- Cognitive and Behavioural Ecology Program, Psychology Department, Memorial University of Newfoundland, St. John's, NL, Canada
Diving seabirds that overwinter at high latitudes experience persistent cold exposure, short days and associated declines in ocean productivity that can challenge their ability to balance daily energy budgets. We used dive-immersion geo-locators to test the hypothesis that pursuit-diving Common murres (Uria aalge) will respond to the challenges of winter in the North Atlantic through increased daily energy expenditures (DEE) that will be met by increased foraging effort and adjustments in dive tactics. Largely flightless in winter (<5% of daylight hours flying), murres spent most of their time on the water (>85% resting and swimming). Accordingly, when sea surface temperatures (SST) were consistently near freezing in late winter (1.9 ± 0.8°C), mean DEE (2463.2 ± 10.9 kJ day−1) exceeded the theoretical limit to sustainable energy expenditure in vertebrates (i.e., 7 X Basal Metabolic Rate or 2450 kJ day−1 for murres). Consistently deep (70% >50 m) and long dives in late winter, 38% of which exceeded their calculated aerobic dive limit indicate that targeted prey was distributed in deep (dark) waters. Consequently, foraging was largely diurnal; likely because capture efficiency of deep-water prey is poor under low light. Murres responded to these late winter time and energy constraints with a nearly two-fold increase in daily time spent diving (95.2 ± 5.6 and 178.3 ± 6.3 min day−1 during early and late winter, respectively), an increase in dive bout frequency and duration, and correspondingly less time resting between bouts. Uniquely adapted for deep-diving, pursuit-diving can push their dive limits to maximize daily energy intake when energy demands are high and prey are distributed in deep water. Our study highlights late winter as an extremely challenging phase in the annual cycle of North Atlantic murres and provides critical insights into the behavioral mechanisms underlying their winter survival.
Introduction
Diving seabirds that over-winter at high latitudes contend with high-energy requirements for thermoregulation and challenging foraging conditions during seasonal lows in ocean productivity and short days. Winter survival is a critical life history trait of long-lived seabirds (Votier et al., 2005; Daunt et al., 2007; Frederiksen et al., 2008; Sorensen et al., 2009) but the challenges of studying seabirds at sea limit our understanding of the behavioral strategies that mediate survival. Advances in bio-logging technology are providing unprecedented insights into the behavior of diving seabirds at sea but studies that focus on the critical winter period are scarce and are primarily restricted to the larger divers (e.g., Spheniscidae, Phalacrocoracidae) that are more amenable to carrying data loggers over the annual cycle (Green et al., 2005, 2009; Grémillet et al., 2005a; Daunt et al., 2006).
Thermoregulation, although partially mediated by morphological adaptations that reduce heat loss (water-proof feathers, lipid stores; Wharton, 2002) elevates the daily energy expenditures (DEE) of diving birds that spend most of their time on the water where heat loss is 25 times greater than in air (Croll and McClaren, 1993; Enstipp et al., 2006). Moreover, because thermal conductance increases with decreasing body size, thermal costs in cold water are higher for smaller divers (<1 kg; Croll and McClaren, 1993; Richman and Lovvorn, 2011). Accordingly, elevated energy requirements for thermoregulation must be met with an increase in food energy intake during winter but this can be challenged by degraded foraging conditions. By example, many zooplankton (e.g., Calanus spp.) and forage fish species spend the winter in deep, cold waters (Winters, 1983; Longhurst, 1995; Planque et al., 1997; Maillet and Colbourne, 2007; Olsen et al., 2010), and are less accessible to diving predators. Unpredictable and extreme weather events (i.e., gale-force storms and ice intrusions) can also temporarily impede access to prey (Finney et al., 1999; McFarlane-Tranquilla et al., 2010) and ultimately result in starvation (Gaston, 2004; Grémillet et al., 2005a; Daunt et al., 2007). Diving seabirds, that are primarily visual predators (c.f. Regular et al., 2011; Berge et al., 2015) also face additional constraints on foraging time during the shorter days of winter and correspondingly longer periods of nocturnal fasting (Grémillet et al., 2005a,b; Daunt et al., 2007). This conflict between resource demand and availability in winter raises the critical, but poorly resolved question of how diving seabirds meet the energy demands of survival.
We use dive-immersion geo-locators to study the behavioral strategies of pursuit-diving Common Murres (Uria aalge, hereafter murres) during winter in the Northwest Atlantic. Murres are the largest of the pursuit-diving alcids (c.a. 1 kg) and play a key role in the energy flow through circumpolar marine food webs in temperate and sub-Arctic waters (Gaston and Jones, 1998; Montevecchi, 2000; de L Brooke, 2004). Owing to small wings that reduce underwater drag, they are the deepest diving bird that can fly (250 m; Chimienti et al., 2017) with lower than expected metabolic costs during diving but higher than expected flight costs (Elliott et al., 2013). Emerging insights into the physiological processes during diving, involving reductions in blood flow to metabolically expensive organs (Niizuma et al., 2007) and reductions in core temperature and heart rate (Wilson et al., 1992; Elliott et al., 2013) suggest the metabolic costs of diving for murres may be lower than previously predicted, and possibly even decrease with depth which may allow them to extend their aerobic dive limit (Gerlinsky et al., 2013).
Tracking studies of adult murres from Newfoundland and Labrador colonies have established their core wintering area on the Newfoundland-Labrador Shelf (NL Shelf), centered on the eastern Grand Bank (Templeman, 2010; Hedd et al., 2011; McFarlane-Tranquilla et al., 2013). The defining climatic feature of this region is the south-flowing Labrador Current that transports sub-polar waters from the Canadian Arctic across the southern extent of the NL Shelf (Colbourne et al., 2015). A previous study demonstrated that related North Atlantic Thick-billed Murres and Dovekies (Alle alle) that overwinter on the NL Shelf experience a late winter energy bottleneck, driven by harsh climatic conditions (Fort et al., 2009). The NL Shelf supports significant concentrations of fish and invertebrates year-round (Fuller and Myers, 2004), including capelin (Mallotus villosus) and sandlance (Ammodytes spp.) that are the preferred prey of murres during the summer breeding season (Davoren and Montevecchi, 2003; Burke and Montevecchi, 2008). Capelin concentrate in large inactive schools in cold, deep water (>200 m; Winters, 1970; Lily, 1982) during winter and attain maximum somatic lipid content (Montevecchi and Piatt, 1984), but may be outside the maximum diving range of murres. Sandlance occupy relatively shallow plateau areas of the southeastern Grand Bank (<80 m maximum) where they partially burrow in the substrate during spawning (Nov–Jan; Winslade, 1974; Winters, 1983) and are well within the foraging range of murres. Information on the winter diets of murres in the Northwest Atlantic is sparse and comes primarily from birds taken during the inshore “turr” hunt (Gaston et al., 1983; Elliot et al., 1990; Rowe et al., 2000; Moody and Hobson, 2007). Diet samples from the hunt are biased to conspecific Thick-billed Murres (Uria lomvia) that generally feed at a relatively lower trophic position (McFarlane-Tranquilla, 2014), and to juvenile murres (both species) that comprise the highest proportion of murres taken in the hunt but represent 16% of the at sea population in winter (Elliot et al., 1990). While these studies indicate a mixed diet of fish (capelin, sandlance and Arctic cod Boreogadus saida) and zooplankton (Parathemisto spp., Thysanoessa spp.) they may not be entirely representative of the winter diets of adult Common murres in offshore waters.
We test the hypothesis that murres will respond to harsh environmental conditions in winter through increased DEE (and nutritional requirements), which will be met through adjustments in daily foraging effort and dive tactics (i.e., how, when, and where). Our overall objective is to gain insights into the behavioral strategies that mediate survival of murres during long, harsh North Atlantic winters.
Materials and Methods
Ethics Approval Statement
This study was carried out in strict accordance with ethical guidelines outlined by the Canadian Council on Animal Care, and approved by Memorial University of Newfoundland's Institutional Animal Care Committee (Permit Numbers: 10-01-WM, 11-01-WM, 12-01-WM, 13-01-WM). Fieldwork was carried out under a Canadian Wildlife Service Migratory Bird Banding permit WAM-10322K. Access to the Funk Island and Witless Bay Islands Provincial Seabird Ecological Reserves was permitted through the Newfoundland and Labrador Parks and Natural Areas Division.
Study Sites and Species
Fieldwork focused on breeding murres at two Northwest Atlantic colonies in Newfoundland, Canada: Gull Island in the Witless Bay Ecological Reserve (47°16′N, 52°46 W) with c.a. 1,632 breeding pairs (Robertson et al., 2003) and the Funk Island Ecological Reserve (49°45′N, 53°11′W) with c.a. 472,259 pairs (Wilhelm et al., 2015). Lotek dive-immersion, geo-locators (Model LAT 2500; 5.9 g with attachment, c.a. 0.7% body mass; 8 × 35 mm, cylindrical in shape) were attached to plastic leg bands (Pro-Touch Engraving) with cable ties, and placed on the left leg of 51 actively breeding murres during late chick-rearing: 15 at Funk Island (2009) and 36 at Gull Island (2010-2013). Twenty-nine devices were retrieved in subsequent years (3 of 15 at Funk Island and 26 of 36 at Gull Island) including four with data failures (final sample of 25 individuals). Low logger return rates at Funk Island (3 of 15 retrieved) was due to the presence of an Arctic Fox that caused significant disturbance and breeding failures at this typically mammalian predator free offshore colony (detailed in Burke et al., 2011). Of these, the 17 loggers that recorded dive records for more than 30 continuous days were included in our analysis of winter foraging behavior (Supplementary Material S1). Winter is defined as the period from 15 November to 15 February (c.a. 93 days), a delineation that ensures all individuals had completed pre-basic molt (conservatively estimated to end in mid-November; Burke, CMB, unnpubl data) and that our late winter sample included > 8 individuals. Specific details regarding capture and handling are provided in Burke et al. (2015).
Device Effects
The mass of the Lotek 250A geo-locator model used in this study (5 g with attachment) is between 0.6 and 0.7% of the average body mass of logger-equipped murres, and is well below the recommended 1–3% (Phillips et al., 2003; c.f. Vandenabeele et al., 2012). Similarities in body mass between logger-equipped (982.6 ± 6.7 g) and control birds (977.1. ± 11.9 g) suggest negligible device effects, and therefore we assume that behavioral information gathered from our logger-equipped birds reflects normal behavior of adult murres.
Spatial Data Processing
Comparison of raw Lotek positions with those generated from British Antarctic Society (BAS) geolocators (McFarlane-Tranquilla, 2014) deployed on Common murres at the same colonies (Gull Island and Funk Island) revealed a striking difference in latitude. Based on the approach of Frederiksen et al. (2016), we ran latitudinal adjustments on our data, the details of which are explained in Supplementary Material (S2). Further processing followed the methods outlined by Burke et al. (2015) involving a two position smoothing, and exclusion of data points representing improbable daily movements (i.e., >500 km/day; Hedd et al., 2011; McFarlane-Tranquilla et al., 2013). The total number of retained, post-processing winter positions represented 67% (n = 1034 days) of the original 1,544 raw positions (n = 17 individuals). Monthly kernal home ranges were evaluated for unsmoothed positions using a least squared cross validation method with a 50 km grid size, applying the “kernelUD” function in the “adehabitatHR” package (Calenge, 2006) in Cran R (ver. 3.1.3), and 50% kernal density contours were used to represent the core winter foraging areas (Linnebjerg et al., 2013).
Environmental Features
Sea surface temperatures (SST) were extracted to the daily locations of individual birds using the Marine Geospatial Ecology Tools (MGET; version 0.8a64) in ESRI ArcGIS (ver 10). SST is an Aqua MODIS product with a daily, 9-km resolution. Habitat variables for missing days (i.e., dates with inaccurate locations) were extracted to a median weekly location for each individual. Estimates of day length were derived for all individuals on a daily basis using the “twilight” function in the R package “GeoLight” (Lisovski and Hahn, 2013) that uses latitude and longitude (from geologgers) to calculate time of nautical sunrise and sunset on a given date. Bathymetry data were also extracted from ETOPO2 grids (http://www.ngdc.noaa.gov/mgg/global/etopo2.html) at a 0.2° degree resolution using the xtracto function in the “xtractomatic” R package.
Activity-Specific Daily Energetic Expenditures
DEE was calculated by combining time-activity budgets with model-derived estimates of activity-specific energy expenditures from Elliott et al. (2013), using a modified activity-specific energy expenditure equation from Elliott and Gaston (2014):
where, Tf is hours spent flying per day, Duration is dive time in minutes, Ts and Tw represent hours per day spent active and inactive on the water respectively, and T = SST (Aqua MODIS). The activity-specific metabolic rates for Tw and Ts are reversed from the original equation (cf (Elliott and Gaston, 2014), pers. comm. K. Elliott, 2016) and our equation excludes the colony-specific metabolic value for time spent at the colony (33Tc).
Daily time-activity budgets were calculated for individual murres using logger derived estimates of daily time spent diving >3 m (i.e., total time submerged >3 m or greater) and flying (total dry time from wet-dry activity), with the remaining time assumed to represent daily time spent on the water. During winter when birds are exclusively at sea, estimates of the daily time spent flying and resting on the water can be accessed via immersion data (wet-dry) where dry events indicate flight. However, for murres and other alcids delineation of flight behavior using this approach is confounded by occasional leg-tucking behavior when birds resting on the water withdraw their leg and foot into their plumage (Harris et al., 2009; Linnebjerg et al., 2014). This behavior can lead to spurious identification of flights, resulting in over-estimation of the total daily time spent flying. We programmed our loggers to eliminate dry records associated with leg-tucking behavior (detailed in Burke et al., 2015), which was successful for 8 individuals only (possibly due to inaccurate temperature reading for some loggers). We restrict our activity-budget analysis to these eight birds for which we are certain that dry periods during the day represent flight time and not some combination of flight and leg-tucking time.
Our modified DEE equation, based on Elliott and Gaston (2014) differentiates time spent on the water into active (i.e., swimming, preening) and inactive (i.e., rest) periods, where time spent with leg(s) tucked is assumed to be equivalent to rest time on the water (Elliott and Gaston, 2014). Because our sample (n = 8 individuals) does not include information on time spent resting (tucking), we relied on estimates from Elliott and Gaston (2014) for Thick-billed murres based on mean time spent with leg(s) tucked according to time of day by month (Sept-Dec; from their Figure 2). We calculated the average time spent with leg(s) tucked during the day and night for November and December that we applied across all our winter data (15 Nov−15 Feb). This resulted in approximate estimates of 30.5 ± 1.8 and 5.8 ± 1.9% of time spent with leg(s) tucked during the night and day, respectively.
Foraging Behavior of Wintering Murres
Explanation of sampling rates, dive and bout processing procedures are provided in detail in Burke et al. (2015). Post-dive intervals >1800 s (30 min) representing 6.7% of all dives by number (n = 83,703) were excluded from analysis of inter-bout rest time since pauses >30 min were conservatively deemed to be related to inter-foraging activity (i.e., flying or resting on surface). Individual dives were assigned to a specific light phase (i.e., daylight, twilight, and night) as defined by sun-angle position (day: sun angle >0°; twilight: sun angle ≤ 0° and >−12°; night: sun angle ≤ −12°). This was calculated using astronomical models (Regular et al., 2011) using an R script validated by P. Regular. Dives that exceeded 162 s were considered anaerobic dives, based on the calculated aerobic dive limit of breeding Thick-billed Murres (Elliott et al., 2013).
Data Analysis
Seasonal changes in foraging behavior and the DEE of murres were examined using linear mixed effects models (LME), fit by restricted maximum likelihood. Mixed modeling was used to account for potential pseudoreplication, with individual set as a random effect, and the inclusion of an autocorrelation term where deemed necessary (evaluated with auto-correlation plots of residuals; Zuur et al., 2009). When assumptions of parametric tests were violated, non-parametric tests were used. F-tests were used to assess the significance of effects and model fits were assessed using parameter estimates (±95% upper and lower confidence intervals). All statistics were run in Cran R software, and unless stated otherwise, values are presented as means ± standard error.
Winter Mass of Hunted Murres (Uria spp.)
To investigate murre condition in winter, we calculated mean body mass from a sample of winter birds collected by the Canadian Wildlife Service during the Newfoundland hunt of murres (“turr hunt”). Though the sample (n = 145; over 9 years between 1985 and 2015) is weighted to juvenile murres (74%) that dominate the age structure of hunted birds, Gaston et al. (1983) demonstrated very similar winter body mass dynamics for adult and juvenile (for Thick-billed murres). Therefore, age classes were grouped and mean body mass was assessed according to early (Nov-Dec) and late winter (Jan-Feb). Differences in body mass between early and late winter were assessed using a one-way ANOVA.
Results
Core Winter Habitat
Murres over-wintered in offshore waters on the Newfoundland Shelf (Figure 1). During November and December, murres were highly concentrated over the northeastern Grand Bank (kernal home ranges: 134,390 and 193,036 km2, respectively). Kernal home ranges increased in January (316,597 km2) and February (459,128 km2) as murres expanded their range to inshore waters (Figure 1). Murres foraged in waters ranging between 50 and 300 m throughout winter (96.1 ± 1.5 m) with no significant effect of month on bathymetric depth (P < 0.3).
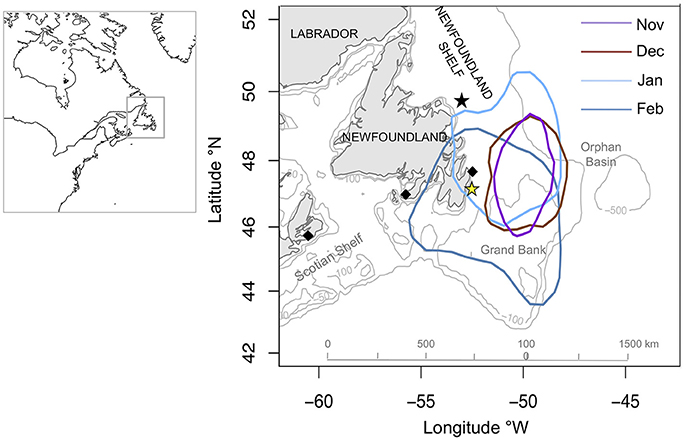
Figure 1. Core foraging areas (50% KHR) of Common Murres (n = 17) according to month. Colored stars indicate Funk Island (black) and Gull Island (yellow). Bathymetric contours (by 100 m) obtained from GEBCO Digital Atlas (GEBCO 1-min grid, ver. 2, www.gebco.net) and mapped in Cran R (ver. 3.1.3).
Activity Specific Daily Energy Expenditure (DEE)
Murres spent on average <2% (1.6 ± 0.1%) of their time flying in winter (4.1 ± 0.1% of daylight hours) and 9.6 ± 0.2% of their time diving, with the remaining time spent on the water (17.3 ± 0.03% resting and 71.5 ± 0.2% active). Mean DEE increased significantly over winter [F(1, 643) = 319.2, P < 0.0001] and exceeded 7 X BMR (>2450 kJ day−1) throughout most of January and February (2463.2 ± 10.9 kJ day−1; Figure 2). The maximum time spent resting scenario (12% day, 37% night) resulting in lower DEE values (Figure 2), with an average difference of 138 kJ day−1 (93–168 kJ day−1) between the maximum and minimum scenarios.
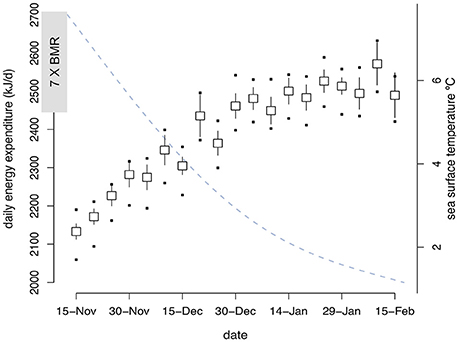
Figure 2. Mean (± SE) daily energy expenditure (DEE) of murres (n = 8) over winter (15-Nov−15 Feb) averaged over 5-day intervals. The dotted blue line shows associated daily sea surface temperature (from generalized additive mixed model output). Black symbols indicate estimated DEE using minimum and maximum time spent resting (on the water) scenarios.
Daily Time Spent Diving
All murres dove every day in winter with total daily dive time ranging from 10 to 468 min. Mean daily time spent diving (i.e., time spent submerged >3 m) increased significantly over winter [F(1, 1273) = 260.2, P < 0.0001], with no significant effect of year (P = 0.6), colony (P > 0.09), or sex (P > 0.84). Murres increased daily dive time at an average rate of c.a. 4 min day−1 before reaching a plateau around winter solstice (Figure 3). This trend highlights two potentially distinct phases of winter during which murres may experience different foraging conditions and (or) nutritional demands. Consequently, we consider differences in behavior between these two unique phases of winter in all further analyses; defined hereafter as early winter (EW: 15 Nov−21 Dec) and late winter (LW: 22 Dec−15 Feb) according to the timing of winter solstice (c.a. 21 Dec) after which day length begins to gradually increase.
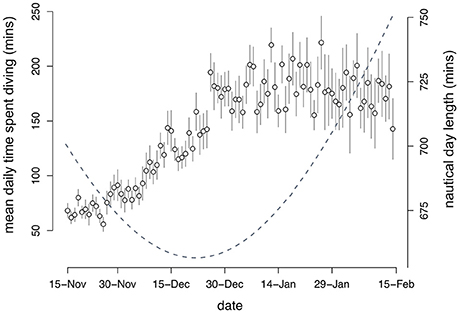
Figure 3. Mean (SE) daily dive effort (minutes) by Common murres (n = 17) in winter (15 Nov−15 Feb). Continuous line (dashed gray) shows day length (minutes) at 47.6° N from nautical sunrise to nautical sunset. Linear mixed model output for differences in mean daily dive effort during EW (95.2 ± 5.6 min; 95% CI: 84.2–106.3 min) and LW (178.3 ± 6.3 min; 95% CI: 165.9–191.0 min).
Individual Dive Characteristics
Eighty three thousand seven hundred and three dives (>3 m) were recorded over winter. As predicted, murres dove deep in winter with 65.2% of all dives ≥50 m. The mean percent frequency of dives peaked at intermediate depths (50–80 m) during both winter phases (Figure 4), with intermediate dives accounting for 54.7 ± 2.7 and 56.5 ± 4.7% of all dives in EW and LW, respectively. The percent frequency of shallow dives (<50 m) decreased over winter (40.5 ± 3.2 and 29.8 ± 5.2% in EW and LW, respectively) while the frequency of deep dives (>80 m) increased (4.9 ± 1.6 and 13.7 ± 4.1% in EW and LW, respectively). Overall there was a significant increase in mean dive depth (daily average) over winter [F(1, 1273) = 167.1, P < 0.0001] and dives were significantly deeper in LW (59.8 ± 1.2 m; 95% CI: 56.0–63.8 m) relative to EW (49.9 ± 1.9 m; 95% CI: 46.0–53.7 m). Figure 5 presents information on individual dive characteristics as a function of dive depth during EW and LW (categorized by shallow, intermediate, and deep dives). Mean dive duration increased with depth and was significantly higher for intermediate dives in LW (155.8 ± 6.0 s; Figure 5A) relative to EW (143.8 ± 4.1 s; Figure 5A). Percentage of dives exceeding the calculated aerobic dive limit for murres (162 s) accounted for 16.7 ± 3.0% of dives in EW vs. 37.4 ± 5.4% in LW, with high variability between individuals (Supplementary Table S1). Mean bottom duration of dives peaked at intermediate depths during EW and LW (Figure 5B). Bottom duration was significantly higher in LW for intermediate (45.5 ± 1.6 and 56.1 ± 1.3 s for EW and LW, respectively) and deep dives (40.3 ± 1.5 and 49 ± 1.9 s for EW and LW, respectively; Figure 5B). Mean descent rate decreased significantly with depth (Figure 5C) with significantly higher values during LW for intermediate (122.8 ± 1.6 and 128.7 ± 0.8 cm/s for EW and LW) and deep dives (132.3 ± 1.0 and 139.6 ± 1.2 cm/s for EW and LW, respectively). Mean bottom velocity followed a reverse trend to bottom duration. During EW, bottom velocity of intermediate dives was significantly lower (10.5 ± 0.4 cm/s, Figure 5D) than shallow (17.8 ± 0.6 cm/s) and deep dives (14.9 ± 0.9 cm/s). During LW, mean bottom velocity of shallow dives was significantly higher (15.8 ± 0.5 cm/s; Figure 5D) relative to intermediate (11.0 ± 0.6 cm/s) and deep dives (11.8 ± 1.0 cm/s) that were not different. There was no significant phase effect on mean bottom velocity for shallow or intermediate dives, but mean bottom velocity of deep dives was significantly higher in EW (14.9 ± 0.9 cm/s) than LW (11.8 ± 1.0 cm/s; Figure 5D). Mean ascent rate increased significantly with depth (in both phases), with overlapping ascent rates between EW and LW at all depths (Figure 5E).
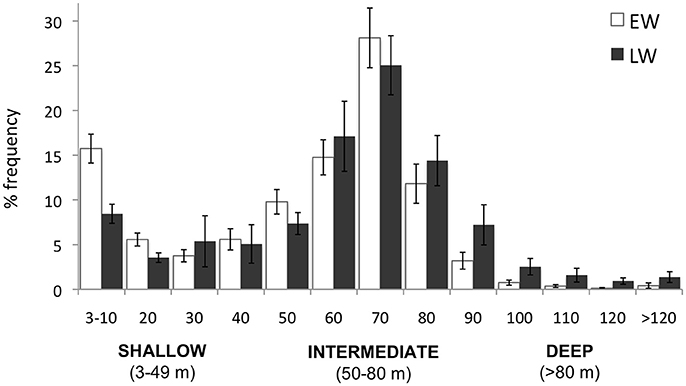
Figure 4. Percentage dive depth frequency according to 10-m depth bins. Values are mean percent frequency of dives averaged across individuals in early winter (EW; white bars) and late winter (LW; black bars).
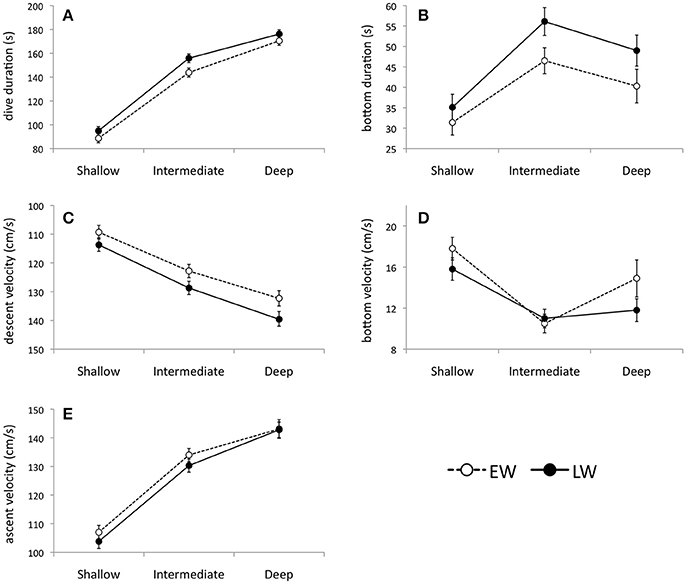
Figure 5. Individual dive characteristics according to shallow (3–49 m), intermediate (50–80 m), and deep (>80 m) depth intervals during early winter (EW) and late winter (LW). Parameters include: (A) dive duration (n = 73946; dives ≥10 and ≤150 m), (B) bottom duration (n = 72108 dives; dives ≥10 and ≤150 m, excludes V dives), (C) descent velocity (n = 66370 dives; dives ≥30 and ≤150 m), (D) bottom velocity (n = 70586 dives; dives ≥10 and ≤150 m, excludes V dives) and (E) ascent velocity (n = 66352 dives; dives ≥30 and ≤150 m), and (F) inter-bout pause duration (n = 39925 bouts, pauses ≤1800 s). Values are linear mixed model and generalized linear mixed model (where appropriate) model fits ± 95% confidence intervals where non-overlapping confidence intervals indicate significant differences. Note: due to high variability in the parameter estimates for shallow dives, dive data were truncated by depth to overcome problems with heterogeneity (≥10 m, except ≥30 m for ascent and descent velocities).
Diel Dive Behavior
The majority of dives occurred during daylight hours throughout winter (Figure 6; 76.0 ± 1.9 and 85.7 ± 1.6% of all dives in EW and LW, respectively), however the proportional frequency of daylight dives increased over time as twilight and night dives decreased (Figure 6A). Twilight dives accounted for 14.5 ± 0.9% in EW and 10.9 ± 0.7% in LW and night dives accounted for 9.6 ± 1.7% in EW and 3.3 ± 1.3% in LW. During EW, daylight dives were significantly deeper (54.7 ± 2.1 m; 95% CI: 50.7–58.8 m) than twilight (46.1 ± 2.1 m; 95% CI: 42.1–50.2 m) and night dives (28.5 ± 2.1 m; 95% CI: 24.4–32.6 m; Figure 6B). Mean depth of daylight (63.4 ± 4.4 m; 95% CI: 54.9–72.0 m) and twilight dives (57.1 ± 4.4 m; 95% CI: 48.5–65.6 m) was not significantly different in LW (Figure 6C), and both were significantly deeper than night dives (34.6 ± 4.5 m; 95% CI: 25.8–43.4 m).
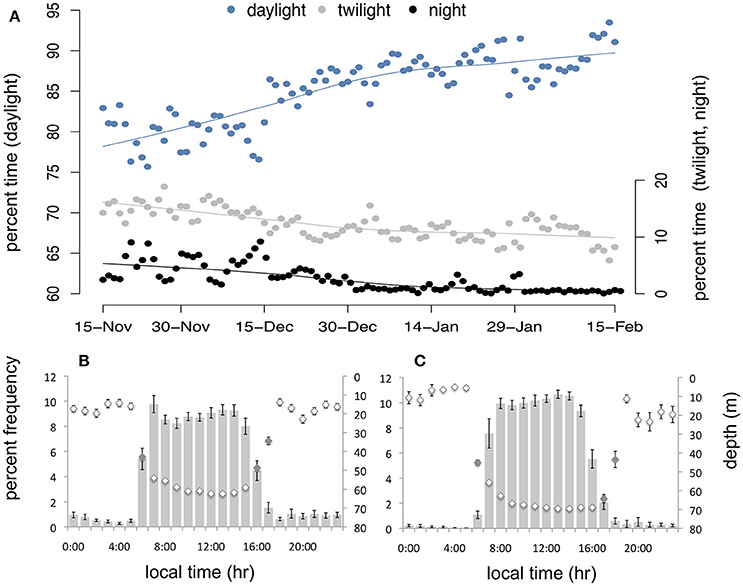
Figure 6. (A) Proportion of total daily dive activity occurring during daylight (blue), twilight (gray), and night (black) over time in winter (with a loess smoothing function for each light period). Mean percentage number of dives (bars) and mean dive depth (diamonds; inverted y-axis) according to time of day (local time) during early winter (B) and late winter (C). Gray diamonds (B,C) indicate approximate transitional hours during twilight (i.e., sun angle ≥-12 and ≤0).
Organization of Foraging Bouts
Forty five thousand five hundred and forty three foraging bouts were recorded over winter, the majority of which consisted of a single dive: 74.3% in EW and 80.5% in LW. There were significantly more dive bouts per day in LW (41.7 ± 2.0; 95% CI: 37.7–45.7) relative to EW (28.0 ± 2.0, 95% CI: 24.0–32.0; Table 1). Mean duration of dive bouts containing multiple dives (excluding post-pause time of terminal dives) was significantly higher in LW (831.2 ± 2.4 s; 95% CI: 826.6–835.9 s) relative to EW (595.7 ± 1.8 s; 95% CI: 592.1–599.2 s; Table 1). Similarly, mean bout duration of single dive bouts was significantly higher in LW (154.7 ± 0.6 s; 95% CI: 153.6–155.9 s) relative to EW (130.1 ± 0.5 s; 95% CI: 129.3–130.9 s). The time spent resting on the water between dive bouts (for single and multiple dive bouts) was also lower in late winter (309.5 ± 15.2 s) relative to EW (364.0 ± 15.3 s), but the difference was not significant (Table 1).
Winter Mass of Hunted Murres
Murres collected during the Newfoundland “turr” hunt were significantly heavier [F(1, 143) = 4.6, P = 0.03] in late winter (Jan-Feb: 1043.8 ± 7.1 g), relative to early winter (Nov-Dec: 988.8 ± 23.6 g).
Discussion
Seabirds over-wintering at high latitudes face the double jeopardy of high DEE and degraded foraging conditions. Winter survival strategies while poorly known are vital aspects of the annual cycle of marine birds (Frederiksen et al., 2008). Using fine-scale behavioral information gathered from pursuit-diving murres equipped with dive-immersion geo-locators, we demonstrate overlapping trends in mean DEE (Figure 2) and mean daily time spent diving (Figure 3) over winter, both showing a steep increase through EW followed by a LW asymptote. These results support our prediction that an increase in DEE (and nutritional requirements) would be met by a corresponding increase in foraging effort, and balanced energy budgets. Patterns in the diving profiles of murres also indicate a distributional shift in prey over winter (from shallow to deep waters), likely driven by seasonal declines in water temperature. We show that murres meet their daily energy requirements in the face of these challenges by pushing the limits of their diving capabilities. Murres are the largest of the pursuit-diving alcids, and due to small wings (that reduce underwater drag) and physiological adaptations that minimize metabolic costs during diving they can dive deeper, longer, and more efficiently than any bird capable of flight (Elliott et al., 2013). These traits afford murres the flexibility to routinely access prey in deep water and explain, in part explain how they survive the challenges of winter on the NL Shelf.
Daily Energy Expenditures (DEE) of North Atlantic Murres in Winter
Mean DEE of murres during LW exceeds the sustainable level of 7 X BMR for vertebrates (Weiner, 1992; Speakman and Król, 2011). This finding supports previous studies showing higher than expected energy and nutritional requirements for diving seabirds in winter (Grémillet et al., 2005a; Daunt et al., 2007; Fort et al., 2009) and more specifically for North Atlantic alcids (Fort et al., 2009, 2013; Elliott and Gaston, 2014). Diving birds have significant energy requirements for thermoregulation in winter owing to high heat loss during exposure to cold water (Croll and McClaren, 1993; Enstipp et al., 2006). Murres in our study were largely flightless throughout winter (<5% time spent flying) and spent most of their time on the water (>85% of the day in LW). Accordingly, as SST decreased through winter murres experienced a corresponding increase in thermodynamic costs with high DEE estimates throughout LW when SST was consistently near freezing (1.9 ± 0.8°C). Murres also spent more time diving in LW, however diving expenditures contributed relatively little to overall DEE (12 ± 0.3%), presumably due to low metabolic activity during deep-diving (Wilson et al., 1992; Niizuma et al., 2007; Elliott et al., 2013).
While we took great care in our approach and used the most current information available, we consider specific limitations and assumptions that may bear, in particular on the magnitude of our DEE estimates that are significantly higher than those reported by Fort et al. (2009) for Thick-billed Murres during North Atlantic winters. The applied DEE equation (Equation 1, adapted from Elliott and Gaston, 2014) incorporates thermodynamic costs during rest and activity periods on the water (based on SST data extracted from Aqua MODIS using the daily locations of logger-equipped murres). Activity-specific metabolic rates are derived from studies of captive murres held in 16°C water (Croll and McClaren, 1993), and could therefore overestimate the thermoregulatory costs of wild birds acclimated to cold water (e.g., 0–12°C seasonal range for Northwest Atlantic Common murres; McFarlane-Tranquilla, 2014). We also acknowledge that activity-specific metabolic rates, derived from breeding birds may not be representative of the winter period when seasonal variations in body mass and composition (lean mass vs. lipid loading) could influence diving and flight costs (Elliott et al., 2008b). Indeed, the effects of seasonal physiological adjustments on the metabolic machinery of murres are largely unaccounted for in our study. For example, it is possible that if seasonal increases in DEE are sufficiently predictable; adjustments in the size of digestive organs (occurring over sufficient time) would allow murres to increase their energy assimilation rates (Wu et al., 2014) and to function at a higher level of energy expenditure. In addition, assumptions regarding the estimated time spent resting vs. time spent active on the water could also influence our DEE results. Our activity data did not provide reliable estimates of time spent resting since we programmed our loggers to eliminate tucking behavior (assumed to represent rest time; Elliott and Gaston, 2014). Therefore, we relied on estimates of time spent with leg(s) tucked from Elliott and Gaston (2014) for Thick-billed murres, with average values of 5% (day) and 30% (night) respectively. To assess how variability around these assumptions influenced our DEE estimates, we calculated minimum and maximum time spent resting scenarios (also based on Elliott and Gaston, 2014) using 25% (minimum) and 75% (maximum) inter-quartile ranges of mean percent time spent with legs tucked during the day (0.1 and 11.7% for minimum and maximum, respectively) and night (23.9 and 37.0%, for minimum and maximum, respectively), averaged over the entire winter period. The maximum resting scenario resulted in lower DEE since correspondingly less time is spent active on the water (which involves higher energy costs), and the average difference between the maximum and minimum time spent resting scenarios was 138 kJ day−1 (93–168 kJ day−1). The reality of these scenarios for murres in the Northwest Atlantic is largely unknown, however Robertson et al. (2012) estimated “many hours” of dry records at night (assumed to represent tucking) for murres equipped with time-depth recorders. Therefore, it is likely that the mean (30% or 3.5 h tucking) or maximum (37% or 4.5 h) time spent resting scenarios are the most realistic. Given these assumptions, we acknowledge that our DEE results are largely “theoretical” and should be considered as best current estimates. Nonetheless we emphasize the striking overlap in seasonal DEE trends with Fort et al. (2009) and the similarly high values reported by Elliott and Gaston (2014) for Thick-billed murres during December; all of which support the notion of late winter as an extremely challenging phase in the annual cycle of North Atlantic murres.
Optimal Foraging Strategies in a Marginal Winter Environment
Prey behavior influences the foraging strategies of diving predators. In particular, the vertical distribution of prey in the water column determines dive depth and to a large extent, the duration of time spent feeding at depth. Water masses on the NL Shelf undergo pronounced seasonal modifications in their properties due to heat flux, wind-forced mixing and shifting ice extent (Colbourne et al., 2015; Richaud et al., 2016). Over the progression of winter, the vertical extent of warm water in the upper water column diminishes, and by late December cold water persists throughout the water column (Petrie et al., 1988; Richaud et al., 2016). When surface waters are cold, prey that otherwise migrate from deep waters during the day into warm surface waters at night (i.e., diel vertical migration) remain at depth. This could explain the observed decrease in the frequency of shallow dives in LW (Figure 4) and the corresponding increase in daylight dives (with fewer crepuscular and nocturnal dives). Consequently, murres facing high-energy demands in LW spend more time foraging in deeper waters where capture efficiency of prey is limited by light availability (Hedd et al., 2009; Regular et al., 2011), resulting in a shorter foraging day (Figure 6B).
We suggest that murres operating under severe time (short days) and energy (high DEE, deep diving) constraints in LW push the limits of their diving capabilities to maximize their total net daily energy gain (i.e., rate maximizing behavior; Houston, 1987; Ydenberg et al., 1994). Specifically, they spend more time feeding in productive areas and dive intensively where and when foraging efficiency is maximized. Murres dove most frequently to intermediate depths (50–80 m) throughout winter (Figure 4), which suggests that prey was most abundant or of the highest quality within this foraging zone. Though we have no corresponding information on winter diets, intermediate diving would potentially place murres within range of sandlance (and possibly cod, sculpin Cottidae spp.; Winters, 1970, 1983; Lily, 1982; Montevecchi and Piatt, 1984) that occur over large portions of the Grand Bank (<100 m; Figure 1). Congeneric Thick-billed Murres on the NL Shelf were estimated to consume approximately half their body weight (c.a. 550 g) in wet food every day (Fort et al., 2009) during LW, which we assume is a realistic estimate for our murres. Forage fish are energy-dense and would be highly suitable prey for murres facing such demanding nutritional requirements. They are also rich in lipids, which make them profitable from an energy assimilation perspective since lipid-rich prey have relatively higher assimilation efficiencies (Brekke and Gabrielsen, 1994). For murres that are primarily restricted to daylight diving in winter (see below), the ability to quickly process prey may be critically important to attaining sufficient energy reserves within a relatively limited foraging window.
Although murres performed fewer shallow and more deep dives in late winter (Figure 4), there was no change in the frequency of intermediate dives (55 ± 2.7 and 57 ± 4.7% in EW and LW, respectively). They did however spend significantly more time on the bottom phase of intermediate dives in LW (Figure 5B), 43% of which exceeded their aerobic dive limit (ADL). Frequent anaerobic diving results in acidotic blood and eventual fatigue (Butler, 2001) and is considered unsustainable, yet murres and many diving species regularly exceed ADL during deep diving (e.g., 40% for King Penguin Aptenodytes patagonicus; Butler, 2001). Theoretical explanations as to why (rather than how) divers frequently exceed their ADL involve energy tradeoffs whereby individuals extend the duration of dives when the quality or density of prey is sufficiently high to ensure higher capture success and energy intake (Ydenberg and Clark, 1989; Kooyman and Ponganis, 1998). Accordingly, we suggest that the high frequency of ADL dives at intermediate depths in LW reflects such a trade-off whereby murres facing high nutritional requirements extend feeding times at high quality prey patches (i.e., 50–80 m or intermediate depths) where energy intake per unit of time is maximized. Murres also exceeded their ADL during 85% of deep dives (n = 6497) in LW, however time spent on the bottom of deep dives was significantly lower relative to intermediate dives (Figure 5B) and suggests travel time (vs. feeding time) explains the high frequency of ADL dives in deep water. To our knowledge, the high frequency of ADL dives by murres in LW (36% over all dives) exceeds previous records (21% during chick-rearing; Elliott et al., 2008a), most of which come from the breeding season. Consequently, an increase in the frequency of ADL dives could represent an important behavioral adjustment for winter survival (possibly facilitated by physiological adjustments; Gerlinsky et al., 2013).
We also show that visually-oriented murres respond to an increasingly deep-water prey field in LW by increasing diving intensively during daylight hours (Figure 6). During LW, murres exhibited significant increases in mean dive (and dive bout) frequency and duration (relative to EW) and a corresponding decrease in inter-bout rest time (Table 1). While the difference in inter-bout rest times between EW and LW was not significant (Table 1), it is striking that murres that are diving more (and frequently exceeding ADL) are spending less time resting at the surface between dive bouts. Presumably, murres are expending maximum effort when foraging efficiency is highest (i.e., during daylight hours) which allows them to satisfy their significant food energy requirements when visually hunting for prey in deep water.
Intensive diurnal foraging (possibly consuming lipid rich prey) could also allow murres to gather surplus energy reserves to sustain them during nocturnal fasting (McNamara and Houston, 2008). This behavior could explain our finding of significantly heavier birds in LW (>1000 g) that is supported by frequent reports of relatively heavy seabirds in winter (e.g., murres and kittiwakes; Erikstad, 1990; Falk and Durnik, 1993; Harris et al., 2000) including an observation of murres collected during the Newfoundland “turr” hunt carrying heavy subcutaneous fat (Gaston et al., 1983). Accumulation of lipid reserves would also improve insulation and significantly reduce heat loss to the water (Richman and Lovvorn, 2011) resulting in potentially lower thermodynamic costs (and DEE). Whether murres carry enough reserves to sustain them through more prolonged periods of fasting is uncertain, however the occurrence of large numbers of dead and starving alcids coming ashore following extreme weather events (winter wrecks) suggests that the amount of reserves that these relatively small birds can carry is insufficient over extended periods, particularly in late winter when energy demands are high.
Conclusions
Our results contribute to our understanding of the tolerances of murres in response to a prolonged and demanding LW period in a Low Arctic ecosystem, and demonstrate that murres are capable of coping with predictable, seasonal environmental extremes over an extended period. During the LW period when energy demands are high and foraging conditions are relatively poor, murres push the physiological limits of their diving capabilities to balance their energy budgets. Evidence from studies on foot-propelled cormorants indicate that although birds can sustain high rates of feeding activity when energy demands are high in winter, they deplete reserves over time and come close to, or succumb to starvation by early spring (Grémillet et al., 2005a; Daunt et al., 2007). The physiological consequences associated with intensive foraging during a prolonged period of peak energy demand are unclear but if murres are working at maximum capacity, predicted extremes in environmental conditions associated with future climate change (Reid and Valdés, 2011) could have potentially catastrophic consequences for winter survival, particularly if prey conditions are negatively affected. Future research investigating the physiological mechanisms that drive such extreme behavioral responses are needed to better understand and predict the tolerances of murres to environmental extremes and variability.
High DEE during late winter for related alcids (Fort et al., 2009, 2013; Elliott and Gaston, 2014) suggests the existence of a potential energy bottleneck for these pursuit divers, driven by climatic factors during long, harsh North Atlantic winter. Our results indicate that murres can, and indeed need to forage intensively on a consistent schedule to avoid an energy bottleneck in LW. Whether, other alcids rely to such an extent on intensive feeding to sustain them during periods of extreme energy demand is unclear, and requires further study.
Author Contributions
CB and WM conceived and designed the telemetry study. CB analyzed and interpreted the telemetry data, and devised the hypotheses. Both authors contributed to the writing and editing of the submitted manuscript.
Funding
Funding for this project was provided by National Science and Engineering Research Council of Canada (CB), NSERC Discovery Grant (WM), and the Newfoundland and Labrador Murre Fund Grant (WM).
Conflict of Interest Statement
The authors declare that the research was conducted in the absence of any commercial or financial relationships that could be construed as a potential conflict of interest.
Acknowledgments
We thank N. Laite, A. Hann, and S. Bennett for assistance in the field. Key logistical support was provided by Environment Canada (G. Robertson, S. Wilhelm, P. Ryan). Special thanks to the crews of the Lady Easton (Easton family) and EcoTours for safe transport to and from colonies. We thank P. Regular for the valuable input and insight throughout the progression of the manuscript. We thank M. Frederikson for providing a copy of his R script and L. McFarlane-Tranquilla for access to BAS data to run adjustments on Lotek positions. G. Robertson (Env. Can.) and S. Wilhelm (CWS) also generously provided data on the winter masses of murres from the Newfoundland Turr hunt. Research support was provided by an NSERC Post-graduate Fellowship to CB, the Graduate School of Memorial University of Newfoundland, and NSERC Discovery Grants to WM.
Supplementary Material
The Supplementary Material for this article can be found online at: https://www.frontiersin.org/articles/10.3389/fmars.2018.00063/full#supplementary-material
References
Berge, J., Renaud, P. E., Darnis, G., Cottier, F., Last, K., Gabrielsen, T. M., et al. (2015). In the dark: a review of ecosystem processes during the Arctic polar night. Prog. Oceanogr. 139, 258–271. doi: 10.1016/j.pocean.2015.08.005
Brekke, B., and Gabrielsen, G. W. (1994). Assimilation efficiency of adult Kittiwakes and Brunnich's Guillemots fed Capelin and Arctic Cod. Polar Biol. 14, 279–284. doi: 10.1007/BF00239177
Brooke Mde, L. (2004). The food consumption of the world's seabirds. Proc. R. Soc. 271, 246–248. doi: 10.1098/rsbl.2003.0153
Burke, C. M., Hedd, A., Montevecchi, W. A., and Regular, P. M. (2011). Effects of an Arctic Fox visit to a low arctic seabird colony. Arctic 64, 302–306. doi: 10.14430/arctic4120
Burke, C. M., and Montevecchi, W. A. (2008). Fish and chicks: forage fish and chick success in co-existing auks. Waterbirds 31, 372–384. doi: 10.1675/1524-4695-31.3.372
Burke, C. M., Montevecchi, W. A., and Regular, P. M. (2015). Seasonal variation in parental care drives sex-specific foraging in a monomorphic seabird. PLoS ONE 10:e0141190. doi: 10.1371/journal.pone.0141190
Butler, P. J. (2001). Diving beyond the limits. New Physiol. Sci. 16, 222–227. doi: 10.1152/physiologyonline.2001.16.5.222
Calenge, C. (2006). The package adehabitat for R software: a tool for the analysis of space and habitat use by animals. Ecol. Model. 197, 516–519. doi: 10.1016/j.ecolmodel.2006.03.017
Chimienti, M., Cornulier, T., Owen, E., Bolton, M., Davies, I. M., Travis, J. M. J., et al. (2017). Taking movement data to new depths: inferring prey availability and patch profitability from seabird foraging behavior. Ecol. Evol. 7, 10252–10256. doi: 10.1002/ece3.3551
Colbourne, E., Holden, J., Senciall, D., Bailey, W., Craig, J., and Snook, S. (2015). Physical Oceanographic Conditions on the Newfoundland-Labrador Shelf in 2014. CSAS. Available online at: http://waves-vagues.dfo-mpo.gc.ca/Library/362068.pdf
Croll, D. A., and McLaren, E. (1993). Diving metabolism and thermoregulation in Common and thick-billed Murres. J. Comp. Physiol. B 163, 160–166. doi: 10.1007/BF00263602
Daunt, F., Afanasyev, V., Adam, A., Croxall, J. P., and Wanless, S. (2007). From cradle to early grave: juvenile mortality in juvenile European Shags Phalacrocorax aristotelis results from inadequate development of foraging proficiency. Biol. Lett. 3, 371–374. doi: 10.1098/rsbl.2007.0157
Daunt, F., Afanasyev, V., Silk, J. R. D., and Wanless, S. (2006). Extrinsic and intrinsic determinants of winter foraging and breeding phenology in a temperate seabird. Behav. Ecol. Sociobiol. 59, 381–388. doi: 10.1007/s00265-005-0061-4
Davoren, G. K., and Montevecchi, W. A. (2003). Signals from seabirds indicate changing biology of capelin biology. Mar. Ecol. Prog. Ser. 258, 253–261. doi: 10.3354/meps258253
Elliott, K. H., and Gaston, A. J. (2014). Dive behavior and daily energy expenditure in Thick-billed Murres Uria lomvia after leaving the breeding colony. Mar. Ornithol. 42, 183–189.
Elliott, K. H., Ricklefs, R. E., Gaston, A. J., Hatch, S. A., Speakman, J. R., and Davoren, G. K. (2013). High flight costs, but low dive costs, in auks support the biomechanical hypothesis for flightlessness in penguins. Proc. Natl. Acad. Sci. U.S.A. 110, 9380–9384. doi: 10.1073/pnas.1304838110
Elliot, R. D., Ryan, P. C., and Lidster, W. W. (1990). The winter diet of Thick-billed Murres in coastal Newfoundland waters. Stud. Avian Biol. 14, 125–138.
Elliott, K. H., Davoren, G. K., and Gaston, A. J. (2008a). Time allocation by a deep-diving bird reflects prey type and energy gain. Anim. Behav. 75, 1301–1310. doi: 10.1016/j.anbehav.2007.09.024
Elliott, K. H., Jacobs, S. R., Ringrose, J., Davoren, G. K., and Gaston, A. J. (2008b). Is mass loss in guillemots an adaptation for improved flight performance or improved dive performance? J. Avian Biol. 39, 619–628. doi: 10.1111/j.1600-048X.2008.04316.x
Enstipp, M. R., Grémillet, D., and Jones, D. R. (2006). The effects of depth, temperature and food ingestion on the foraging energetics of a diving endotherm, the Double-crested Cormorant Phalacrocorax auritus. J. Exp. Biol. 209, 845–859. doi: 10.1242/jeb.02064
Erikstad, K. E. (1990). Winter diets of four seabird species in the Barents Sea after a crash in the capelin stock. Pol. Biol. 10, 619–627. doi: 10.1007/BF00239373
Falk, K., and Durnik, J. (1993). The winter diet of Thick-billed Murres, in western Greenland, 1988-1989. Can. J. Zool. 71, 264–272. doi: 10.1139/z93-038
Finney, S. K., Wanless, S., and Harris, M. P. (1999). The effect of weather conditions on the feeding behaviour of a diving bird, the Common Guillemot Uria aalge. J. Avian Biol. 30, 23–30. doi: 10.2307/3677239
Fort, J., Porter, W. P., and Grémillet, D. (2009). Thermodynamic modelling predicts energetic bottleneck for seabirds wintering in the northwest Atlantic. J. Exp. Biol. 212, 2483–2490. doi: 10.1242/jeb.032300
Fort, J., Steen, H., Strom, H., Tremblay, Y., Gronningsaeter, E., Pettex, E., et al. (2013). Energetic consequences of contrasting winter strategies in a sympatric Arctic seabird duet. J. Avian Biol. 44, 255–262. doi: 10.1111/j.1600-048X.2012.00128.x
Frederiksen, M., Daunt, F., Harris, M. P., and Wanless, S. (2008). The demographic impact of extreme events: stochastic weather drives survival and population dynamics in a long-lived seabird. J. Anim. Ecol. 77, 1020–1029. doi: 10.1111/j.1365-2656.2008.01422.x
Frederiksen, M., Descamps, S., Erikstad, K. E., Gaston, A. J., Gilchrist, G., Grémillet, D., et al. (2016). Migration and wintering of a declining seabird, the Thick-billed Murre Uria lomvia, on an ocean basin scale: conservation implications. Biol. Conserv. 200, 26–35. doi: 10.1016/j.biocon.2016.05.011
Fuller, S. D., and Myers, R. A. (2004). The Southern Grand Bank: A Marine Protected Area for the World. Halifax, NS: WWF.
Gaston, A. J., Goudie, R. I., Noble, D. G., and MacFarlane, A. (1983). Observations on “Turr” hunting in Newfoundland: age, body condition, and diet of Thick-billed Murres Uria lomvia, and proportions of other seabirds, killed off Newfoundland in winter. Can. Wildl. Ser. Prog. Notes 141, 1–7.
Gaston, A. J., and Jones, I. L. (1998). Bird Families of the World: The Auks. Oxford: Oxford University Press.
Gerlinsky, C. D., Rosen, D. A., and Trites, A. W. (2013). High diving metabolism results in a short aerobic dive limit for Steller sea lions Eumetopias jubatus. J. Comp. Physiol. B 183, 699–708. doi: 10.1007/s00360-013-0742-7
Green, J. A., Boyd, I. L., Woakes, A. J., Warren, N. L., and Butler, P. J. (2005). Behavioural flexibility during year-round foraging in macaroni penguins. Mar. Ecol. Prog. Ser. 296, 183–196. doi: 10.3354/meps296183
Green, J. A., Boyd, I. L., Woakes, A. J., Warren, N. L., and Butler, P. J. (2009). Evaluating the prudence of parents: daily energy expenditure throughout the annual cycle of a free-ranging bird. J. Avian. Biol. 40,: 529–538. doi: 10.1111/j.1600-048X.2009.04639.x
Grémillet, D., Kuntz, G., Gilbert, C., Woakes, A. J., Butler, P. J., and Maho, Y. (2005b). Cormorants dive through the polar night. Biol. Lett. 1, 469–471. doi: 10.1098/rsbl.2005.0356
Grémillet, D., Kuntz, G., Woakes, A. J., Gilbert, C., Robin, J. P., Le Maho, Y., et al. (2005a). Year-round recording of behavioural and physiological parameters reveal the survival strategy of a poorly insulated diving endotherm during the Arctic winter. J. Exp. Biol. 208, 4231–4241. doi: 10.1242/jeb.01884
Harris, M. P., Daunt, F., Newell, M., Phillips, R. A., and Wanless, S. (2009). Wintering areas of adult Atlantic puffins Fratercula arctica from a North Sea colony as revealed by geolocation technology. Mar. Biol. 157, 827–836. doi: 10.1007/s00227-009-1365-0
Harris, M. P., Wanless, S., and Webb, A. (2000). Changes in body mass of Common Guillemots Uria aalge in southeast Scotland throughout the year: implications for the release of cleaned birds. Ring. Mig. 20, 134–142. doi: 10.1080/03078698.2000.9674235
Hedd, A., Montevecchi, W. A., McFarlane-Tranquilla, L., Burke, C. M., Fifield, D. A., Robertson, G. J., et al. (2011). Reducing uncertainty on the Grand Bank: tracking and vessel surveys indicate mortality risks for Common Murres Uria aalge in the Northwest Atlantic. Anim. Conserv. 14, 630–641. doi: 10.1111/j.1469-1795.2011.00479.x
Hedd, A., Regular, P. M., Montevecchi, W. A., Buren, A. D., Burke, C. M., and Fifield, D. A. (2009). Going deep: common Murres dive into frigid water for aggregated, persistent and slow-moving capelin. Mar. Biol. 156, 741–751. doi: 10.1007/s00227-008-1125-6
Houston, A. I. (1987). Optimal foraging by parent birds feeding dependent young. J. Theor. Biol. 124, 251–274. doi: 10.1016/S0022-5193(87)80115-7
Kooyman, G. L., and Ponganis, P. J. (1998). The physiological basis of diving to depth: birds and mammals. Annu. Rev. Physiol. 60, 19–32. doi: 10.1146/annurev.physiol.60.1.19
Lily, G. (1982). Influence of the Labrador current on predation by cod on capelin and sandlance off eastern Newfoundland. NAFO Sci. Coun. Stud. 3, 77–82.
Linnebjerg, J. F., Fort, J., Guilford, T., Reuleaux, A., Mosbech, A., and Frederiksen, M. (2013). Sympatric breeding auks shift between dietary and spatial resource partitioning across the annual cycle. PLoS ONE 8:e72987. doi: 10.1371/journal.pone.0072987
Linnebjerg, J. F., Fort, J., Guilford, T., Reuleaux, A., Mosbech, A., and Frederiksen, M. (2014). Inferring seabird activity budgets from leg-mounted time–depth recorders. J. Ornithol. 155, 301–306. doi: 10.1007/s10336-013-1015-7
Lisovski, S., and Hahn, S. (2013). GeoLight – processing and analyzing light-based geolocator data in R. Methods Ecol. Evol. 3, 1055–1059. doi: 10.1111/j.2041-210X.2012.00248.x
Longhurst, A. (1995). Seasonal cycles of pelagic production and consumption. Prog. Oceanogr. 36, 77–167. doi: 10.1016/0079-6611(95)00015-1
Maillet, G., and Colbourne, E. (2007). Variations in the Labrador Current Transport Zooplankton Abundance on the NL Shelf. NAFO SCR Doc. 07/42. Ser. No. N5394.
McFarlane-Tranquilla, L. (2014). Ecological Segregation of Murres (Uria lomvia, Uria aalge) During the Nonbreeding Season in the Northwest Atlantic Ocean. Dissertation/Doctoral thesis, Memorial University of Newfoundland, St. John's, NL.
McFarlane-Tranquilla, L. A., Hedd, A., Burke, C. M., Montevecchi, W. A., Regular, P. M., Robertson, G. J., et al. (2010). High Arctic sea ice conditions influence marine birds wintering in Low Arctic regions. Estuar. Coast Shelf Sci. 89, 97–106. doi: 10.1016/j.ecss.2010.06.003
McFarlane-Tranquilla, L. A., Montevecchi, W. A., Hedd, A., Fifield, D. A., Burke, C. M., et al. (2013). Multiple-colony winter habitat use by murres Uria spp. in the Northwest Atlantic Ocean: implications for marine risk assessment. Mar. Ecol. Prog. Ser. 472, 287–303. doi: 10.3354/meps10053
McNamara, J. M., and Houston, A. I. (2008). Optimal annual routines: behaviour in the context of physiology and ecology. Philos. Trans. R Soc. Lond. B Biol. Sci. 363, 301–319. doi: 10.1098/rstb.2007.2141
Montevecchi, W. A. (2000). “Seabirds,” in A Mass Balance Model of the Newfoundland-Labrador Shelf, Canadian Technical Report of Fisheries and Aquatic Sciences, eds A. Bundy, G. R. Lilly, and P. A. Shelton (St. John's, NL), 15–18.
Montevecchi, W. A., and Piatt, J. F. (1984). Composition and energy contents of mature inshore spawning capelin (Mallotus villosus): implications for seabird predators. Comp. Biochem. Physiol. A Mol. Inter. Physiol. 78, 15–20. doi: 10.1016/0300-9629(84)90084-7
Moody, A., and Hobson, K. A. (2007). Alcid winter diet in the Northwest Atlantic determined by stable isotope analysis. Mar. Ornithol. 35, 39–46.
Niizuma, Y., Gabrielsen, G. W., Sato, K., Watanuki, Y., and Naito, Y. (2007). Brünnich's Guillemots Uria lomvia maintain high temperature in the body core during dives. Comp. Biochem. Physiol. A Mol. Integr. Physiol. 147, 438–444. doi: 10.1016/j.cbpa.2007.01.014
Olsen, E., Aanes, S., Sigbjørn, M., Holst, J. C., Aglen, A., and Gjøsæter, H. (2010). Cod, haddock, saithe, herring and capelin in the Barents Sea and adjacent waters: a review of the biological value of the area. ICES J. Mar. Sci. 67, 87–101. doi: 10.1093/icesjms/fsp229
Petrie, B., Akenhead, S., Lazier, J., and Loder, J. (1988). The cold intermediate layer on the Labrador and Northeast Newfoundland Shelves, 1978-86. NAFO Sci.Counc. Stud. 12, 57–69.
Phillips, R. A., Xavier, J. C., and Croxall, J. P. (2003). Effect of satellite transmitters on albatrosses and petrels. Auk 120, 1082–1090. doi: 10.1642/0004-8038(2003)120[1082:EOSTOA]2.0.CO;2
Planque, B., Hays, G. C., Ibanez, F., and Gamble, J. C. (1997). Large scale spatial variations in the seasonal abundance of Calanus finmarchicus. Deep Sea Res. 44, 315–326. doi: 10.1016/S0967-0637(96)00100-8
Regular, P. M., Hedd, A., and Montevecchi, W. A. (2011). Fishing in the dark: a pursuit-diving seabird modifies foraging behavior in response to nocturnal light levels. PLoS ONE 6:e26763. doi: 10.1371/journal.pone.0026763
Reid, E. P. C., and Valdés, L. (2011). ICES Status Report on Climate Change in the North Atlantic. ICES Cooperative Research Report No. 310. 262.
Richaud, B., Kwon, Y., Joyce, T. M., Fratantoni, P. S., and Lentz, S. J. (2016). Surface and bottom temperature and salinity climatology along the continental shelf off the Canadian and U.S. east coasts. Cont. Shelf Res. 124, 165–181. doi: 10.1016/j.csr.2016.06.005
Richman, S. E., and Lovvorn, J. R. (2011). Effects of air and water temperature on resting metabolism of auklets and other diving birds. Physiol. Biochem. Zool. 84, 316–332. doi: 10.1086/660008
Robertson, G. J., Fifield, D. A., Montevecchi, W. A., Gaston, A. J., Burke, C. M., Byrne, R., et al. (2012). Miniaturized data loggers and computer programming improve seabird risk and damage assessments for marine oil spills in Atlantic Canada. J. Ocean Technol. 7, 41–58.
Robertson, G. J., Wilhelm, S. I., and Taylor, P. A. (2003). Population Size and Trends of Seabirds Breeding on Gull and Great Islands, Witless Bay Islands Ecological Reserve, Newfoundland up to 2003. Can. Wildl. Ser. Tech. Rep. Ser. No. 418.
Rowe, S., Jones, I. J., Chardine, J. W., Elliot, R. D., and Veitch, B. G. (2000). Recent changes in the winter diet of murres Uria spp. in coastal Newfoundland waters. Can. J. Zool. 78, 495–500. doi: 10.1139/z99-221
Sorensen, M. C., Hipfner, J. M., Kyser, T. K., and Norris, D. R. (2009). Carry-over effects in a Pacific seabird: stable isotope evidence that pre-breeding diet quality influences reproductive success. J. Anim. Ecol. 78, 460–467. doi: 10.1111/j.1365-2656.2008.01492.x
Speakman, J. R., and Król, E. (2011). Limits to sustained energy intake. XIII. Recent progress and future perspectives. J. Exp. Biol. 214, 230–241. doi: 10.1242/jeb.048603
Templeman, N. (2010). Ecosystem Status and Trend Reports for the Newfoundland and Labrador Shelf. CSAS Res. Doc. 2010/026
Vandenabeele, S. P., Shepard, E. L., Grogan, A., and Wilson, R. P. (2012). When three per cent may not be three per cent: device-equipped seabirds experience variable flight constraints. Mar. Biol. 159, 1–14. doi: 10.1007/s00227-011-1784-6
Votier, S. C., Hatchwell, B. J., Beckerman, A., McCleery, R. H., Hunter, F. M., Pellatt, J., et al. (2005). Oil pollution and climate have wide-scale impacts on seabird demographics. Ecol. Lett. 8, 1157–1164. doi: 10.1111/j.1461-0248.2005.00818.x
Weiner, J. (1992). Physiological limits to sustainable energy budgets in birds and mammals: ecological implications. Trends Ecol. Evol. 7, 384–388. doi: 10.1016/0169-5347(92)90009-Z
Wharton, D. A. (2002). Life at the Limits: Organisms in Extreme Environments. Cambridge: Cambridge University Press.
Wilhelm, S. I., Mailhiot, J., Arany, J., Chardine, J. W., Robertson, G. J., and Ryan, P. C. (2015). Update and trends of three important seabird populations in the western North Atlantic using a geographic information system approach. Mar. Ornithol. 43, 211–222.
Wilson, R. P., Hustler, K., Ryan, P. G., Burger, A. E., and Noldeke, E. C. (1992). Diving birds in cold water: do Archimedes and Boyle determine energetic costs? Am. Nat. 140, 179–200. doi: 10.1086/285409
Winslade, P. (1974). Behavioral studies on the lesser sandeel Ammodytes marinus (Raitt) III. The effect of temperature on activity and the environmental control of the annual cycle of activity. J. Fish. Biol. 6, 587–599. doi: 10.1111/j.1095-8649.1974.tb05102.x
Winters, G. H. (1970). Biological changes in coastal capelin from over-wintering to the spawning condition. J. Fish. Res. Board Can. 27, 2215–2224. doi: 10.1139/f70-250
Winters, G. H. (1983). Analysis of the biological and demographic parameters of northern sand lance, Ammodytes dubius, from the Newfoundland Grand Bank. Can. J. Fish. Aquat. Sci. 40, 409–419. doi: 10.1139/f83-059
Wu, M., Xiao, Y., Yang, F., Zhou, L., and Zheng Liu, J. (2014). Seasonal variation in body mass and energy budget in Chinese Bulbuls Pycnonotus sinensis. Avian Res. 5:4. doi: 10.1186/s40657-014-0004-8
Ydenberg, R. C., and Clark, C. W. (1989). Areobiosis and anaerobiosis during diving by western grebes: an optimal foraging approach. J. Theor. Biol. 139, 437–447. doi: 10.1016/S0022-5193(89)80064-5
Ydenberg, R. C., Welham, C. V. J., Schmid-Hempel, P., Schmid-Hempel, R., and Beauchamp, G. (1994). Time and energy constraints and the relationships between currencies in foraging theory. Behav. Ecol. 5, 28–34. doi: 10.1093/beheco/5.1.28
Keywords: winter survival, North Atlantic, daily energy expenditure, diving seabird, flexible foraging behavior, common murre, bird-borne loggers
Citation: Burke CM and Montevecchi WA (2018) Taking the Bite Out of Winter: Common Murres (Uria aalge) Push Their Dive Limits to Surmount Energy Constraints. Front. Mar. Sci. 5:63. doi: 10.3389/fmars.2018.00063
Received: 05 September 2017; Accepted: 12 February 2018;
Published: 23 March 2018.
Edited by:
Filipe Rafael Ceia, University of Coimbra, PortugalReviewed by:
Martina Muller, University of Rhode Island, United StatesJan Marcin Weslawski, Institute of Oceanology (PAN), Poland
Maelle Connan, Nelson Mandela Metropolitan University, South Africa
Copyright © 2018 Burke and Montevecchi. This is an open-access article distributed under the terms of the Creative Commons Attribution License (CC BY). The use, distribution or reproduction in other forums is permitted, provided the original author(s) and the copyright owner are credited and that the original publication in this journal is cited, in accordance with accepted academic practice. No use, distribution or reproduction is permitted which does not comply with these terms.
*Correspondence: Chantelle M. Burke, Y2hhbnRlbGJAbXVuLmNh