KP, and MÁ-N).
Conflict of Interest Statement
The authors declare that the research was conducted in the absence of any commercial or financial relationships that could be construed as a potential conflict of interest.
The reviewer AWL and handling Editor declared their shared affiliation.
Acknowledgments
We thank staff from Lizard Island Research Station for assistance with field operations. We also thank J Madin for temperature data. This research was funded by the Australian Research Council to the ARCCOE for Coral Reef Studies CE140100020, and James Cook University.
Supplementary Material
The Supplementary Material for this article can be found online at: https://www.frontiersin.org/articles/10.3389/fmars.2017.00376/full#supplementary-material
References
Abrego, D., Ulstrup, K. E., Willis, B. L., and van Oppen, M. J. (2008). Species–specific interactions between algal endosymbionts and coral hosts define their bleaching response to heat and light stress. Proc. R. Soc. Lond. B Biol. Sci. 275, 2273–2282. doi: 10.1098/rspb.2008.0180
Aceret, T. L., Sammarco, P. W., and Coll, J. C. (1995). Toxic effects of alcyonacean diterpenes on scleractinian corals. J. Mar. Biol. Ecol. 188, 63–78. doi: 10.1016/0022-0981(94)00186-H
Ahamada, S., Bijoux, J., Bigot, L., Cauvin, B., Kooonjul, M., Maharavo, J., et al. (2004). “Status of coral reefs of the South West Indian Ocean Island States,” in Status of Coral Reefs of the World 2004, Vol. 1, ed C. R. Wilkinson (Townsville, QLD: AIMS), 189–212.
Alieva, N. O., Konzen, K. A., Field, S. F., Meleshkevitch, E. A., Hunt, M. E., Beltran-Ramirez, V., et al. (2008). Diversity and evolution of coral fluorescent proteins. PLoS ONE 3:e2680. doi: 10.1371/journal.pone.0002680
Ampou, E. E., Johan, O., Menkes, C. E., Ni-o, F., Birol, F., Ouillon, S., et al. (2017). Coral mortality induced by the 2015-2016 El-Ni-o in Indonesia: the effect of rapid sea level fall. Biogeosciences 14:817. doi: 10.5194/bg-14-817-2017
Anthony, K. R. N., and Hoegh-Guldberg, O. (2003). Variation in coral photosynthesis, respiration and growth characteristics in contrasting light microhabitats: an analogue to plants in the forest gaps and understoreys? Funct. Ecol. 17, 246–259. doi: 10.1046/j.1365-2435.2003.00731.x
Aronson, R. B., Precht, W. F., Toscana, M. A., and Koltes, K. H. (2002). The 1998 bleaching event and its aftermath on a coral reef in Belize. Mar. Biol. 141, 435–447. doi: 10.1007/s00227-002-0842-5
Baird, A. H., and Hughes, T. P. (2000). Competitive dominance by tabular corals: an experimental analysis of recruitment and survival of understorey assemblages. J. Exp. Mar. Biol. Ecol. 251, 117–132. doi: 10.1016/S0022-0981(00)00209-4
Baird, A. H., and Marshall, P. A. (1998). Mass bleaching of corals on the Great Barrier Reef. Coral Reefs 17:376. doi: 10.1007/s003380050142
Baird, A. H., and Marshall, P. A. (2002). Mortality, growth and reproduction in scleractinian corals following bleaching on the Great Barrier Reef. Mar. Ecol. Prog. Ser. 237, 133–141. doi: 10.3354/meps237133
Baker, A. C. (2003). Flexibility and specificity in coral-algal symbiosis: diversity, ecology, and biogeography of Symbiodinium. Annu. Rev. Ecol. Evol. Syst. 34, 661–689. doi: 10.1146/annurev.ecolsys.34.011802.132417
Berkelmans, R., and Van Oppen, M. J. (2006). The role of zooxanthellae in the thermal tolerance of corals: a ‘nugget of hope’ for coral reefs in an era of climate change. Proc. R. Soc. Lond. B Biol. Sci. 273, 2305–2312. doi: 10.1098/rspb.2006.3567
Berkelmans, R., De'ath, G., Kininmonth, S., and Skirving, W. J. (2004). A comparison of the 1998 and 2002 coral bleaching events on the Great Barrier Reef: spatial correlation, patterns, and predictions. Coral Reefs 23, 74–83. doi: 10.1007/s00338-003-0353-y
Bradbury, D. (2013). “Chapter 5: Changes in bleaching susceptibility among corals subject to ocean warming and recurrent bleaching in Moorea, French Polynesia,” in Inter- and Intra- Specific Variation in Bleaching Susceptibility among Scleractinian Corals. Ph.D. thesis, James Cook University.
Brakel, W. H. (1979). Small-scale spatial variation in light available to coral reef benthos: quantum irradiance measurements from a Jamaican Reef. Bull. Mar. Sci. 29, 406–413.
Brandt, M. E. (2009). The effect of species and colony size on the bleaching response of reef-building corals in the Florida Keys during the 2005 mass bleaching event. Coral Reefs 28, 911–924. doi: 10.1007/s00338-009-0548-y
Brown, B. E. (1997). Coral bleaching: causes and consequences. Coral Reefs 16, S129–S138. doi: 10.1007/s003380050249
Brown, B. E., Dunne, R. P., Scoffin, T. P., and Le Tissier, M. D. A. (1994). Solar damage in intertidal corals. Mar. Ecol. Prog. Ser. 105, 219–230. doi: 10.3354/meps105219
Brown, B. E., and Suharsono (1990). Damage and recovery of coral reefs affected by El Nino related seawater warming in the Thousand Islands, Indonesia. Coral Reefs 8, 163–170. doi: 10.1007/BF00265007
Bruno, J. F., Siddon, C. E., Witman, J. D., Colin, P. L., and Toscano, M. A. (2001). El nino related coral bleaching in Palau, Western Caroline Islands. Coral Reefs 20, 127–136. doi: 10.1007/s003380100151
Cacciapaglia, C., and van Woesik, R. (2016). Climate-change refugia: shading reef corals by turbidity. Glob. Chang. Biol. 22, 1145–1154. doi: 10.1111/gcb.13166
Celliers, L., and Schleyer, M. H. (2002). Coral bleaching on high-latitude marginal reefs at Sodwana Bay, South Africa. Mar. Pollut. Bull. 44, 1380–1387. doi: 10.1016/S0025-326X(02)00302-8
Cooper, T. F., Uthicke, S., Humphrey, C., and Fabricius, K. E. (2007). Gradients in water column nutrients, sediment parameters, irradiance and coral reef development in the Whitsunday Region, central Great Barrier Reef. Estuar. Coast. Shelf Sci. 74, 458–470. doi: 10.1016/j.ecss.2007.05.020
Correa, A., and Baker, A. C. (2009). Understanding diversity in coral-algal symbiosis: a cluster-based approach to interpreting fine-scale genetic variation in the genus Symbiodinium. Coral Reefs 28, 81–93. doi: 10.1007/s00338-008-0456-6
Critchell, K., and Hoogenboom, M. O. (2017). Acropora Bleaching Data, Lizard Island 2016. Townsville: James Cook University. doi: 10.4225/28/591abebf0e781
Davies, J. M., Dunne, R. P., and Brown, B. E. (1997). Coral bleaching and elevated sea-water temperature in Milne Bay Province, Papua New Guinea, (1996). Mar. Freshw. Res. 48, 513–516. doi: 10.1071/MF96128
Dornelas, M., Madin, J. S., Baird, A. H., and Connolly, S. R. (2017). Allometric growth in reef-building corals. Proc. R. Soc. B Biol. Sci. 284:20170053. doi: 10.1098/rspb.2017.0053
Drollet, J. H., Faucon, M., and Martin, P. V. M. (1995). Elevated seawater temperature and solar UV-B flux associated with two succesive coral mass bleaching events in Tahiti. Mar. Freshw. Res. 46, 1153–1157. doi: 10.1071/MF9951153
Drollet, J. H., Faucon, M., Maritorena, S., and Martin, P. M. V. (1994). A survey of environmental physico-chemical parameters during a minor coral mass bleaching event in Tahitit in 1993. Austr. J. Mar. Freshw. Res. 45, 1149–1156. doi: 10.1071/MF9941149
Eakin, C. M., Liu, G., Gomez, A. M., De La Cour, J. L., Heron, S. F., Skirving, W. J., et al. (2016). Global coral bleaching 2014-2017 status and an appeal for observations. Reef Encounter 31, 20–26.
Eakin, C. M., Morgan, J. A., Heron, S. F., Smith, T. B., Liu, G., Alvarez-Filip, L., et al. (2010). Carribean corals in crisis: record thermal stress, bleaching and mortality in 2005. PLoS ONE 5:e13969. doi: 10.1371/journal.pone.0013969
Eriksson, H., Wickel, J., and Jamon, A. (2013). Coral bleaching and associated mortality at Mayotte, Western Indian Ocean. Western Indian Ocean J. Mar. Sci. 11, 113–118.
Fagerstrom, J. A., and Rougerie, F. (1994). 1994 Coral bleaching event, Society Islands, French Polynesia. Mar. Pollut. Bull. 29, 34–35. doi: 10.1016/0025-326X(94)90423-5
Faure, G., Guillaume, M., Payri, C., Thomassin, B. A., van Praet, M., and Vasseur, P. (1984). Sure un phenomene remarquable de blanchiment et de mortalite massive des madreporaires dans le complexe recifal de I'ile Mayotte (SW Ocean Indien). CR Acad. Sci. Paris 299, 637–642.
Fenner, D., and Heron, S. F. (2008). “Annual summer bleaching of a multi-species coral community in backreef pools of American Samoa: a window of the future,” Proceedings of the 11th International Coral Reef Symposium (Ft. Lauderdale, FL).
Fisk, D. A., and Done, T. J. (1985). “Taxonomic and bathymetric patterns of bleaching in corals, Myrmidon Reef (Queensland),” in Proceedings of the 5th International Coral Reef Congress (Tahiti).
Franklin, E. C., Stat, M., Pochon, X., Putnam, H. M., and Gates, R. D. (2012). GeoSymbio: a hybrid, cloud-based web application of global geospatial bioinformatics and ecoinformatics for Symbiodinium-host symbioses. Mol. Ecol. Resour. 12, 369–373. doi: 10.1111/j.1755-0998.2011.03081.x
Fulton, C. J., and Bellwood, D. R. (2005). Wave-induced water motion and the functional implications for coral reef fish assemblages. Limnol. Oceanogr. 50, 255–264. doi: 10.4319/lo.2005.50.1.0255
Gleason, M. G. (1993). Effects of disturbance on coral communities: bleaching in Moorea, French Polynesia. Coral Reefs 12, 193–201. doi: 10.1007/BF00334479
Glynn, P. W. (1984). Widespread coral mortality and the 1982-83 El Nino warming event. Environ. Conserv. 11, 133–146. doi: 10.1017/S0376892900013825
Glynn, P. W. (1990). Coral mortality and disturbances to coral reefs in the tropical Eastern Pacific. Elsevier Oceanogr. Ser. 52, 55–126. doi: 10.1016/S0422-9894(08)70033-3
Glynn, P. W. (1993). Coral reef bleaching: ecological perspectives. Coral Reefs 12, 1–17. doi: 10.1007/BF00303779
Goenaga, C., Vincene, V. P., and Armstrong, R. A. (1989). Bleaching induced mortalities in reef corals from La Parguera, Puerto Rico: a precursor of change in the community structure of coral reefs. Caribb. J. Sci. 25, 59–65.
Goreau, T., and Hayes, R. L. (1994). A Survey of Coral Reef Bleaching in the South Central Pacific during 1994. Coral Reef Initiative Report US Department of State, American Samoa, 108–118.
Gorospe, K. D., and Karl, S. A. (2011). Small-scale spatial analysis of in-situ sea temperature throughout a single coral patch reef. J. Mar. Biol. 2011:719580. doi: 10.1155/2011/719580
Grigg, R. W. (2006). Depth limit for reef building corals in the Au'au channel, S. E. Hawaii. Coral Reefs 25, 77–84. doi: 10.1007/s00338-005-0073-6
Grimsdich, G., Mwaura, J. M., Kilonzo, J., and Amiyo, N. (2010). The effects of habitat on coral bleaching responses in Kenya. AMBIO J. Hum. Environ. 39, 295–304. doi: 10.1007/s13280-010-0052-1
Grottoli, A. G., Rodrigues, L. J., and Palardy, J. E. (2006). Heterotrophic plasticity and resilience in bleached corals. Nature 440, 1186–1189. doi: 10.1038/nature04565
Guest, J. R., Baird, A. H., Maynard, J. A., Muttaqin, E., Edwards, A. J., Campbell, S. J., et al. (2012). Contrasting patterns in coral bleaching susceptibility in 2010 suggest an adaptive response to thermal stress. PLoS ONE 7:e33353. doi: 10.1371/journal.pone.0033353
Hardman, E. R., Meunier, M. S., Turner, J. R., Lynch, T. L., Taylor, M., and Klaus, R. (2004). The extent of coral bleaching in Rodrigues, (2002). J. Nat. Hist. 38, 23–24. doi: 10.1080/00222930410001695051
Harriott, V. J. (1985). Mortality rates of scleractinian corals before and during a mass bleaching event. Mar. Ecol. Prog. Ser. 21, 81–88. doi: 10.3354/meps021081
Hendee, J. C., and Berkelmans, R. (2000). “Expert system generated coral bleaching alerts for Myrmidon and Agincourt reefs, Great Barrier Reef, Australia,” in Proceedings of the 9th International Coral Reef Symposium, Vol. 2 (Bali), 1099–1104.
Heron, S. F., Maynard, J. A., van Hooidonk, R., and Eakin, C. M. (2016). Warming trends and bleaching stress of the world's coral reefs 1985-2012. Sci. Rep. 6:38402. doi: 10.1038/srep38402
Hoegh-Guldberg, O., and Salvat, B. (1995). Periodic mass-bleaching and elevated sea temperatures: bleaching of outer reef slope communities in Moorea, French Polynesia. Mar. Ecol. Prog. Ser. 121, 181–190. doi: 10.3354/meps121181
Hongo, C., and Yamano, H. (2013). Species-specific responses of corals to bleaching events on anthropogenically turbid reefs on Okinawa Island, Japan, over a 15-year period (1995-2009). PLoS ONE 8:e60952. doi: 10.1371/journal.pone.0060952
Hoogenboom, M. O., and Connolly, S. R. (2009). Defining fundamental niche dimensions of corals: synergistic effects of colony size, light and flow. Ecology 90, 767–780. doi: 10.1890/07-2010.1
Hoogenboom, M. O., Anthony, K. R. N., and Connolly, S. R. (2008). Interactions between morphological and physiological plasticity optimize energy acquisition in corals. Ecology 89, 1144–1154. doi: 10.1890/07-1272.1
Hoogenboom, M. O., Connolly, S. R., and Anthony, K. R. N. (2011). Biotic and abiotic correlates of tissue quality for common scleractinian corals. Mar. Ecol. Prog. Ser. 438, 119–128. doi: 10.3354/meps09271
Horwitz, R., Hoogenboom, M. O., and Fine, M. (2017). Spatial competition dynamics between reef corals under ocean acidification. Sci. Rep. 7:40288. doi: 10.1038/srep40288
Howells, E. J., Berkelmans, R., van Oppen, M. J. H., Willis, B. L., and Bay, L. K. (2013). Historical thermal regimes define limits to coral acclimatization. Ecology 94, 1078–1088. doi: 10.1890/12-1257.1
Hughes, T. P., Kerry, J. T., Alvarez-Noriega, M., Alvarez-Romero, J., Anderson, K. D., Baird, A. H., et al. (2017). Global warming and recurrent mass bleaching of corals. Nature 543, 373–377. doi: 10.1038/nature21707
Jaap, W. C. (1979). Observation on zooxanthellae expulsion at middle Sambo Reef, Florida Keys. Bull. Mar. Sci. 29, 414–422.
Jackson, J. B. C. (1979). “Morphological Strategies of Sessile Animals,” in Biology and Systematics of Colonial Organisms, eds G. Larwood and B.B. Rosen(London; New York, NY: Academic Press), 499–555.
Jokiel, P. L., and Coles, S. L. (1974). Effects of heated effluent on hermatypic corals at Kale Point, Oahu. Pac. Sci. 28, 1–18.
Jokiel, P. L., and Coles, S. L. (1990). Response of Hawaiian and other indo-Pacific reef corals to elevated temperature. Coral Reefs 8, 155–162. doi: 10.1007/BF00265006
Jones, A. M., Berkelmans, R., van Oppen, M. J. H., Mieog, J. C., and Sinclair, W. (2008). A community change in the algal endosymbionts of a scleractinian coral following a natural bleaching event: field evidence of acclimatization. Proc. R. Soc. B Biol. Sci. 275, 1359–1365. doi: 10.1098/rspb.2008.0069
Jones, R. J., Ward, S., Amri, A. Y., and Hoegh-Guldberg, O. (2000). Changes in quantum efficiency of Photosystem II of symbiotic dinoflagellates of corals after heat stress, and of bleached corals sampled after the 1998 Great Barrier Reef mass bleaching event. Mar. Freshw. Res. 51, 63–71. doi: 10.1071/MF99100
Kavousi, J., Tavakoli-Kolour, P., Mohammadizadeh, M., Bahrami, A., and Barkhordari, A. (2014). Mass coral bleaching in the northern Persian Gulf 2012. Sci. Mar. 78, 397–404. doi: 10.3989/scimar.03914.16A
Kayanne, H., Harii, S., Yoichi, I., and Akimoto, F. (2002). Recovery of coral populations after the 1998 bleaching on Shiraho reef, in the southern Ryukyus, NW Pacific. Mar. Ecol. Prog. Ser. 239, 93–103. doi: 10.3354/meps239093
Kimura, T., Hasegawa, H., Igarashi, T., Inaba, M., Iwao, K., Iwase, F., et al. (2006). “Status of Coral Reefs in Japan,” Proceedings of the 10th International Coral Reef Symposium (Okinawa).
Klinthong, W., and Yeemin, T. (2012). ”Impact of coral bleaching at Mu Koh Similan national park,” in Proceedings of the 12th International Coral Reef Symposium (Cairns, QLD).
Kramer, P. A., and Kramer, P. R. (2000). “Transient and lethal effects of the 1998 coral bleaching event on the Mesoamerican reef system,” in Proceedings of the 9th International Coral Reef Symposium, Vol. 2 (Bali), 23–27.
LaJeunesse, T. C., Wham, D. C., Pettay, D. T., Parkinson, J. E., Keshavmurthy, S., and Chen, C. A. (2014). Ecologically differentiated stress-tolerant endosymbionts in the dinoflagellate genus Symbiodinium (Dinophyceae) Clade D are different species. Phycologia 53, 305–319. doi: 10.2216/13-186.1
Lang, J. C., Wicklund, R. I., and Dill, R. F. (1988). “Depth and habitat-related bleaching of zooxanthellate reef organisms near Lee Stocking Island, Exuma Cays, Bahamas,” Proceedings of the 6th International Coral Reef Symposium (Townsville, QLD).
Lasker, H. R., Peters, E. C., and Coffroth, M. A. (1984). Bleaching of reef coelenterates in the San Blas Islands, Panama. Coral Reefs 3, 183–190. doi: 10.1007/BF00288253
Lesser, M. P., Slattery, M., Stat, M., Ojimi, M., Gates, R. D., and Grottoli, A. (2010). Photoacclimatization by the coral Montastrea cavernosa in the mesophotic zone: light, food, and genetics. Ecology 91, 990–1003. doi: 10.1890/09-0313.1
Lesser, M. P., Stochaj, W. R., Tapley, D. W., and Shick, J. M. (1990). Bleaching in coral reef anthozoans: effects of irradiance, ultraviolet radiation, and temperature on the activities of protective enzymes against active oxygen. Coral Reefs 8, 225–232. doi: 10.1007/BF00265015
Loya, Y., Sakai, K., Yamazato, K., Nakano, Y., Sambali, H., and van Woesik, R. (2001). Coral bleaching: the winners and the losers. Ecol. Lett. 4, 122–131. doi: 10.1046/j.1461-0248.2001.00203.x
Lundgren, I., and Hillis-Starr, Z. (2008). Variation in Acropora palmata bleaching across benthic zones at Buck Island Reef National Monument (St. Croix, USVI) during the 2005 thermal stress event. Bull. Mar. Sci. 83, 441–451.
Marshall, P. A., and Baird, A. H. (2000). Bleaching of corals on the Great Barrier Reef: differential susceptibilities among taxa. Coral Reefs 19, 155–163. doi: 10.1007/s003380000086
McClanahan, T. R., Ateweberhan, M., Muhando, C. A., Maina, J., and Mohammed, M. S. (2007). Effects of climate and seawater temperature variation on coral bleaching and mortality. Ecol. Monogr. 77, 503–525. doi: 10.1890/06-1182.1
McClanahan, T. R., Baird, A. H., Marshall, P. A., and Toscano, M. A. (2004). Comparing bleaching and mortality responses of hard corals between southern Kenya and the Great Barrier Reef, Australia. Mar. Pollut. Bull. 48, 327–335. doi: 10.1016/j.marpolbul.2003.08.024
McClanahan, T. R., Weil, E., Cortes, J., Baird, A. H., and Ateweberhan, M. (2009). “Chapter 8: Consequences of coral bleaching for sessile reef organisms,” in Coral Bleaching: Patterns and Processes, Causes and Consequences, eds M. J. H. van Oppen and J. M. Lough (New York, NY: Springer), 121–138.
McField, M. D. (1999). Coral response during and after mass bleaching in Belize. Bull. Mar. Sci. 64, 155–172.
Mohamed, T., and Mohamed, A. (2005). Some ecological factors affecting coral reef assemblages off Hurghada, Red Sea, Egypt. Egypt. J. Aquat. Res. 31, 133–145. Available online at: http://hdl.handle.net/1834/1302
Mondal, T., and Raghunathan, C. (2011). An observation on the coral bleaching in Andaman Islands. Int. J. Environ. Sci. 1, 37–51.
Monismith, S. G. (2007). Hydrodynamics of coral reefs. Annu. Rev. Fluid Mech. 39, 37–55. doi: 10.1146/annurev.fluid.38.050304.092125
Moothien Pillay, R., Terashima, H., and Kawasaki, H. (2002). The extent and intensity of the 1998 mass bleaching event on the reefs of Mauritius, Indian Ocean. Galaxea 4, 43–52. doi: 10.3755/jcrs.2002.43
Muhando, C. (1999). “Assessment of the extent of damage, socio-economics effects, mitigation and recovery in Tanzania,” in CORDIO Coral Reef Degradation in the Indian Ocean: Status Reports and Project Presentations 1999, SAREC Marine Science Program. Stockholm University, Stockholm.
Nakamura, T., and van Woesik, R. (2001). Water-flow rates and passive diffusion partially explain differential survival of corals during the 1998 bleaching event. Mar. Ecol. Prog. Ser. 212, 301–304. doi: 10.3354/meps212301
Nakamura, T., and Yamasaki, H. (2005). Requirement of water-flow for sustainable growth of Pocilloporid corals during high temperature periods. Mar. Pollut. Bull. 50, 1115–1120. doi: 10.1016/j.marpolbul.2005.06.025
Nakamura, T., van Woesik, R., and Yamasaki, H. (2005). Photoinhibition of photosynthesis is reduced by water flow in the reef-building coral Acropora digitifera. Mar. Ecol. Prog. Ser. 301, 109–118. doi: 10.3354/meps301109
Obura, D. (2001). Can differential bleaching and mortality among coral species offer useful indicators for assessment and management of reefs under stress? Bull. Mar. Sci. 69, 421–442.
Oliver, J. (1985). “Recurrent seasonal bleaching and mortality of corals on the Great Barrier Reef,” in Proceedings of the 5th International Coral Reef Congress, Vol. 4 (Tahiti), 201–206.
Oliver, T. A., and Palumbi, S. R. (2011). Do fluctuating temperature environments elevate coral thermal tolerance? Coral Reefs 30, 429–440. doi: 10.1007/s00338-011-0721-y
Ortiz, J. C., Gomez-Cabrera M del, C., and Hoegh-Guldbert, O. (2009). Effect of colony size and surrounding substrate on corals experiencing a mild bleaching event on Heron Island reef flat (southern Great Barrier Reef, Australia). Coral Reefs 28, 999–1003. doi: 10.1007/s00338-009-0546-0
Paulay, G., and Benayahu, Y. (1999). Patterns and consequences of coral bleaching in Micronesia (Majuro and Guam) in 1992-94. Micronesica 32, 109–124.
Penin, L., Adjeroud, M., Schrimm, M., and Lenihan, H. S. (2007). High spatial variability in coral bleaching around Moorea (French Polynesia): patterns across locations and water depths. C. R. Biol. 330, 171–181. doi: 10.1016/j.crvi.2006.12.003
Pratchett, M. S., McCowan, D., Maynard, J. A., and Heron, S. F. (2013). Changes in bleaching susceptibility among corals subject to ocean warming and recurrent bleaching in Moorea, French Polynesia. PLoS ONE 8:70443. doi: 10.1371/journal.pone.0070443
Pratchett, M. S., Munday, P. L., Wilson, S. K., Graham, N. A. J., Cinner, J. E., Bellwood, D. R., et al. (2008). Effects of climate-induced coral bleaching on coral-reef fishes - Ecological and economic consequences. Oceanogr. Mar. Biol. 46, 251–296. doi: 10.1201/9781420065756.ch,6
R Development Core Team (2017). R: A Language and Environment for Statistical Computing. Vienna: R Foundation for Statistical Computing. Available online at: http://www.R-project.org
ReefBase. (2017). Global Information System for Coral Reefs. Available online at: http://www.reefbase.org
Riegl, B. (2002). Effects of the 1996 and 1998 positive sea-surface temperature anomalies on corals, coral diseases and fish in the Arabian Gulf (Dubai, UAE). Mar. Biol. 140, 29–40. doi: 10.1007/s002270100676
Riggs, B. (1997). Colorimetry and CIE System in Colour Physics for the Industry, 2nd Edn. Edinburgh: R. McDonald Society of Dyers and Colorists.
Rodríguez, S., Cróquer, A., Bone, D., and Bastidas, C. (2010). Severity of the 1998 and 2005 bleaching events in Venezuela, southern Caribbean. Rev. Biol. Trop. 58(Suppl. 3), 189–196.
Sampayo, E. M., Ridgway, T., Bongaerts, P., and Hoegh-Guldberg, O. (2008). Bleaching susceptibility and mortality of corals are determined by fine-scale differences in symbiont type. Proc. Natl. Acad. Sci. U.S.A. 105, 10444–10449. doi: 10.1073/pnas.0708049105
Siebeck, U. E., Marshall, N. J., Kluter, A., and Hoegh-Guldberg, O. (2006). Monitoring coral bleaching using a colour reference card. Coral Reefs 25, 453–460. doi: 10.1007/s00338-006-0123-8
Spalding, M. D., and Brown, B. E. (2015). Warm-water coral reefs and climate change. Science 350, 769–771. doi: 10.1126/science.aad0349
Spencer, T., Teleki, K. A., Bradshaw, C., and Spalding, M. D. (2000). Coral bleaching in the southern Seychelles during the 1997-1998 Indian Ocean warm event. Mar. Pollut. Bull. 40, 569–586. doi: 10.1016/S0025-326X(00)00026-6
Stimson, J., Sakai, K., and Sembali, H. (2002). Interspecific comparison of the symbiotic relationship in corals with high and low rates of bleaching-induced mortality. Coral Reefs 21, 409–421. doi: 10.1007/s00338-002-0264-3
Suggett, D. J., Goyen, S., Evenhuis, C., Szabo, M., Pettay, D. T., Warner, M. E., et al. (2015). Functional diversity of photobiological traits within the genus Symbiodinium appears to be governed by the interaction of cell size with cladal designation. New Phytol. 208, 370–381. doi: 10.1111/nph.13483
Suggett, D. J., Warner, M. E., and Leggatt, W. (2017). Symbiotic dinoflagellate functional diversity mediates coral survival under ecological crisis. Trends Ecol. Evol. 32, 735–745. doi: 10.1016/j.tree.2017.07.013
Swain, T. D., Vega-Perkins, J. B., Oesterich, W. K., Triebold, C., Dubois, E., Henss, J., et al. (2016). Coral bleaching response index: a new tool to standardize and compare susceptibility to thermal bleaching. Glob. Chang. Biol. 22, 2475–2488. doi: 10.1111/gcb.13276
Syms, C., and Jones, G. P. (2000). Disturbance, habitat structure, and the dynamics of a coral-reef fish community. Ecology 81, 2714–2729. doi: 10.1890/0012-9658(2000)081[2714:DHSATD]2.0.CO;2
Tchernov, D., Gorbunov, M. Y., de Vargas, C., Yadav, S. N., Milligan, A. J., Häggblom, M., et al. (2004). Membrane lipids of symbiotic algae are diagnostic of sensitivity to thermal bleaching in corals. Proc. Natl. Acad. Sci. U.S.A. 101, 13531–13535. doi: 10.1073/pnas.0402907101
Thornhill, D. J., LaJeunesse, T. C., Kemp, D. W., Fitt, W. K., and Schmidt, G. W. (2006). Multi-year, seasonal genotypic surveys of coral-algal symbioses reveal prevalent stability or post-bleaching reversion. Mar. Biol. 148, 711–722. doi: 10.1007/s00227-005-0114-2
Tuan, V. S. (2000). “The corals at Con Dao archipelago (South Vietnam): before, during and after the bleaching event in 1998,” in Proceedings of the 9th International Coral Reef Symposium, Vol 2 (Bali), 895–899.
Turquet, J., Bigot L Cambert, H., Conand, C., Garnier, R., Mirault, E., and Quod, J. P. (2002). 2001 “Bleaching on acropora dominated reefs in réunion,” in Coral Degradation in the Indian Ocean: Status Report 2002, eds O. Linden, D. Souter, D. Wilhelmsson, and D. Obura (Kalmar: CORDIO, Department of Biology and Environmental Science, University of Kalmar), 179–184.
van Woesik, R., Sakai, K., Ganase, A., and Loya, Y. (2011). Revisiting the winners and the losers a decade after coral bleaching. Mar. Ecol. Prog. Ser. 434, 67–76. doi: 10.3354/meps09203
Veron, J. E. N. (2000). Corals of the World, Vol 1–3, ed M. Stafford-Smith (Townsville, QLD: Australian Institute of Marine Science), p. 1382.
Wagner, D. E., Kramer, P. A., and Van Woesik, R. (2010). Species composition, habitat, and water quality influence coral bleaching in southern Florida. Mar. Ecol. Prog. Ser. 408, 65–78. doi: 10.3354/meps08584
Wallace, C. (1999). Staghorn Corals of the world: A Revision of the Genus Acropora. Melbourne: CSIRO Publishing.
Wallace, C. C., Done, B., and Muir, P. R. (2012). Revision and catalogue of worldwide staghorn corals Acropora and Isopora (Scleractinia: Acroporidae) in the Museum of Tropical Queensland. Memoirs of the Queensland Museum. Nature 57, 1–255.
West, J. M., and Salm, R. V. (2003). Resistance and resilience to coral bleaching: implications for coral reef conservation and management. Conserv. Biol. 17, 956–967. doi: 10.1046/j.1523-1739.2003.02055.x
Wilkinson, C. (1999). “The 1997-1998 mass bleaching event around the world,” in Status of Coral Reefs of the World: 1998, ed C. R. Wilkinson (Townsville, QLD: Australian Institute of Marine Science), 15–37.
Williams, E. H., and Bunkley-Williams, L. (1990). The world-wide coral reef bleaching cycle and related sources of coral mortality. Atoll Res. Bull. 335, 1–73. doi: 10.5479/si.00775630.335.1
Williams, G. J., Knapp, I. S., Maragos, J. E., and Davy, S. K. (2010). Modelling patterns of coral bleaching at a remote Central Pacific atoll. Mar. Pollut. Bull. 60, 1467–1476. doi: 10.1016/j.marpolbul.2010.05.009
Wilson, J. R., Ardiwijaya, R. L., and Prasetia, R. (2012). A Study of the Impact of the 2010 Coral Bleaching Event on Coral Communities in Wakatobi National part. The Nature Conservancy.
Woodley, J. D., Bone, D., Buchan, K., Bush, P., DeMeyer, K., Garzon-Ferreira, J., et al. (1997). “CARICOMP 1997 studies on Caribbean coral bleaching, 1995-1996,” in Proceedings of the 8th International Coral Reef Symposium, Vol. 1 (Panama: Smithsonian Tropical Research Institute), 673–678.
Wooldridge, S., and Done, T. (2004). Learning to predict large-scale coral bleaching from past events: a Bayesian approach using remotely sensed data, in situ data, and environmental proxies. Coral Reefs 23, 96–108. doi: 10.1007/s00338-003-0361-y
Yusuf, S., and Jompa, J. (2012). “First quantitative assessment of coral bleaching on Indonesian Reefs,” in Proceedings of the 12th International Coral Reef Symposium (Cairns, QLD).
Keywords: spatial refugia, environmental gradients, Symbiodinium, niche construction, thermal performance
Citation: Hoogenboom MO, Frank GE, Chase TJ, Jurriaans S, Álvarez-Noriega M, Peterson K, Critchell K, Berry KLE, Nicolet KJ, Ramsby B and Paley AS (2017) Environmental Drivers of Variation in Bleaching Severity of Acropora Species during an Extreme Thermal Anomaly. Front. Mar. Sci. 4:376. doi: 10.3389/fmars.2017.00376
Received: 27 June 2017; Accepted: 09 November 2017;
Published: 27 November 2017.
Edited by:
David Suggett, University of Technology Sydney, AustraliaReviewed by:
David Obura, Coastal Oceans Research and Development in the Indian Ocean, KenyaAnthony William Larkum, University of Technology Sydney, Australia
Copyright © 2017 Hoogenboom, Frank, Chase, Jurriaans, Álvarez-Noriega, Peterson, Critchell, Berry, Nicolet, Ramsby and Paley. This is an open-access article distributed under the terms of the Creative Commons Attribution License (CC BY). The use, distribution or reproduction in other forums is permitted, provided the original author(s) or licensor are credited and that the original publication in this journal is cited, in accordance with accepted academic practice. No use, distribution or reproduction is permitted which does not comply with these terms.
*Correspondence: Mia O. Hoogenboom, mia.hoogenboom1@jcu.edu.au