- 1College of Science and Engineering, ARC Centre of Excellence for Coral Reef Studies, James Cook University, Townsville, QLD, Australia
- 2Australian Institute of Marine Science and James Cook University, Townsville, QLD, Australia
- 3Australian Institute of Marine Science, Townsville, QLD, Australia
The majority of corals acquire their photo-endosymbiont Symbiodinium from environmental sources anew each generation. Despite the critical role that environmental availability of Symbiodinium plays in the potential for corals to acclimate and adapt to changing environments, little is known about the diversity of free-living Symbiodinium communities and how variation in these communities influences uptake and in hospite communities in juvenile corals. Here we characterize Symbiodinium community diversity in sediment samples collected from eight reefs representing latitudinal and cross-shelf variation in water quality and temperature regimes. Sediment-associated Symbiodinium communities were then compared to in hospite communities acquired by A. tenuis and A. millepora juveniles following 11–145 days of experimental exposure to sediments from each of the reefs. Communities associated with juveniles and sediments differed substantially, with sediments harboring four times more unique OTUs than juveniles (1,125 OTUs vs. 271). Moreover, only 10.6% of these OTUs were shared between juveniles and sediments, indicating selective uptake by acroporid juveniles. The diversity and abundance of Symbiodinium types differed among sediment samples from different temperature and water quality environments. Symbiodinium communities acquired by juveniles also differed among the sediment treatments, despite juveniles having similar parentage. Moreover, Symbiodinium communities displayed different rates of infection, mortality, and photochemical efficiencies, important traits for coral fitness. This study demonstrates that the biogeography of free-living Symbiodinium types found within sediment reservoirs follows patterns along latitudinal and water quality environmental gradients on the Great Barrier Reef. We also demonstrate a bipartite strategy for Symbiodinium uptake by juvenile corals of two horizontally-transmitting acroporid species, whereby uptake is selective within the constraints of environmental availability.
Introduction
Symbiosis between dinoflagellates in the genus Symbiodinium and scleractinian corals is fundamental for the formation, health, and survival of coral reefs. Over 75% of coral species establish this relationship anew each generation through the acquisition of Symbiodinium from environmental sources, a process termed horizontal transmission (Fadlallah, 1983; Baker, 2003). Environmental conditions on tropical reefs, including the Great Barrier Reef (GBR), are changing rapidly, with increases in mean temperatures, pCO2 concentrations, and nutrients of particular concern (Donner, 2009; Lønborg et al., 2016). As climate change and other human impacts continue to devastate reefs worldwide, knowledge of the capacity of corals to expand or change their symbiotic partners to types better suited to changing climate conditions may help predict species and community survival. Equally as important, knowledge of Symbiodinium availability and the environmental conditions that shape the composition of free-living communities is essential for projecting how environmental change will impact symbiont acquisition and coral physiological flexibility.
Environmental pools of Symbiodinium are thought to influence the uptake of Symbiodinium by both larvae and juvenile corals (Adams et al., 2009; Cumbo et al., 2013; Nitschke et al., 2015), although the full extent of free-living Symbiodinium diversity is uncharacterized. Heritability estimates of the Symbiodinium community in acroporid juveniles also suggest that in hospite communities are partly regulated by symbiont availability in the environmental pool and suggest that this association is flexible and adaptable (Quigley et al., 2017a,b). For example, communities in the sediments act as a link between in hospite communities in adult corals, which continually re-seed the environmental pool, and the establishment of symbiont communities in juveniles through re-seeding from the environmental pool (Nitschke et al., 2015), and have been shown to significantly increase acquisition of Symbiodinium in larvae compared to the water column (Adams et al., 2009). However, lack of knowledge of the diversity, distribution and abundance of free-living dinoflagellates is currently limiting our understanding of environmental impacts on the establishment of coral-Symbiodinium symbioses.
Sediments, the water column, coral rubble, and algal/cyanobacterial surfaces all have distinct Symbiodinium communities in the Pacific and Caribbean oceans. Comparative studies of Caribbean and Hawaiian reefs demonstrate that the dominant Symbiodinium clades found in local corals reflect environmental diversity in the respective region, with clades A and B being the most abundant in both the water column and sediments in the Caribbean, and clades A and C being the most abundant on Pacific reefs (Takabayashi et al., 2012). Clade A was also recovered from coral larvae exposed to sediments, and B and C from juveniles exposed to reef waters in Japan (Adams et al., 2009). In addition, larvae infected using sediments sourced from the northern and central GBR contained Symbiodinium C1, C2, and D, supporting the presence of these types in sediments from these regions (Cumbo et al., 2013). Deep sequencing technology has begun to reveal free-living diversity of Symbiodinium (Cunning et al., 2015b), but further studies across diverse habitats are needed to fully elucidate biogeographical patterns in the distribution and abundance of these important symbionts.
Physiological responses of in hospite vs. cultured Symbiodinium differ in response to thermal, irradiance, and nutrient regimes at the clade and type level (Baker, 2003; van Oppen et al., 2009), but there is limited knowledge of whether variation in these environmental factors shapes free-living distributions of this symbiont. There is some evidence from in hospite and cultured studies that temperature regimes may influence local availability of thermally tolerant (clades D and F, Sawall et al., 2014) vs. thermally sensitive Symbiodinium types [A and B, although A1 is thermo-tolerant (e.g., Karim et al., 2015)]. Biogeographic patterns may also follow water quality gradients, as high levels of suspended sediments change light environments and nutrient levels (Storlazzi et al., 2015). Parameters affecting irradiance, such as mud content and Secchi disc depth, correlate well with distribution patterns of in hospite Symbiodinium on the GBR (Cooper et al., 2011) and also with the abundance of clade C in some coral species in the Caribbean (Garren et al., 2006). Nitrogen (N) and phosphorus (P) utilization varies amongst Symbiodinium clades/types during photosynthesis [e.g., greater nitrate uptake by C vs. D (Devlin, 2015; Iluz and Dubinsky, 2015)] and is potentially host-regulated and limiting for Symbiodinium in hospite (Rees, 1991). Inorganic forms are readily available in interstitial waters of carbonate sediments (Entsch et al., 1983) and may therefore represent important nutrients in structuring environmental Symbiodinium populations and communities. Although environmental availability of different Symbiodinium types is essential for establishing symbioses and for the potential formation of more stress-tolerant partnerships, it is unknown if gradients in temperature, light, or nutrients influence the distributions of free-living Symbiodinium.
To estimate the capacity of corals to respond to climate change through the establishment of symbiosis with novel or locally-adapted symbionts, the diversity and abundance of Symbiodinium types within sediment communities were characterized using deep sequencing across latitudinal (temperature) and cross-shelf (water quality) gradients. Aposymbiotic juveniles of Acropora tenuis and A. millepora were exposed to eight sediment treatments, and subsequent in hospite diversity was characterized after 11–145 days of exposure (corresponding to ~2 week to 6 month old juveniles) to determine if the availability of free-living Symbiodinium types impacts uptake during early ontogeny. Finally, the initial infection dynamics and longer term physiological impacts of in hospite Symbiodinium communities on the health and survival of coral juveniles were also determined. We demonstrate that juveniles are highly selective in the Symbiodinium they take up, but are also influenced by the availability of locally abundant types. Symbiont availability and the corresponding variability in symbiont uptake also correlated with variations in infection and survival of juvenile corals.
Materials and Methods
Experimental Design and Sample Collection
Sediment Collections
Sediments were collected from four sites representing an inshore-offshore water-quality gradient in each of the northern and central sectors of the GBR. Samples were collected in October in both 2013 (northern sector) and 2014 (central sector) just prior to the mass coral spawning in each year. Wallace Islets and Wilkie Island (northern sector) and Pandora and Magnetic Island (central sector) are located inshore, where water quality is characterized by relatively high loads of fine particulate matter and nutrients (Figures S1A, S3). Sites at Great Detached and Tydeman reefs (northern sector) and at Rib and Davies reefs (central sector) are offshore and experience fewer terrestrial inputs. As a consequence, they typically have lower exposure to fine particulate matter and nutrient loads. At each site, surface sediments (top 10 cm) were collected at a water depth of 4 m in three replicate 1 L containers. Sediments were maintained in separate bins supplied with 0.1 μM filtered, flow-through seawater under shaded conditions until experimentation (one bin with 3 L of sediment per site in 2013; three replicate bins, each with 3 L of sediment per site in 2014). An additional 3 L of sediment per site at all northern and central reefs were immediately frozen at −20°C for later analysis. In 2013, experiments were run at Orpheus Island Research Station (OIRS), and in 2014, at the National Sea Simulator (Seasim) at the Australian Institute of Marine Sciences (AIMS). Seawater supplied to sediment treatments at OIRS follow ambient temperature conditions on Orpheus Island reefs and was filtered to 0.1 μM (Figure S1B). Seawater supplied to sediment treatments at AIMS was filtered to 0.4 μM and, during the first month of infection, followed a regime representing the average of seawater temperatures at the four central sector reefs for that time of year; seawater temperatures were then maintained at 27.4°C for the grow-out period (Figures S1A,B). In both years, experimental tanks were shaded to ~50 μmol photon illumination. To compare Symbiodinium communities in sediments pre- and post-experimental sediment treatments, an additional liter of sediment was frozen after 145 days of experimental exposure from central sediment locations.
Breeding and Husbandry of Acropora tenuis and Acropora millepora Juveniles
Eggs and sperm from the broadcast spawning corals A. tenuis and A. millepora were isolated from colonies collected from Wilkie Bay and Orpheus Island in 2013, and from Magnetic Island and Trunk Reef in 2014. Spawning, gamete fertilization and larval rearing were performed as described in Quigley et al. (2016) (see Supplementary Methods for further details about spawning and settlement). For the northern sediment experiments, larvae were settled onto autoclaved terracota tiles and then randomly placed into one of four experimental treatments that corresponded to sediments from one of the four northern reefs (one tank per treatment), at 11 days post-fertilization (A. tenuis: n = 6 tiles per tank, providing a total of 4,012 juveniles across the four sediment treatments; A. millepora: n = 2 tiles per tank for the Wilkie sediment treatment, seven for Wallace, four for Great Detached, seven for Tydeman; 903 juveniles overall). For central sediment experiments, juveniles were settled onto plastic six-well plates and roughly evenly distributed among the four tanks (A. tenuis: n = 3–5 plates per tank; A. millepora: 1–2 plates per tank). All surviving juveniles from each treatment were sampled following either 35 days of exposure (days of exposure: d.o.e.) to northern sediments, or over 12 time points between 11 to 145 days of exposure to central sediments, and stored in 100% ethanol at −20°C until DNA extraction (Table S1).
Genotyping Symbiodinium Communities in Sediments and within Juveniles
Filtration and Concentration of Sediments
To concentrate Symbiodinium cells prior to DNA extraction, frozen sediments were thawed, then filtered with a series of Impact Vibratory Sieves (500, 250, 125, and 63 μM stainless steel mesh) using filtered seawater (0.1 μM filtration) and concentrated into 5.5 L of 0.1 μM filtered seawater. To fully detach Symbiodinium cells from sediment grains, the filtration process was repeated five times. All equipment was washed and autoclaved between samples at 124°C and 200 kPa for 20 min (Sabac T63). The 5.5 L of filtrate was concentrated into a pellet (<50 ml) with serial centrifugation at 4°C: 4,500 rcf for 5 min (x2), 10 min (x3), 15 min (x3), 30 min (x1) (Allegra X-15R: Beckman-Coulter). The pellet was stored at −20°C until the time of extraction.
DNA Extraction
Symbiodinium DNA was extracted from three replicate sediment filtrate samples (each 10 g) per site (3 × 9 sites, including Orpheus Island), and from pre- and post-experiment samples for central sediments (3 × 4 sites; total n = 39 sediment samples). DNA was extracted using the Mo Bio Powermax Soil DNA Isolation Kit (Carlsbad, CA) following a modification of the manufacturer's instructions, i.e., including an additional digestion step in a rotating oven with buffer C1 at 65°C and 80 rpm rotation for 30 min. The samples also underwent three, 20-s, bead-beating steps (6.0 m/s) with 1 mm silica spheres (MPBio) on the FastPrep-24 5G (MP Biomedicals). DNA was extracted from 59 and 176 coral juveniles from the northern and central sector sediment studies, respectively, using a SDS digestion and ethanol precipitation protocol (Wilson et al., 2002; see Table S1 for number of juveniles used per treatment). This extraction included 3 × 30 s of agitation at 4.0 m/s with 1 mm silica spheres at the lysis buffer step.
Sequencing and Data Analysis
Next generation amplicon sequencing of the ITS-2 locus, data processing, and bioinformatics were performed as detailed in Quigley et al. (2016, 2017b). Briefly, after quality checks and filtering, a total of 2,188 OTUs (Operational Taxonomic Units) were identified based on a custom Symbiodinium database. This total was reduced to 1,562 OTUs retrieved across all juvenile and sediment samples with further quality-checks (further details in Supplementary Methods). Mapped reads were variance-normalized using the “DESeq2” package (v. 1.6.3, Love et al., 2014b) implemented in R (R Team, 2013). Abundances of Symbiodinium OTUs were compared among treatments using negative binomial generalized linear models using “DESeq2.” Adjusted p-values were derived using the Benjamini-Hochberg Multiple-Inference correction for alpha = 0.05. Differential abundances calculated in “DESeq2” were expressed in multiplicative (log2 fold) terms within or among treatments (Love et al., 2014a).
Seven analyses were performed to compare Symbiodinium communities among the different treatments and samples (temporal sampling design in Table S1) using differential abundance testing implemented in “DESeq2.” Briefly, comparisons included differential abundance testing among sample types (all juveniles vs. all sediment samples), cross-shelf positions (inshore vs. offshore), sectors (northern vs. central sector), and interactions among these factors (type*shore*sector), as well as further comparisons within each of the factors (further details in Supplementary Methods).
Nonmetric multidimensional scaling (NMDS) was performed on variance-normalized OTU abundances using the packages “Phyloseq,” “vegan” and “ellipse” with a Bray-Curtis dissimilarity matrix (Murdoch et al., 2007; McMurdie and Holmes, 2013; Oksanen et al., 2013). NMDS analysis does not assume linear relationships between underlying variables, and distances between samples are indicative of their similarity in OTU diversity and abundance (Ramette, 2007). Permutational multivariate analysis of variance was used to determine if Symbiodinium communities differed significantly between the factors listed in detail above using the “adonis” function in “vegan.” Correlation coefficients of (variance-normalized, averaged) abundances of each of the 1,562 OTUs across the eight treatments for juveniles and eight sites for sediment samples were calculated and visualized using the package “corrplot” (Wei, 2013).
Juvenile Physiological Measures
Time to Infection and Survival
The trait “time to initial infection” was measured as the first day that Symbiodinium cells were visible throughout the whole juvenile (i.e., within the oral disc, tentacles, and polyp column). Survival was defined as the total number of days an individual juvenile was observed alive, which was determined with a stereo dissection microscope at each sampling date (Table S1). To determine the effect of sediment source on the number of days to infection and the number of days surviving, generalized linear mixed models were fit in “lme4” (Bates et al., 2014; further details about model parameterization in Supplementary Methods).
Photosynthetic Measurements
Photophysiological performance of in hospite Symbiodinium was assessed in A. tenuis juveniles with Imaging Pulse Amplitude Modulated (iPAM) fluorometery and its affiliated software (Walz, Effeltrich, Germany). The iPAM allows resolution at 100 μM, thus provides accurate photosynthetic measures of small juvenile corals (Hill and Ulstrup, 2005). Symbiont densities were large enough to obtain detectable measurements at 41 days of exposure (d.o.e). The actinic light was calibrated with an Apogee quantum sensor (Model MQ-200, USA) with the following settings: measuring intensity = 3, saturation pulse intensity = 3, gain = 2. Juveniles were dark adapted for 10 min prior to measurements beginning at 10:00 a.m., and maximum potential quantum yield (ratio of maximum to variable fluorescence: Fv/Fm) was calculated. This measurement is routinely used to determine photosynthetic productivity in studies of plant physiology (Maxwell and Johnson, 2000), reflects the efficiency of PSII (Krause and Weis, 1991), and is a widely accepted indicator of stress in corals (Jones et al., 1999).
To detect differences in Fv/Fm among A. tenuis juveniles exposed to the four central sector sediment treatments, Generalized Additive Mixed Models (GAMMs) were used to account for non-linear trends over time (Wood, 2006) using the package “mgcv” (Wood, 2006, 2008). Penalized regression spline smoothing functions were applied to the interaction between sampling date and site, whilst site itself was also included as a fixed effect. Plate was treated as a random effect and the variance structure was allowed to vary through time using the varIdent weights argument. Temporal autocorrelation from sequential measurements was dealt with using first-order autoregressive correlation structure at the deepest level (plate) (Pinheiro and Bates, 2006). Model selection was performed with AIC and the log-likelihood ratio tests using the “anova” function from the “nlme” package. A log-normal distribution was used, as Fv/Fm values are non-integer values inherently greater than zero. ACF plots and normalized residual plots vs. fitted values conformed to assumptions of no autocorrelation and heterogeneity of variance.
To examine if there were any relationships between Fv/Fm measurements and normalized OTU abundances in A. tenuis juveniles exposed to sediments from central sector sites from day 41 to day 90, nine of the overall most abundant OTUs (OTU2_B1, OTU3_C1, OTU2129_C15, OTU115_C, OTU1_A3, OTU9_A13, OTU4_D1, OTU7_A3, OTU427_C15), which represented the majority of reads retrieved from juveniles from these time points, were selected for correlation analysis of normalized abundances and Fv/Fm values. OTU abundances and Fv/Fm values were averaged across replicate A. tenuis juveniles, thereby providing one Spearman's Rho Correlation coefficient (R2) per OTU per time point per sediment site [R base package “Stats” (R Team, 2013)]. This metric is robust to non-normal bivariate distributions (Becker et al., 1988), as was the case here.
Environmental Covariates
Mud and carbonate content, average sea surface temperatures (SST) from 2000–2006, and 10 water quality measures were used to identify environmental drivers of ex hospite (sediment) Symbiodinium diversity and allow for comparisons with in hospite Symbiodinium diversity (Cooper et al., 2011). Water quality, irradiance and temperature data were collected from datasets generated by AIMS, the GBR Marine Park Authority, and the Department of Primary Industry and Fisheries from 1976 to 2006, and retrieved from eAtlas (Furnas, 2003; Furnas et al., 2005; Brodie et al., 2007; De'ath, 2007; De'ath and Fabricius, 2008). Values for each covariate per site were extracted from interpolated modeled data using the R packages “dismo” (Hijmans et al., 2013) and “raster” (Hijmans and van Etten, 2014), and figures for each were created using the package “mapping” and a custom Queensland spatialPolygons file created by Logan (2016). Mud and carbonate content were categorized as per Maxwell's (1968) scheme for the GBR (full description in Supplementary Materials). Water quality measures consisted of: total dissolved nutrients [dissolved inorganic nitrogen (DIN: NO2, NO3, NH4), total dissolved phosphorus (TDP), total dissolved nitrogen (TDN), particulate phosphorus (PP), particulate nitrogen (PN)] and irradiance (suspended solids (SS), chlorophyll a, secchi depth). Central sediment sites were further characterized by nutrient profiles and particle size distributions (Supplementary Methods).
To address the highly correlated nature of many of the irradiance and water quality measures, each of the 10 measures were z-score standardized using the scale function in base R, and then summed for each site to create a Water Quality Index measure (WQI), as per methods in Cooper et al. (2011). Correlations between environmental covariates (WQI, mud, and carbonate content, SST) and specific Symbiodinium OTUs found in the sediments were based on pre-experimental sediment samples (n = 27 samples in total, i.e., northern sediments = 3 reps × 4 sites, central samples = 3 reps × 5 sites; 1,160 of the 1,562 OTUs). Sixteen types with known taxonomic information (Guiry and Guiry, 2016) of the possible 1160 OTUs from the pre-experimental samples were selected for Generalized Additive Model analysis (GAMS) across seven of the nine clades. GAMS and partial plots were constructed using the packages “mgcv” (Wood, 2000, 2006, 2008) of normalized abundance data generated from the full data set. Non-significant covariates and regression spline smoothing functions (smoothers) were dropped to achieve final models and normalized abundances of OTUs identified to the same type were summed. A non-metric multidimensional scaling plot (NMDS) of the full data set was constructed using the Bray-Curtis distance metric, with the positioning of environmental covariates as vectors created using the packages “vegan,” and “ggplot2” (Wickham, 2009).
Results
Overall Comparison of Symbiodinium Communities between Coral Juveniles and Sediments across All Factors and Time Points
Symbiodinium communities differed significantly between juvenile corals and sediments when data were combined across all samples of sediments, juveniles (both species) and time points (Figures 1, 2, Table 1). Of the 1,562 OTUs that passed the E-value filter, 72% (1,125) were found only in sediment samples, whereas 17.3% (271) of OTUs were unique to juveniles. Only 10.6% (166) were shared between juveniles and sediments (Figure S2C, Table S2). In hospite and ex hospite diversities were only moderately correlated among sites (mean R2 = 0.229 ± 0.04, Figure 3).
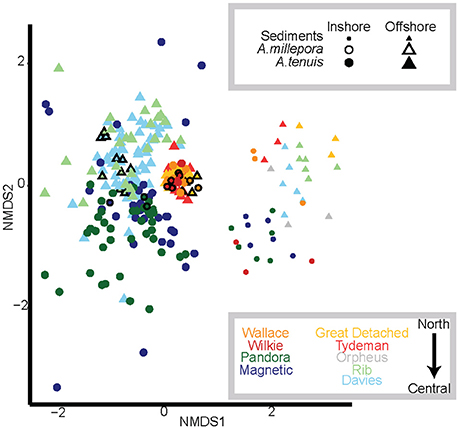
Figure 1. Nonmetric multidimensional scaling (NMDS) of variance-normalized abundances of Symbiodinium OTU's using Bray-Curtis distances. Symbols and their sizes represent: sediment samples (small circles and triangles), A. millepora juveniles (black outlined symbols) and A. tenuis juveniles (no outlines). Colors denote sector and cross shelf origin of sediment samples, where yellow and red denote northern sediments and juveniles exposed to them; blue, green, and gray colors denote central sediments and juveniles exposed to them. Darker shades represent inshore reef sediments and treatments (Wallace, Wilkie, Pandora, Magnetic); lighter shades represent offshore reef sediments and treatments (Great Detached, Tydeman, Orpheus, Rib, Davies).
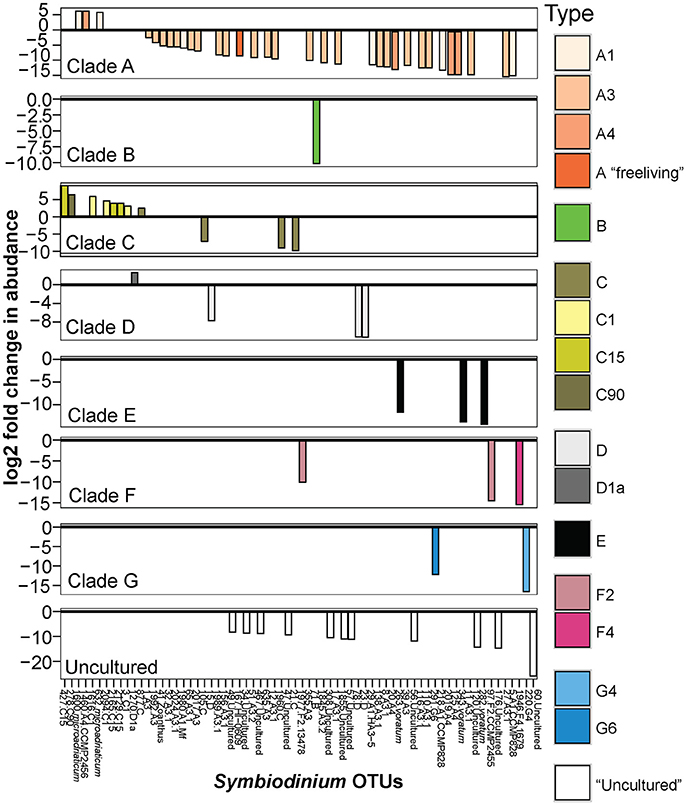
Figure 2. Significant log2 fold changes in normalized abundances of Symbiodinium OTUs in sediment-exposed juveniles compared to sediment samples. Colors represent the classification of different Symbiodinium types. Positive values above the y = 0 line (in bold and black) denote Symbiodinium OTUs found in significantly greater abundances in juveniles compared to sediments. Note the different scales on the y-axis.
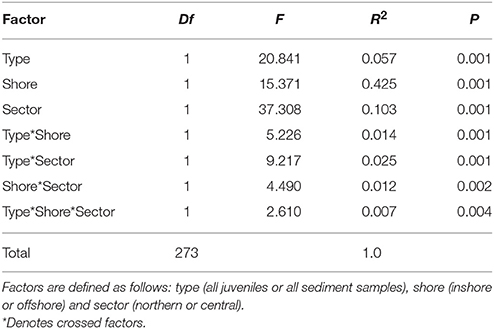
Table 1. Summary of Permutational Multivariate Analysis of Variance tests to compare Symbiodinium community composition among juveniles and sediments.
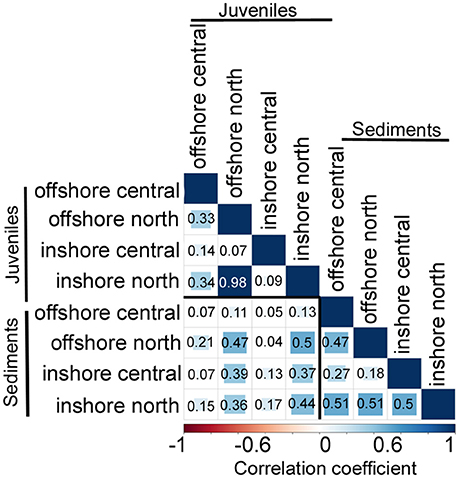
Figure 3. Matrix of pairwise correlation coefficients between Symbiodinium communities associated with juveniles vs. in the sediments across latitudes (northern vs. central GBR) and water quality gradients (inshore vs. offshore). The scale bar represents coefficients from −1 in red (100% negatively correlated) to 1 in dark blue (100% positively correlated).
OTUs shared between juveniles and sediments belonged predominantly to clades A and C (39 and 27 OTUs, respectively). Symbiodinium in clades D–H were also present in both sample types, but at lower diversities (<40 OTUs in total) (Figure S2). Overall, within-clade diversity was much lower in juveniles (<100 OTUs) than in sediments (<300 OTUs). The abundance of 69 OTUs differed significantly between sediment and juvenile samples and included types from clades A–G (Figures 2, B-H adjusted p-values, log2 fold adjusted p-values according to Benjamini-Hochberg (B-H) multiple-inference correction for alpha < 0.05). In general, juveniles had up to 2.3 times greater abundances of an A4 and an A1 species (S. microadriaticum), as well as of multiple types belonging to C1, C15, C90, and D1a, compared to the sediments (Figure 2). In contrast, the sediments had greater abundances of types within clades B, E, F, G and “uncultured” Symbiodinium that have not yet been identified taxonomically. The sediments also held a larger abundance of diverse types within clade A, including free-living types from A1, A3, and A4.
Distribution and Abundance of Symbiodinium Communities within Sediments
Overall Patterns in Sediment Communities across All Locations
Clades A and C were the most abundant Symbiodinium clades in the sediments (A: 19.5% of total sediment reads, 177 of 1291 OTU sediment diversity, C: 11.5%, 148 of 1291, respectively), particularly a free-living type from clade A (OTU_10). A majority of the unique OTU diversity retrieved from sediment samples was identified as “uncultured” Symbiodinium (i.e., novel Symbiodinium without taxonomic descriptions, >300 OTUs), which represented 82% of all uncultured Symbiodinium OTUs across the whole dataset (i.e., sediment and juvenile samples combined; Figure S2). Approximately 100 unique OTUs were represented from each of clades A, C, D, E, and F, along with a smaller number from clades B, I, and G (Figure S2). Greater than 91% of all clade E OTUs were retrieved exclusively from the sediments. Ex hospite Symbiodinium community diversity in sediment samples was only moderately correlated among sites (mean R2 = 0.41 ± 0.06, Figure 3, Supplementary Results), mostly due to strong differences in diversity across the inshore to offshore gradient.
Inshore vs. Offshore Sediment Community Comparisons
Symbiodinium communities in the sediments differed significantly between inshore and offshore locations (p = 0.001, Table 1; Figure 1). In total, the abundances of 58 OTUs differed between inshore and offshore sediments (Figure 4). Inshore sediments had up to 3.3 times greater abundances of D1a, D1, a B type, and F3 compared to offshore sediments (B-H adjusted p-values < 0.05, Figure 4). Offshore locations had up to 3.6 times greater abundances of types from clades A (including A1, A3, A4), S. voratum (clade E), and F2.
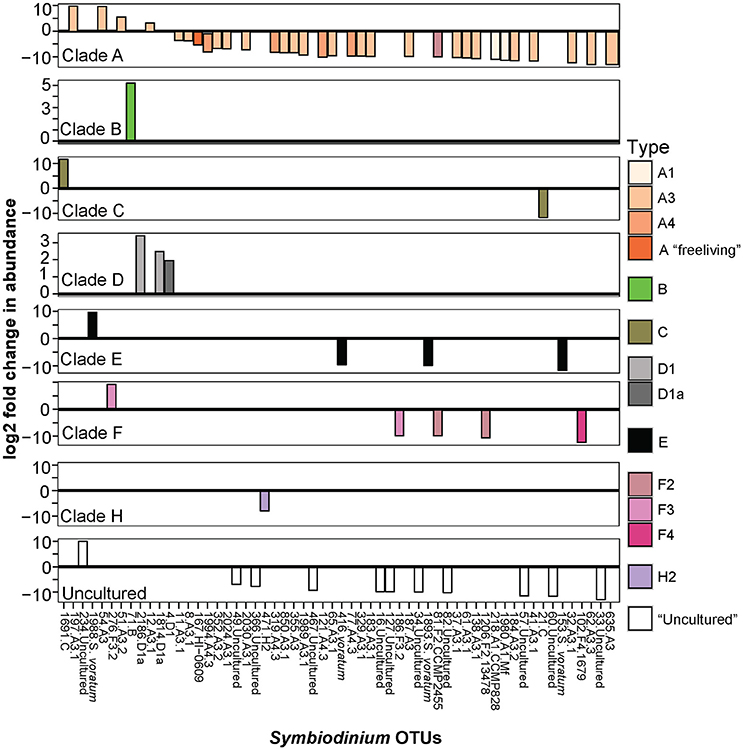
Figure 4. Significant log2 fold changes in normalized abundances of Symbiodinium OTUs in inshore compared to offshore sediments. Positive values above the y = 0 line (in bold and black) denote Symbiodinium OTUs found in significantly greater abundances in inshore sediments. Note different scales on the y-axis.
Northern vs. Central Sector Sediment Community Comparisons
Symbiodinium communities within northern sector sediments also differed significantly from those detected within central sector sediments (p = 0.001, Table 1; Figure 1). The abundances of 47 OTUs differed significantly between northern and central sector sediments (Figure 5). Northern sediments had up to 3.6 times greater abundances of 10 different A-types, including A1, A3, A13, and up to 3.1 times greater abundances of C and C1 types, which were not found in sediments from central reefs (B-H adjusted p-values < 0.05, Figure 5). There were also up to 3.6 times higher abundances of F3, F5, G6, and S. voratum in northern sediments. Central sediments had up to 2.6 times greater abundances of a B type and a new A type (Oku17), as well as 3.1 times greater abundances of cultured A Symbiodinium (HI-0609).
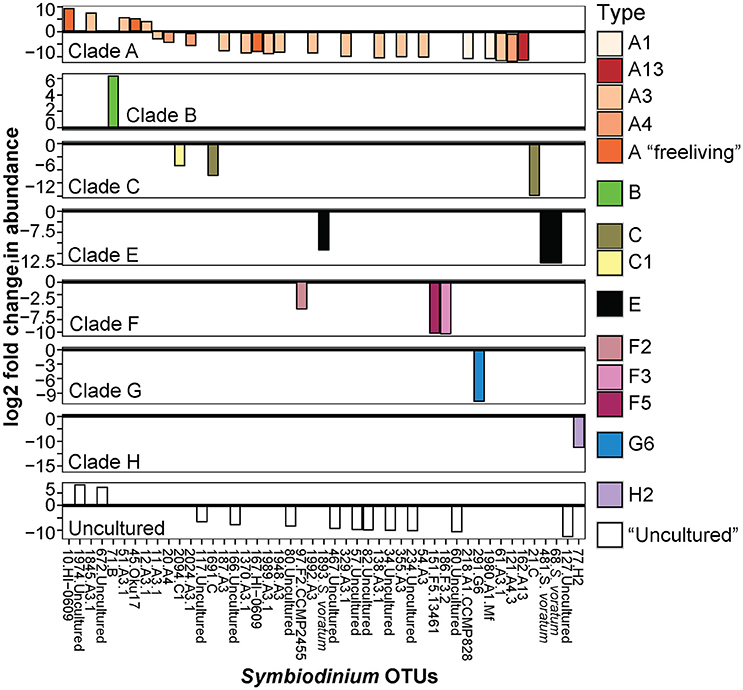
Figure 5. Significant log2 fold changes in normalized abundances of Symbiodinium OTUs in central compared to northern sector sediments. Positive values above the y = 0 line (in bold and black) denote Symbiodinium OTUs found in significantly greater abundances in central sediments.
Distribution and Abundance of Symbiodinium Communities within Juveniles (27–41 d.o.e.)
Symbiodinium Communities in Juveniles Compared among Sediment Treatments
Of the OTUs unique to juveniles, clade C had the greatest type diversity (99 OTUs), although each type was relatively rare in abundance, thus C types comprised only 9.8% of juvenile reads overall. In terms of abundance, unique juvenile OTUs belonged predominantly to B1, with this type representing 79.5% of all reads unique to juveniles (Figure S2). As found for sediments, in hospite Symbiodinium community diversity was only moderately correlated among juveniles exposed to sediments from the different sites (mean R2 = 0.33 ± 0.14, Figure 3, Supplementary Results). In addition, patterns in community composition for juveniles exposed to sediments from inshore vs. offshore sites differed between sectors (p = 0.004, Table 1).
Inshore vs. Offshore Sediment Treatments
The early Symbiodinium community in juveniles differed significantly when juveniles were exposed to inshore vs. offshore sediments (p = 0.001, Table 1; Figure 1). The abundances of 12 OTUs differed significantly in juveniles exposed to inshore vs. offshore sediments (Figure 6A). Juveniles exposed to inshore sediments were dominated by B1 and had up to 3.2 times greater abundances of D1, D1a, and A4 compared to juveniles exposed to offshore sediments (B-H adjusted p-values < 0.05). Juveniles exposed to offshore sediments were dominated by A3, D1, and C15, and had up to 2.9 times greater abundances of A3 and C15 types compared to juveniles exposed to inshore sediments (B-H adjusted p-values < 0.05).
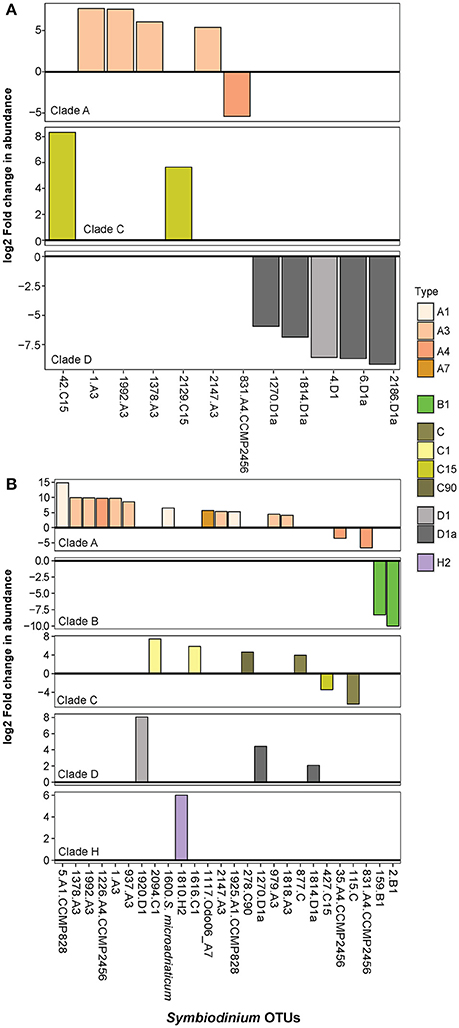
Figure 6. Significant log2 fold changes in normalized abundances of Symbiodinium OTUs in juveniles exposed to: (A) inshore vs. offshore sediments, and (B) central vs. northern sector sediments. OTUs above the y = 0 line (in bold and black) are Symbiodinium OTUs that were found in significantly greater abundances in: (A) offshore than in inshore sediments, and (B) in northern sector sediments than central sediments.
Northern vs. Central Sector Sediment Treatments
The early Symbiodinium community in juveniles exposed to northern vs. central sector sediments differed significantly in the abundances of 26 OTUs (p = 0.001, Table 1; Figure 6B). Juveniles exposed to northern sediments were dominated by A3 and D1 and had up to 3.9 times greater abundances of A1, A3, A7, and A4, D1a, D1, C1, C90, and C (B-H adjusted p-values < 0.05). Juveniles exposed to sediments from the central sector were dominated by B1, C15, C1, and C-types, and had up to 3.3 times more B1, C, C15, and A4 compared to juveniles exposed to northern sediments.
Temporal Variation in Symbiodinium Communities among Juveniles Exposed to Central Sector Sediments
Symbiodinium communities in A. tenuis juveniles exposed to central sector sediments differed significantly over time (permutational multivariate analysis of variance: Df 6,140, F = 2.71, R2 = 0.09, p = 0.001), by reef (Df 3,140, F = 7.86, R2 = 0.13, p = 0.001), and over time in a reef-dependent manner (reef*time point, Df 18,140, F = 1.24, R2 = 0.13, p = 0.017). At the earliest time point sampled (day 11), juveniles exposed to sediments were all dominated by A3.2 (Davies, Magnetic, and Rib sediments) and A13 (Pandora) (Figure 7). Subsequently, types C1, D1, C, and A3 varied in abundance by site and over time (B-H adjusted p-values < 0.05, Table S3). In particular, large community shifts were seen 8 days later (day 19) at each site. Communities in juveniles exposed to central offshore reef (Davies and Rib) sediments closely resembled each other, whereas communities in juveniles exposed to central inshore reef (Magnetic and Pandora) sediments remained distinct. At this second time point, the dominant types (A3.2 or A13) were replaced by B1 (Pandora sediment treatment), D1 (Magnetic), C15 (Davies), and C15/A3 (Rib). By the fifth time point (day 48), Symbiodinium communities in juveniles exposed to offshore sediments were beginning to resemble each other, particularly in their abundances of C15, A3, and C1. In contrast, it wasn't until day 75 that Symbiodinium communities in juveniles exposed to inshore sediments started to resemble each other, most notably in their abundances of B1, C1, and D1. After ~3 months of exposure to sediments (day 90), the abundances of C1 and C15 had increased to become dominant in juveniles exposed to sediments from all sites, following steady increases between days 41 and 90 (Figures 7B–H adjusted p-values < 0.05, Table S3).
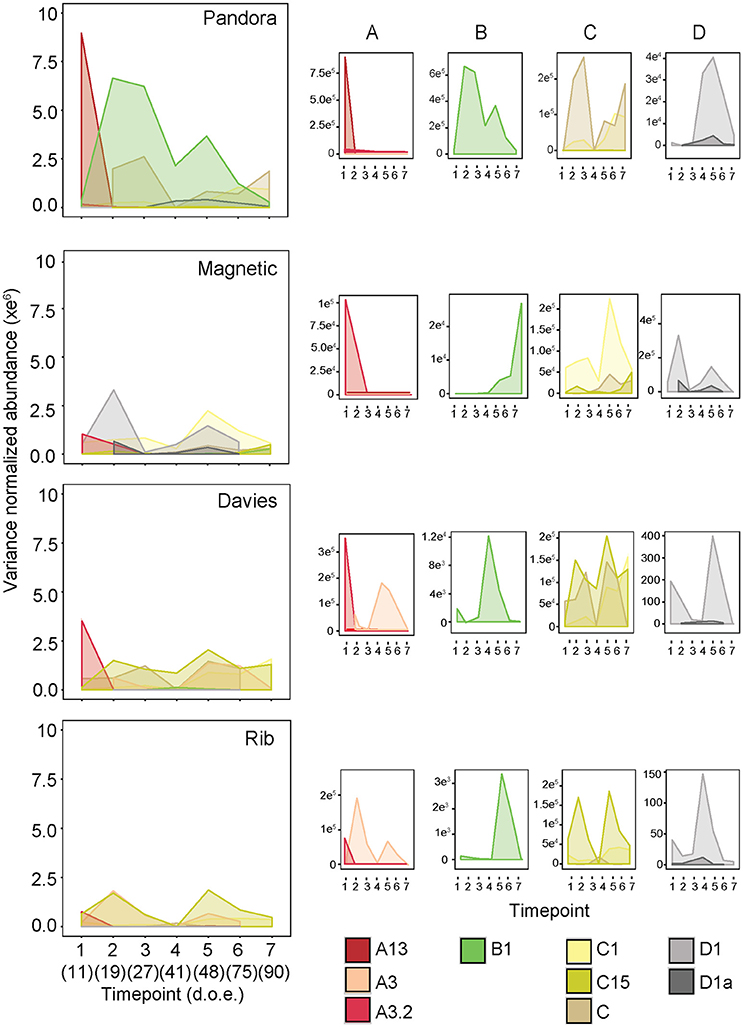
Figure 7. Population dynamics of key Symbiodinium types in A. tenuis juveniles exposed to central sector sediments. Abundances of Symbiodinium types were variance normalized. Analyses were restricted to seven time points between day 11 and day 90 of sediment exposure (d.o.e.). The larger panels are all to the same scale, whilst the smaller panels (A–D) for each site have y-axes that are scaled to best show abundance dynamics for each Symbiodinium type. Colors represent nine key Symbiodinium types, whose abundances differed significantly among time points, as well as among sites.
Time to Infection and Survival of Juveniles Exposed to Central Sector Sediments
Infection was significantly more rapid in juveniles exposed to offshore sediments (19.1 days ± 0.09) than in juveniles exposed to inshore sediments (24.3 days ± 2.13) (negative binomial generalized linear mixed model: p = 0.014), with no effect of location (p = 0.45; Figures 8A,D). The cross-shelf (shore) effect was predominantly driven by significantly lower mean times to infection for juveniles exposed to offshore Rib and Davies sediments (19 ± 0 days and 19.3 ± 0.15 days, respectively) compared to inshore Pandora sediments (26.2 days ± 4.37, p = 0.0145). Juveniles exposed to inshore Magnetic sediments had intermediate times to infection (22.54 days ± 1.06) that were not significant once the fixed effect of gradient was dropped.
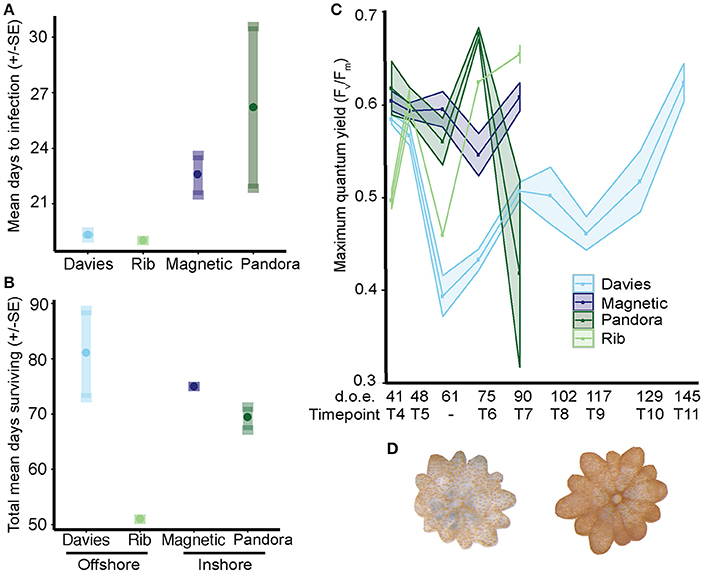
Figure 8. Time to infection and survival of Acropora tenuis juveniles exposed to sediments from central GBR sites. Inshore reefs are in darker shades and offshore sites in lighter shades (see x-axis). (A) Mean time to infection ± SE per sediment treatment. Circles represent means; minimum and maximum values are located at the end of each standard error whisker. (B) Juvenile survival in days (mean ± SE) per sediment treatment. (C) Maximum quantum yield (Fv/Fm) of juveniles from 52–166 days post-fertilization. Due to low survivorship and cumulative sampling of Rib recruits, only one individual was measured at days 59 and 72, and two individuals at day 102, which represents the number of days of exposure to treatments. (D) Two juveniles from the Davies sediment treatment at day 117, highlighting the level of variation in symbiont proliferation within juveniles exposed to sediments from different sites.
Survival of juveniles did not differ when juveniles were exposed to offshore (68.9 ± 5.7 days) vs. inshore (71.95 ± 1.2) sediments (Poisson GLMM: p = 0.179), although significant differences did exist at the site level (p = 0.002) (Figure 8B). Juveniles exposed to Rib sediments survived significantly fewer days (51 ± 0 days) compared to those exposed to Davies (80.8 ± 7.9, TPH: p = 0.003) and Magnetic (75 ± 0, TPH: p = 0.03) sediments. However, survival did not differ significantly between juveniles exposed to Rib vs. Pandora sediments (69.1 ± 2.1, TPH: p = 0.1). Survival of juveniles exposed to sediments from Davies, Magnetic and Pandora did not differ significantly (TPH: p = 0.5–0.9).
Photo-Physiology of Juveniles Exposed to Sediment Treatments
Temporal Patterns in Photochemical Efficiency Compared among Juveniles Exposed to Central Sector Sediments
The photochemical efficiency (Fv/Fm) of juveniles varied significantly among sediment treatments and over time (Figure 8C; GAM: p = 0.0283, Table S4). Fv/Fm values for juveniles exposed to Davies sediments were initially ~0.6, but dropped sharply by day 48, and then generally increased until day 145. In comparison to juveniles exposed to Davies sediments, temporal patterns in Fv/Fm values differed significantly for juveniles exposed to sediments from the other three locations: Magnetic (t = 2.275, p = 0.0236), Pandora (t = 2.137, p = 0.0334), and Rib (t = 1.997, p = 0.0467). Differences in Fv/Fm yields among juveniles exposed to sediments from different locations were also correlated with distinct shifts in Symbiodinium communities in these juveniles, for example, the increase in A3 coincided with decreases in Fv/Fm (Figures 7, 8C, Table 2; Supplementary Results).
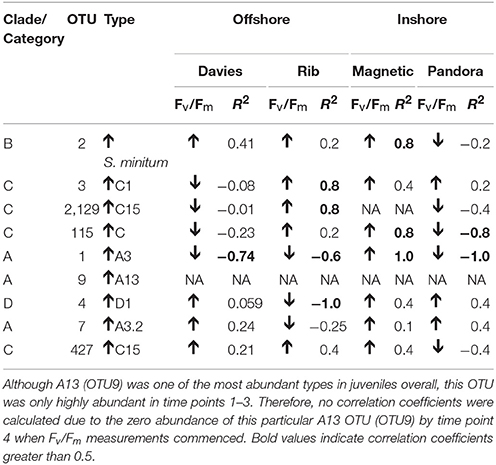
Table 2. Spearman's rho R2 correlation coefficients calculated for each of the nine most highly abundant OTUs in juveniles compared to Fv/Fm values per site.
Variation in Symbiodinium Communities between Sediment-Exposed Juveniles of A. tenuis and A. Millepora
In northern sediment treatments, juveniles of both A. millepora and A. tenuis were dominated by Symbiodinium A3 and D1, and to a lesser extent by D1a in A. millepora. Overall, Symbiodinium communities did not differ between A. tenuis and A. millepora juveniles exposed to northern sediments after 35 days (permutational multivariate analysis of variance: Df 1,58, F = 2.036, R2 = 0.034, p = 0.095), although the abundance of nine OTUs varied between species (B-H adjusted p-values < 0.05, Table S5). In contrast, overall Symbiodinium communities hosted by juveniles of these two species did differ significantly after 27–30 days of exposure to central sediments (Df 1,41, F = 4.83, R2 = 0.107, p = 0.001). A. tenuis juveniles exposed to central sediments were more diverse and had significantly greater abundances of six A types, B1, and C1 (B-H adjusted p-values < 0.05, Table S6) compared to A. millepora, which was characterized by additional diversity within C15, D1, and D1a types.
Environmental Covariates of Symbiodinium Communities in Sediments
WQI, SST, carbonate, and mud content correlated with the normalized abundances of Symbiodinium types from clades A, B, C, and D, but not types within clades E, F, and G (Figure 9, Figure S5, Table S7). Responses of free-living Symbiodinium to these environmental covariates were generally linear, in contrast to the non-linear responses of in hospite Symbiodinium found in an earlier study (Cooper et al., 2011). Types from clade A were most variable in the covariates that influenced their distributions, with S. natans increasing in abundance with increasing WQI and decreasing SST, and decreasing in abundance with lower carbonate and mud content. This contrasted with A3 abundances, which decreased with increasing WQI. The abundance of type A2 was significantly influenced by SST, with greater abundances at higher temperatures. B2 and B4 increased in abundance with increasing SST and sand content (low mud). C1 increased in abundance as carbonate content decreased, whereas none of the environmental covariates were particularly important in explaining the distributions of types C15, C3, or C90. Interestingly, temperature did not significantly explain D1 or D1a abundance, which significantly increased as carbonate decreased in contrast to in hospite patterns (i.e., higher abundance in offshore locations Figure 6A). S. voratum (E) distributions increased with less carbonate. Finally, none of the environmental covariates tested here explained patterns in the abundances of types S. kawaguti (F1), G3 and G6, suggesting that other, unmeasured environmental covariates are important in explaining the distributions of these types. Correspondingly, nutrient profiles and percent of sediments in the <2,000 μM particle size class differed significantly among locations (Supplementary Results, Figures S4A,B, Table S8).
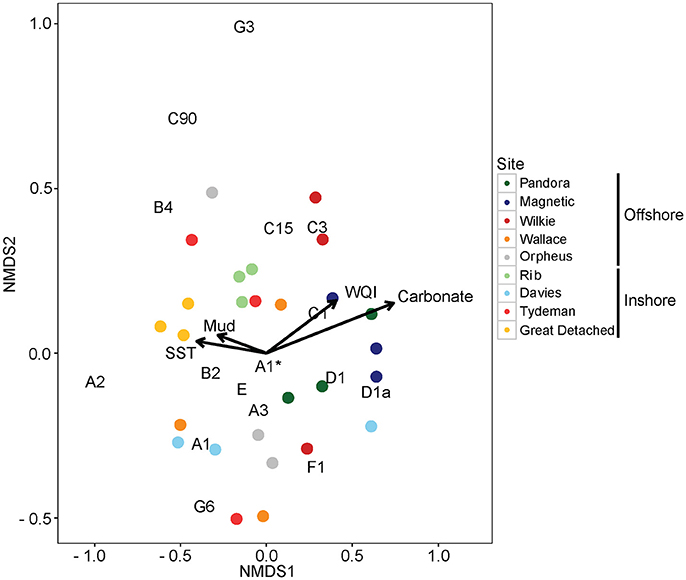
Figure 9. Nonmetric multidimensional scaling (NMDS) of variance-normalized Symbiodinium type abundances (summed OTUs that belong to the same type) in pre-experimental sediment samples using Bray-Curtis distances. Northern sediments are in yellow and red, and central sediments are in gray, blue and green colors. Inshore and offshore sediment samples are in dark and light hues, respectively. Environmental covariates (mud, carbonate, Sea Surface Temperature, and Water Quality Index) are overlaid as vectors. Large vector values for mud and carbonate represent low percent mud and carbonate (see Materials and Methods). Symbiodinium types in black are also overlaid onto the ordination space. As an example, high normalized abundances of types D1 and D1a correspond to high mud content (i.e., low vector values of mud). A1* is equivalent to S. natans.
Discussion
Comparisons between free-living, sediment-associated Symbiodinium communities and endosymbiotic communities in juveniles of the horizontally-transmitting corals A. tenuis and A. millepora reveal a bipartite strategy governing Symbiodinium acquisition. Low overlap between OTUs in the sediments and those taken up by juveniles indicates that corals are capable of a degree of selectivity in the symbionts they acquire from environmental sources. At the same time, a component of the variation in Symbiodinium communities acquired by juveniles reflected variation amongst sediment sources, indicating that local environmental availability also plays a role, albeit a lesser one, in structuring in hospite Symbiodinium communities. Variation in the diversity and abundance of Symbiodinium types in sediment sources across thermal and water quality gradients suggests that environmental conditions influence biogeographical partitioning of free-living communities. Detection of previously unknown levels of Symbiodinium diversity in the sediments, combined with evidence that the composition of in hospite Symbiodinium communities influences infection rates, survival, and photo-physiology of coral juveniles, has implications for local adaptation of both free-living Symbiodinium populations and symbiotic partnerships.
Symbiodinium Uptake by Acropora Juveniles Is Selective but Varies with Local Availability
Only 10.6% of the 1,562 Symbiodinium OTUs recovered in this dataset were shared between juveniles and sediments, and OTUs taken up did not necessarily reflect those dominant in sediments. In combination, these findings do not support previous suggestions that the acquisition of Symbiodinium from environmental sources is “promiscuous” in early life-history stages of corals (Cumbo et al., 2013). Instead, the low overlap found between these two communities is consistent with a degree of selectivity in juvenile uptake, a conclusion supported by other studies that have compared seawater or benthic communities with those in hospite, in association with either adult hard corals (Pochon et al., 2010; Huang et al., 2013; Sweet, 2013) or juveniles of both octocorals and acroporids (Coffroth et al., 2006; Yamashita et al., 2013). Despite only a small proportion of Symbiodinium types being taken up out of the wide range available, communities in juveniles were not highly conserved at the majority of locations. Such differences in in hospite communities among sediment source treatments suggest that local availability of Symbiodinium in the sediments can influence in hospite juvenile diversity to a degree. These results align with previous estimates of the influence that environmental availability of Symbiodinium has on the formation of those communities in A. tenuis juveniles ( = 71%, Quigley et al., 2017b). For example, juveniles exposed to inshore sediments, which had higher abundances of clades B and D than offshore sediments, concomitantly had higher abundances of these two clades than juveniles exposed to offshore sediments. Linkages between local sediment diversity and in hospite communities for the subset of types taken-up may have important implications for local adaptation of coral hosts.
Predisposition for Up-Take of Specific Symbiodinium Types
Although local availability of free-living Symbiodinium was a contributing factor to the structure of in hospite symbiont communities, overall, correlation analyses revealed low similarity in the abundance and diversity of Symbiodinium between juvenile and sediment communities at the type/OTU level. The general lack of congruence between sediment communities and communities found in juveniles exposed to them suggests that there is also a genetic component to symbiont regulation in corals (Quigley et al., 2017a,b). A predisposition for acquiring certain Symbiodinium types is supported by the acquisition of clade C symbionts in offshore sediment treatments when their comparative availability in offshore sediments was low, potentially signifying the presence of recognition mechanisms, consistent with findings that clade C ultimately dominates adult communities of these corals (Abrego et al., 2009). A bipartite system, involving both genetic and environmental contributions to the structuring of symbiont communities, has also been observed in closely-related Nematostella-bacterial partnerships (Mortzfeld et al., 2015). Knowledge of the environmental availability of symbionts, in conjunction with an understanding of the genetic mechanisms regulating symbiont uptake by the host, are therefore required to predict the potential for differential selection of Symbiodinium to affect coral health.
Symbiodinium communities in juveniles varied through time, although it is unclear whether patterns reflected changes associated with juvenile development or dynamics among Symbiodinium types. Similar fine-scale successional patterns over short time-scales (1–2 weeks) in bacterial communities have been tied to developmental (including immunological) remodeling in Nematostella, amphibians and insects (Mortzfeld et al., 2015). Lack of change in relevant symbiont availability within the sediments from the start to the end of the experiment indicates that lack of symbionts available for infection was not the underlying reason, but does suggest that active selection by the host or competition among symbionts was driving temporal changes in community structure. Although developmental changes associated with metamorphosis in other invertebrate systems may be more extreme, skeletogenesis, and developmental changes occurring during the juvenile stage have large impacts on gene expression, differentiating between patterns expressed by larval and adult stages (Reyes-Bermudez et al., 2009). Accordingly, temporal differences and successional patterns in Symbiodinium communities associated with A. tenuis juveniles may be tied to changing requirements associated with the onset of specific physiological processes (e.g., calcification) or changing colony morphology (e.g., the onset of vertical colony growth; Abrego et al., 2009). In either case, a preference for increasing abundances of clade C over D symbionts may reflect a need for greater translocation of photosynthates, as provided by clade C in experimental studies (Cantin et al., 2009), which can cause 3-fold greater juvenile growth rates in field studies (Little et al., 2004). Further work is needed to determine if selection for Symbiodinium from clade C is tied to calcification or other physiological processes during early ontogeny.
Differences in the Symbiodinium communities acquired by juveniles of A. millepora and A. tenuis highlight the diversity in the evolutionary trajectories that underpin the development of symbioses in these two congeneric species. Although there was limited overlap between Symbiodinium diversity in juveniles and the sediments, other coral species may utilize and potentially benefit from the Symbiodinium diversity in sediments not taken up by these acroporid species. Additionally, A. millepora juveniles in both the northern and central sediment treatments had order of magnitude greater abundances of Symbiodinium D1 and D1a (generally heat tolerant types), than A. tenuis. If species-specific preferences for D types exist, then by not hosting thermally tolerant types, A. tenuis juveniles may be at a comparative disadvantage under future climate change scenarios. Further studies comparing the selection of Symbiodinium across different species are required to fully understand the adaptive benefits of flexibility during symbiont acquisition in juvenile corals.
Differences in Diversity among Sediment Symbiodinium Communities Reflect Variation in Water Quality, Temperature, and Sediment Characteristics
Four times more unique Symbiodinium OTUs were recovered from the sediments than from juveniles, representing 72% (1,125) of the overall OTU diversity detected, and indicating that diversity of the free-living community is high. Whilst results of this study are consistent with previous reports of clades A and C occurring in high abundances in Pacific sediment communities (Manning and Gates, 2008; Takabayashi et al., 2012), the majority of sediment reads detected here belonged to the “uncultured” category. This indicates that many undiscovered and presently uncharacterized types exist within GBR sediments and may represent a reservoir of diversity with potentially adaptive benefits for corals. Symbiodinium types that were unique to the sediments differed among the four regions, with the most extreme differences in type diversity being between the northern offshore and central inshore sediments. Inshore central sediments harbored the most distinct Symbiodinium communities found and warrants further exploration.
Differences in the abundances of free-living Symbiodinium types were also explained by different environmental covariates. For example, C types dominated communities in cooler, nutrient-poor reefs (central offshore sediments) and A types dominated in warmer, higher nutrient, turbid waters (northern inshore sediments). These same patterns were found in the Arabian Gulf, where C1 dominates P. verrucosa colonies in colder, nutrient poor waters, whilst A1 dominates warmer, higher nutrient and more turbid waters (Sawall et al., 2014). Offshore reef corals in Palau were also found to be dominated by C types, whilst warmer inshore corals harbored D1a or D1–4 (Russell et al., 2016). Strong biogeographic patterns of in hospite Symbiodinium types associated with a soft coral have also been found within clades (Howells et al., 2009) and may be related to variation in local temperature, nutrient and irradiance regimes. Detection of C types in central offshore sediments and A types in northern inshore sediments in both free-living and in hospite communities provides compelling evidence that environmental factors contribute to the structure of both symbiotic and environmental communities.
The partitioning of Symbiodinium communities in the sediments can be ascribed to balancing selection exerted by hosts continually inoculating the sediments, as well as exposure to different environmental conditions, which in combination maintain overall high diversity of Symbiodinium in sediment reservoirs. This reservoir of diversity within sediments might be important to counter loss of in hospite diversity from disturbance events and competitive exclusion amongst symbiont types, as has been observed in diverse soil bacterial communities (Martorell et al., 2015). Recent evidence that environmental conditions explain significant variation in the genetic structure of clade C communities (Davies et al., 2016) also highlights the substantial potential for local adaptation of symbionts. Further work on Symbiodinium communities in the sediments is needed to describe this important niche and the potentially stress-tolerant species it may contain, including the sequencing of samples collected across environmental gradients representing more extreme environments.
Changes in Diversity and Abundance of Dominant and Background Symbiodinium Types Impact Coral Juvenile Physiology
Variation in juvenile survival amongst sediment treatments in the current study may have been driven by differences in the composition and dynamics of the Symbiodinium communities acquired. Even fine-scale changes in the relative abundances of some types may translate to large scale impacts at the colony level (Cunning et al., 2015a; Quigley et al., 2016). For example, small shifts in bacterial community composition in Nematostella resulted in changes to host fitness, potentially caused by variation in the immune responses elicited by different symbionts under different selective pressures (Mortzfeld et al., 2015). Alternatively, the early acquisition of certain types may have led to greater long-term juvenile survival. For example, 6-fold greater abundances of C and D1 in juveniles exposed to Davies and Magnetic sediments at the first time point corresponded to higher survival compared to juveniles exposed to sediments from other locations. Early acquisition of C and D1 by Acropora yongei juveniles has also been correlated with increased juvenile survival on Japanese reefs (Suzuki et al., 2013). Further work is needed to understand how the dynamics of mixed Symbiodinium communities contribute to long-term coral juvenile survival, and how this relationship is modulated by the environment and changing environmental regimes.
Variation in the in hospite Symbiodinium community among juveniles may also explain the differences we found in time to infection and photosynthetic parameters among communities. Symbiodinium infection/proliferation occurred more quickly when juveniles were exposed to offshore sediments (characterized by more abundant C15, A3, and C-types) compared to inshore sediments (characterized by B1, D-types or C1/C-types). Slower infection by cultured D-types compared to C1 is commonly observed in the lab (K. Quigley, pers. obs.), potentially explaining the slower rates of infection when juveniles were exposed to inshore sediments. More rapid rates of infection in juveniles exposed to Rib and Davies (central offshore) sediment treatments may reflect an increased capacity of A3, C15, and C to infect and proliferate within coral juveniles. The presence of A3, both when dominant or at background levels of abundance, also strongly impacted Fv/Fm in juveniles and provides corroborative support that numerically rare background symbionts contribute to changes in the photo-physiological performance of their hosts (Erwin et al., 2012; Hoadley et al., 2015; Karim et al., 2015; Mortzfeld et al., 2015). Lower Fv/Fm values in juveniles that acquired symbionts from offshore sediments and spikes in chlorophyll fluorescence may indicate that the symbionts acquired were adapted to higher light environments (due to increased sediment particle size or decreased turbidity) and a stress response (Shapiro et al., 2016), respectively. These results highlight that the establishment of symbiosis is a highly dynamic process governed by a range of interacting factors.
Conclusion
Low overlap between Symbiodinium communities in the sediments and in hospite Symbiodinium communities in juveniles exposed to those sediments suggests that overall, symbiont uptake is a selective process. Local environmental availability of Symbiodinium also contributes to in hospite community structure, although to a lower degree. Evidence that sediment Symbiodinium communities vary across temperature and water quality gradients highlights the potential of changing environments to disrupt environmental sources of Symbiodinium diversity and therefore the establishment of symbiosis, an important consideration given that the vast majority of coral species on the GBR take up Symbiodinium from environmental sources anew each generation. Newly discovered Symbiodinium diversity in coral reef sediments presented here highlights the presence of a hitherto unknown reservoir of diversity that may enable corals to adapt to environmental change through the utilization of symbionts at initially low background abundances or by potentially forming new stress-resistant partnerships. The low overlap in diversity between sediment and in hospite biomes, paired with evidence of strong biogeographical partitioning of free-living Symbiodinium communities in the sediments, has implications for local adaptation of symbiotic partnerships and highlights the need for studies of how climate change and other anthropogenic stressors might disrupt linkages between free-living and in hospite Symbiodinium communities.
Author Contributions
KQ, LB, and BW conceived and designed the experiment. KQ performed the laboratory work, data analysis and figure preparation and wrote the manuscript. KQ, LB, and BW critically revised the draft and approved the final version and are in agreement to be accountable for all aspects of the work.
Funding
Funding was provided by the Australian Research Council through ARC CE1401000020, ARC DP130101421 to BW and AIMS to LB.
Conflict of Interest Statement
The authors declare that the research was conducted in the absence of any commercial or financial relationships that could be construed as a potential conflict of interest.
Acknowledgments
We thank Orpheus Island Research Station and the Seasim staff for assistance in the set-up of the filtration and tank systems as well as Marie Strader for Acropora millepora juveniles. Assistance with sediment collections was provided by Ray Berkelmans, Melissa Rocker, David Stump and Carly Kenkel; Stephan Boyle provided assistance with the nutrient analysis; Andrew Negri for use of sonication filter; Kathryn Evans for her help with filtering sediments. Permits for coral collections and manipulations in 2013 were as follows: A. tenuis colonies from Wilkie Bay (Permit number: G12/35236.1) and eight A. tenuis and six A. millepora from south Orpheus Island (Permit number: G13/36318.1). Permits for coral collections and manipulations in 2014 were as follows: four A. tenuis colonies from Magnetic Island (Permit Number: G13/36318.1) and eight A. millepora colonies from Trunk Reef (Permit Number: G12/35236.1).
Supplementary Material
The Supplementary Material for this article can be found online at: https://www.frontiersin.org/articles/10.3389/fmars.2017.00401/full#supplementary-material
References
Abrego, D., van Oppen, M. J., and Willis, B. L. (2009). Onset of algal endosymbiont specificity varies among closely related species of Acropora corals during early ontogeny. Mol. Ecol. 18, 3532–3543. doi: 10.1111/j.1365-294X.2009.04276.x
Adams, L. M., Cumbo, V., and Takabayashi, M. (2009). Exposure to sediment enhances primary acquisition of Symbiodinium by asymbiotic coral larvae. Mar. Ecol. Prog. Ser. 377, 156–179. doi: 10.3354/meps07834
Baker, A. C. (2003). Flexibility and specificity in coral-algal symbiosis: diversity, ecology, and biogeography of Symbiodinium. Annu. Rev. Ecol. Evol. Syst. 34, 661–689. doi: 10.1146/annurev.ecolsys.34.011802.132417
Bates, D., Maechler, M., Bolker, B., and Walker, S. (2014). lme4: Linear Mixed-Effects Models using Eigen and S4. R Packag. version 1. Ithaca, NY.
Becker, R. A., Chambers, J. M., and Wilks, A. R. (1988). The New S Language. Pacific Grove, CA: Wadsworth Brooks.
Brodie, J., De'Ath, G., Devlin, M., Furnas, M., and Wright, M. (2007). Spatial and temporal patterns of near-surface chlorophyll a in the Great Barrier Reef lagoon. Mar. Freshw. Res. 58, 342–353. doi: 10.1071/MF06236
Cantin, N., van Oppen, M., Willis, B., Mieog, J., and Negri, A. (2009). Juvenile corals can acquire more carbon from high-performance algal symbionts. Coral Reefs 28, 405–414. doi: 10.1007/s00338-009-0478-8
Coffroth, M. A., Lewis, C. F., Santos, S. R., and Weaver, J. L. (2006). Environmental populations of symbiotic dinoflagellates in the genus Symbiodinium can initiate symbioses with reef cnidarians. Curr. Biol. 16, R985–R987. doi: 10.1016/j.cub.2006.10.049
Cooper, T. F., Berkelmans, R., Ulstrup, K. E., Weeks, S., Radford, B., Jones, A. M., et al. (2011). Environmental factors controlling the distribution of Symbiodinium harboured by the coral Acropora millepora on the great barrier reef. PLoS ONE 6:e25536. doi: 10.1371/journal.pone.0025536
Cumbo, V. R., Baird, A. H., and van Oppen, M. J. H. (2013). The promiscuous larvae: flexibility in the establishment of symbiosis in corals. Coral Reefs 32, 111–120. doi: 10.1007/s00338-012-0951-7
Cunning, R., Silverstein, R. N., and Baker, A. C. (2015a). Investigating the causes and consequences of symbiont shuffling in a multi-partner reef coral symbiosis under environmental change. Proc. R. Soc. B 282:20141725. doi: 10.1098/rspb.2014.1725
Cunning, R., Yost, D. M., Guarinello, M. L., Putnam, H. M., and Gates, R. D. (2015b). Variability of Symbiodinium communities in waters, sediments, and corals of thermally distinct reef pools in American Samoa. PLoS ONE 10:e0145099. doi: 10.1371/journal.pone.0145099
Davies, S. W., Wham, D. C., Kanke, M. R., and Matz, M. V. (2016). Ecological factors rather than physical barriers to dispersal shape genetic structure of algal symbionts in horizontally-transmitting corals. bioRxiv. doi: 10.1101/037994
De'ath, G. (2007). The Spatial, Temporal and Structural Composition of Water Quality of the Great Barrier Reef, and Indicators of Water Quality and Mapping Risk. Report to Marine and Tropical Sciences Research Facility (MTSRF), Cairns. Australian Institute of Marine Science. 66 p.
De'ath, G., and Fabricius, K. (2008). Water Quality of the Great Barrier Reef: Distributions, Effects on Reef Biota and Trigger Values for the Protection of Ecosystem Health. Townsville, QLD: Great Barrier Reef Marine Park Authority.
Devlin, Q. (2015). Nutrient Dynamics in the Coral-Algal Symbiosis: Developing Insight from Biogeochemical Techniques. Available online at: http://scholarlyrepository.miami.edu/oa_dissertations/1514
Donner, S. D. (2009). Coping with commitment: projected thermal stress on coral reefs under different future scenarios. PLoS ONE 4:e5712. doi: 10.1371/journal.pone.0005712
Entsch, B., Boto, K. G., Sim, R. G., and Wellington, J. T. (1983). Phosphorus and nitrogen in coral reef sediments. Limnol. Oceanogr. 28, 465–476. doi: 10.4319/lo.1983.28.3.0465
Erwin, P. M., Pita, L., López-Legentil, S., and Turon, X. (2012). Stability of sponge-associated bacteria over large seasonal shifts in temperature and irradiance. Appl. Environ. Microbiol. 78, 7358–7368. doi: 10.1128/AEM.02035-12
Fadlallah, Y. H. (1983). Sexual reproduction, development and larval biology in scleractinian corals. Coral Reefs 2, 129–150. doi: 10.1007/BF00336720
Furnas, M. J. (2003). Catchments and Corals: Terrestrial Runoff to the Great Barrier Reef. Australian Institute of Marine Science & CRC Reef Research Centre. p. 334.
Furnas, M., Mitchell, A., Skuza, M., and Brodie, J. (2005). In the other 90%: phytoplankton responses to enhanced nutrient availability in the Great Barrier Reef Lagoon. Mar. Pollut. Bull. 51, 253–265. doi: 10.1016/j.marpolbul.2004.11.010
Garren, M., Walsh, S. M., Caccone, A., and Knowlton, N. (2006). Patterns of association between Symbiodinium and members of the Montastraea annularis species complex on spatial scales ranging from within colonies to between geographic regions. Coral Reefs 4, 503–512. doi: 10.1007/s00338-006-0146-1
Guiry, M., and Guiry, G. (2016). AlgaeBase. Natl. Univ. Irel. Available online at: http://www.algaebase.org
Hijmans, R. J., and van Etten, J. (2014). Geographic Data Analysis and Modeling. R package raster version 2.5-8.
Hijmans, R. J., Phillips, S., Leathwick, J., and Elith, J. (2013). Dismo: Species Distribution Modeling. R Packag. version 0.8–17.
Hill, R., and Ulstrup, K. E. (2005). “Imaging-PAM: operation and possibilities,” in Understanding the Stress Response of Corals and Symbiodinium in a Rapidly Changing Environment (Mexico), 29.
Hoadley, K. D., Pettay, D. T., Grottoli, A. G., Cai, W.-J., Melman, T. F., Schoepf, V., et al. (2015). Physiological response to elevated temperature and pCO2 varies across four Pacific coral species: understanding the unique host+ symbiont response. Sci. Rep. 5, 1–15. doi: 10.1038/srep18371
Howells, E. J., van Oppen, M. J. H., and Willis, B. L. (2009). High genetic differentiation and cross-shelf patterns of genetic diversity among Great Barrier Reef populations of Symbiodinium. Coral Reefs 28, 215–225. doi: 10.1007/s00338-008-0450-z
Huang, H., Zhou, G., Yang, J., Liu, S., You, F., and Lei, X. (2013). Diversity of free-living and symbiotic Symbiodinium in the coral reefs of Sanya, South China Sea. Mar. Biol. Res. 9, 117–128. doi: 10.1080/17451000.2012.708045
Iluz, D., and Dubinsky, Z. (2015). Coral photobiology: new light on old views. Zoology 118, 71–78. doi: 10.1016/j.zool.2014.08.003
Jones, R. J., Kildea, T., and Hoegh-Guldberg, O. (1999). PAM chlorophyll fluorometry: a new in situ technique for stress assessment in scleractinian corals, used to examine the effects of cyanide from cyanide fishing. Mar. Pollut. Bull. 38, 864–874. doi: 10.1016/S0025-326X(98)90160-6
Karim, W., Nakaema, S., and Hidaka, M. (2015). Temperature effects on the growth rates and photosynthetic activities of Symbiodinium cells. J. Mar. Sci. Eng. 3, 368–381. doi: 10.3390/jmse3020368
Krause, G. H., and Weis, E. (1991). Chlorophyll fluorescence and photosynthesis: the basics. Annu. Rev. Plant Biol. 42, 313–349. doi: 10.1146/annurev.pp.42.060191.001525
Little, A. F., van Oppen, M. J. H., and Willis, B. L. (2004). Flexibility in algal endosymbioses shapes growth in reef corals. Science 304, 1492–1494. doi: 10.1126/science.1095733
Lønborg, C., Devlin, M., Waterhouse, J., Brinkman, R., Costello, P., da Silva, E., et al. (2016). Marine Monitoring Program: Annual Report for Inshore Water Quality Monitoring 2014 to 2015. Report for the Great Barrier Reef Marine Park Authority. Australian Institute of Marine Science, Townsville. p. 160.
Love, M. I., Anders, S., and Huber, W. (2014a). Differential analysis of count data–the DESeq2 package. Genome Biol. 15:550.
Love, M. I., Huber, W., and Anders, S. (2014b). Moderated estimation of fold change and dispersion for RNA-seq data with DESeq2. Genome Biol. 15, 1–21. doi: 10.1186/s13059-014-0550-8
Manning, M. M., and Gates, R. D. (2008). Diversity in populations of free-living Symbiodinium from a Caribbean and Pacific reef. Limnol. Oceanogr. 53, 1853–1861. doi: 10.4319/lo.2008.53.5.1853
Martorell, C., Almanza-Celis, C. A. I., Pérez-García, E. A., and Sánchez-Ken, J. G. (2015). Co-existence in a species-rich grassland: competition, facilitation and niche structure over a soil depth gradient. J. Veg. Sci. 26, 674–685. doi: 10.1111/jvs.12283
Maxwell, K., and Johnson, G. N. (2000). Chlorophyll fluorescence—a practical guide. J. Exp. Bot. 51, 659–668. doi: 10.1093/jexbot/51.345.659
McMurdie, P. J., and Holmes, S. (2013). Phyloseq: an R package for reproducible interactive analysis and graphics of microbiome census data. PLoS ONE 8:e61217. doi: 10.1371/journal.pone.0061217
Mortzfeld, B. M., Urbanski, S., Reitzel, A. M., Künzel, S., Technau, U., and Fraune, S. (2015). Response of bacterial colonization in Nematostella vectensis to development, environment and biogeography. Environ. Microbiol. 18, 1764–1781. doi: 10.1111/1462-2920.12926
Murdoch, D., Chow, E. D., and Celayeta, J. M. F. (2007). Ellipse: Functions for Drawing Ellipses and Ellipse-like Confidence Regions. R Packag. version 0.3-5.
Nitschke, M. R., Davy, S. K., and Ward, S. (2015). Horizontal transmission of Symbiodinium cells between adult and juvenile corals is aided by benthic sediment. Coral Reefs 35, 335–344. doi: 10.1007/s00338-015-1349-0
Oksanen, J., Blanchet, F. G., Kindt, R., Legendre, P., Minchin, P. R., O'Hara, R. B., et al. (2013). Package “Vegan.” Community Ecol. Packag. version 2.
Pinheiro, J., and Bates, D. (2006). Mixed-Effects Models in S and S-PLUS. New York, NY: Springer Science & Business Media.
Pochon, X., Stat, M., Takabayashi, M., Chasqui, L., Chauka, L. J., Logan, D. D. K., et al. (2010). Comparison of endosymbiotic and free-living Symbiodinium (Dinophyceae) diversity in a Hawaiian reef environment. J. Phycol. 46, 53–65. doi: 10.1111/j.1529-8817.2009.00797.x
Quigley, K., Warner, P., Bay, L., and Willis, B. (2017a). Unexpected mixed-mode transmission and moderate genetic regulation of Symbiodinium communities in a brooding coral. bioRxiv. doi: 10.1101/173591
Quigley, K. M., Willis, B. L., and Bay, L. K. (2016). Maternal effects and Symbiodinium community composition drive differential patterns in juvenile survival in the coral Acropora tenuis. R. Soc. Open Sci. 3, 1–17. doi: 10.1098/rsos.160471
Quigley, K. M., Willis, B. L., and Bay, L. (2017b). Heritability of the Symbiodinium community in vertically-and horizontally-transmitting broadcast spawning corals. Sci. Rep. 7, 8219. doi: 10.1038/s41598-017-08179-4
R Team, C. (2013). R Core Team. 2013. R: A Language and Environment for Statistical Computing. Vienna: R Foundation for Statistical Computing.
Ramette, A. (2007). Multivariate analyses in microbial ecology. FEMS Microbiol. Ecol. 62, 142–160. doi: 10.1111/j.1574-6941.2007.00375.x
Rees, T. A. V. (1991). Are symbiotic algae nutrient deficient? Proc. R. Soc. Lond. B Biol. Sci. 243, 227–233. doi: 10.1098/rspb.1991.0036
Reyes-Bermudez, A., DeSalvo, M. K., Voolstra, C. R., Sunagawa, S., Szmant, A. M., Iglesias-Prieto, R., et al. (2009). Gene expression microarray analysis encompassing metamorphosis and the onset of calcification in the scleractinian coral Montastraea faveolata. Mar. Genomics 2, 149–159. doi: 10.1016/j.margen.2009.07.002
Russell, B. J., Dierssen, H. M., LaJeunesse, T. C., Hoadley, K. D., Warner, M. E., Kemp, D. W., et al. (2016). Spectral reflectance of Palauan reef-building coral with different symbionts in response to elevated temperature. Remote Sens. 8:164. doi: 10.3390/rs8030164
Sawall, Y., Al-Sofyani, A., Banguera-Hinestroza, E., and Voolstra, C. R. (2014). Spatio-temporal analyses of Symbiodinium physiology of the coral Pocillopora verrucosa along large-scale nutrient and temperature gradients in the Red Sea. PLoS ONE 9:e103179. doi: 10.1371/journal.pone.0103179
Shapiro, O. H., Kramarsky-Winter, E., Gavish, A. R., Stocker, R., and Vardi, A. (2016). A coral-on-a-chip microfluidic platform enabling live-imaging microscopy of reef-building corals. Nat. Commun. 7:10860. doi: 10.1038/ncomms10860
Storlazzi, C. D., Norris, B. K., and Rosenberger, K. J. (2015). The influence of grain size, grain color, and suspended-sediment concentration on light attenuation: why fine-grained terrestrial sediment is bad for coral reef ecosystems. Coral Reefs 34, 967–975. doi: 10.1007/s00338-015-1268-0
Suzuki, G., Yamashita, H., Kai, S., Hayashibara, T., Suzuki, K., Iehisa, Y., et al. (2013). Early uptake of specific symbionts enhances the post-settlement survival of Acropora corals. Mar. Ecol. Prog. Ser. 494, 149–158. doi: 10.3354/meps10548
Sweet, M. (2013). Symbiodinium diversity within Acropora muricata and the surrounding environment. Mar. Ecol. 35, 343–353. doi: 10.1111/maec.12092
Takabayashi, M., Adams, L., Pochon, X., and Gates, R. (2012). Genetic diversity of free-living Symbiodinium in surface water and sediment of Hawai'i and Florida. Coral Reefs 31, 157–167. doi: 10.1007/s00338-011-0832-5
van Oppen, M. J. H., Baker, A. C., Coffroth, M. A., and Willis, B. L. (2009). “Bleaching resistance and the role of algal endosymbionts coral bleaching,” in Coral Bleahcing: Patterns, Processes, Causes and Consequences, eds M. van Oppen and J. Lough (Berlin; Heidelberg: Springer), 83–102.
Wickham, H. (2009). ggplot2: Elegant Graphics for Data Analysis. New York, NY: Springer Science & Business Media.
Wilson, K., Li, Y., Whan, V., Lehnert, S., Byrne, K., Moore, S., et al. (2002). Genetic mapping of the black tiger shrimp Penaeus monodon with amplified fragment length polymorphism. Aquaculture 204, 297–309. doi: 10.1016/S0044-8486(01)00842-0
Wood, S. (2008). Fast stable direct fitting and smoothness selection for generalized additive models. J. R. Stat. Soc. B Stat. Methodol. 70, 495–518. doi: 10.1111/j.1467-9868.2007.00646.x
Wood, S. N. (2000). Modelling and smoothing parameter estimation with multiple quadratic penalties. J. R. Stat. Soc. B Stat. Methodol. 62, 413–428. doi: 10.1111/1467-9868.00240
Keywords: Symbiodinium, free-living, juvenile, uptake, symbiosis, coral reefs, sediments
Citation: Quigley KM, Bay LK and Willis BL (2017) Temperature and Water Quality-Related Patterns in Sediment-Associated Symbiodinium Communities Impact Symbiont Uptake and Fitness of Juveniles in the Genus Acropora. Front. Mar. Sci. 4:401. doi: 10.3389/fmars.2017.00401
Received: 07 October 2017; Accepted: 27 November 2017;
Published: 15 December 2017.
Edited by:
Michael Sweet, University of Derby, United KingdomReviewed by:
Lyndon Mark DeVantier, Coral Reef Research, AustraliaAnthony William Larkum, University of Technology Sydney, Australia
Copyright © 2017 Quigley, Bay and Willis. This is an open-access article distributed under the terms of the Creative Commons Attribution License (CC BY). The use, distribution or reproduction in other forums is permitted, provided the original author(s) or licensor are credited and that the original publication in this journal is cited, in accordance with accepted academic practice. No use, distribution or reproduction is permitted which does not comply with these terms.
*Correspondence: Kate M. Quigley, a2F0ZW1hcmllLnF1aWdsZXlAbXkuamN1LmVkdS5hdQ==
†Present Address: Kate M. Quigley, Australian Institute of Marine Science, Townsville, QLD, Australia