- Israel Oceanographic and Limnological Research, National Institute of Oceanography, Haifa, Israel
Mesopelagic prokaryotes (archaea and bacteria), which are transported together with nutrient-rich intermediate-water to the surface layer by deep convection in the oceans (e.g., winter mixing, upwelling systems), can interact with surface microbial populations. This interaction can potentially affect production rates and biomass of surface microbial populations, and thus play an important role in the marine carbon cycle and oceanic carbon sequestration. The Eastern Mediterranean Sea (EMS) is one of the most oligotrophic and warm systems in the world's oceans, with usually very shallow winter mixing (<200 m) and lack of large-size spring algal blooms. In this study, we collected seawater (0–1,500 m) in 9 different cruises at the open EMS during both the stratified and the mixed seasons. We show that the EMS is a highly oligotrophic regime, resulting in low autotrophic biomass and primary productivity and relatively high heterotrophic prokaryotic biomass and production. Further, we simulated deep water mixing in on-board microcosms using Levantine surface (LSW, ~0.5 m) and intermediate (LIW, ~400 m) waters at a 9:1 ratio, respectively and examined the responses of the microbial populations to such a scenario. We hypothesized that the LIW, being nutrient-rich (e.g., N, P) and a “hot-spot” for microbial activity (due to the warm conditions that prevail in these depths), may supply the LSW with not only key-limiting nutrients but also with viable and active heterotrophic prokaryotes that can interact with the ambient surface microbial population. Indeed, we show that LIW heterotrophic prokaryotes negatively affected the surface phytoplankton populations, resulting in lower chlorophyll-a levels and primary production rates. This may be due to out-competition of phytoplankton by LIW populations for resources and/or by a phytoplankton cell lysis via viral infection. Our results suggest that phytoplankton in the EMS may not likely form blooms, even after exceptionally deep winter mixing, and therefore have a very small overall effect on the vertical flux of organic matter to the deep sea.
Introduction
Vast regions of the oceans are considered nutrient-limited and are characterized by low rates of new production and an overall low phytoplankton/microbial biomass. Thus, it has been accepted that nutrient availability plays a key role in controlling the marine phytoplankton/microbial biomass, diversity and activity (Arrigo, 2005; Shi et al., 2012). This ultimately determines to a great extent the drawdown potential of atmospheric CO2 by the oceanic biological pump and final carbon sequestration in the deep ocean water masses and sediments (Falkowski, 1997; Raven and Falkowski, 1999; Sisma-Ventura et al., 2016).
The Levantine Basin in the Eastern Mediterranean Sea (EMS) is one of the most oligotrophic marine environments in the world's oceans (Krom et al., 2010; Siokou-Frangou et al., 2010; Herut et al., 2016). Despite the overall increasing trends in terrestrial-based nutrient inputs to the EMS over the past few decades with increasing coastal populations, these loads are currently still relatively low and have a spatially limited impact on the narrow band of shallow coastal water along the boundaries of the basin (Krom et al., 2014). As a result of these conditions, the majority of the EMS basin surface layer water has extremely low levels of nitrogen (nitrate+nitrite, N) and phosphorus (orthophosphate, P) (Krom et al., 2010; Tanhua et al., 2013; Kress et al., 2014; Rahav et al., 2018). Correspondingly, small-size autotrophic and heterotrophic bacterioplankton prevail (Van Wambeke et al., 2011; Rahav et al., 2013a) with low rates of primary production (Rahav et al., 2013b; Pitta et al., 2016; Tsiola et al., 2016). Compared to the nutrient-poor photic layer (0–150 m), N and P levels in the aphotic layers are 2–3 orders of magnitude higher (Tanhua et al., 2013; Kress et al., 2014).
Previous studies from the photic layer of the EMS showed that autotrophic microbial biomass and activity are co-limited by N+P (Kress et al., 2005; Zohary et al., 2005; Tsiola et al., 2016), whereas heterotrophic prokaryotes (archaea and bacteria) are limited by either P (Thingstad et al., 2005; Rahav et al., 2013a; Pitta et al., 2016) or by organic-carbon (Rahav et al., 2016a) during summertime/the stratification period. To date, nearly no information is available on the limiting nutrient/s for autotrophic/heterotrophic microbial biomass and activity during wintertime/the mixed period in the EMS.
In the oceans, convective mixing of the water column is the main mechanism responsible for the massive transport of nutrients to the photic zone that increases phytoplankton biomass and activity. In the EMS, it is generally accepted that winter mixing rarely exceeds 200 m depth (Brenner et al., 1991; D'Ortenzio et al., 2005) and therefore may reach only the very top of the nutricline with relatively small amounts of nutrient transport to the photic layer (Brenner et al., 1991; Zohary et al., 1998). However, deep winter mixing has been observed in the EMS down to depths of ~500 m during extremely cold winters such as that of 1989 and 1992 (Brenner et al., 1991; Zohary et al., 1998) as opposed to ~200 m in ‘typical’ winters in different years such as that of 1990 (Hecht et al., 1988; D'Ortenzio et al., 2005). In these cold winters, the depth integrated chlorophyll a in the mixed water column was 59–70 mg m−2, far below the definition for algal bloom (Smayda, 1997). Contrary, during “typically-warm” winters the depth integrated chlorophyll a was significantly lower; 10–13 mg m−2 (Brenner et al., 1991; Zohary et al., 1998), concurrent with the ultra-oligotrophic nature of the basin (Siokou-Frangou et al., 2010 and references herein) and/or that other factor/s prevent algae to proliferate (i.e., biological interactions, other nutrients than N or P etc.).
To date, very little is known regarding how picophytoplankton and heterotrophic prokaryotes respond to naturally occurring nutrient fluxes in the EMS (e.g., Astrahan et al., 2016; Herut et al., 2016), particularly those that occur due to deep winter mixing (Shi et al., 2012). In this study, we simulated the effect of deep water mixing (as occurs in exceptionally cold winters described above) of Levantine intermediate water (LIW, 400 m) on autotrophic and heterotrophic prokaryotic abundance and production rates in the Levantine surface water (LSW, ~0.5 m) during short-term (2 days) microcosm experiments. Specifically, we evaluated the effect of LIW microbial communities and nutrients on surface water microbial populations. We hypothesized that elevated nutrients from the mesopelagic-rich LIW to the LSW will benefit with the autotrophic and heterotrophic prokaryotic communities that are potentially limited by N+P or P, respectively. We also hypothesized that the LIW, being a hot-spot for microbial activity in the EMS due to its relatively warm temperatures which are ~10°C higher than in any oceanic system of similar depths (Van Wambeke et al., 2011; Luna et al., 2012; Keuter et al., 2015), may also supply to the surface water with viable and active heterotrophic prokaryotes that can interact (whether positively or negatively) with the surface microbial populations.
Materials and Methods
Experimental Design
Water samples were collected on-board the R/V Shikmona and R/V Bat-Galim during nine research campaigns between 2013 to 2017 (March 2013, December 2013, August 2014, March 2015, August 2015, November 2016, March 2017, May 2017 and August 2017) at a the routinely monitored station H05 located 54 km northwest of the Carmel Headland, Israel (Lat. 33° 00.00 N; Lon. 34° 30.00 E). Seawater was sampled at discrete depths between the surface (~0.5 m) and the bottom (~1,500 m) using Niskin bottles (8 L) mounted on a rosette equipped with a Conductivity Temperature Depth (CTD) sensor (Seabird 19 Plus), a fluorometer (Turner designs, Cyclops-7) and an oxygen optode (Seabird SBE 63). Water column samples were analyzed for different physiochemical and biological variables detailed below. The raw data can be freely downloaded in the ISRAMAR website (www.isramar.ocean.org.il). Sampling depth were chosen based on the real-time CTD profile.
Microcosm bioassay experiments were carried out during the winter of March 2014 and the summer of August 2014 at the same location in triplicates in 4.6-L acid-washed polycarbonate bottles with three different treatments. In the first treatment, nutrient enriched seawater from the base of the Levantine intermediate water (LIW, ~400 m, representative of deep water mixing as occurs in extremely cold winters) was added to Levantine surface water (LSW, ~0.5 m) at a ratio of 1:9 (LIW: LSW). This ratio was chosen in order to add “sufficient” amount of nutrients to relieve phytoplankton bottom-up stress on the one hand (~1.4 μM NO3 and ~0.05 μM PO4, Zohary et al., 2005), and to not over dilute the surface water on the other hand (resulting in unmeasurable autotrophic and heterotrophic biomass and production). Further, depth integrated values simulating mixing down to 400 m of NO3 and PO4 agree well with the 9:1 ratio addition treatment concentration (~2 μM NO3 and ~0.07 μM PO4). Thus, the mixing scenario tested is not unrealistic. In the second treatment, the LIW water was first gently pre-filtered (~50 mmHg) onto a 0.2-μm filter and then added to LSW at the same ratio (thus removing LIW's autochthonous bacteria and archaea but retain the dissolved nutrients, F-LIW, Table 1). In the third control treatment, no LIW or F-LIW water was added to the LSW bottles. The differences between the response to LIW addition (nutrients + bacteria + archaea) and the response of the filtered F-LIW addition (nutrients only) were used to determine the potential contribution of deep-water microbes to the measured parameters throughout the incubations. All treatment bottles were incubated for 48 h on-deck in a ~1 m3 flow-through seawater tank in order to maintain the incubation bottles at the ambient sea surface temperature. The water temperature in the tank was recorded on an hourly basis for the entire experiment duration (48 h) using an HOBO Pendant Temperature data logger (model UA-002-64, Onset Computer Corporation). The tank was covered with a neutral density screening mesh to simulate in situ light conditions (Dishon et al., 2012). Subsamples from each of the treatment bottles were taken at 0, 0.5, 24, and 48 h after the beginning of the experiment for different variables detailed below. The addition of LIW to the LSW resulted in a net surface water enrichment of ~1.4 μM N and ~0.05 μM P. Such additions correspond to ~4 fold increase of the ambient N and P (Table 1), and is similar, yet somewhat higher, to that previously reported in the EMS during deep water mixing in the Cyprus Eddy (450 m) vs. its boundary (200 m) (Krom et al., 1991). Furthermore, similarly to our experimental mixing manipulations, the duration of the deep winter mixing in the EMS is usually very short and limited to days to a few weeks (Krom et al., 1992). In addition to nutrients, the unfiltered LIW also included 32.0 × 106 to 61.6 × 106 heterotrophic prokaryotic cells (i.e., the total prokaryotes in the addition of 400 mL of LIW water), 30–40% of which were attributed to high nucleic acid (HNA) cells; ~2-fold higher than their percentage in the surface water (Table 1).
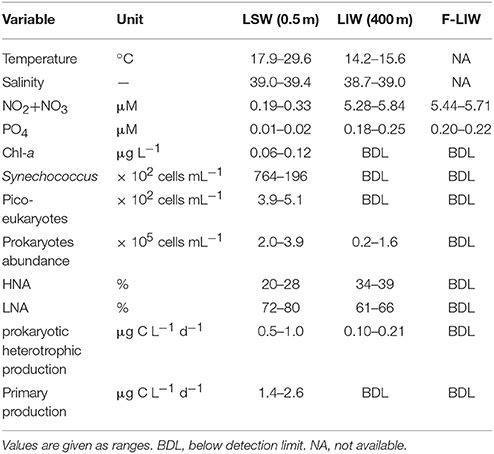
Table 1. The initial chemical and biological characteristics of the LSW, the LIW and the pre-filtered (0.2 μm) LIW (F-LIW) that were used in the bioassays during winter and summer.
Laboratory Analysis
Inorganic Nutrients
Inorganic nutrients samples were kept frozen at −20°C in 15 mL plastic vials until analysis in the Israel Oceanographic and Limnological Research (IOLR) laboratory using a segmented flow Seal Analytical AA-3 instrument (Kress and Herut, 2001; Kress et al., 2014).
Chlorophyll-a (chl-a)
Samples for chl-a were filtered (300–500 mL) on deck through a Whatman GF/F filter (~0.7 μm pore size). The filters were frozen at −20°C for less than a month before analysis at the IOLR laboratory. Chl-a pigments were extracted overnight in cold acetone (90%) in the dark and determined by the non-acidification method (Welschmeyer, 1994) using a Turner Designs (Trilogy) fluorometer.
Pico-Phytoplankton and Bacterial Abundance
Water samples (1.5 ml) were fixed with glutaraldehyde (Sigma-Aldrich G7651), frozen in liquid nitrogen and stored at −80°C until analysis (Vaulot and Marie, 1999). Pico-phytoplankton (Synechococcus and pico-eukaryotes) and heterotrophic prokaryotes abundances (bacteria and archaea) were determined using an Attune® Acoustic Focusing Flow Cytometer (Applied Biosystems) (Bar-Zeev and Rahav, 2015). Note that Prochlorococcus were not considered as their abundance at the surface water of the EMS, where the water for the microcosm experiments were collected from, are negligible (Mella-Flores et al., 2011; Rahav et al., 2013b). The taxonomic discrimination of pico-phytoplankton was based on cell side-scatter (a proxy of cell volume), forward scatter (a proxy of cell size), and orange and red fluorescence of phycoerythrin and chl-a (585 nm and 630 nm, respectively). Prokaryotes were first stained with SYTO®9 and enumerated by discrimination based on green fluorescence (530 nm) and side scatter. The pico-phytoplankton and heterotrophic prokaryotic carbon biomasses were calculated from cell counts, assuming 175 fg C cell−1 for Synechococcus, 2100 fg C cell−1 for pico-eukaryotes and 20 fg C cell−1 for heterotrophic prokaryotes (Campbell and Yentsch, 1989).
Heterotrophic prokaryotes were separated into two distinct groups based on their side scatter (cell density and morphology) and green fluorescence (nucleic acid content) signals and were collectively named high nucleic acid (HNA) and low nucleic acid (LNA) cells (Lebaron et al., 2001). Several studies suggested that HNA prokaryotes are larger and more active than LNA prokaryotes (Servais et al., 1999; Lebaron et al., 2001; Astrahan et al., 2016). Further, a study across the Mediterranean Sea showed that the percentage of HNA prokaryotes increased significantly with depth, with high contribution below the deep chlorophyll maxima (DCM) and the aphotic waters (Van Wambeke et al., 2011), suggesting that HNA cells are an important group to be considered when studying deep-water prokaryotes in this marine system.
Primary Production
Water samples (50 ml) were spiked upon sampling with 5 μCi of NaH14CO3 (Perkin Elmer, specific activity 56 mCi mmol−1), as described in Steemann-Nielsen (Steemann-Nielsen, 1952). The samples were incubated for 4 h under in situ natural illumination and temperature in the flow through tank on deck covered with a neutral density screening mesh. The incubations were terminated by filtering the spiked seawater through GF/F filters (Whatman, 0.7 μm pore size) at low pressure (~50 mmHg). Measurements for the added activity and dark controls (250–500 m) were also performed. The filters were placed overnight in 5 mL scintillation vials containing 50 μl of 32% HCl in order to remove excess 14C after which 3 mL of scintillation cocktail (Ultima-Gold) were added. Radioactivity was measured using a TRI-CARB 2100 TR (Packard) liquid scintillation counter.
Prokaryotic Heterotrophic Production
Prokaryotic heterotrophic production (bacteria and archaea) was estimated using the 3H-leucine incorporation method (Perkin Elmer, specific activity 123 Ci mmol−1), as described in Simon et al. (1990). Three replicates (1.7 mL each) from each water sample/microcosm were incubated in the dark (wrapped in silver foil) with 100 nmol leucine L−1 for 4 h under in situ conditions (Table 1). Preliminary experiments indicated that this was a saturating level of 3H-leucine and that incorporation was linear during this time period (Rahav et al., 2013a,c; Rahav and Bar-Zeev, 2017). A triplicate addition of trichloroacetic acid (TCA) served as control. The incubations were terminated with 100 μL of cold (4°C) trichloroacetic acid (100%) and were later processed following the micro-centrifugation technique (Smith et al., 1992). After adding 1 ml of scintillation cocktail (Ultima-Gold) to each vial, the samples were counted using a TRI-CARB 2100 TR (Packard) liquid scintillation counter. We used a conversion factor of 1.5 kg C mol−1 per every mole leucine incorporated (Simon and Azam, 1989).
Statistical Analyses
The significance of the effect of each treatment combination on the different variables was tested using analysis of variance (ANOVA) followed by a FISHER LSD multiple comparison post hoc test. Prior to analyses, the data was log-transformed to ensure normality distribution of residuals and homogeneity of variance. Eta-squared was calculated as a measure of effect size of factors and co-variables (Tabachnick and Fidell, 2013). The analysis was performed using the Microsoft add-on to excel XLSTAT software. The confidence level of all the analyses was set at 95% (α = 0.05).
Results
Water Column Characterization
The surface layer of the study site differed between winter and summer samplings (Figure S1, Figures 1, 2). In winter, the upper 150–200 m were well mixed, whereas during summer the water column was much warmer and stratified, with maximal water mixing of ~50 m (Figure S1). The surface temperatures during winter and summer were ~15°C and 29°C, respectively (Figure S1). During both seasons, the surface layer salinity was ~39 (Figure S1). The water column was well oxygenated (>170 μM) from the surface to the bottom during both seasons (Figure S1). The mean inorganic nutrient concentrations in the upper 150 m were slightly above the detection limit during both seasons (Figure S1). During winter and summer, the surface layer mean (0–150 m) NO2+NO3 (N) was 0.35 ± 0.08 μM and 0.25 ± 0.07 μM, and PO4 (P) was 0.02 ± 0.02 μM and 0.01 ± 0.01 μM, respectively. The maximum levels of N and P were measured at the base of the nutricline (~400 m) during both seasons, with concentrations 1-2 orders of magnitude higher than in the LSW. Dissolved inorganic N in the LIW was >5 μM and P was >0.2 μM (Figure S1). From ~400 m downwards to the bottom there was no apparent seasonality in the physicochemical parameters measured (Figure S1).
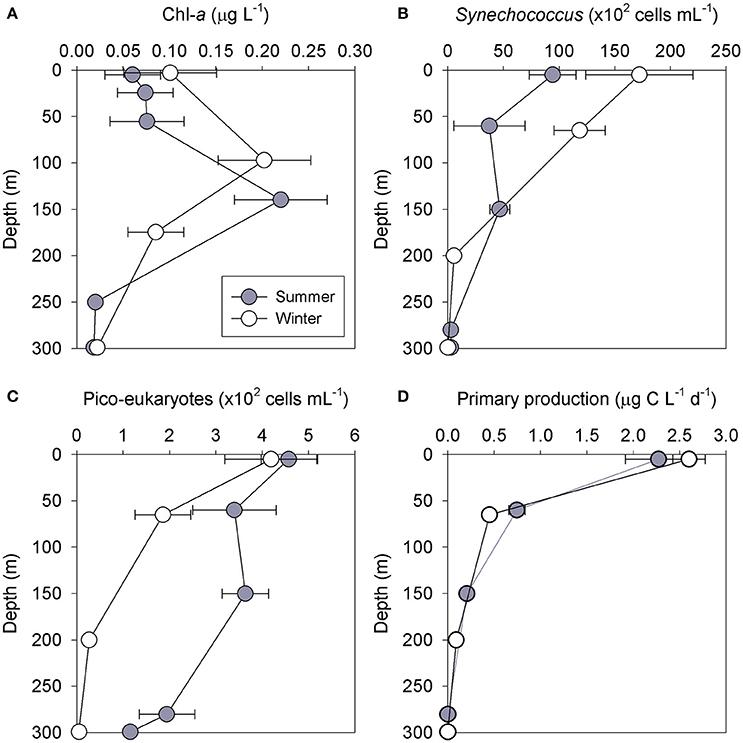
Figure 1. Vertical distribution of chl-a (A), Synechococcus (B), pico-eukaryotes (C) and primary production (D) during 5 winter (white) and 4 summer (gray) cruises at station H05 during 2013–2017. Values are the averages ± SD of measurements between cruises (n = 4–5 for each depth).
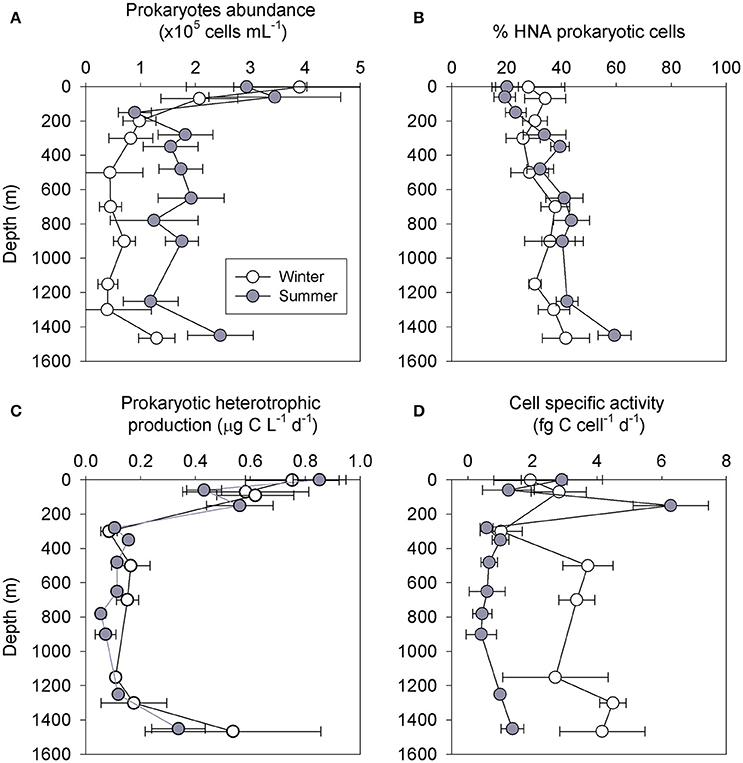
Figure 2. Vertical distribution of heterotrophic prokaryotic abundance (A), the percentage of high nucleic acid (HNA) prokaryotes (B), prokaryotic heterotrophic production (C) and heterotrophic cells specific activity (D) during 5 winter (white) and 4 summer (gray) cruises at station H05 during 2013–2017. Values are the averages ± SD of measurements between cruises (n = 4–5 for each depth).
Surface chl-a concentrations (upper 20 m) were low during both seasons, with 0.10 ± 0.05 μg L−1 during the winter and 0.06 ± 0.03 μg L−1 in the summer months (Figure 1A). A DCM was detected during most cruises, with the shallowest DCM (90–100 m) recorded during winter and the deepest reaching up to 145 m in the summer of August 2014 (Figure 1A). The chl-a concentrations in the DCM during both seasons were ~0.20 μg L−1. Although the depth profiles were different, the integrated chl-a levels (photic layer, surface to 150 m), were overall similar (~31 mg chl-a m−2). Surface Synechococcus abundance reached 171 ± 48 × 102 cells mL−1 during the winter (equivalent to 3000 ng C L−1) and 94 ± 21 × 102 cells mL−1 during the summer (1,650 ng C L−1) samplings (Figure 1B). Synechococcus abundance decreased with depth to 118 ± 23 × 102 cells mL−1 in the DCM during the winter (2050 ng C L−1) and 46 ± 9 × 102 cells mL−1 during the summer (815 ng C L−1) cruises (Figure 1B). These differences resulted in an integrated Synechococcus biomass during winter that was ~1.5 fold higher than in summer (316 mg m−2 vs. 195 mg m−2). The surface pico-eukaryotes abundance was ~4 × 102 cells mL−1 in both summer and winter (~900 ng C L−1, Figure 1C). The pico-eukaryotes concentration in the DCM was 2-fold higher during the summer (3.6±0.5 x102 cells mL−1, 760 ng C L−1) compared to the winter (1.8 ± 0.6 × 102 cells mL−1, 388 ng C L−1) months (Figure 1C), resulting in a nearly threefold greater integrated biomass, respectively (199 mg C m−2 vs. 74 mg C m−2). The primary production rates decreased with depth during both seasons and ranged from 2.6 μg C L−1 d−1 at the surface to below-detection limit at the bottom of the photic layer (Figure 2D). The integrated primary production did not differ significantly (p = 0.16) between the seasons and ranged from 0.15 to 0.18 g C m−2 d−1.
The highest surface prokaryotic abundance was measured during the winter sampling, with 3.8 ± 1.2 × 105 cells mL−1 (~8 ng C L−1) relative to an abundance of 2.9 ± 1.1 × 105 cells mL−1 (~6 ng C L−1) during the summer cruises (Figure 2A). Below the photic layer, the prokaryotic abundance was higher during the summer (1.2 to 2.4 × 105 cells mL−1) than the winter (0.4 to1.3 × 105 cells mL−1) (Figure 2A), resulting in a higher integrated prokaryotic biomass (0–1450 m) during the summer (3.8 ng C L−1) than the winter (~1 ng C L−1). The abundances of high nucleic acid (HNA) and low nucleic acid (LNA) cells, showed that HNA cells comprised 20–30% of the prokaryotes in the upper photic layer and ~40% below it (Figure 2B), with no distinct differences between the summer and winter samplings. The prokaryotic heterotrophic production rates were highest in the surface waters, with 0.75 ± 0.17 μg C L−1 d−1 during the winter and 0.85 ± 0.11 μg C L−1 d−1 during the summer samplings. The prokaryotic heterotrophic production decreased significantly below 200 m and remained uniformly low down to 1,300 m (~0.15 μg C L−1 d−1) during all cruises (Figure 2C). The integrated prokaryotic heterotrophic production (0–1450 m) were not significantly different between the cruises (0.25–0.30 g C m−2 d−1, p > 0.05). The resulting specific activity of the heterotrophic prokaryotic cells was overall higher during the winter (1–4 fg C cell−1 d−1) than the summer (0.4–3 fg C cell−1 d−1) cruises (Figure 2D).
Microcosm Bioassay Experiments
The LIW addition triggered a significant increase of 1.5-fold in the chl-a levels compared to the control incubations during winter and a > 2-fold increase during the summer (Figures 3A,E, Table 2). Synechococcus abundance responded similarly to the chl-a trend, with a moderate (~20%), yet significant, increase in cyanobacterial abundance following the addition of LIW during wintertime and an insignificant increase during the summer (Figures 3B,F, Table 2). Pico-eukaryotes increased following the LIW addition by ~2-fold relative to the control incubations (Figure 3C,G, Table 2), especially during the winter sampling after 48 h. These changes in algal biomass were also evident in the primary production rates measured during both seasons with 1.5-2-fold increase in rates (Figures 3D,H, Table 2).
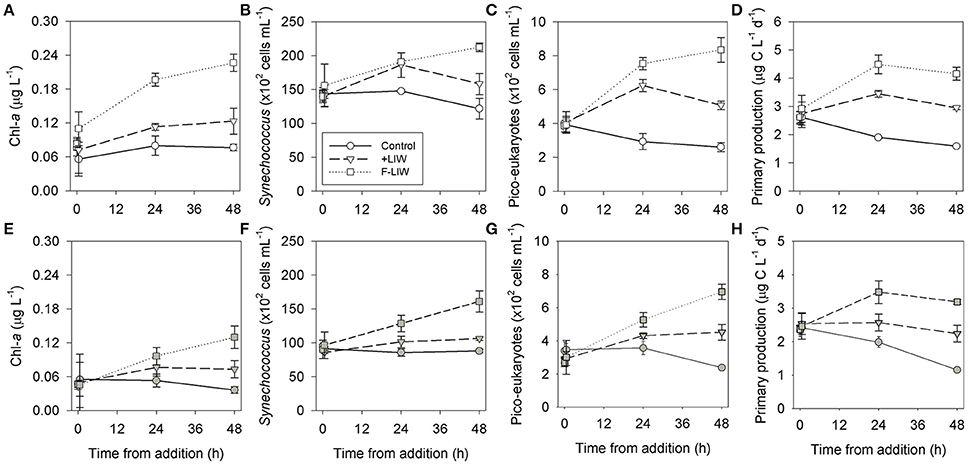
Figure 3. Dynamics of surface (~0.5 m) chl-a (A,E), Synechococcus (B,F), pico-eukaryotes (C,G) and primary production (D,H) following deep water addition (400 m, 10% of total microcosm volume) during winter, March 2014 (A–D, white) and summer, August 2014 (E–H, gray). Circles represent the control, triangles the LIW and squares the F-LIW treatments. Values presented are the averages from 3 independent replicates and their corresponding standard deviation.
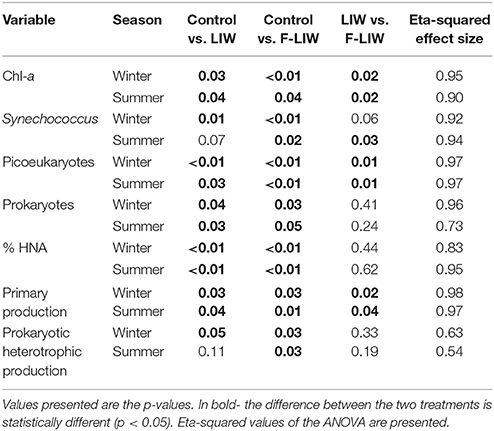
Table 2. Results of the statistical comparison (ANOVA, FISHER LSD multiple comparison post hoc test) between the different treatments (control, LIW, F-LIW) at the end of the experiment (T48) for the different variables tested and experiments (March 2014 and August 2014).
When the filtered LIW (0.2 μm, removing ~99% of all prokaryotes, Table 1) was added to the LSW (F-LIW treatment), a stronger and significant response was recorded than the LIW treatment, with a ~2.5 fold increase in chl-a levels relative to the control incubations in both the summer and winter experiments (Figures 3A,E, Table 2). Similarly, Synechococcus increased ~2-fold 48 h after its addition in both the summer and the winter experiments (Figures 3B,F, Table 2) and pico-eukaryotes increased up to 4-fold (Figures 3C,G, Table 2). Primary production increased ~3 fold following the addition of F-LIW (Figures 3D,H, Table 2).
Prokaryotic cell abundance increased significantly by 1.5-2 fold 24-48 h after the addition of LIW during both the winter and summer microcosm experiments (Figures 4A,D, Table 2). Among the prokaryotic cells, 30-50% had a high nucleic acid content compared to ~25% of the prokaryotic cells in the control incubations during winter (Figures 4B,E). Concurrent with the increase in prokaryotes abundance and %HNA, the prokaryotic heterotrophic production rates were 25–60% higher 24–48 h after addition (Figures 4C,F, Table 2).
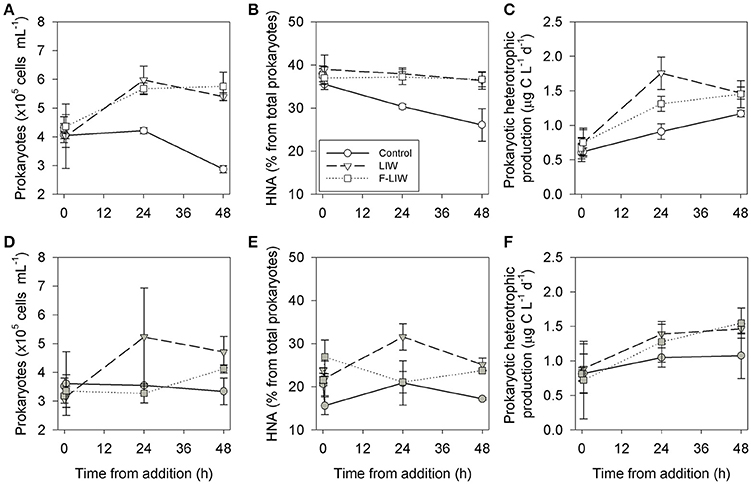
Figure 4. Dynamics of surface (0.5 m) prokaryotic abundance (A,D), the percentage of high nucleic acid (HNA) prokaryotes (B,E) and heterotrophic prokaryotes production (C,F) following the addition of deep water (400 m, 10% of total microcosm volume) during winter, March 2014 (A–D, white) and summer, August 2014 (E–H, gray). Circles represent the control, triangles the LIW and squares the F-LIW treatments. Values presented are the averages from 3 independent replicates and their corresponding standard deviation.
The addition of F-LIW resulted in an overall similar response as observed for the LIW treatment for most variables during the winter and to a lesser extent during summer (Figure 4). Thus, prokaryotes abundance significantly increased by 1.5-2-fold 24–48 h after F-LIW addition (Figures 4A,D) and high nucleic acid prokaryotes comprised ~20–30% of all prokaryotes cells (Figures 4B,E). Prokaryotic heterotrophic production rates were 1.5-fold higher than the unamended controls at 48 h measurement during both experiments (Figures 4C,F). Yet, it is to be noted, that the differences between the responses to F-LIW and LIW addition were not statistically significant at the 48 h time-point (Table 2).
Discussion
Addition of limiting nutrients to surface waters during winter mixing (Lindell and Post, 1995; Behrenfeld, 2010), upwelling gyres (Groom et al., 2005; Rahav et al., 2013a), coastal upwelling systems (Anabalón et al., 2016), or following external nutrient inputs (Mills et al., 2004; Paerl et al., 2011; Rahav et al., 2016a), often results in phytoplankton blooms (Moore et al., 2013; Rahav and Bar-Zeev, 2017). While this phenomena occurs in most marine environments (Smayda, 1997), phytoplankton blooms rarely occur in the EMS (Groom et al., 2005), despite the fact that deep water mixing (>450m) that replenish the surface water with N and P has been observed in extremely cold winters (Brenner et al., 1991; Krom et al., 1992; Zohary et al., 1998).
A number of factors can explain the lack of large-scale phytoplankton blooms following winter mixing, including top-down (i.e., grazing, viral infection) and bottom up (i.e., nutrient deficiency) interactions that preclude algal biomass enhancement (Billen et al., 1990). Our results, thought, suggest that the supply of autochthonous prokaryotic microbes inhabit the LIW (Van Wambeke et al., 2011; Luna et al., 2012; Keuter et al., 2015) may explain this phenomenon (Figures 3, 4). We show that once the deep microbes inhabiting the LIW mix with LSW, they negatively affect the surface microbial autotrophic populations and result in a moderate increase in phytoplankton biomass and production (Figure 3). In contrary, a stronger increase in autotrophic biomass and activity rates is observed when these autochthonous prokaryotic microbes are being excluded from the LIW (i.e., F-LIW), suggesting a “biological” inhibition of surface autotrophic populations (Figure 3).
We hypothesize that this is likely due to competition imposed on the surface phytoplankton by the LIW microbes and/or by lysogenic viruses/ bacterial-associated viruses that were carried with the microbes of the unfiltered (i.e., LIW) treatment which triggered a phytoplankton cell lysis via viral infection. A similar response was reported from a Lagrangian experiment in the EMS during May 2002 (Thingstad et al., 2005) and recently in a mesocosm experiment (Pitta et al., 2016) upon external P addition. In these experiments, P addition to the surface water caused a decrease in algal biomass and an increase in bacterial production and copepod egg abundance (Thingstad et al., 2005; Pitta et al., 2016). The authors concluded that the added P was transferred through the microbial food web to copepods, bypassing phytoplankton. Furthermore, virus–host interactions are known to have substantial roles in marine systems by altering microbial dynamics and processes (Suttle, 2007; Brum et al., 2015, 2016). It is therefore possible that viral infection of phytoplankton may also been in play. Yet, as viruses were not quantified in this study (using plaque assays and/or flow-cytometry), we cannot conclude whether phytoplankton and cyanobacteria were outcompeted by other bacteria or were negatively affected by viruses.
Several authors reported that the surface phytoplankton populations of the EMS are N or N+P co-limited, whereas heterotrophic prokaryotes are P limited (Zohary et al., 2005; Pitta et al., 2016; Tsiola et al., 2016). These authors concluded that if the nutrients stress is relieved by external addition, the autotrophic and heterotrophic biomass and production are enhanced several times fold (Kress et al., 2005; Zohary et al., 2005; Herut et al., 2016; Tsiola et al., 2016). Thus, any input of N and P to the surface layer via water mixing should potentially relieve the phytoplankton and prokaryotic heterotrophic cellular growth limitations and consequently trigger blooms. Our results, however, suggest that the increased observed in these laboratory/on-deck bioassays (% change relative to unamended control) may be lower in situ if deep water mixing is the source for the added nutrients (rather than other external nutrient sources such as dust deposition, i.e., Rahav et al., 2016b). Here, the addition of F-LIW, which resulted in similar net N and P enrichment as tested in those laboratory/on-deck bioassays (yet without the LIW's microbes, Table 1), yielded 1.5-3-fold higher responses than observed by these previous studies (Kress et al., 2005; Zohary et al., 2005; Herut et al., 2016; Tsiola et al., 2016) or by our LIW addition (Figures 3, 4). We surmise that this discrepancy may be explained by the prokaryotes that inhabit the LIW that interacted (symbiosis, competition, allelopathy etc.) with the ambient surface microbial populations (Guixa-boixereu et al., 1999; Bassler and Losick, 2006; Weinbauer et al., 2011). Currently, we cannot say which of the interactions between the LIW microbes and LSW autotrophic populations were in play. Nevertheless, previous studies demonstrate that such interactions may balance phytoplankton loss processes and growth (Ribalet et al., 2015) as well as enhance/inhibit the growth of algae (Grossart et al., 2006; Mayali et al., 2008) and cyanobacteria (Sher et al., 2011).
The deep Mediterranean waters are known hotspots for heterotrophic prokaryotic production and enzymatic activity (Danovaro et al., 2010; Van Wambeke et al., 2011; Luna et al., 2012; Rahav et al., 2013c). This is most likely a result of the warm temperatures measured at the deep waters throughout the year (Figure S1A), which are ~10°C higher than in any oceanic system of similar depths (Danovaro et al., 2004, 2010; Luna et al., 2012; Keuter et al., 2015). Moreover, the LIW Mediterranean waters are considered young compared to other oceanic systems (70–100 years) (Bergamasco and Malanotte-Rizzoli, 2010); thereby their associated dissolved organic carbon pool potentially represents a more labile and available food source for prokaryotes (Santinelli et al., 2010). Thus, the LIW heterotrophic microbial assemblages from the Mediterranean Sea have a much higher affinity for carbon-based substrates than surface populations (Luna et al., 2012). It is therefore expected that the deep-water conditions of the EMS will support relatively high prokaryotic growth rates and fast metabolism (relative to other oceanic environments in similar water depths). Indeed, high prokaryotic cell specific activity was measured in the LIW during this study, especially during wintertime (Figure 2D). These values were within at the upper range reported for the same depth in other oceanic environments worldwide (Arístegui et al., 2009; Baltar et al., 2009; Luna et al., 2012).
In contrast to the apparently significant affect deep microbes has on autotrophic surface populations (Figure 3), the surface heterotrophic prokaryotes were usually less affected by the input of LIW-microbial populations upon water mixing (Figure 4), resulting in insignificant changes between the LIW and F-LIW treatments following 48 h of incubation (Table 2). It is to be noted that some significant changes in the prokaryotic variables were found following 24 h of incubation (i.e., Figures 4C,E), yet the overall responses (24–48 h) was insignificantly different between the LIW and F-LIW treatments (p > 0.05, not shown). Our results suggest that the changes observed in prokaryotes abundance or activity following LIW addition (especially following 48 h of mixing) are presumably triggered by the elevated nutrients and not by the LIW microbes as no significant change was found between the LIW and F-LIW treatments (Table 2). It may also suggest a synergistic relationship between the LSW and LIW prokaryotes rather than competition. Alternatively, it may suggest that the LSW prokaryotes are more adept at utilizing the external nutrient supply as they constantly “live” under nutrient impoverished conditions, in contrast to the microbes habitat the nutrient-rich LIW. Yet, more studies should be carried out, covering more sampling points, seasons and genetic analyses, in order to evaluate which mechanisms were in play.
Conclusions
Our results imply that even in extremely cold winters in which cooling triggers a deep convection that elevates LIW, primary production may not likely increase substantially above the current rates (~70–150 g C m−2 y−1) (Béthoux et al., 1998; Zohary et al., 2005). Yet, this trend may be affected by the spatiotemporal variability of heterotrophic prokaryotes in the EMS's LIW (Keuter et al., 2015; Dubinsky et al., 2016), as well as by the vertical mixing depth. Thus, under “typically shallow mixing” scenario (<200 m), different microbial responses may occur. These vertical (and possibly also temporal) changes may play an important role in the control, development and aggregation of phytoplankton in marine systems (Grossart et al., 2006). We therefore stress that more studies should be carried out which includes different mixing depths and duration and with additional treatments such as adding the LIW microbes in the absence of any nutrient addition (i.e., use the material that was accumulated on a 0.2-μm filter) as well as adding stock nutrients (N and P) at equivalent concentration as found in the LIW. Such experiments will enable to fully evaluate how LIW microbes interact with the LSW populations.
Finally, it is possible that LIW microbes can introduce new functions to the surface water, thereby affecting nutrient cycling and production indirectly. For example, deep-water diazotrophs that inhabit the LIW (Rahav et al., 2013c; Benavides et al., 2016) might fix N2 when transported to the surface layer during the winter mixing, thus adding to the usually low N2 fixation rates (Berman-Frank and Rahav, 2012). This in turn may also affect the exceptionally high N:P ratio at the surface layers (~28:1) (Krom et al., 2010). Knowledge regarding deep-EMS food web dynamics (and particularly of the LIW) is scarce and the vertical transport rates of organic matter and its recycling are largely unknown. This study has started to fill these knowledge gaps for the EMS.
Author Contributions
ER: Conceived and designed the experiments; OH, ES-F, and ER: Performed the experiments; OH, JS, NK, GS-V, TO, IG, and ER: Analyzed the data; JS, NK, and ER: Contributed reagents, materials, analysis tools; OH, JS, NK, GS-V, TO, IG, and ER: Wrote the paper.
Funding
This work was supported by grants awarded by the Ministry of National Infrastructures, Energy and Water Resources (grant number 3-11519) to ER, by the Israeli National Monitoring Program to ER, and partly by the DeepLev campaign.
Conflict of Interest Statement
The authors declare that the research was conducted in the absence of any commercial or financial relationships that could be construed as a potential conflict of interest.
The reviewer AR and handling Editor declared their shared affiliation.
Acknowledgments
We would like to thank the captains and crew of the R/V Shikmona and R/V Bat-Galim for their help at sea. We would also like to thank the reviewers for their most beneficial suggestions that greatly improved the manuscript.
Supplementary Material
The Supplementary Material for this article can be found online at: https://www.frontiersin.org/articles/10.3389/fmars.2018.00001/full#supplementary-material
Figure S1. Vertical distribution of temperature (A), salinity (B), dissolved oxygen (C), nitrate and nitrite (D), phosphorus (E) and silicic acid (F) during 5 winter (white) and 4 summer (gray) cruises at station H05 during 2013–2017. Values are the averages ± SD of measurements between cruises (n = 4–5 for each depth). Insert—the upper 300 m.
References
Anabalón, V., Morales, C. E., González, H. E., Menschel, E., Schneider, W., Hormazabal, S., et al. (2016). Micro-phytoplankton community structure in the coastal upwelling zone off concepción (central Chile): annual and inter-annual fluctuations in a highly dynamic environment. Prog. Oceanogr. 149, 174–188. doi: 10.1016/j.pocean.2016.10.011
Arístegui, J., Gasol, J. M., Duarte, C. M., and Herndl, G. J. (2009). Microbial oceanography of the dark oceans pelagic realm. Limnol. Oceanogr. 54, 1501–1529. doi: 10.4319/lo.2009.54.5.1501
Arrigo, K. (2005). Marine microorganisms and global nutrient cycles. Nature 437, 349–355. doi: 10.1038/nature04159
Astrahan, P., Herut, B., Paytan, A., and Rahav, E. (2016). The impact of dry atmospheric deposition on the sea-surface microlayer in the SE Mediterranean Sea: an experimental approach. Front. Mar. Sci. 3:222. doi: 10.3389/fmars.2016.00222
Baltar, F., Arístegui, J., Gasol, J. M., Sintes, E., and Herndl, G. J. (2009). Evidence of prokaryotic metabolism on suspended particulate organic matter in the dark waters of the subtropical North Atlantic. Limnol. Oceanogr. 54, 182–193. doi: 10.4319/lo.2009.54.1.0182
Bar-Zeev, E., and Rahav, E. (2015). Microbial metabolism of transparent exopolymer particles during the summer months along a eutrophic estuary system. Front. Microbiol. 6:430. doi: 10.3389/fmicb.2015.00403
Bassler, B. L., and Losick, R. (2006). Bacterially speaking. Cell 125, 237–246. doi: 10.1016/j.cell.2006.04.001
Behrenfeld, M. J. (2010). Abandoning Sverdrup's critical depth hypothesis on phytoplankton blooms. Ecology 91, 977–989. doi: 10.1890/09-1207.1
Benavides, M., Bonnet, S., Hernández, N., Martínez-Pérez, A. M., Nieto-Cid, M., Álvarez-Salgado, X. A., et al. (2016). Basin-wide N2 fixation in the deep waters of the Mediterranean Sea. Glob. Biogeochem. Cycles 30, 1–10. doi: 10.1002/2015GB005326
Bergamasco, A., and Malanotte-Rizzoli, P. (2010). The circulation of the Mediterranean Sea: a historical review of experimental investigations. Adv. Oceanogr. Limnol. 1, 11–28. doi: 10.4081/aiol.2010.5293
Berman-Frank, I., and Rahav, E. (2012). “Nitrogen fixation as a source for new production in the Mediterranean Sea: a review,” in Life in the Mediterranean Sea: A Look at Habitat Changes (New York, NY: Nova Science Publishers), 199–226.
Béthoux, J. P., Morin, P., Chaumery, C., Connan, O., Gentili, B., and Ruiz-Pino, D. (1998). Nutrients in the Mediterranean Sea, mass balance and statistical analysis of concentrations with respect to environmental change. Mar. Chem. 63, 155–169. doi: 10.1016/S0304-4203(98)00059-0
Billen, G., Servais, P., and Becquevort, S. (1990). Dynamics of bacterioplankton in oligotrophic and eutrophic aquatic environments: bottom-up or top-down control? Hydrobiologia 207, 37–42. doi: 10.1007/BF00041438
Brenner, S., Rozentraub, Z., Bishop, J., and Krom, M. (1991). The mixed-layer/thermocline cycle of a persistent warm core eddy in the eastern Mediterranean. Dyn. Atmos. Ocean. 15, 457–476. doi: 10.1016/0377-0265(91)90028-E
Brum, J. R., Hurwitz, B. L., Schofield, O., Ducklow, H. W., and Sullivan, M. B. (2016). Seasonal time bombs: dominant temperate viruses affect Southern Ocean microbial dynamics. ISME J. 10, 437–449. doi: 10.1038/ismej.2015.125
Brum, J. R., Ignacio-Espinoza, J. C., Roux, S., Doulcier, G., Acinas, S. G., Alberti, A., et al. (2015). Patterns and ecological drivers of ocean viral communities. Science 348:1261498. doi: 10.1126/science.1261498
Campbell, J. W., and Yentsch, C. M. (1989). Variance within homogeneous phytoplankton populations, II: analysis of clonal cultures. Cytometry 10, 596–604. doi: 10.1002/cyto.990100515
D'Ortenzio, F., Iudicone, D., de Boyer Montegut, C., Testor, P., Antoine, D., Marullo, S., et al. (2005). Seasonal variability of the mixed layer depth in the Mediterranean Sea as derived from in situ profiles. Geophys. Res. Lett. 32, 1–4. doi: 10.1029/2005GL022463
Danovaro, R., Company, J. B., Corinaldesi, C., D'Onghia, G., Galil, B., Gambi, C., et al. (2010). Deep-sea biodiversity in the Mediterranean Sea: the known, the unknown, and the unknowable. PLoS ONE 5:e11832. doi: 10.1371/journal.pone.0011832
Danovaro, R., Dell'Anno, A., and Pusceddu, A. (2004). Biodiversity response to climate change in a warm deep sea. Ecol. Lett. 7, 821–828. doi: 10.1111/j.1461-0248.2004.00634.x
Dishon, G., Dubinsky, Z., Caras, T., Rahav, E., Bar-Zeev, E., Tzubery, Y., et al. (2012). Optical habitats of ultraplankton groups in the Gulf of Eliat (Aqaba), Northern Red Sea. Int. J. Remote Sens. 33, 2683–2705. doi: 10.1080/01431161.2011.619209
Dubinsky, V., Haber, M., Burgsdorf, I., Saurav, K., Lehahn, Y., Malik, A., et al. (2016). Metagenomic analysis reveals unusually high incidence of proteorhodopsin genes in the ultraoligotrophic Eastern Mediterranean Sea. Environ. Microbiol. 19, 1077–1090. doi: 10.1111/1462-2920.13624
Falkowski, P. (1997). Evolution of the nitrogen cycle and its influence on the biological sequestration of CO2 in the ocean. Nature 387, 272–275. doi: 10.1038/387272a0
Groom, S., Herut, B., Brenner, S., Zodiatis, G., Psarra, S., Kress, N., et al. (2005). Satellite-derived spatial and temporal biological variability in the Cyprus Eddy. Deep Sea Res. Part II Top. Stud. Oceanogr. 52, 2990–3010. doi: 10.1016/j.dsr2.2005.08.019
Grossart, H. P., Czub, G., and Simon, M. (2006). Algae-bacteria interactions and their effects on aggregation and organic matter flux in the sea. Environ. Microbiol. 8, 1074–1084. doi: 10.1111/j.1462-2920.2006.00999.x
Guixa-boixereu, N., Lysnes, K., Guixa-boixereu, R. I. A., and Lysnes, K. (1999). Viral lysis and bacterivory during a phytoplankton bloom in a coastal water microcosm. Appl. Environ. Microbiol. 65, 1949–1958.
Hecht, A., Pinardi, N., and Robinson, A. R. (1988). Currents, water mass, eddies and jets in the Mediterranean Levantine Basin. J. Phys. Oceanogr. 18, 1320–1352.
Herut, B., Rahav, E., Tsagaraki, T. M., Giannakourou, A., Tsiola, A., Psarra, S., et al. (2016). The potential impact of Saharan dust and polluted aerosols on microbial populations in the east Mediterranean Sea, an overview of a mesocosm experimental approach. Front. Mar. Sci. 3, 1–16. doi: 10.3389/fmars.2016.00226
Keuter, S., Rahav, E., Herut, B., and Rinkevich, B. (2015). Distribution patterns of bacterioplankton in the oligotrophic south-eastern Mediterranean Sea. Microbiol. Fems Adv. Ecol. 91, 1–39. doi: 10.1093/femsec/fiv070
Kress, N., Frede Thingstad, T., Pitta, P., Psarra, S., Tanaka, T., Zohary, T., et al. (2005). Effect of P and N addition to oligotrophic Eastern Mediterranean waters influenced by near-shore waters: a microcosm experiment. Deep. Res. Part II Top. Stud. Oceanogr. 52, 3054–3073. doi: 10.1016/j.dsr2.2005.08.013
Kress, N., Gertman, I., and Herut, B. (2014). Temporal evolution of physical and chemical characteristics of the water column in the Easternmost Levantine Basin (Eastern Mediterranean Sea) from 2002 to 2010. J. Mar. Syst. 135, 6–13. doi: 10.1016/j.jmarsys.2013.11.016
Kress, N., and Herut, B. (2001). Spatial and seasonal evolution of dissolved oxygen and nutrients in the Southern Levantine Basin (Eastern Mediterranean Sea): chemical characterization of the water masses and inferences on the N: P ratios. Deep. Res. I 48, 2347–2372. doi: 10.1016/S0967-0637(01)00022-X
Krom, M. D., Emeis, K. C., and Van Cappellen, P. (2010). Why is the Eastern Mediterranean phosphorus limited? Prog. Oceanogr. 85, 236–244. doi: 10.1016/j.pocean.2010.03.003
Krom, M. D., Kress, N., Berman-Frank, I., and Rahav, E. (2014). “Past, present and future patterns in the nutrient chemistry of the eastern mediterranean,” in The Mediterranean Sea: Its history And Present Challenges, eds S. Goffredo and Z. Dubinsky (New York, NY: Springer), 49–68.
Krom, M. D., Kress, N., Brenner, S., and Gordon, L. I. (1991). Phosphorus limitation of primary productivity in the eastern Mediterranean Sea. Limnol. Oceanogr. 36, 424–432. doi: 10.4319/lo.1991.36.3.0424
Krom, M. D., Brenner, S., Kress, N., Naori, A., and Gordon, L. I. (1992). Nutrient dynamics and new production in a warm-core eddy from the Eastern Mediterranean Sea. Deep Sea Res. Part I Oceanogr. Res. Pap. 39, 467–480. doi: 10.1016/0198-0149(92)90083-6
Lebaron, P., Servais, P., Agogué, H., Courties, C., and Joux, F. (2001). Does the high nucleic acid content of individual bacterial cells allow us to discriminate between active cells and inactive cells in aquatic systems? Appl. Environ. Microbiol. 67, 1775–1782. doi: 10.1128/AEM.67.4.1775-1782.2001
Lindell, D., and Post, A. F. (1995). Ultraphytoplankton succession is triggered by deep winter mixing in the Gulf of Aqaba (Eilat), Red Sea. Limnol. Oceanogr. 40, 1130–1141. doi: 10.4319/lo.1995.40.6.1130
Luna, G. M., Bianchelli, S., Decembrini, F., De Domenico, E., Danovaro, R., and Dell'Anno, A. (2012). The dark portion of the Mediterranean Sea is a bioreactor of organic matter cycling. Glob. Biogeochem. Cycles 26, 1–14. doi: 10.1029/2011GB004168
Mayali, X., Franks, P. J. S., and Azam, F. (2008). Cultivation and ecosystem role of a marine Roseobacter clade-affiliated cluster bacterium. Appl. Environ. Microbiol. 74, 2595–2603. doi: 10.1128/AEM.02191-07
Mella-Flores, D., Mazard, S., Humily, F., Partensky, F., Mahe, F., Bariat, L., et al. (2011). Is the distribution of Prochlorococcus and Synechococcus ecotypes in the Mediterranean Sea affected by global warming? Biogeosciences 8, 2785–2804. doi: 10.5194/bg-8-2785-2011
Mills, M. M., Ridame, C., Davey, M., La Roche, J., and Geider, R. J. (2004). Iron and phosphorus co-limit nitrogen fixation in the eastern tropical North Atlantic. Nature 429, 292–294. doi: 10.1038/nature02550
Moore, C. M., Mills, M. M., Arrigo, K. R., Berman-Frank, I., Bopp, L., Boyd, P. W., et al. (2013). Processes and patterns of oceanic nutrient limitation. Nat. Geosci. 6, 701–710. doi: 10.1038/ngeo1765
Paerl, H. W., Hall, N. S., and Calandrino, E. S. (2011). Controlling harmful cyanobacterial blooms in a world experiencing anthropogenic and climatic-induced change. Sci. Total Environ. 409, 1739–1745. doi: 10.1016/j.scitotenv.2011.02.001
Pitta, P., Nejstgaard, J. C., Tsagaraki, T. M., Zervoudaki, S., Egge, J. K., Frangoulis, C., et al. (2016). Confirming the “Rapid phosphorus transfer from microorganisms to mesozooplankton in the Eastern Mediterranean Sea” scenario through a mesocosm experiment. J. Plankton Res. 38, 502–521. doi: 10.1093/plankt/fbw010
Rahav, E., and Bar-Zeev, E. (2017). Sewage outburst triggers Trichodesmium bloom and enhance N2 fixation rates. Sci. Rep. 7, 4367. doi: 10.1038/s41598-017-04622-8
Rahav, E., Bar-Zeev, E., Ohayon, S., Elifantz, H., Belkin, N., Herut, B., et al. (2013c). Dinitrogen fixation in aphotic oxygenated marine environments. Front. Microbiol. 4:227. doi: 10.3389/fmicb.2013.00227
Rahav, E., Giannetto, M., and Bar-Zeev, E. (2016a). Contribution of mono and polysaccharides to heterotrophic N2 fixation at the eastern Mediterranean coastline. Sci. Rep. 6:27858. doi: 10.1038/srep27858
Rahav, E., Herut, B., Levi, A., Mulholland, M., and Berman-Frank, I. (2013b). Springtime contribution of dinitrogen fixation to primary production across the Mediterranean Sea. Ocean Sci. 9, 489–498. doi: 10.5194/os-9-489-2013
Rahav, E., Herut, B., Stambler, N., Bar-Zeev, E., Mulholland, M. R., and Berman-Frank, I. (2013a). Uncoupling between dinitrogen fixation and primary productivity in the eastern Mediterranean Sea. J. Geophys. Res. Biogeosciences 118, 195–202. doi: 10.1002/jgrg.20023
Rahav, E., Paytan, A., Chien, C., Ovadia, G., Katz, T., and Herut, B. (2016b). The impact of atmospheric dry deposition associated microbes on the southeastern Mediterranean Sea surface water following an intense dust storme. Front. Mar. Sci. 3:127. doi: 10.3389/fmars.2016.00127
Rahav, E., Raveh, O., Hazan, O., Gordon, N., Kress, N., Silverman, J., et al. (2018). Impact of nutrient enrichment on productivity of coastal water along the SE Mediterranean shore of Israel–A bioassay approach. Mar. Pollut. Bull. 127, 559–567. doi: 10.1016/j.marpolbul.2017.12.048
Raven, J., and Falkowski, P. (1999). Oceanic sinks for atmospheric CO2. Plant Cell Environ. 22, 741–755. doi: 10.1046/j.1365-3040.1999.00419.x
Ribalet, F., Swalwell, J., Clayton, S., Jiménez, V., Sudek, S., Lin, Y., et al. (2015). Light-driven synchrony of Prochlorococcus growth and mortality in the subtropical Pacific gyre. Proc. Natl. Acad. Sci. U.S.A. 112, 8008–8112. doi: 10.1073/pnas.1424279112
Santinelli, C., Nannicini, L., and Seritti, A. (2010). DOC dynamics in the meso and bathypelagic layers of the Mediterranean Sea. Deep. Res. Part II Top. Stud. Oceanogr. 57, 1446–1459. doi: 10.1016/j.dsr2.2010.02.014
Servais, P., Courties, C., Lebaron, P., and Troussellier, M. (1999). Coupling bacterial activity measurements with cell sorting by flow cytometry. Microb. Ecol. 38, 180–189. doi: 10.1007/s002489900160
Sher, D., Thompson, J. W., Kashtan, N., Croal, L., and Chisholm, S. W. (2011). Response of Prochlorococcus ecotypes to co-culture with diverse marine bacteria. ISME J. 5, 1125–1132. doi: 10.1038/ismej.2011.1
Shi, Y., McCarren, J., and Delong, E. F. (2012). Transcriptional responses of surface water marine microbial assemblages to deep-sea water amendment. Environ. Microbiol. 14, 191–206. doi: 10.1111/j.1462-2920.2011.02598.x
Simon, M., Alldredge, A., and Azam, F. (1990). Bacterial carbon dynamics on marine snow. Mar. Ecol. Prog. Ser. 65, 205–211. doi: 10.3354/meps065205
Simon, M., and Azam, F. (1989). Protein content and protein synthesis rates of planktonic marine bacteria. Mar. Ecol. Prog. Ser. 51, 201–213. doi: 10.3354/meps051201
Siokou-Frangou, I., Christaki, U., Mazzocchi, M. G., Montresor, M., Ribera d'Alcalá, M., Vaqué, D., et al. (2010). Plankton in the open Mediterranean Sea: a review. Biogeosciences 7, 1543–1586. doi: 10.5194/bg-7-1543-2010
Sisma-Ventura, G., Yam, R., Kress, N., and Shemesh, A. (2016). Water column distribution of stable isotopes and carbonate properties in the South-eastern Levantine basin (Eastern Mediterranean): Vertical and temporal change. J. Mar. Syst. 158, 13–25. doi: 10.1016/j.jmarsys.2016.01.012
Smayda, T. J. (1997). Bloom dynamics: physiology, behavior, trophic effects. Limonaol. Oceanogr. 42, 1132–1136. doi: 10.4319/lo.1997.42.5_part_2.1132
Smith, D. C., Smith, D. C., Azam, F., and Azam, F. (1992). A simple, economical method for measuring bacterial protein synthesis rates in seawater using 3H-leucine. Mar. Microb. food web 6, 107–114.
Steemann-Nielsen, E. (1952). On the determination of the activity for measuring primary production. J. Cons. Int. Explor. Mer. 18, 117–140.
Suttle, C. A. (2007). Marine viruses - major players in the global ecosystem. Nat. Rev. Microbiol. 5, 801–812. doi: 10.1038/nrmicro1750
Tabachnick, B. G., and Fidell, L. S. (2013). Using Multivariate Statistics, 6th Edn. Harlow: Pearson Education Limited.
Tanhua, T., Hainbucher, D., Schroeder, K., Cardin, V., Álvarez, M., and Civitarese, G. (2013). The Mediterranean Sea system: A review and an introduction to the special issue. Ocean Sci. 9, 789–803. doi: 10.5194/os-9-789-2013
Thingstad, T. F., Krom, M. D., Mantoura, R. F. C., Flaten, G., A, F., Groom, S., Herut, B., et al. (2005). Nature of phosphorus limitation in the ultraoligotrophic eastern Mediterranean. Science 309, 1068–1071. doi: 10.1126/science.1112632
Tsiola, A., Pitta, P., Fodelianakis, S., Pete, R., Magiopoulos, I., Mara, P., et al. (2016). Nutrient limitation in surface waters of the oligotrophic eastern Mediterranean Sea: an enrichment microcosm experiment. Microb. Ecol. 71, 575–588. doi: 10.1007/s00248-015-0713-5
Van Wambeke, F., Catala, P., Pujo-Pay, M., and Lebaron, P. (2011). Vertical and longitudinal gradients in HNA-LNA cell abundances and cytometric characteristics in the Mediterranean Sea. Biogeosciences 8, 1853–1863. doi: 10.5194/bg-8-1853-2011
Vaulot, D., and Marie, D. (1999). Diel variability of photosynthetic picoplankton in the equatorial Pacific. Appl. Environ. Microbiol. 104, 3297–3310. doi: 10.1029/98JC01333
Weinbauer, M. G., Bonilla-Findji, O., Chan, A. M., Dolan, J. R., Short, S. M., Imek, K., et al. (2011). Synechococcus growth in the ocean may depend on the lysis of heterotrophic bacteria. J. Plankton Res. 33, 1465–1476. doi: 10.1093/plankt/fbr041
Welschmeyer, N. A. (1994). Fluorometric analysis of chlorophyll a in the presence of chlorophyll b and pheopigments. Limnol. Oceanogr. 39, 1985–1992. doi: 10.4319/lo.1994.39.8.1985
Zohary, T., Brenner, S., and Krom, M. (1998). Buildup of microbial biomass during deep winter mixing in a Mediterranean warm-core eddy. Mar. Ecol. Prog. Ser. 167, 47–57. doi: 10.3354/meps167047
Keywords: phytoplankton, primary production, bacterial production, Eastern Mediterranean Sea, bacterial infections, nutrients
Citation: Hazan O, Silverman J, Sisma-Ventura G, Ozer T, Gertman I, Shoham-Frider E, Kress N and Rahav E (2018) Mesopelagic Prokaryotes Alter Surface Phytoplankton Production during Simulated Deep Mixing Experiments in Eastern Mediterranean Sea Waters. Front. Mar. Sci. 5:1. doi: 10.3389/fmars.2018.00001
Received: 03 October 2017; Accepted: 09 January 2018;
Published: 24 January 2018.
Edited by:
Peter R. Girguis, Harvard University, United StatesReviewed by:
Dolors Vaque, Consejo Superior de Investigaciones Científicas (CSIC), SpainAspen Reese, Harvard University, United States
Copyright © 2018 Hazan, Silverman, Sisma-Ventura, Ozer, Gertman, Shoham-Frider, Kress and Rahav. This is an open-access article distributed under the terms of the Creative Commons Attribution License (CC BY). The use, distribution or reproduction in other forums is permitted, provided the original author(s) or licensor are credited and that the original publication in this journal is cited, in accordance with accepted academic practice. No use, distribution or reproduction is permitted which does not comply with these terms.
*Correspondence: Eyal Rahav, ZXlhbC5yYWhhdkBvY2Vhbi5vcmcuaWw=