- Department of Marine Sciences-Tjärnö, University of Gothenburg, Strömstad, Sweden
The life cycle of many marine benthic species includes a pelagic larval stage that governs the connectivity between populations. Larval transport is a function of hydrodynamic and biological processes. Knowledge of how larval traits affect dispersal will increase the accuracy of biophysical models used to predict connectivity, and is of paramount importance for management and conservation. This study examines the larval traits of the cold-water coral Lophelia pertusa that forms widespread and highly diverse ecosystems in the deep ocean. We monitored development, swimming behavior, and survival under different environmental conditions. We found that the embryonic development rate doubled when the rearing temperature was increased from normal conditions of 7–8°C to 11–12°C. Pre-competent planulae migrated vertically upwards at a speed of 0.5–0.7 mm s−1 and crossed salinity gradients with a maximum tested difference of 5 psu with no hesitation. At 3 weeks, planulae had a fully developed mouth and started feeding on animal derivatives, picoplankton, and possibly smaller size microalgae. Presence of food significantly altered the swimming pattern, and feeding was corroborated by direct observation. Planulae survived for up to 10 months in a salinity of 25 psu, which together with the vertical migration pattern and feeding indicates that larvae may spend a period of their pelagic phase in the photic zone. After 50 days, larvae were still in a very good condition as deduced by maintained high swimming speed. Survival rate of developed planulae was on average 60% over a 3-month period, and maximum longevity was a full year, in laboratory cultures.
Introduction
Knowledge of the hydrodynamic and biological factors that determine dispersal routes and population connectivity is fundamental in any marine ecosystem, and even more so in the deep-sea where monitoring is logistically difficult and very expensive. While rapid progress is being made considering biophysical modeling, the biological data to accommodate these models are largely lacking (Davies and Guinotte, 2011; Hilário et al., 2015; Treml et al., 2015). Besides the importance of ocean currents and hydrodynamics at different scales, the species' life history traits and larval behavior significantly affect dispersal direction and distance (Shanks, 2009), and it is increasingly recognized that hydrodynamic models must include intrinsic larval properties and behavior to successfully predict dispersal routes and population connectivity (e.g., Siegel et al., 2003; Aiken et al., 2007; Cowen and Sponaugle, 2009; Moksnes et al., 2014; Fox et al., 2016). For deep-sea organisms, this knowledge is particularly challenging to obtain and biological data are scarce (Hilário et al., 2015).
Biological traits that may affect dispersal patterns include buoyancy of embryos, development rate, ontogenetic shifts in properties and behavior, vertical migration, mortality, food availability, and physiological tolerances (Hilário et al., 2015). Treml et al. (2015) identified key biophysical drivers of connectivity among marine populations, using a subtidal rocky reef ecosystem as a model. The three parameters consistently found to be most influential on population connectivity were larval mortality during the dispersal phase followed by pelagic larval duration (PLD), and the extent of the pre-competency period. For dispersal distance, PLD was most important. The vertical positioning of larvae in the water column critically affects both dispersal distance and connectivity (e.g., Fiksen et al., 2007; Paris et al., 2007; Corell et al., 2012; Moksnes et al., 2014; Fox et al., 2016), and for deep-sea organisms the effect of being transported in the surface layer compared to deeper layers can be dramatic (Fox et al., 2016).
Empirical measures of larval mortality under natural conditions are scarce despite its importance for dispersal outcomes (Treml et al., 2015). Mortality rates of larvae under laboratory conditions will not reflect mortality rates in the field where predation is an important controlling factor. The pre-competency period, i.e., the time spent in the pelagic before larvae are competent to settle can, however, be inferred from laboratory studies. The length of this period will significantly affect chances of self-recruitment onto the natal reef, with shorter pre-competency periods increasing the proportion of larvae settling locally (Treml et al., 2015). The PLD affects the geographic distance larvae will disperse with longer planktonic duration generally resulting in greater dispersal distance (Siegel et al., 2003; Selkoe and Toonen, 2011; Treml et al., 2015). The realized dispersal distance and direction can, in addition, be influenced significantly by larval behavior (Shanks, 2009). The vertical position of larvae in the water column can change over time (ontogenetic shifts) or with diel or tidal cycles. The most accurate way of finding out where in the water column larvae reside is to collect larvae in the field at different depths and time periods as done by Arellano et al. (2014). Alternatively, the probable in situ depth distribution can be deduced from larval behavior in laboratory experiments, i.e., the tendency of positive or negative buoyancy or active vertical swimming, the timing for settling competency, and the onset of bottom-searching (Tay et al., 2011; Coelho and Lasker, 2016).
The cold-water coral Lophelia pertusa inhabits the deep sea at depths from 39 m in Norwegian fjords (Fosså et al., 2002) down to more than 3,000 m in the Atlantic (Squires, 1959; Zibrowius, 1980). The species is almost exclusively found in oceanic waters at temperatures between 4 and 12°C (Rogers, 1999) although it appears in water masses of ca. 13°C in the Mediterranean (e.g., Taviani et al., 2017). Lophelia pertusa is the most common framework forming cold-water coral (Roberts et al., 2009) and the associated biodiversity is high; in the northeast Atlantic more than 1,300 species are linked to reefs of this coral. Individual colonies within reefs are gonochoric (have separate sexes) and fecundity is high (Waller and Tyler, 2005). Fertilization is external, i.e., eggs and sperm are spawned into the water column where fertilization takes place. Spawning is seasonal, with differences in timing depending on geographical location (Waller and Tyler, 2005; Brooke and Järnegren, 2013; Larsson et al., 2014; Pires et al., 2014). In Scandinavian waters spawning occurs between January and March (Brooke and Järnegren, 2013; Larsson et al., 2014).
In our previous studies of L. pertusa planulae, we have established that the pre-competency period is at least 3 weeks in planulae reared at 7–8°C (Larsson et al., 2014; Strömberg et al., 2018). Embryos reached the 64-cell stage after 48 h, and at 5 days they were ciliated blastulae. Embryos were positively buoyant, and blastulae were modest swimmers, slowly ascending. Gastrulation occurred asynchronously throughout days 6–9 during which the embryos were mainly inactive, and not until day 9 were they fully developed planulae with a complete capacity of vertical adjustment by swimming. The development of an oral pore at 2 weeks that further developed into a protractible and adjustable mouth after about 3 weeks indicated that larvae might be feeding. During the first weeks, larvae resided in the top layer of the water column, and onset of bottom probing behavior was first observed 3 weeks after spawning. This behavior became increasingly common 4–5 weeks after spawning (Larsson et al., 2014). Yet another indication of readiness for settlement was the observation of cnidocyst discharge starting 20 days after spawning, and the use of these for temporary attachment to the substrate (Strömberg et al., 2018).
Cold-water corals have been found growing on oil and gas platforms in the North Sea, with a depth distribution of 60–130 m (Gass and Roberts, 2006), indicating the depth distribution of planulae at the time they are competent for settling. The upper limit for pre-competency planulae during their dispersal stage is, however, more difficult to establish. The food choice of the planulae could give an indication of how high up in the water column they may ascend: that is, if they eat microalgae it is plausible that they reside in the photic zone during part of their planktonic phase, with consequences for their dispersal. Although open ocean surface water usually is fully oceanic in salinity, larvae released from coastal reefs, such as those in the Skagerrak or larvae from North Sea populations transported into the Skagerrak (Fox et al., 2016), could potentially be exposed to less saline surface waters. Temperature and salinity gradients (density gradients) are commonly present in these coastal waters, hence, vertically migrating L. pertusa larvae in coastal areas can be exposed to density gradients. Larvae from deeper L. pertusa populations may also encounter density gradients since water mass boundaries are present in the deep ocean interior especially in more southerly latitudes (e.g., Stewart, 2008). The presence of haloclines has been found to inhibit vertical migration of other marine larvae (Sameoto and Metaxas, 2008).
Here, we describe the responses of L. pertusa larvae to various environmental conditions with the aim to advance our understanding of larval behavior and its consequences for dispersal. The length of the PLD and vertical distribution during the pelagic phase was deduced by observations of vertical migration pattern, response to encounters with haloclines, and survival in different salinities. In addition, planulae were introduced to various possible food sources with the further aim to assess the importance of larval feeding considering the achievement of competency for settling and metamorphosis.
Materials and Methods
Collection and Rearing
Samples of L. pertusa for larval breeding were collected from 108 to 117 m depth by means of remotely operated vehicle (ROV, Ocean Modules V8 Sii) from the Tisler reef in Norway on November 20, 2014. All necessary permits were in place: the Ytre Hvaler National Park Board and the Norwegian Directorate for Nature Management permit for coral collections, 2010/107-432.3; 2013-46; the Norwegian Fisheries Directorate CITES export permit EX-23-2014, and the Swedish Board of Agriculture CITES import permit Dnr: 4.10.18-10009/14 Nr: 51200-14.
Corals were maintained in four 18 L tanks with a flow-through of filtered seawater (5 μm Ametek polypropylene cartridge). Temperature was kept close to in situ values for the season (7–8°C) and salinity usually had a range of 33–34 psu. Corals were fed twice a week with homogenized Calanus sp. copepods (CALANUS AS, Norway). Spawning occurred at several occasions between January 22 and February 23, 2015, and embryos were maintained in large 2.5 L glass bowls. Once the embryos had developed into swimming planulae they were transferred to 1–3 L glass or polycarbonate E-flasks that were either kept with the wide bottom up, or laid down, since larvae tend to gather in the uppermost layer of water. Some results from previous spawning seasons are also included in this paper, and collections and maintenance of those corals and larvae are described in Larsson et al. (2014).
Larval Development and Survival under Different Environmental Conditions
With the aim to investigate the effect of different environmental conditions on embryo and larval development and survival, and the possible effect of different feeding regimes on metamorphosis and settling, a series of experiments were set up. The larvae were exposed to various salinities and potential food sources. Small standard culture flasks of 64 or 72 mL (Nunclon™Δ) were used for the trials. Each experiment was run on a separate batch of embryos or larvae.
Development Rate at Increased Rearing Temperature
In order to increase the fertilization rate in a batch of gametes with low sperm concentration, two 2.5 L glass bowls with newly released eggs and sperm were maintained on a shaking table with gentle sideways movements. Normal rearing temperature was at 7–8°C, but the water temperature in the bowls rose to 11–12°C, most likely due to the turbulence and induced friction as water moved along the boundaries of the bowl. The developing embryos were kept on the shaking table and development rate was checked at 3 and 8 days after spawning.
Survival in Different Salinities
Larvae were reared in 34, 31, 28, and 25 psu, starting with 17-days-old larvae. The highest salinity was reached by adding salt (Red Sea Coral Pro SeaSalt) to the seawater from the flow-through system (32.8 psu at the time), and the lower salinities by dilution with tap water. Ten larvae were placed in each of three replicate flasks per treatment and checked at 14, 19, and 82 days into the experiment (larval ages of 31, 36, and 99 days, respectively), and once a month thereafter.
Survival in Surface Water
In complement to the above, larvae were exposed to natural surface water collected from the docks outside the lab. The water was filtered through a 60-μm sieve to remove larger plankton (e.g., copepod nauplii), but smaller plankton was left as a potential food source for the planulae. The experiment started March 13 when larvae where 18 days old. The salinity of the surface water was 28.5 psu at the time. Twenty larvae were placed in each of 3 flasks containing surface water, and corresponding controls were set up using filtered deep seawater (5 μm Ametek cartridge filter) with a salinity of 33–34 psu. Larvae were checked twice a week for signs of metamorphosis. The water in the flasks was refreshed and larvae counted at 12, 25, 38, 49, 66, and 81 days into the experiment (larval ages of 30, 43, 56, 67, 84, and 99 days, respectively). The salinity of the surface water was lower (25–27 psu) during the weeks following the start of the experiment, and it was therefore mixed with filtered deep seawater to keep the salinity of the treatment constant at 28.5 psu throughout the experimental period. After 81 days only a few larvae in one of the surface water flasks were still alive and the water in this flask was then changed to deep seawater. From 81 days and onward the flasks were only checked once a month for presence of live planulae, and the water was not exchanged.
Development and Survival under Different Feeding Regimes
Larvae from the age of 14 days were studied under various nutritional conditions. The larvae were fed with five different potential foods: (1) microalgae from algal culture (mixture of 50 μL Isochrysis galbana and 50 μL Rhodomonas salina); (2) the fine fraction of homogenized and centrifuged Calanus sp. copepods (50 μL); (3) fish blood (50 μL); (4) coral nutrition concentrate Ultra Min D (0.1 ppm), and (5) Ultra Min S (0.1 ppm) (Fauna Marine GMBH). The coral nutrition contains dissolved amino acids, vitamins, and trace elements, with Ultra Min D developed especially for azooxanthellate corals. These nutrient solutions are commonly used to feed tropical corals in aquaria. The fish blood was extracted from a whiting (Merlangius merlangus) and a cod (Gadus morhua). Two replicate flasks per food source were set up containing 25 larvae each; there were also 2 control flasks containing filtered (5 μm) deep seawater (33–34 psu) with no food added. Larvae were visually inspected twice a week for survival and signs of metamorphosis. After 14 days the water was exchanged, and after 39 days the feeding was ended and the water in all flasks with live planulae was changed to filtered deep seawater whereafter only survival was monitored. The water was changed after 53, 70, and 85 days. Number of larvae in the flasks was counted at day 14, 39, 53, 70, and 85 (larval ages of 28, 53, 67, 84, and 99 days, respectively), and after that the flasks were only checked once a month for presence of live planulae.
Larval Behavior in Response to Food
Swimming Behavior
To test behavioral responses to different types of food, ten 72 mL standard culture flasks (Nunclon™Δ) were filled with filtered (5 μm) seawater and 25 14-days-old planulae added to each flask. Two flasks were used as controls, with no food added (NO), and to the remaining flasks four different food types were added: fine fraction copepod homogenate (COP); microalgae (MA); fish blood (FB); and, a combination of Ultra Min S and D (SD). Two flasks were set up for each food type. The microalgae were a combination of I. galbana (size: c. 5 μm) and R. salina. (c. 10–20 μm). 1 mL of a mixture of Ultra Min S and D were added to 100 mL of filtered (5 μm) seawater to produce a stock solution, from which 1 mL was added to the culture flasks. To be able to see the microalgae and dissolved nutrients, a small amount of dye (Rhodamine 6G) was added to the suspensions.
To analyze behavioral response to food, planulae were filmed in the 72 mL culture flasks (65 × 40 mm surface area, 20 mm depth, visual frame on images 59 × 33 mm). Recordings were made at 25 Hz with a Canon Eos 5D Mark II equipped with a Canon EF100 mm macro lens (f/2.8L), mounted on a stand at a fixed distance from the table surface and culture flasks. Two Canon flashlights (Speedlite 320EX) on video mode (LED lights) were set up to give lateral light sources, and the flasks were put in a tray with a bottom cover of water and a matte black plastic sheet underneath to provide a black background. A ruler and other reference points were used to be able to set the scale at the time of analysis.
The 14-days-old planulae were filmed in their respective flasks with the controls (no food) and different food types filmed separately. In addition, three replicate flasks (25 planulae per flask) were filmed at larval ages of 34, 43, and 50 days, for approximately 5 min each. Filming was done first without food, and then again after adding food to the same flasks in a repeated measures design. Only fine fraction copepod homogenate was used as food treatment for these older larvae. Healthy planulae for all recording sessions were collected from large culture E-flasks and randomly distributed over the experimental flasks soon before filming. Between recording sessions with the older larvae, the flasks were stored in a constant temperature room set at 7–8°C. Soon after the second take, with food, the water in the culture flasks was changed. The temperature during filming was monitored in a reference flask kept close to the flask being filmed. Starting temperature was approximately 8°C, and rising to 11°C during filming. We had no means of keeping the temperature constant while filming, however, conditions were approximately the same for all larvae. The results from the control treatments (no food) were also used to analyze swimming velocity depending on larval age. From the resulting videos, three subsamples of 10 s each were extracted and larval movements were tracked manually in ImageJ/Fiji (Schindelin et al., 2015) with the MTrackJ plug-in (Meijering et al., 2012). For each track, the average swimming velocity and the sum of angular change in swimming direction (∑Δdegree) was quantified. The ∑Δdegree was calculated from MTrackJ points data where the change in the angle between consecutive pairs of points of the track was squared and summed up for each track. The square root of the sum was then entered into the dataset for each individual planula.
Observations under Light Microscopy
To get more qualitative observations of larval behavior and food handling, larvae were studied under an Olympus Stereo Microscope SZX10, and an Olympus BX51 Light Microscope (LM) equipped with an Olympus DP70 camera. Glass embryo bowls, glass petri dishes, or homemade small chambers made of a slice of plastic tubing with an 8-mm inner diameter glued onto a glass slide, were used under the stereomicroscope. Under LM, regular glass slides with coverslips were used. The coverslips were given small feet of modeling clay to avoid squashing the larvae. In addition to the above described food types, picoplankton was also tested. The picoplankton was cultured from small amounts of copepod residue in seawater, producing mostly rod-shaped bacteria.
Larval Swimming in Response to Salinity Gradients
Larval response to horizontal salinity gradients was studied in specially designed aquaria made of Plexiglas panels, with a long slit at the bottom for gently filling of higher salinity water below a layer of lower salinity water. Experiments were performed in February 2013, and in March 2015.
In the February 2013 experiments, salinity gradients were set up in a 10 × 10 × 10 cm aquarium. The swimming behavior of 27-days-old larvae was monitored in response to single salinity gradients of different strengths in three consecutive trials. The salinity of the bottom layer was the same as the salinity of the intake water of the flow-through system at the time of the experiments (32.9–33.0 psu, 7.5°C). For the establishment of the lower salinity top layer, filtered seawater was diluted with tap water to 31.9, 29.7, and 28.1 psu, giving a difference (Δ) of 1, 3, and 5 psu between layers. The aquaria were filled to a height of 4 cm with the top layer salinity water, after which the bottom layer was added until the total height of water was 9 cm. The bottom layer water was stained with a low concentration of food color (2 drops in 700 mL) for clear distinction of the layers, and the resulting halocline (concentration gradient) was c. 5 mm thick for the 1 psu gradient, and very sharp (<1 mm) for the 3 and 5 psu gradients. Larvae were added, 10 at a time, within 1 cm from the bottom of the experimental aquaria using long glass pipettes. For each of the salinity gradients (Δ1, Δ3, Δ5 psu) three replicate rounds were run, giving a total of 30 larvae per gradient and a grand total of 90 larvae. The travel time and behavior was noted.
In the March 2015 experiment, two consecutive trials with 10 larvae each were done in an 11 × 6 × 21 (L × W × H) cm aquarium. The larvae were 36-days-old and three salinity layers were set up, each 6 cm thick, with 33.6 (bottom), 31.5 (mid), and 29.5 psu (top layer) (7.5°C). Larval behavior was recorded with the Canon Eos 5D, and their movements were tracked manually in ImageJ/Fiji with the MTrackJ plug-in. Tracks were traced both for the full transport from bottom to surface, but also split in two 60-s-sections covering the passage through the gradients to get more detail in the movements around the gradient interface.
Statistical Analyses
Swimming velocity depending on age was analyzed with a 1-way ANOVA with four levels of the factor age (14, 34, 43, and 50-days-old planulae). The controls in the response to food experiments were used and there were two replicate flasks for the 14-days-old, and three replicates for the remaining age groups.
1-way ANOVA's were run on the 14-days-old planulae exposed to different types of food, with two replicate flasks for each of the 5 treatments (including controls). Differences in swimming velocity and sum of angular change in swimming direction (∑Δdegree) were tested in separate analyses.
The feeding trials on the older planulae (34, 43, and 50 days old) were analyzed by repeated measures ANOVA with the formula.
The within effects factor (W) was “food” (no food vs. food, 2 levels, 1 df), the between effects factor (B) was “age” (34, 43, and 50 days, 3 levels, 2 df). As “subjects” the unit of flask (3 replicates per treatment) was used. Separate analyses were done with the response factor Y as swimming velocity (mm s−1), and sum of angular change (∑Δdegree).
The three statistical tests described above were all done within the experiment in section 2.3.1, swimming behavior in response to food. All analyses were done in the statistical software R with the RStudio interface (version 3.2.3, R Core Team, 2015; R Studio Team, 2015).
Results
Larval Development and Survival under Different Environmental Conditions
Development Rate at Increased Rearing Temperature
Embryo development in the bowls kept on a shaking table at a rearing temperature of 11–12°C was close to twice as fast compared to those reared at 7–8°C. Already 3 days after spawning the embryos were ciliated swimming blastulae, compared to 5 days at 7–8°C, and gastrulation had already started. At 8 days, some larvae had developed an oral pore, which is usually not present until after14 days for larvae reared at 7–8°C.
Survival in Different Salinities
Unfortunately, by the second day larvae in all salinities were observed to be in bad condition, likely due to an infestation of parasites, and most of the larvae died soon after the start of the experiment. However, two larvae in each of two 25-psu flasks and one larva in each of two 28-psu flasks were alive at 99 days of age.
Survival in Surface Water
For larvae reared in surface water with naturally occurring particulate organic matter, microalgae, and smaller zooplankton, survival was comparable to in the controls for the first 25 (flask a) and 49 (flask b) days before the cultures suddenly crashed and larvae died (Figure 1A). Larvae in surface water flask c showed a more gradual deterioration, and when 99 days old, survival was lower than in any of the three control flasks with filtered deep water (Figure 1A). Only looking at larvae in the all-together 5 control flasks (including the two controls from the experiment below) with filtered high-salinity water, the average survival over 3 months was close to 60%.
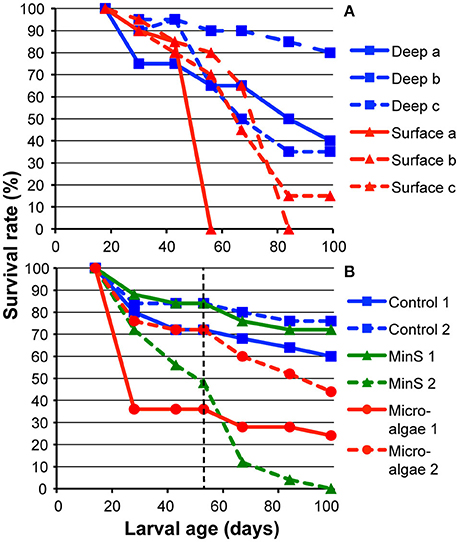
Figure 1. Survival rates of L. pertusa larvae treated in culture flasks at 7–8°C. Controls contained filtered (5 μm) deep-sea water with a salinity of 33–34 psu. (A) Survival of larvae from 18 days old in filtered deep-sea water and surface water of 28.5 psu. The surface water was filtered to 60 μm hence containing naturally occurring microalgae and smaller zooplankton. (B) Survival of larvae from 14 days old in deep-sea water with potential nutritional sources in form of dissolved amino acids and microalgae. The larvae were fed until 53 days old when feeding ended (indicated by dashed line), and water was changed to deep-sea water in all flasks.
Development and Survival under Different Feeding Regimes
Larvae fed with fish blood and homogenized copepods were doing well for the first 14 days (larval age up to 28 days) with survival comparable to those in the controls. But after exchange of water the cultures deteriorated, and when counted after 29 days (larval age: 43 days), only 2 larvae in one of the copepod flasks remained. In one of the Min D flasks, the larvae were observed to be in bad condition already after 2 days and no larvae survived the first 14 days. In the other Min D flask, more than half of the larvae survived for 14 days, but after 39 days (larval age: 53 days) only a few larvae in very bad condition remained; again, the larvae were infested with parasites. There were no morphological signs of metamorphosis into a distinctive settling form of larvae in any of the food treatments. The number of larvae in controls, Min S, and microalgae treatments was monitored until 99 days old (Figure 1B). In total, survival was highest in the controls, and in the Min S treatment there was a large variation between flasks. Average survival of larvae fed with microalgae was lower than in controls (Figure 1B).
From 99 days of age, all flasks with remaining larvae from all three of the above larval experiments were checked once per month for the presence or absence of live planulae. In total, there were 13 flasks containing live planulae at this point and that number decreased gradually during the following months. Twelve months after spawning, two larvae in the previous Min S flask (remember this treatment was ended when larvae were 53 days old) were still alive. In the 25-psu water larvae survived for 8 and 10 months respectively (one in each flask) indicating L. pertusa planulae can survive for months also in relatively low salinity water. The larvae became more and more inactive with age and were during the last months not seen actively swimming unless flasks were gently rotated; otherwise larvae rested at the bottom or on a wall. The 12-month-old larvae were observed in the flask under a stereomicroscope. The larvae were rather small at that point, only 100 μm long. The larvae looked transparent, with only a small opaque core, indicating that energy reserves were running low.
Larval Behavior in Response to Food
Swimming Behavior
There was a weak tendency of increased swimming velocity with age (14, 34, 43, and 50 days) [1-way ANOVA, F(3, 7) = 1.78, p = 0.24]. The mean velocity in 14-days-old was 0.55 mm s−1, gradually rising to 0.78 mm s−1 in the 50-days-old. The maximum velocity was 0.88 mm s−1, observed in the latter group. The 50-days-old larvae were thus still in a very good condition as deduced by maintained high swimming speed (Figure 2).
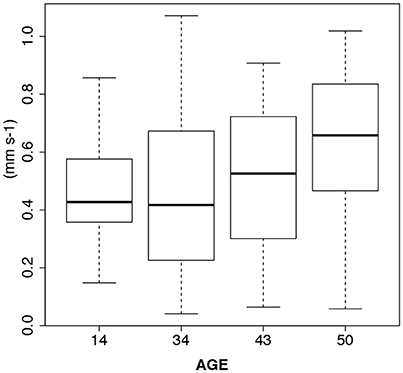
Figure 2. Boxplots of L. pertusa larvae swimming velocity (mm s−1) depending on age (days) in control treatments of the feeding experiments. There is no sign of senescence in the oldest age group.
For the 14-days-old planulae exposed to various types of food (including controls with no food), there was no effect of treatment detected in either swimming velocity [1-way ANOVA, F(4, 5) = 1.30, p = 0.38] or sum of angular change of direction [1-way ANOVA, F(4, 5) = 1.34, p = 0.37] (Figure 3). The planulae given homogenized copepods (COP) had a slightly higher mean sum of angular change of direction due to a few extreme values (Figure 3B).
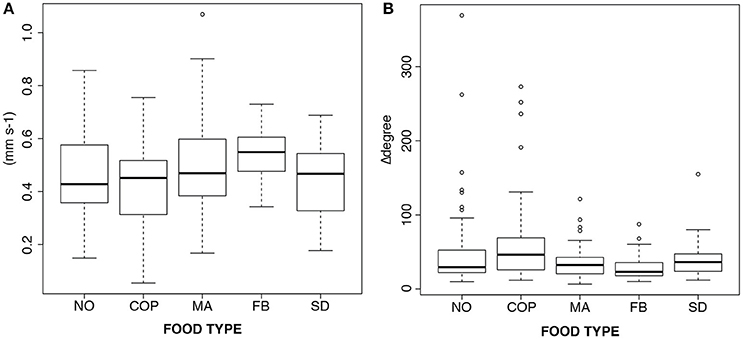
Figure 3. Boxplots of the response to different types of food in 14-days-old planulae. (A) Swimming velocity (mm s−1), and (B) Swimming behavior (sums of change of angles). NO, no food; COP, copepod homogenate; MA, microalgae; FB, fish blood; SD, dissolved amino acids and trace elements.
In 34–50-days-old planulae there was a strong effect of food (copepod homogenate) on swimming behavior (Figure 4). In the figure, box-plots of the response to copepod homogenate in the 14-days-old larvae are also included for comparison, although they were not included in the analysis. The results from the repeated measures ANOVAs show that the swimming velocity decreased significantly after food was added [F(1, 6) = 51.9, p < 0.001, Figure 4A]. There is also an increase in the frequency of change in swimming direction as indicated by the increase in the sum of angular change [F(1, 6) = 96.5, p < 0.001, Figure 4B]. There were no significant interactions between age and food in either analysis.
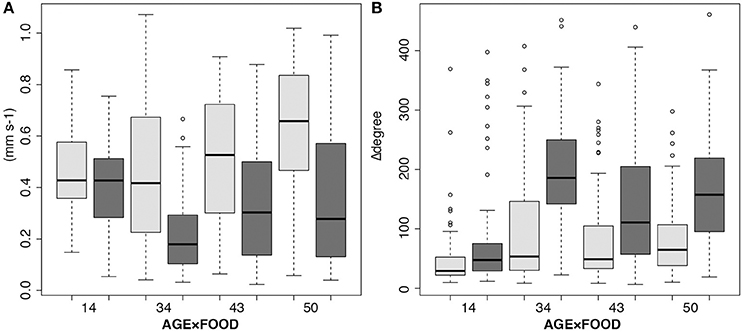
Figure 4. Interaction boxplots showing age-dependent (days) larval response to food (copepod homogenate). The statistical analyses included 34 to 50-days-old planulae, but the 14-days-old are shown in the graphs for comparison. (A) Mean swimming velocity (mm s−1), and (B) swimming behavior (sums of change of angles). Light gray, controls (no food); dark gray, after adding food.
Observations under Light Microscopy
Small particle foods, especially picoplankton, elicited a specific response in the planulae. They stopped swimming, and using ciliary currents they moved particles along the body sides (aboral to oral end), and particles accumulated at the mouth opening. A few particles were observed getting swept into the mouth. With a collection of particles adjacent to the mouth, the planulae resumed swimming, capturing particles in a mucus string that was trailed after the swimming planula. No observations of actual ingestion of the particles in the mucus string were made. As larvae were still, only beating the cilia to move particles along the body, the cilia were radiating outwards from the larval body. The resumed swimming was accomplished by crossing of the long apical cilia, and the larvae were then immediately propelled forward (apical/aboral end first).
Copepod homogenate, picoplankton, and the small size microalgae (Isochrysis galbana, 5 μm) all elicited mucus string production (Figures 5A–C). The larger microalgae (Rhodomonas salina, 10–20 μm) were frequently seen interacting with the larval cilia (Figure 5D) but were simply swept off by the ciliary currents, or swimming off by themselves, never accumulating at the oral opening or eliciting any mucus string production. The fish blood was not observed to elicit any mucus string production, however, the larvae reacted to it by expanding their mouths while remaining still, as if feeding by ciliary movements (Figure 5E). One larva exposed to fish blood was seen shedding a coat of mucus, as if needing to rid itself of contaminants (Figure 5F). Larger particles of copepod tissue could attract several planulae. They would attach to the particles with their oral ends (Figure 5A, inset), and stick to it for long periods. Much of the mortality and deterioration of cultures was caused by a protozoan parasite (Figure 5G shows an infested planula). With every exchange of water there was a risk of introducing the parasites, and therefore we simply stopped changing it.
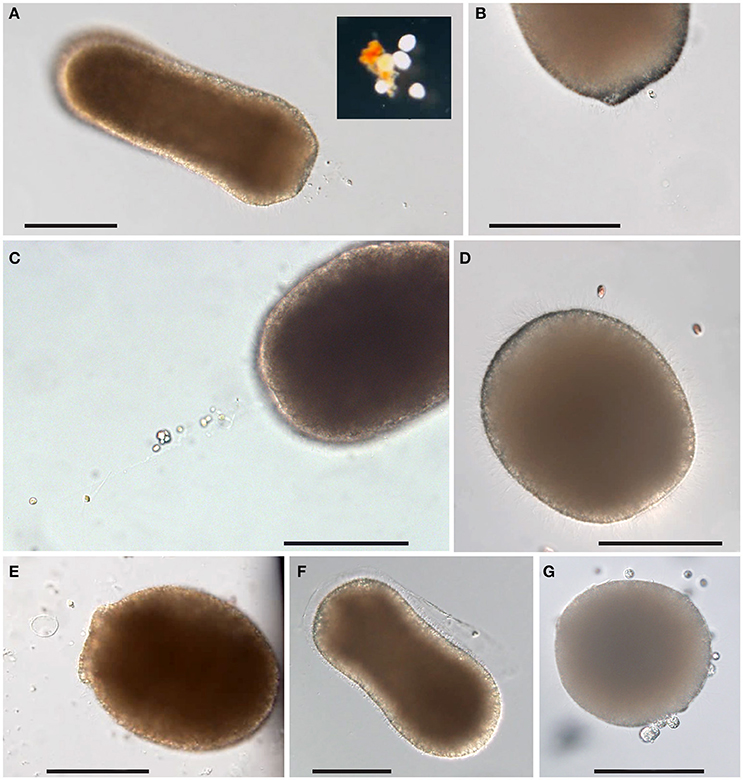
Figure 5. Response of L. pertusa planulae to food treatments. (A) Mucus string in response to copepod homogenate. Inset: a cluster of planulae attached to larger particles of copepod tissues. (B) Mucus string with adhering picoplankton. (C) Mucus string with adhering microalgae (Isochrysis sp.). (D) Larger size microalgae (Rhodomonas sp.) in contact with planula close to the aboral end. (E) A planula with extended mouth in response to fish blood. (F) A planula is shedding a sheet of mucus after exposure to fish blood. (G) Planula infested with parasites. Scale bars 100 μm.
Although L. pertusa planulae seem to be opportunistic feeders, the homogenized copepods were the food type that elicited the greatest response. Copepods are rich in carotenoids, and coloration from that could frequently be seen through the body wall of planulae after feeding. Larvae could be seen reacting to clouds of potential food by halting upon encounter and then resume swimming (Supplementary Video 1 with description in Supplementary Figure 1). Swimming in food was slower and in a more spiral or circular manner than the normal bullet-style swimming (i.e., rotating around the oral-aboral axis, but swimming in a straight line). In flasks where the food remained in a distinct cloud (rather than being spread out evenly in the full volume), larvae swimming outside the cloud were usually swimming in the normal bullet-style, while those within a cloud swam slower and with more frequent change of angle. Detection distance for food presence seemed short, since those that accidentally encountered food reacted to it by an abrupt change of behavior, while other larvae kept swimming in their normal manner in the remaining volume and did not appear to be attracted to the food from a distance (Supplementary Figure 2).
Larval Swimming in Response to Salinity Gradients
When introduced at the bottom of aquaria, all of the 27-days-old larvae (90 in total) in the experiments performed in February 2013, responded by swimming upwards. In all salinity gradients (Δ1, Δ3, Δ5 psu), all larvae passed through the gradient into the overlaying lower salinity layer with no noticeable hesitation or reduction in swimming speed, and continued toward the surface. After 60 min in the experimental aquaria, all larvae were residing at the surface (within 1 cm from the surface), independent of the salinity of the surface layer (32.9, 29.7, 28.1 psu). In all salinities, the larvae were seen swimming seemingly in good condition. The speed of vertical transport toward the surface varied between 0.32 and 0.64 mm s−1. There was no difference in traveling speed in the different salinity layers. In the bottom high-salinity layer the traveling speed was 0.47 ± 0.11 mm s−1 (mean ± SD) in the Δ3-psu trial, and 0.48 ± 0.13 mm s−1 in the Δ5-psu trial. Corresponding traveling speed for the whole distance to the top of the experimental aquaria was 0.47 ± 0.08 mm s−1 in the Δ3-psu trial, and 0.48 ± 0.07 mm s−1 in the Δ5-psu trial.
In the March 2015 experiment, it took 3–4 min for the 36-days-old planulae to ascend from the bottom to the surface. Total length traveled was 147.0 ± 32.3 mm in the first round, and 152.3 ± 40.7 mm in the second (mean ± SD), with a mean velocity of 0.72 ± 0.18 mm s−1 and 0.77 ± 0.23 mm s−1, respectively. The video sequences were 222 and 235 s long in total. There was no apparent reaction, e.g., change in speed or direction, as planulae passed the haloclines (Figure 6). The swimming tracks from the two rounds were very similar so only tracks from the first round are presented. Most planulae ascended without hesitation, and only little sideways movements. Only one planula in round one (Figure 6C) halted at the first halocline, turned and swam downwards again. It later turned and ascended, passing through that first halocline (Figure 6A). In the second round one planula started swimming downwards, but corrected its path and later swam upwards. Planulae did not change their speed during the passage through haloclines: i.e., the segments of the trajectories in the 60-sec-sequences focused at the gradient interface reveal no changes in lengths of the segments in the transition between layers (Figures 6B,C).
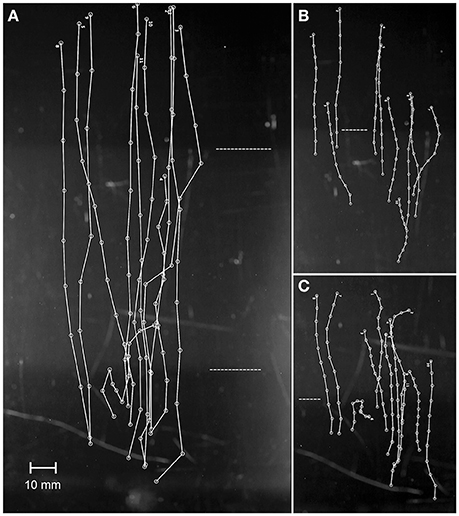
Figure 6. The response of planulae to haloclines, the first trial of two in 2015. Larvae were video recorded shortly after being introduced near the bottom of the aquarium. (A) The complete trajectories from the full video sequence (222 s). (B) Trajectories from the gradient between middle and top layer (60 s). (C) Trajectories from the gradient between bottom and middle layer (60 s). The dashed horizontal lines indicate the haloclines. Visual frame in A = 107.4 × 191.0 mm.
Discussion
The longevity and behavior of Lophelia pertusa planulae in this study indicate high potential for long distance dispersal. Larvae survived for up to a full year under laboratory conditions, even without regular feeding or water change. The maximum lifespan can possibly be even longer since larvae were documented to directly feed on animal derivatives and picoplankton, and were observed to collect smaller sized microalgae in mucous strings. Planulae showed a distinct pattern of ontogenetic shifts in vertical migration, with larvae up to at least 5 weeks of age migrating upwards in the water column and crossing salinity gradients before shifting to a predominantly downwards migration. This behavior together with long-term survival also at lower salinity (25 psu), and the possible feeding on small-size microalgae, suggest that larvae may spend a period of their pelagic phase in the relatively faster currents of the photic zone, significantly impacting both dispersal distance and direction.
Effects of Larval Development Rate and Behavior on Dispersal
The 4–5 weeks old larvae in this study reacted to being introduced at the bottom of an aquarium by consistently swimming upwards, crossing salinity gradients, until reaching the surface. This behavior indicates that L. pertusa planulae might exercise ontogenetic vertical migration, which has previously been detected in other species inhabiting the deep sea (Arellano et al., 2014). Due to low levels of vertical mixing in the deep-sea water column, vertical swimming can be both efficient and necessary for dispersal in the vertical plane. In a study by Ledwell et al. (1993), a tracer sheet released at 310 m depth in the water column vertically spread no more than ±60 m in 5 months. This implies that larvae from deeper L. pertusa populations, once leaving the immediate vicinity of bottom topography where turbulence levels can be enhanced, effectively can travel upwards in spite of their moderate vertical swimming speed of 0.5–0.7 mm s−1 (40–60 m day−1). Our observation of vertical swimming in the larvae is supported in the recent biophysical dispersal study by Fox et al. (2016). Using larval biology data from Larsson et al. (2014), these authors ran a particle-tracking model to predict dispersal of larvae among marine protected areas (MPA's) in the NE Atlantic. The predicted network connections among the MPA's studied were almost non-existing if larvae were treated as passive particles remaining in the deep waters with its weak currents. If larvae on the other hand were given a migrating behavior partly dispersing near the surface, the network connections were much stronger, a result which conformed to the estimated connectivity from genetic studies by Dahl (2013).
In comparison, larvae of two cold-seep mollusc species found in a depth range of 500–2,000 m in the Gulf of Mexico migrate to surface waters (Arellano et al., 2014). These long living larvae feed and grow in the water column where they might reside for more than a year allowing them to take advantage of faster surface currents that can facilitate long-distance dispersal. Young et al. (2012) used physical oceanographic data to model the dispersal of these two species (the mussel “Bathymodiolus” childressi and the gastropod Bathynerita naticoidea) and showed that ontogenetic vertical migration increased the maximum dispersal distance compared to if larvae would reside in deeper waters. Since both the current direction and velocity normally differ with depth (Sponaugle et al., 2002), knowledge of the position of larvae in the water column is crucial for more accurate biophysical modeling of larval dispersal in a specific area. The most direct and accurate way of finding out where in the water column larvae reside is to collect larvae in the field at different depths as done by Arellano et al. (2014). While this is possible, it involves extensive sampling efforts in expensive field surveys, during the period when larvae are in the plankton. Unlike mollusc larvae that wear a shell, deep-sea coral planulae are soft-bodied and prone to disintegrate when filtered out from the seawater. It would therefore demand genetic screening of species-specific DNA sequences to verify presence, a scientific methodology still in its infancy. Amplification and characterization of genomic DNA from tissue of L. pertusa planulae is previously successfully performed (Larsson et al., 2014) indicating such sampling could be feasible.
Contrary to Sameoto and Metaxas (2008) who found that haloclines inhibited vertical migration in larvae of three temperate coastal species, the planulae of L. pertusa in this study showed no noticeable reaction when crossing salinity gradients entering salinities down to 28 psu. Although open ocean surface water usually is fully oceanic in salinity, larvae released from coastal reefs such as those in the Skagerrak or larvae from North Sea populations transported into the Skagerrak (Fox et al., 2016), will likely be exposed to lower salinity surface waters. Hence potentially, the planulae of L. pertusa can reside in the photic zone during part of their pelagic phase as indicated by crossing of haloclines and long-term survival in low salinity water (25 psu). In this study, survival of larvae in cultures with surface water containing naturally occurring particulate organic matter and organisms was lower compared to larvae in filtered (5 μm) deep-sea water (Figure 1A). It is however difficult to know whether this reflects natural conditions since larvae were enclosed in small culture flasks. Outbreaks of parasites caused increased mortality, adding a random factor. The surface water with particles and organisms likely contained higher abundances of bacteria than the filtered seawater. The more sudden deterioration of the surface cultures compared to the filtered seawater cultures (Figure 1A) may indicate bacterial outbreaks. The other cultures containing food particles may have suffered from the same problem.
Besides vertical position in the water column, the length of the pelagic larval duration (PLD) affects the geographic distance larvae will disperse, with in average longer dispersal distance with longer PLD (Siegel et al., 2003; Selkoe and Toonen, 2011; Treml et al., 2015). Considering the chances of larvae settling locally, i.e., in the vicinity of the natal reef, the pre-competency period is more instrumental than the length of the PLD. A short pre-competency period will increase the proportion of larvae recruiting locally (Treml et al., 2015). The general longevity of the planulae observed in the present study suggests the possibility of a very long PLD in this species. There were no signs of senescence in 50-days-old larvae as deduced from maintained high swimming speed, and under control conditions more than half of the post-embryonic planulae survived until 14 weeks of age, and a few larvae survived for a full year. The longer survival time noticed in this study compared to in Larsson et al. (2014) where the maximum longevity was 8 weeks, is supported by biophysical dispersal modeling results from Fox et al. (2016). Their predicted transport of larvae into the North Sea was weak for larvae with a life span of 8 weeks but much greater if the life span was doubled to 16 weeks, which is consistent with the observed distribution and presence of L. pertusa on oil and gas installations in the northern North Sea (Fox et al., 2016). Temperature exerts a significant control of organism metabolism and the long survival of these larvae is most certainly explained by the low rearing temperature (7–8°C) corresponding to approximate in situ temperature at the Skagerrak reefs during the spawning season. An upturn in metabolic rate is also the most likely explanation to the twofold increase in embryo development rate when rearing temperature was increased from 7–8°C to 11–12°C in this study. The gentle water movement caused by the shaking table likely had none to very marginal effects on the development rate since at this low Reynolds number (< < 1, moving with the flow), the effect on the diffusive boundary layer surrounding the embryos should be slight (e.g., Webster and Weissburg, 2009). A faster embryo development rate will in turn shorten the pre-competency period from 3 to 5 weeks (Larsson et al., 2014 and this study) to c. 2 weeks.
The effect of temperature on L. pertusa larval duration remains to be tested but will likely conform to general patterns shared by other marine pelagic larvae. O'Connor et al. (2007) used data from 72 species of marine animals to test the generality of temperature-dependence of larval duration. They found a universally strong reverse effect of temperature on PLD across species, with PLD increasing by over an order of magnitude as temperature varies from tropical conditions (30°C) to cold temperate waters (5°C). Furthermore, Kelly and Eernisse (2007) analyzed mitochondrial DNA data from 28 chiton species along the western coast of North America and found a distinct latitudinal connectivity gradient among species. The species inhabiting higher latitudes generally had less isolated populations and the authors hypothesize that lower sea surface temperatures contribute to longer larval duration and by extension greater connectivity.
Lophelia pertusa is a cosmopolitan species found at various depths and at both low and high latitudes and consequently its embryos and larvae may encounter a large range of temperatures. This species typically thrives at 4–12°C (Rogers, 1999) but surface water temperatures above these habitats will vary much more. The surface temperature in coastal waters of Scandinavia can be between 0 and 6°C (Wisshak et al., 2005) during the weeks after spawning (February-April) when larvae potentially reside in the surface layers. Larvae of L. pertusa populations further south in Europe—e.g., in the Mediterranean—can instead be exposed to temperatures of 20–25°C, or higher. The deep sea is accordingly characterized by water mass boundaries although with less dramatic temperature differences. As a result, both pre-competency periods and PLD likely varies with depth distribution and geographic location and will be different from those found in the present study and in Larsson et al. (2014). In a warming ocean, due to climate change, it can also be expected to see a reduction in dispersal distance and connectivity. In conclusion, with the present knowledge of longevity and vertical migration pattern in L. pertusa planulae, it is important to consider the ambient temperatures at various reef sites and the effect it may have on larval development rate and larval duration. Future studies should beside temperature controlled development rate include finding the survivable extremes in temperature for these larvae.
Larval Traits and Effects on Connectivity Patterns
Estimates of connectivity in L. pertusa through genetic structuring have been conducted on various scales. Among north Atlantic populations, these estimates have given somewhat contrasting results (Le Goff-Vitry et al., 2004; Morrison et al., 2011; Dahl et al., 2012; Flot et al., 2013; Becheler et al., 2017). Le Goff-Vitry et al. (2004) and Morrison et al. (2011) found high levels of inbreeding (heterozygote deficits) in a majority of the populations examined. Le Goff-Vitry et al. (2004) suggested the pattern is caused by spatially restricted gene flow and high rates of local recruitment of sexually reproduced larvae. The hypothesis of high rates of self-recruitment on a site is somewhat conflicting with our observations of L. pertusa larval biology in this study and in Larsson et al. (2014). The length of the pre-competency period (at least 3–5 weeks) and the vertical migration into free-flowing water masses above the seabed do not promote self-recruitment. In Larsson et al. (2014), bottom-probing behavior did not become more common until 5 weeks of age and in the present study both 4- and 5-weeks-old larvae still consistently migrated upwards. Retention of individuals within a natal site decreases primarily with an increase in PLD and pre-competency period (Treml et al., 2015). Also, the longevity of L. pertusa planulae—indicating the possibility of a several months long PLD—speak against high likeliness of self-recruitment. In the dispersal model studies by Fox et al. (2016) and Ross et al. (2017), retention of L. pertusa larvae was evident though. Even when larvae where attributed vertical migration behavior according to the observations made by Larsson et al. (2014), allowing the larvae to disperse with surface currents, self-recruitment occurred in most of the studied sites for more than half of the modeled years (Fox et al., 2016). The properties of L. pertusa planulae thus increase the chances of escaping the natal patch but do not guarantee it. Oceanographic features such as alternating current directions, or larval migration through water masses with different current directions, may cause larvae to end up where they started.
Both Le Goff-Vitry et al. (2004) who investigated sampling sites distributed along the European margin, and Morrison et al. (2011) who sampled four regions in the North Atlantic Ocean, estimated weak to moderate connectivity among populations within regions. Results of both studies also indicate that some larvae are widely dispersed. In contrast, Flot et al. (2013) estimated high connectivity between L. pertusa populations 7,500 km apart in the Mediterranean Sea and the Barents Sea off northern Norway. It should be stressed though that some of these genetic studies may not have tested enough markers to adequately resolve the genetic patterns of interest. High dispersal potential in L. pertusa is also indicated by the rapid and widespread colonization in northern European waters following the last glacial period. From deglacial populations in the Gulf of Cádiz and/or the Mediterranean Sea, colonization of habitats over 7,500 km away took less than 400 years (Henry et al., 2014). Dahl (2013) found weak genetic structuring of L. pertusa at the scale of the north-eastern Atlantic harmonizing well with the biophysical dispersal modeling results in this region by Fox et al. (2016) and Ross et al. (2017), which show that the populations are linked to each other in a connectivity network. Unambiguously, biophysical modeling of larval dispersal is a valuable complement to genetically derived connectivity estimates which will increase our knowledge and understanding of L. pertusa population connectivity.
Estimates of connectivity in other deep-sea coral species using genetic data have shown species specific variation. Miller et al. (2010) assessed connectivity among populations of nine deep-sea coral species on seamounts and slopes in the Australian and New Zealand region, spanning thousands of kilometers. They found conflicting estimates of connectivity with evidence of genetic subdivision among regions for three of the species whereas levels of genetic variation were low for the remaining species. The authors hypothesize that differences in larval traits and behavior, of which virtually nothing is known for the studied species, may explain the observed differences in connectivity patterns among species.
Similar to Miller et al. (2010), Becheler et al. (2017) found contrasting patterns of connectivity between L. pertusa and Madrepora oculata. These two reef-building species often occur together in the northeast Atlantic (Arnaud-Haond et al., 2017) and were here studied in continental slope canyons of the Bay of Biscay. Genetic structuring was detected among the 5 canyons spanning a distance of ~500 km for populations of M. oculata whereas L. pertusa was panmictic on this scale. Since the coral species co-occur in all the canyons studied by Becheler et al. (2017), hence sharing the hydrodynamic environment, and sampling of corals has been conducted in a similar way, it is likely that one or several of the biological parameters important for dispersal of larvae differ between these species. Compared to properties of L. pertusa larvae found in this study, planulae of M. oculata may have a shorter pre-competency period, and/or a shorter PLD, and/or show a different vertical migration pattern (if any). Treml et al. (2015) identified key biophysical drivers of connectivity among marine populations, using a subtidal rocky reef ecosystem as a model. The three parameters consistently found to be most influential on population connectivity were larval mortality during the dispersal phase followed by PLD and the extent of the pre-competency period. For both median and maximum geographical distance of downstream connectivity, PLD was most important. The time of spawning also had considerable impact on connectivity outcomes due to e.g., differences in hydrodynamic conditions among seasons (Treml et al., 2015), and may additionally explain the differences in connectivity found by Becheler et al. (2017) since L. pertusa reproduces seasonally whereas M. oculata produces multiple cohorts annually (Waller and Tyler, 2005; Pires et al., 2014). Hopefully the larval biology of many more deep-sea coral species can be studied in the future to help explain such differences in connectivity patterns.
Larval Behavior in Response to Food
When exposing 14-days-old planulae to different food types, they showed no signs of interest in the food as deduced by the lack of difference in swimming behavior compared to control conditions (Figure 3). A probable explanation is that planulae at this age have just opened the oral pore, as observed in previous work (Larsson et al., 2014), and is not yet capable of ingesting food. In older planulae (34–50 days old), fed solely with copepod homogenate, the results clearly show that food presence elicit a specific feeding response with slower swimming speed and more frequent changes of direction (Figure 4). Feeding was corroborated by the observations of carotenoid coloration inside the gut of the planulae after feeding. The tendency of planulae to attach to larger food particles by their mouths suggests that they can benefit from food scraps from other marine animals' sloppy feeding, or perhaps from dead organisms decomposing in the water column. Additional experiments testing flocculate particulate matter (POM), so called marine snow, as a food source would be interesting.
The aim was to continue the filming of larval feeding behavior, testing all food types, but due to the parasite outbreaks the stock of healthy larvae were running low, and we therefore chose to continue using only copepod homogenate. Other food types were, however, tested in direct observations under stereomicroscope, and both small size microalgae and picoplankton was found to elicit mucus string production, as well as the copepod homogenate. Actual ingestion of the mucus strings was never confirmed. Ingestion of mucus strings has previously been observed in the planulae of the temperate cup coral Caryophyllia smithii (Tranter et al., 1982), in the tropical coral Fungia scutaria (Schwarz et al., 1999), and in sea anemone planulae (Schwarz et al., 2002). In all three of these studies the planulae were fed homogenates of crustaceans (Artemia or other) and planulae reactions to the introduction of food were immediate and voracious, eliciting both feeding by ciliary movements, expansion of the mouth, and mucus string production and ingestion. Interestingly, in the study of Schwarz et al. (2002), a diet of microalgae, or even Symbiodinium californium (the dinoflagellate symbiont) did not elicit feeding behavior on its own account in the planulae of the sea anemone Anthopleura elegantissima. The symbionts were instead ingested together with animal-derived tissues.
Beside copepod homogenate, the only actual observation of food ingestion in the present study was of picoplankton, although not via mucus strings, but via ciliary motion. The rod-shaped bacteria in the picoplankton, cultured from copepod residue, could be an especially beneficial form of bacteria for coral planulae. Since the bacteria seem specialized in breaking down copepod tissue, they should make good bacterial symbionts for the larval gut, and subsequently in the adult polyps' gastrovascular cavity, and aid in nutrient turnover. The affinity for larvae to feed on these bacteria could thus be a means of picking up symbionts rather than feeding on the bacteria themselves, just like planulae of zooxanthellate corals and sea anemones acquire their symbionts by ingestion (Schwarz et al., 1999, 2002).
The adult L. pertusa polyps are opportunistic feeders capable of exploiting different food sources including zooplankton, microalgae (in the form of marine snow, POM), bacteria, and dissolved organic carbon and other nutrients (Mueller et al., 2014). It seems as though the planulae share the opportunistic heterotrophic diet of the adults. The photic zone and water layers immediately beneath is likely where the highest concentration of high quality food can be found, and the interest in small size microalgae suggests that larvae might feed in the photic zone. Beside the possibility to pick up symbiotic bacteria by feeding, the larval energy reserves may be filled resulting in a life span even longer than that documented in the present study. Feeding may however also in average decrease the PLD if ingestion of certain nutrients is a prerequisite for gaining competency for settling and metamorphosis as competent larvae are expected to descend toward the sea floor. The question whether feeding is a prerequisite for achieving competency for metamorphosis was meant to be resolved through the test of survival and development under different feeding regimes. During this specific experiment, no metamorphosis was noticed but signs thereof were observed in some fed planulae in the experiments where larval swimming behavior in response to food was documented. The planulae had a bulging edge adjacent to the mouth, indicating onset of tentacle bud formation (Strömberg et al., 2018). Thus, it seems as if planulae start metamorphosis before settling. Development of mesenteries and even tentacles, or tentacle buds, prior to settling is common within anthozoan planulae (Martin and Koss, 2002). Our planulae were mainly fed copepod homogenate, so further testing needs to be done with other food types to fully resolve the question.
Conclusions
Planulae of the cold-water coral L. pertusa exhibit traits that indicate high potential for long distance dispersal. A pre-competency period of minimum 3–5 weeks with continuous upwards migration into free-flowing currents during this time will increase the chances of escaping the natal spot. The willingness to cross salinity gradients and survival for many months also in water of coastal surface salinity will enable long distance transportation in the relatively faster surface currents. We also observed that embryo development rate increased with raising temperature which will decrease the pre-competency period. This implies the dispersal potential of L. pertusa is depending on ambient temperatures at and above the reef sites and is expected to vary with latitudes. Our observations can be fed into biophysical dispersal models significantly increasing the accuracy of predicted dispersal and connectivity in this coral.
For management purposes, and for the design of marine protected areas, knowledge about the level of exchange of individuals among geographically separated populations is fundamental. Up to recently (see Cardona et al., 2016; Fox et al., 2016), estimates of connectivity among deep-sea coral populations have exclusively been based on studies of genetic structure. One difficulty with such estimations is to discern between historical and present connectivity since individuals of reef building cold-water corals like L. pertusa through colonial growth and asexual reproduction through fragmentation can be very long-lived (thousands of years). As a result, genetic connectivity estimates could mirror historical patterns with extinct stepping-stone populations that previously connected geographically distant populations, and establishing MPA networks based only on such connectivity estimates can be fatal. Clearly, complementing genetically derived connectivity estimates with biophysical modeling of larval dispersal, and intrinsic traits and behavior of the propagules, will increase our knowledge and understanding of population connectivity and its regulating mechanisms in deep-sea coral populations.
Author Contributions
SS and AL conceived and designed the experiments, SS and AL performed the experiments, SS analyzed the data, SS and AL interpreted the data and SS and AL wrote and critically reviewed the manuscript.
Funding
This work was financed by the Swedish Research Council FORMAS through contracts 2010-1604 and 215-2012-1134, with additional contributions from the Colliander Foundation (Rådman och Fru Ernst Collianders stiftelse för välgörande ändamål).
Conflict of Interest Statement
The authors declare that the research was conducted in the absence of any commercial or financial relationships that could be construed as a potential conflict of interest.
Acknowledgments
We are grateful to Jon Havenhand for assistance with the statistical analyses of swimming behavior, and to Marina Panova and Mikael Dahl for fruitful discussions about interpretation of genetic connectivity data.
Supplementary Material
The Supplementary Material for this article can be found online at: https://www.frontiersin.org/articles/10.3389/fmars.2017.00411/full#supplementary-material
References
Aiken, C. M., Navarrete, S. A., Castillo, M. I., and Castilla, J. C. (2007). Along-shore dispersal kernels in a numerical ocean model of the central Chilean coast. Mar. Ecol. Prog. Ser. 339, 12–24. doi: 10.3354/meps339013
Arellano, S. M., Van Gaest, A. L., Johnson, S. B., Vrijenhoek, R. C., and Young, C. M. (2014). Larvae from deep-sea methane seeps disperse in surface waters. Proc. R. Soc. B 281:20133276. doi: 10.1098/rspb.2013.3276
Arnaud-Haond, S., Van den Beld, I. M. J., Becheler, R., Orejas, C., Menot, L., Frank, N., et al. (2017). Two “pillars” of cold-water coral reefs along Atlantic European margins: prevalent association of Madrepora oculata with Lophelia pertusa, from reef to colony scale. Deep Sea Res. II. 145, 110–119. doi: 10.1016/j.dsr2.2015.07.013
Becheler, R., Cassone, A. L., Noël, P., Mouchel, O., Morrison, C. M., and Arnaud-Haond, S. (2017). Low incidence of clonality in cold water corals revealed through the novel use of a standardized protocol adapted to deep sea sampling. Deep Sea Res. II. 145, 120–130. doi: 10.1016/j.dsr2.2015.11.013
Brooke, S., and Järnegren, J. (2013). Reproductive periodicity of the scleractinian coral Lophelia pertusa from the Trondheim Fjord, Norway. Mar. Biol. 160, 139–153. doi: 10.1007/s00227-012-2071-x
Cardona, Y., Ruiz-Ramos, D. V., Baums, I. B., and Bracco, A. (2016). Potential connectivity of coldwater black coral communities in the northern Gulf of Mexico. PLoS ONE 11:e0156257. doi: 10.1371/journal.pone.0156257
Coelho, M. A. G., and Lasker, H. R. (2016). Larval behavior and settlement dynamics of a ubiquitous carribean octocoral and its implications for dispersal. Mar. Ecol. Prog. Ser. 561, 109–121. doi: 10.3354/meps11941
Corell, H., Moksnes, P. O., Engqvist, A., Döös, K., and Jonsson, P. R. (2012). Depth distribution of larvae critically affects their dispersal and the efficiency of marine protected areas. Mar. Ecol. Prog. Ser. 467, 29–46. doi: 10.3354/meps09963
Cowen, R. K., and Sponaugle, S. (2009). Larval dispersal and marine population connectivity. Annu. Rev. Mar. Sci. 1, 443–466. doi: 10.1146/annurev.marine.010908.163757
Dahl, M. P. (2013). Conservation Genetics of Lophelia pertusa [dissertation thesis]. University of Gothenburg.
Dahl, M. P., Pereyra, R. T., Lundälv, T., and Andr,é, C. (2012). Fine-scale spatial genetic structure and clonal distribution of the cold-water coral Lophelia pertusa. Coral Reefs 31, 1135–1148. doi: 10.1007/s00338-012-0937-5
Davies, A. J., and Guinotte, J. M. (2011). Global habitat suitability for framework-forming cold-water corals. PLoS ONE 6:e18483. doi: 10.1371/journal.pone.0018483
Fiksen, Ø., Jørgensen, C., Kristiansen, T., Vikeb,ø, F., and Huse, G. (2007). Linking behavioural ecology and oceanography: larval behaviour determines growth, mortality and dispersal. Mar. Ecol. Prog. Ser. 347, 195–205. doi: 10.3354/meps06978
Flot, J. F., Dahl, M., and André, C. (2013). Lophelia pertusa corals from the Ionian and Barents seas share identical nuclear ITS2 and near-identical mitochondrial genome sequences. BMC Res. Notes 6:144. doi: 10.1186/1756-0500-6-144
Fosså, J. H., Mortenssen, P. D., and Furevik, D. M. (2002). The deep-water coral Lophelia pertusa in Norwegian waters: distribution and fishery impacts. Hydrobiologia 471, 1–12. doi: 10.1023/A:1016504430684
Fox, A. D., Henry, L. A., Corne, D. W., and Roberts, J. M. (2016). Sensitivity of marine protected area network connectivity to atmospheric variability. R. Soc. Open. Sci. 3:160494. doi: 10.1098/rsos.160494
Gass, S. E., and Roberts, J. M. (2006). The occurrence of the cold-water coral Lophelia pertusa (Scleractinia) on oil and gas platforms in the North Sea: colony growth, recruitment and environmental controls on distribution. Mar. Pollut. Bull. 52, 549–559. doi: 10.1016/j.marpolbul.2005.10.002
Henry, L.-A., Frank, N., Hebbeln, D., Wienberg, C., Robinson, L., de Flierdt, T., et al. (2014). Global ocean conveyor lowers extinction risk in the deep sea. Deep Sea Res. I 88, 8–16. doi: 10.1016/j.dsr.2014.03.004
Hilário, A., Metaxas, A., Gaudron, S., Howell, K., Mercier, A., Mestre, N., et al. (2015). Estimating dispersal distance in the deep sea: challenges and applications to marine reserves. Front. Mar. Sci. 2:6. doi: 10.3389/fmars.2015.00006
Kelly, R. P., and Eernisse, D. J. (2007). Southern hospitality: a latitudinal gradient in gene flow in the marine environment. Evolution 61, 700–707. doi: 10.1111/j.1558-5646.2007.00055.x
Larsson, A. I., Järnegren, J., Strömberg, S. M., Dahl, M. P., Lundälv, T., and Brooke, S. (2014). Embryogenesis and larval biology of the cold-water coral Lophelia pertusa. PLoS ONE 9:e102222. doi: 10.1371/journal.pone.0102222
Le Goff-Vitry, M. C., Pybus, O. G., and Rogers, A. D. (2004). Genetic structure of the deep-sea coral Lophelia pertusa in the northeast Atlantic revealed by microsatellites and internal transcribed spacer sequences. Mol. Ecol. 13, 537–549. doi: 10.1046/j.1365-294X.2004.2079.x
Ledwell, J. R., Watson, A. J., and Law, C. S. (1993). Evidence for slow mixing across the pycnocline from an open-ocean tracer-release experiment. Nature 364, 701–703. doi: 10.1038/364701a0
Martin, V. J., and Koss, R. (2002). “Phylum cnidaria,” in Atlas of Marine Invertebrate Larvae, ed C. M. Young (San Diego, CA: Academic Press), 51–108.
Meijering, E., Dzyubachyk, O., and Smal, I. (2012). Methods for cell and particle tracking. Method. Enzymol. 504, 183–200. doi: 10.1016/B978-0-12-391857-4.00009-4
Miller, K., Williams, A., Rowden, A. A., Knowles, C., and Dunshea, G. (2010). Conflicting estimates of connectivity among deep-sea coral populations. Mar. Ecol. Evol. Persp. 31, 144–157. doi: 10.1111/j.1439-0485.2010.00380.x
Moksnes, P. O., Corell, H., Tryman, K., and Jonsson, P. R. (2014). Larval behavior and dispersal mechanisms in shore crab larvae (Carcinus maenas): local adaptations to different tidal environments? Limnol. Oceanogr. 59, 588–602. doi: 10.4319/lo.2014.59.2.0588
Morrison, C. L., Ross, S. W., Nizinski, M. S., Brooke, S., Järnegren, J., Waller, R. G., et al. (2011). Genetic discontinuity among regional populations of Lophelia pertusa in the North Atlantic Ocean. Conserv. Genet. 12, 713–729. doi: 10.1007/s10592-010-0178-5
Mueller, C. E., Larsson, A. I., Veuger, B., Middelburg, J. J., and van Oevelen, D. (2014). Opportunistic feeding on various organic food sources by the cold-water coral Lophelia pertusa. Biogeosciences 11, 123–133. doi: 10.5194/bg-11-123-2014
O'Connor, M. I., Bruno, J. F., Gaines, S. D., Halpern, B. S., Lester, S. E., Kinlan, B. P., et al. (2007). Temperature control of larval dispersal and the implications for marine ecology, evolution, and conservation. Proc. Natl. Acad. Sci. U.S.A. 104, 1266–1271. doi: 10.1073/pnas.0603422104
Paris, C. B., Chérubin, L. M., and Cowen, R. K. (2007). Surfing, spinning, or diving from reef to reef: effects on population connectivity. Mar. Ecol. Prog. Ser. 347, 285–300. doi: 10.3354/meps06985
Pires, D. O., Silva, J. C., and Bastos, N. D. (2014). Reproduction of deep-sea reef-building corals from the southwestern Atlantic. Deep Sea Res. II 99, 51–63. doi: 10.1016/j.dsr2.2013.07.008
R Core Team (2015). R: A Language and Environment for Statistical Computing. Vienna: R Foundation for Statistical Computing.
Roberts, J. M., Wheeler, A., Freiwald, A., and Cairns, S. D. (2009). Cold-Water Corals: The Biology and Geology of Deep-Sea Coral Habitats. Cambridge: Cambridge University Press.
Rogers, A. D. (1999). The biology of Lophelia pertusa (LINNAEUS 1758) and other deep-water reef-forming corals and impacts from human activities. Int. Rev. Hydrobiol. 84, 315–406. doi: 10.1002/iroh.199900032
Ross, R. E., Nimmo-Smith, W. A. M., and Howell, K. L. (2017). Towards ‘ecological coherence’: assessing larval dispersal within a network of existing marine protected Areas. Deep Sea Res. I 126, 128–138. doi: 10.1016/j.dsr.2017.06.004
Sameoto, J. A., and Metaxas, A. (2008). Interactive effects of haloclines and food patches on the vertical distribution of 3 species of temperate invertebrate larvae. J. Exp. Mar. Biol. Ecol. 367, 131–141. doi: 10.1016/j.jembe.2008.09.003
Schindelin, J., Rueden, C. T., Hiner, M. C., and Eliceiri, K. W. (2015). The ImageJ ecosystem: An open platform for biomedical image analysis. Mol. Reprod. Dev. 82, 518–529. doi: 10.1002/mrd.22489
Schwarz, J. A., Krupp, D. A., and Weis, V. M. (1999). Late larval development and onset of symbiosis in the scleractinian coral Fungia scutaria. Biol. Bull. 196, 70–79. doi: 10.2307/1543169
Schwarz, J. A., Weis, V. M., and Potts, D. C. (2002). Feeding behavior and acquisition of zooxanthellae by planula larvae of the sea anemone Anthopleura elegantissima. Mar. Biol. 140, 471–478. doi: 10.1007/s00227-001-0736-y
Selkoe, K. A., and Toonen, R. J. (2011). Marine connectivity: a new look at pelagic larval duration and genetic metrics of dispersal. Mar. Ecol. Prog. Ser. 436, 291–305. doi: 10.3354/meps09238
Shanks, A. L. (2009). Pelagic larval duration and dispersal distance revisited. Biol. Bull. 216, 373–385. doi: 10.1086/BBLv216n3p373
Siegel, D. A., Kinlan, B. P., Gaylord, B., and Gaines, S. D. (2003). Lagrangian descriptions of marine larval dispersion. Mar. Ecol. Prog. Ser. 260, 83–96. doi: 10.3354/meps260083
Sponaugle, S., Cowen, R. K., Shanks, A., Morgan, S. G., Leis, J. M., Pineda, J. M., et al. (2002). Predicting self-recruitment in marine populations: biophysical correlates and mechanisms. Bull. Mar. Sci. 70, 341–375.
Squires, D. F. (1959). Deep sea corals collected by the lamont geological observatory. 1. Atlantic corals. Am. Mus. 1965, 1–42.
Strömberg, S. M., Östman, C., and Larsson, A. I. (2018). The Cnidome and External Morphology of Late Planulae in Lophelia pertusa (Linnaeus, 1758) – with Implications for Settling Competency. In: Strömberg (2016). Early Life History of the Cold-Water Coral Lophelia pertusa – with Implications for Dispersal, Dissertation Thesis, University of Gothenburg.
Taviani, M., Angeletti, L., Canese, S., Cannas, R., Cardone, F., Cau, A., et al. (2017). The “Sardinian cold-water coral province” in the context of the Mediterranean coral ecosystems. Deep Sea Res. II. 145, 61–78. doi: 10.1016/j.dsr2.2015.12.008
Tay, Y. C., Guest, J. R., Chou, L. M., and Todd, P. A. (2011). Vertical distribution and settlement competencies in broadcast spawning coral larvae: implications for dispersal models. J. Exp. Mar. Biol. Ecol. 409, 324–330. doi: 10.1016/j.jembe.2011.09.013
Tranter, P. R. G., Nicholson, D. N., and Kinchington, D. (1982). A description of spawning and post-gastrula development of the cool temperate coral, Caryophyllia smithii. J. Mar. Biol. Ass. U.K. 62, 845–854. doi: 10.1017/S0025315400070387
Treml, E. A., Ford, J. R., Black, K. P., and Swearer, S. E. (2015). Identifying the key biophysical drivers, connectivity outcomes, and metapopulation consequences of larval dispersal in the sea. Mov. Ecol. 3, 1–16. doi: 10.1186/s40462-015-0045-6
Waller, R. G., and Tyler, P. A. (2005). The reproductive biology of two deep-water, reef-building scleractinians from the NE Atlantic Ocean. Coral Reefs 24, 514–522. doi: 10.1007/s00338-005-0501-7
Webster, D. R., and Weissburg, M. J. (2009). The hydrodynamics of chemical cues among aquatic organisms. Annu. Rev. Fluid Mech. 41, 73–90. doi: 10.1146/annurev.fluid.010908.165240
Wisshak, M., Freiwald, A., Lundälv, T., and Gektidis, M. (2005). “The physical niche of the bathyal Lophelia pertusa in a non-bathyal setting: environmental controls and palaeoecological implications,” in Cold-Water Corals and Ecosystems, eds A. Freiwald and J. M. Roberts (Heidelberg: Springer-Verlag), 979–1001.
Keywords: larval swimming, larval ecology, dispersal, connectivity, ontogenetic shift, vertical migration, competency, planktotrophic
Citation: Strömberg SM and Larsson AI (2017) Larval Behavior and Longevity in the Cold-Water Coral Lophelia pertusa Indicate Potential for Long Distance Dispersal. Front. Mar. Sci. 4:411. doi: 10.3389/fmars.2017.00411
Received: 13 October 2017; Accepted: 30 November 2017;
Published: 12 December 2017.
Edited by:
Anthony Grehan, National University of Ireland Galway, IrelandReviewed by:
Rebecca E. Ross, Plymouth University, United KingdomLorenzo Angeletti, Institute of Marine Sciences, Italy
Copyright © 2017 Strömberg and Larsson. This is an open-access article distributed under the terms of the Creative Commons Attribution License (CC BY). The use, distribution or reproduction in other forums is permitted, provided the original author(s) or licensor are credited and that the original publication in this journal is cited, in accordance with accepted academic practice. No use, distribution or reproduction is permitted which does not comply with these terms.
*Correspondence: Susanna M. Strömberg, c3VzYW5uYS5zdHJvbWJlcmdAbWFyaW5lLmd1LnNl