- 1Davis-Bodega Marine Laboratory, University of California, Bodega Bay, Bodega Bay, CA, United States
- 2Department of Environmental Science and Policy, University of California, Davis, Davis, CA, United States
Planktonic larvae are thought to be very susceptible to offshore advection in upwelling regimes, increasing dispersal and decreasing recruitment. However, larvae of 42 species of nearshore benthic crustaceans primarily developed on the inner shelf at locations both in (98.5%) and away (99.8%) from a perennial upwelling center in the upwelling season of a recruitment-limited region characterized by persistent, strong, upwelling. During three cross-shelf cruises conducted at each location, larvae of 21 species remained on the inner shelf at both sites by occurring beneath seaward-flowing surface currents while larvae of other species migrated to midshelf (four species) or offshore (14 species) by initially developing near the surface. Postlarvae apparently returned to shore either deep in landward-flowing upwelled water or near the surface where behavior allows them to be transported shoreward by internal waves, diel wind cycles or wind relaxation events. Thus, recruitment limitation in upwelling regimes does not appear to be caused by larval mortality from offshore transport, requiring new research directions to advance our understanding of population dynamics, structure and connectivity.
Introduction
Variation in larval recruitment often determines population and community dynamics in the sea. Both larval dispersal and mortality have been challenging to measure rendering estimates of population connectivity uncertain. Moreover, the ability of larvae to regulate transport could have a large impact on dispersal and population connectivity and has been debated for decades. We recently determined that larvae occurred close to shore in persistent, strong upwelling on the Pacific Coast of the USA—rather than lost offshore as was widely expected (Morgan et al., 2009b,c, 2011; Morgan and Fisher, 2010; Fisher et al., 2014; Hameed et al., 2018)—and that larval mortality in the nearshore retention zone was much lower than previous estimates of larval mortality anywhere (White et al., 2014). We then demonstrated that larval behaviors enabling larvae to remain close to shore throughout development also enable them to settle near natal populations (Drake et al., 2013; Hameed et al., 2016). We now investigate whether larvae also completed larval development close to shore at one of the strongest upwelling centers further reducing the scale of population connectivity.
On the west coasts of continents, strong wind and Coriolis generate seaward-flowing surface currents (Ekman transport) dropping sea level along the coast while upwelling deep, cold water (Hickey, 1998). It has been suggested that larvae of nearshore species are lost to offshore transport by persistent, strong upwelling conditions (Parrish et al., 1981; Roughgarden et al., 1988; Menge and Menge, 2013), especially at the apex of headlands and immediately equatorward, where wind stress is most intense (Winant et al., 1988; Koracin et al., 2004) as well as at headlands where alongshore flow separates forming an offshore-directed jet (Barth et al., 2000; Kaplan and Largier, 2006). Meanwhile, flow may curve shoreward on the downstream of headlands resulting in onshore flow (Shannon et al., 1981; Halle and Largier, 2011) or forming a separation eddy where larvae may be accumulated (Wing et al., 1995, 1998; Graham and Largier, 1997; Roughan et al., 2005; Mace and Morgan, 2006a; Morgan et al., 2011; Ryan et al., 2014). Thus, larvae are widely expected to be most prone to offshore transport in upwelling centers and immediately downstream of headlands, limiting recruitment and enhancing dispersal away from this source, whereas farther downstream of headlands, they may be entrained in an eddy or transported onshore increasing recruitment and reducing dispersal away from this source.
Larval behavior may reduce transport at headlands and upwelling centers just as it does elsewhere along the coast. The characteristic vertical circulation in wind-driven upwelling areas enables invertebrate larvae and other zooplankters to reduce transport by regulating depth in vertically sheared currents (reviewed by Peterson, 1998; Morgan, 2014), as they do in other places where vertical shear occurs for different reasons (Epifanio and Garvine, 2001; Queiroga and Blanton, 2005; Morgan, 2006, in press; Epifanio and Cohen, 2016). Most species of larvae and copepods in upwelling regimes complete development close to shore within a coastal boundary layer, where Ekman transport is weak and alongshore currents are slow (Peterson et al., 1979; Morgan et al., 2009a; Shanks and Shearman, 2009; Morgan and Fisher, 2010; Nickols et al., 2013; Fisher et al., 2014; Hameed et al., 2018). They remain below a shallow Ekman layer or undertake diel vertical migrations, ascending near the surface to forage at night when offshore flow slows (Peterson et al., 1979; Peterson, 1998; Batchelder et al., 2002; dos Santos et al., 2008; Morgan et al., 2009a; Shanks and Shearman, 2009; Morgan and Fisher, 2010; Miller and Morgan, 2013). By avoiding upward transport into the seaward-flowing surface layer, they are subject to onshore flow and experience a flux convergence at the coast (Genin et al., 2005; Shanks and Brink, 2005). Different species are transported away from shore by occurring in the Ekman layer early in development and toward shore deep late in development, known as an ontogenetic vertical migration (OVM; Peterson et al., 1979; Grantham, 1997; Peterson, 1998; Mace and Morgan, 2006b; Papastephanou et al., 2006; Tapia and Pineda, 2007; Morgan et al., 2009a,b; Morgan and Fisher, 2010; Morgan, 2014). In some places or seasons, larvae late in development rise near the surface, known as a reverse ontogenetic vertical migration (ROVM), where behavior can allow internal waves, diel wind cycles or wind relaxations to transport them toward shore (Hobbs et al., 1991; Shanks, 1995a; Pineda, 1999; Poulin et al., 2002; Marta-Almeida et al., 2006; Morgan et al., 2009a; Morgan and Fisher, 2010).
We tested whether larval behavior effectively regulates transport at upwelling centers, as elsewhere, or whether it is overwhelmed by the intensity of wind forcing and currents. We compared cross-shelf and depth distributions of larvae of inshore benthic crustaceans along two transects near one of the strongest upwelling centers on the west coast of North America during the peak upwelling season. If larval behavior is overwhelmed, then (1) larvae should occur farther offshore at the upwelling center or (2) interspecific differences in larval behavior should render weak species more prone to offshore transport than others. If larval behavior effectively regulates larval transport, then cross-shore transport should be similar among species along the coast and to previous years. We also sampled beyond the shelf break to determine how many species are observed far offshore and potentially transported to the open ocean.
Materials and Methods
Study System
We conducted the study within the perennial upwelling center of Point Arena in northern California (Figure 1), which is the windiest region along the Pacific Coast of North America (Koracin et al., 2004; Dorman et al., 2005). Prevailing northwesterly winds last for weeks in the spring-summer upwelling season, and near-surface waters (<50 m) flow equatorward and seaward (Hickey, 1998; Roughan et al., 2006). Shallow depths and coastline topography slow flow <10 km from shore (Lentz and Chapman, 1989; Largier et al., 1993; Kaplan et al., 2005; Kirincich et al., 2005; Nickols et al., 2012). This coastal boundary layer widens south of Bodega Bay where flows impinge on the upstream shores of Point Reyes (Robart, 2013) as equatorward flow is deflected offshore, separating from the apex of Point Reyes (Kaplan and Largier, 2006; Vander Woude et al., 2006). Upwelled water and plankton are entrained in an upwelling shadow in the lee of Point Reyes until winds weaken and alongshore flow reverses, transporting larvae poleward and onshore in less than a day (Wing et al., 2003; Roughan et al., 2006; Vander Woude et al., 2006; Morgan et al., 2011, 2012). This occurs about every 4 to 10 days (Send et al., 1987; Largier et al., 1993). Reversing flows result in little net alongshore transport (Largier et al., 1993; Kaplan et al., 2005).
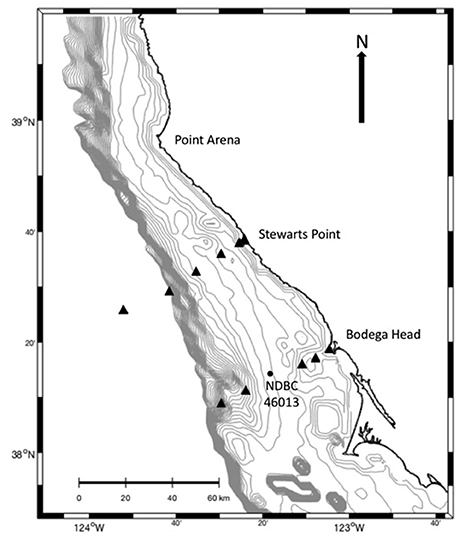
Figure 1. Map of the study area depicting locations of stations along cross-shelf transects off Bodega Head and Stewarts Point in northern California. Location of NDBC buoy 46013 (•) is also shown.
Approach
We sampled stations across the shelf along transects off Bodega Head and in the upwelling center off Stewarts Point (Figure 1) toward the end of prolonged upwelling events when larvae are expected to be farther from shore, if simply advected by near-surface currents. We targeted three distinct upwelling events to sample different larval pools. We planned cruises using marine weather forecasts and offshore conditions at National Data Buoy Center 46013 (38° 13′ 30″ N, 123° 19′ 00″ W; http://www.ndbc.noaa.gov; Figure 1). We considered upwelling conditions to be northwesterly wind >5 m/s and water <10°C or decreasing. We surveyed vertical distributions of larvae at six stations along two cross-shelf transects on consecutive days on 28 and 29 May, 24 and 25 June, and 14 and 15 July 2009. The Bodega Head transect extended farther from shore as the continental shelf is wider. Stations at Bodega Head were located 1, 6, 11, 32, 42, and 63 km from shore at ~20, 25, 80, 90, 100, and 120 m depth, respectively. Stations at Stewarts Point were located 1, 3, 10, 20, 30, and 49 km from shore at ~20, 25, 80, 90, 100 and 120 m depth, respectively.
We obtained wind velocity data from NDBC Buoy 46013 and calculated alongshore wind stress by converting it to true north and rotating it to 320° at an air density of 1.3 kg m−3 and a drag coefficient of 1.4 × 10−3 to place cruises in the context of upwelling conditions. We obtained water temperature and salinity from a Sea-Bird MicroCAT and chlorophyll-a fluoresence from a WET Labs EcoFluorometer, both at 4 m below the surface on moorings placed on the 15 m isobaths off Bodega Head and Stewarts Point from mid-May through July (Figure 1). In addition, temperature 1 m deep and 1 m above the seafloor was recorded with Onset Tidbit thermistors. We measured current using an acoustic Doppler current profiler (ADCP, 1,200 kHz; Teledyne RD Instruments, Poway, California, USA), which were bottom-mounted at moorings off Bodega Head and Stewarts Point at 30 m depth (Figure 1). We also mapped surface currents hourly across the study area using high-frequency (HF) radar.
We conducted three pairs of cruises along the two transects near the end of upwelling events, observed as periods of equatorward wind stress, cold water and low chl-a at the moorings off Bodega Head and Stewarts Point (Figure 2). Sampling on 28–29 May occurred at the onset of relaxation following a short upwelling event, with rising water temperature and chl-a and poleward flow (Figure 3). Sampling on 24–25 June occurred at the end of the strongest prolonged upwelling with temperatures still very low, even with the onset of relaxation on 25 June. Sampling on 14–15 July occurred at the end of a more moderate but prolonged upwelling event with winds and cold temperatures showing that relaxation had not yet started.
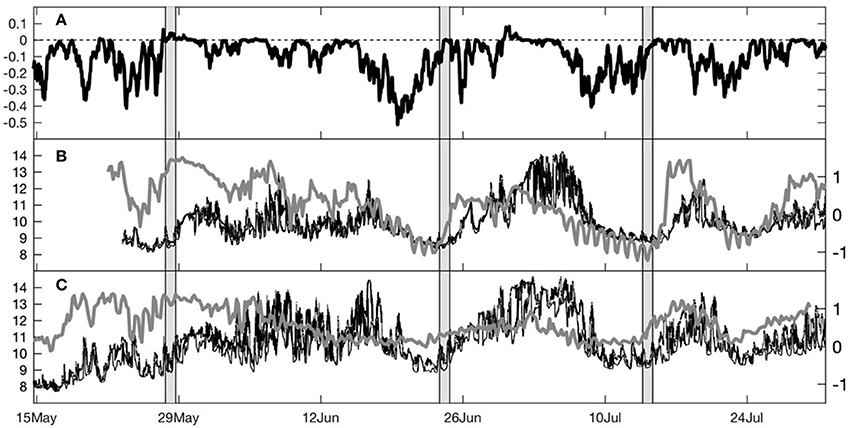
Figure 2. Time series data from 15 May through 26 July 2009: (A) alongshore wind stress at NDBC buoy 46013, (B) Bodega Head temperature and chlorophyll-a fluorescence and (C) Stewarts Point temperature and chlorophyll-a fluorescence. Surface temperature shown as black line and bottom temperature as thin gray line, with chlorophyll-a fluorescence as thick gray line. Survey timing is indicated by vertical gray bars.
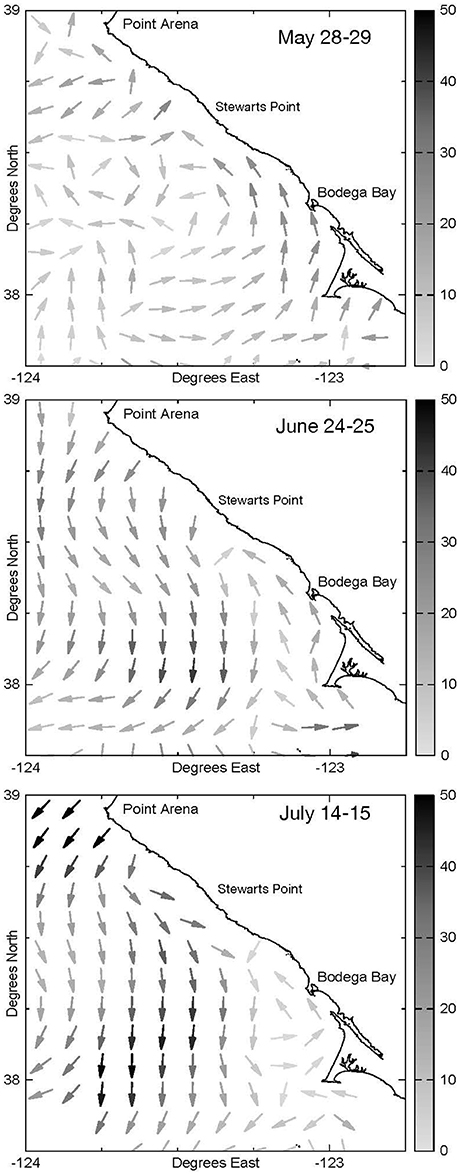
Figure 3. Two-day mean surface current velocity recorded by high-frequency radar across the study region during cruises conducted on 28–29 May, 24–25 June, and 14–15 July 2009. Arrow orientation shows direction while darkness of shading represents speed (cm/s).
We collected plankton in daylight using an electronically tripped Tucker Trawl (0.5 or 1 m2 mouth) with four nets (335-μm mesh) and temperature and depth sensors to take one sample above the thermocline and two samples below it. We sampled the surface of the water column using a neuston net (0.5 m2 mouth, 335-μm mesh). We profiled temperature and salinity with depth at the beginning and end of plankton tows using a conductivity, temperature and depth profiler (Sea-Bird 19-Plus).
We determined cross-shelf distributions for the 42 species of nearshore benthic crustaceans following our previous approach (Morgan et al., 2009b). Larval concentrations per m2 standardized for the depth of the water column. We determined the cross-shelf and depth distributions of the 39 most abundant species over the six cruises with a nonparametric analysis of similarity (ANOSIM) and a hierarchical cluster analysis and ordination. After averaging depths at each station for the cruises, we determined that cross-shelf distributions of larvae were similar among dates (Bodega: ANOSIM R = 0.054, p = 0.035; Stewarts: ANOSIM R = 0.045, p = 0.049), so we averaged dates before conducting analyses on cross-shelf and depth distributions. We tested the resulting dendrogram for differences among groups with a similarity profile test (SIMPROF) and the percent contribution of each species and stage to significant clusters with (SIMPER) to classify cross-shelf and depth distributions for each combination of species and stage. Nonmetric Multidimensional Scaling (nMDS) revealed the separation of larval assemblages by distance from shore and depth. Contours of cross-shore and depth distributions of species by stage were plotted to evaluate whether depth regulation maintained different cross-shelf distributions. We combined species with similar depth distributions during development to simplify presentation and reported the mean depth center of mass (ZCM) and standard error for each species or group.
Results
CTD profiles of temperature and chl-a revealed water column structure across the shelf along the two transects (Figure 4). On 28 and 29 May, a warm surface layer was observed, strongest nearshore and consistent with poleward flow–this layer was also characterized by high chl-a concentrations. On June 25 and 24 following a strong, prolonged upwelling event, cold waters shoaled nearshore with weak stratification off Stewarts Point on 24 June, but evidence of poleward flow and an incipient warm surface layer inshore occurred off Bodega Head on 25 June. Chl-a was generally low across the shelf, representative of newly upwelled waters, although a subsurface chlorophyll maximum was observed well offshore of Stewarts Point, beneath a warmer layer of oceanic water. Again on 14 July, active upwelling conditions were observed off Stewarts Point, with cold water shoaling to the surface nearshore and very low chl-a concentrations. At Bodega Head, farther from the upwelling center and a day later, some nearshore stratification and significant chl-a concentration was observed as well as weak surface currents (Figure 3).
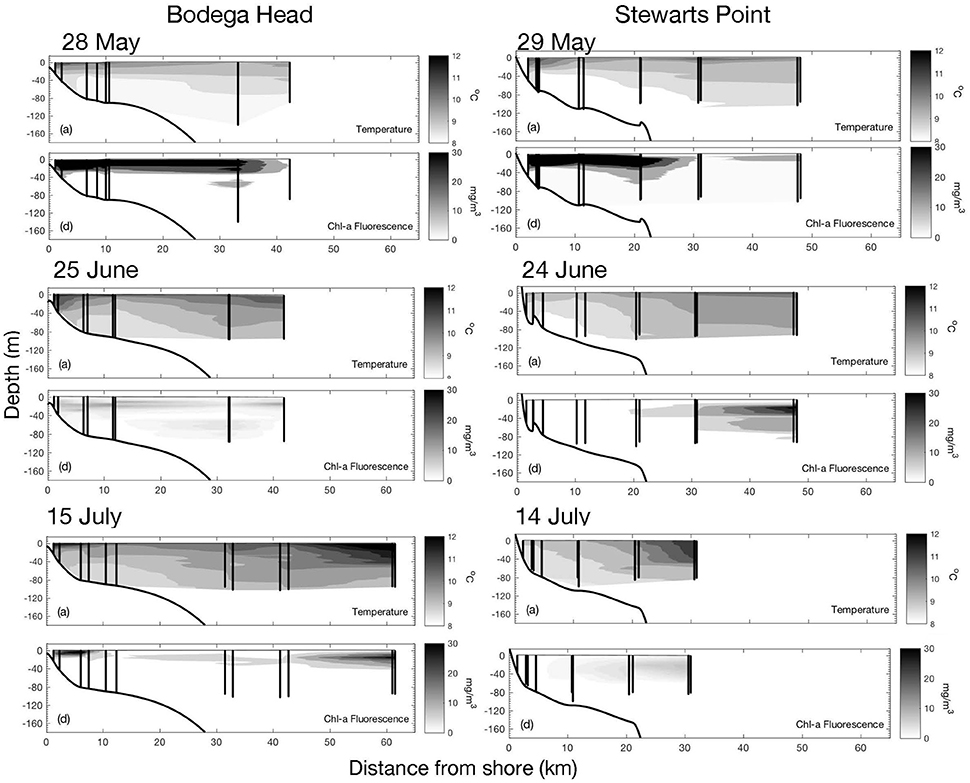
Figure 4. Contours of temperature (°C) and chlorophyll-a from vertical profiles at six stations along transects off Bodega Head and Stewarts Point on 28–29 May, 24–25 June, and 14–15 July 2009. Profile locations at the beginning and end of plankton tows at each station are depicted by black vertical lines.
Surface currents measured by HF radar showed that during the first pair of cruises on 28–29 May flow was poleward nearshore at Bodega Head and Stewarts Point; while offshore flow was observed at Point Arena, onshore flow of surface waters was observed south of Bodega Head (Figure 3). On June 24–25, nearshore flow was poleward from Bodega Head to Stewarts Point and equatorward from Point Arena to Stewarts Point where these currents converged, deflecting alongshore flow offshore. On July 14–15, nearshore flows were very weak in the vicinity of Bodega Head, but equatorward and offshore flow was well developed from Point Arena to south of Stewarts Point.
The progressive vector diagrams showed net poleward transport near-bottom and near-surface at both nearshore sites (Figure 5). Transport was shoreward near-surface and seaward near-bottom off Bodega Head, whereas it was seaward near-surface and near-zero near-bottom off Stewarts Point. At both sites, near-surface transport exhibited greatest excursions during upwelling and relaxation events, indicating that larvae remaining deep in the water column would travel the shortest distance.
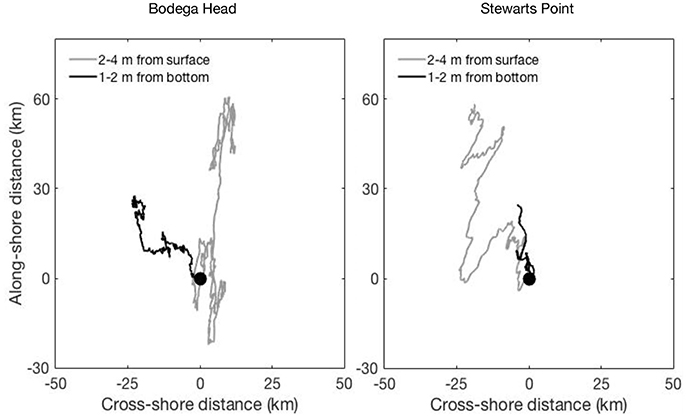
Figure 5. Progressive vector diagrams of potential alongshore and cross-shore displacement due to currents measured near-surface (gray line) and near-bottom (black line) from mid 24 May through 26 July 2009 at Bodega Head and Stewarts Point. Current velocity was measured by a bottom-moored ADCP at 30-m depth at each site. Positive alongshore distances are poleward and positive cross-shore distances are shoreward.
Larvae of 42 species of benthic crustaceans generally did not occur farther offshore at the upwelling center at Stewarts Point than Bodega Head (Figure 6). Larvae largely occurred; l98.7989 < 11 km from shore at both sites, and early larval stages were abundant inshore where larvae are released. All stages of 22 species occurred on the inner shelf <11 km from shore off Bodega Head (99.8%) with the large majority of them occurring just 1 km from shore and most of the rest of them occurring 6 km from shore (Figure 6), revealing larval retention. Similarly off Stewarts Point, all stages of these species occurred on the inner shelf <10 km from shore (98.5%; Figure 6) with most of the rest of them occurring 3 km from shore. All stages of four more species occurred <11 km from shore off Bodega Head (96.1%) with the large majority of them occurring 1 km from shore and most of the rest of them occurring 6 km from shore while late stages occurred <32 km from shore (Figure 6). Similarly off Stewarts Point, all stages of these species occurred <10 km from shore (95.4%) with the large majority of them occurring just 1 km from shore and most of the rest of them occurring 3 km from shore while late stages occurred <30 km from shore (Figure 6). Early stages of 16 more species occurred <11 km from shore (81.8%) and late stages occurred far offshore in much lower abundance off Bodega Head (18.2 %, Figure 6). Similarly off Stewarts Point, early stages of these species occurred <10 km from shore (70.6%) and late stages occurred far offshore in low abundance (Figure 6). Thus, along both transects, larvae of most species were retained on the inner shelf while late stages other species ranged to the midshelf or offshore.
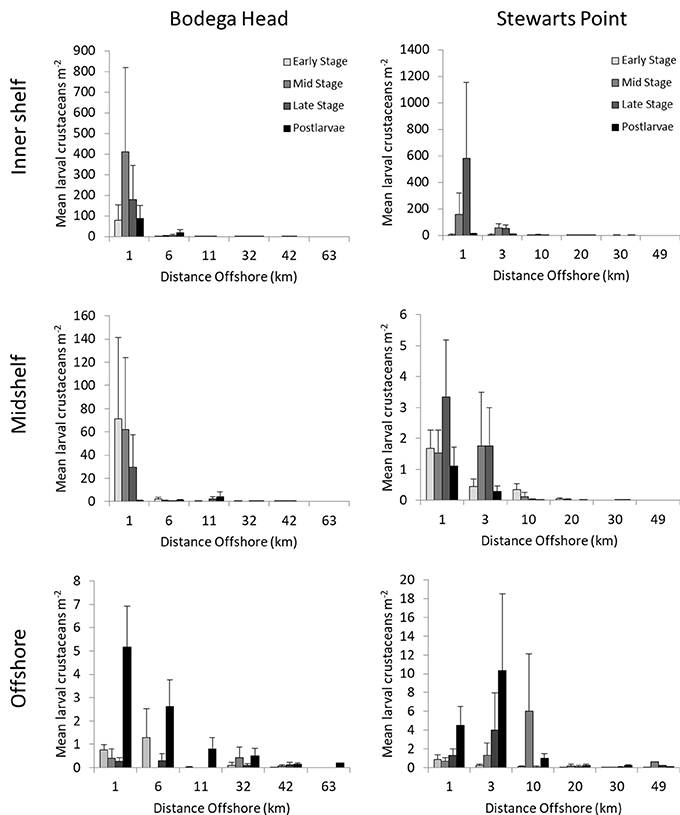
Figure 6. Densities (mean/m2 + 1 SE) of larval stages of 42 species of benthic crustaceans relative to distance from shore for all depths and cruises combined at Bodega Head and Stewarts Point on 28–29 May, 24–25 June, and 14–15 July. Twenty-two species largely remained on the inner shelf off Bodega Head (<11 km from shore) and Stewarts Point (<10 km from shore) throughout development. Four species primarily occurred on the inner shelf and ranged across the midshelf late in development off Bodega Head (<32 km from shore) and Stewarts Point (<30 km from shore). Sixteen species primarily occurred on the inner shelf and ranged offshore late in development off Bodega Head (>32 km) and Stewarts Point (>30 km). Note scale changes.
The 39 most abundant species differed primarily across stations (Bodega Head: ANOSIM R = 0.439, p = 0.001; Stewarts Point: ANOSIM R = 0.373, p = 0.002) but also among depths (Bodega Head: ANOSIM R = 0.107, p = 0.096; Stewarts Point: ANOSIM R = 0.145, p = 0.055), indicating that depth regulation contributed to interspecific differences in cross-shelf transport at both sites. Cluster analysis and nMDS showed pronounced spatial structure in larval assemblages along both transects grouping taxa into inner, mid and offshore categories for both transects with an outlier (neuston at Station 3) for the offshore group at Stewarts Point (Figure 7).
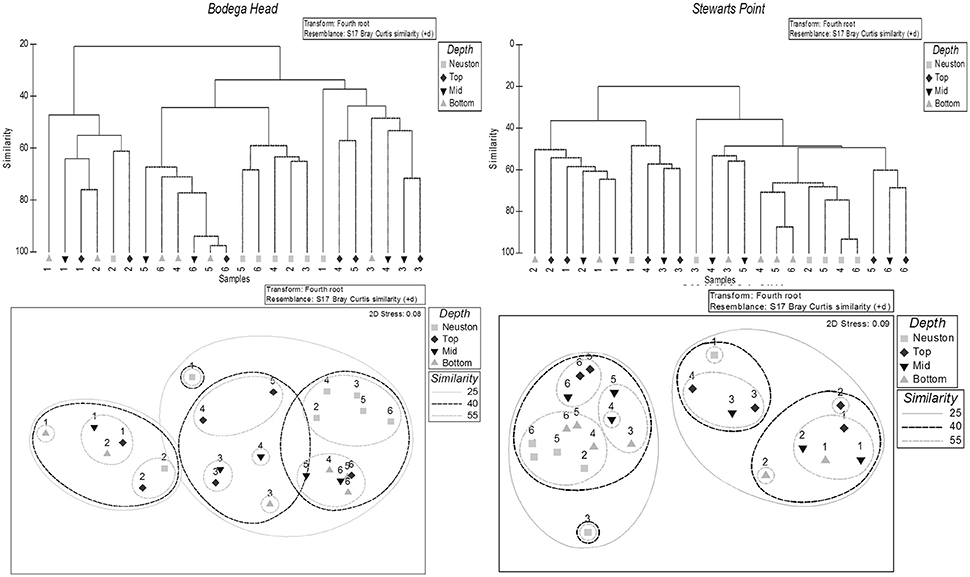
Figure 7. Top panels. Hierarchical clustering dendrogram (using group-average linking) of 48 samples taken from four depths at six sampling stations across the continental shelf: 1, 6, 11, 32, and 63 km from shore off Bodega Head and 1, 3, 10, 20, 30, and 49 km from shore off Stewarts Point. Samples were categorized based on 25 and 40% similarities. Solid black lines indicate significant group structure at the 1% level. Sample distances offshore are reported beneath each group. Bottom panels: Nonmetric multidimensional scaling plots (2D stress: 0.08 for Bodega Head and 0.09 for Stewarts Point) from the 48 samples with superimposed significant clusters at similarity levels of 25% (solid lines) and 40% (dashed lines) off Bodega Head and Stewarts Point.
A nearshore cluster of all stages of 21 species largely occurred <6 km from shore (Figures 7, 8), five species of porcelain crabs (Petrolisthes cinctipes, Pet. eriomerus, Pet. manimaculus, Pachycheles rudis, Pac. pubescens), a mud shrimp (Neotrypaea californiensis), five species of barnacles (Balanus crenatus B. glandula, Semibalanus cariosus, Chthamalus dalli/fissus), three hermit crabs (Pagurus samuelis, P. hirsutiusculus, P. granosimanus) and seven pea crabs (Pinnixa faba, P. tubicola, Pinnotheres pugettensis, Scleroplax granulata, Fabia subquadrata, two unidentified species). However, postlarvae of the three barnacles, three hermit crabs and seven pinnotherids occurred in very low concentrations to ~18 km from shore during the cruise off Bodega Head on 28 May (Figure 8). Pinnotherid postlarvae occurred in low concentrations <30 km off Bodega Head and <20 km off Stewarts Point, because one of the species (F. subquadrata) disperses midshelf (Morgan et al., 2009c). Off Bodega Head, all stages of these species composed 12.9% of all larvae along the transect contributing 11.26% of the similarity to the cluster, and off Stewarts Point, all stages of these species composed 2.4% of all larvae collected along the transect and contributed 3.11% of the similarity to the cluster (Figure 7). Larvae of these species occurred below the shallow Ekman layer (Figure 8). Porcelain crabs undertook a ROVM (Figure 8) with similar ZCMs of larvae at the shallow stations inshore off Bodega Head (11.9 ± 3.0 m) and Stewarts Point (9.7 ± 4.6 m) and postlarvae ascending to the neuston (0.5 m) at both sites. In contrast, pinnotherid crabs undertook an OVM (Figure 8) with similar ZCMs of larvae inshore off Bodega Head (17.2 ± 3.2 m) and Stewarts Point (16.5 ± 3.5 m) and postlarvae descending much deeper in the water column (Bodega 49.0 m; Stewarts 37.4 ± 2.3 m). The rest of these species mostly occurred below the Ekman layer throughout development while perhaps undertaking an OVM with postlarvae occurring deeper than larvae on one or more cruises along both transects (Figure 8). Neotrypaea californiensis larvae occurred higher in the water column (14.5 ± 5.7 m) than postlarvae (24.0 ± 0.7 m) off Stewarts Point, though not off Bodega Head where both larvae (32.4 ± 15.0 m) and postlarvae (26.7 ± 8.9 m) occurred deeper than off Stewarts Point. Late-stage barnacle larvae were shallower (5.1 ± 2.3 m) than postlarvae (12.9 ± 1.0 m) off Bodega Head, though postlarvae were deeper on only one cruise off Stewarts Point. Similarly, hermit crab larvae were shallower (17.7 ± 0.6 m) than postlarvae off Bodega Head (46.8 ± 24.4 m), though postlarvae were deeper on only one cruise off Stewarts Point
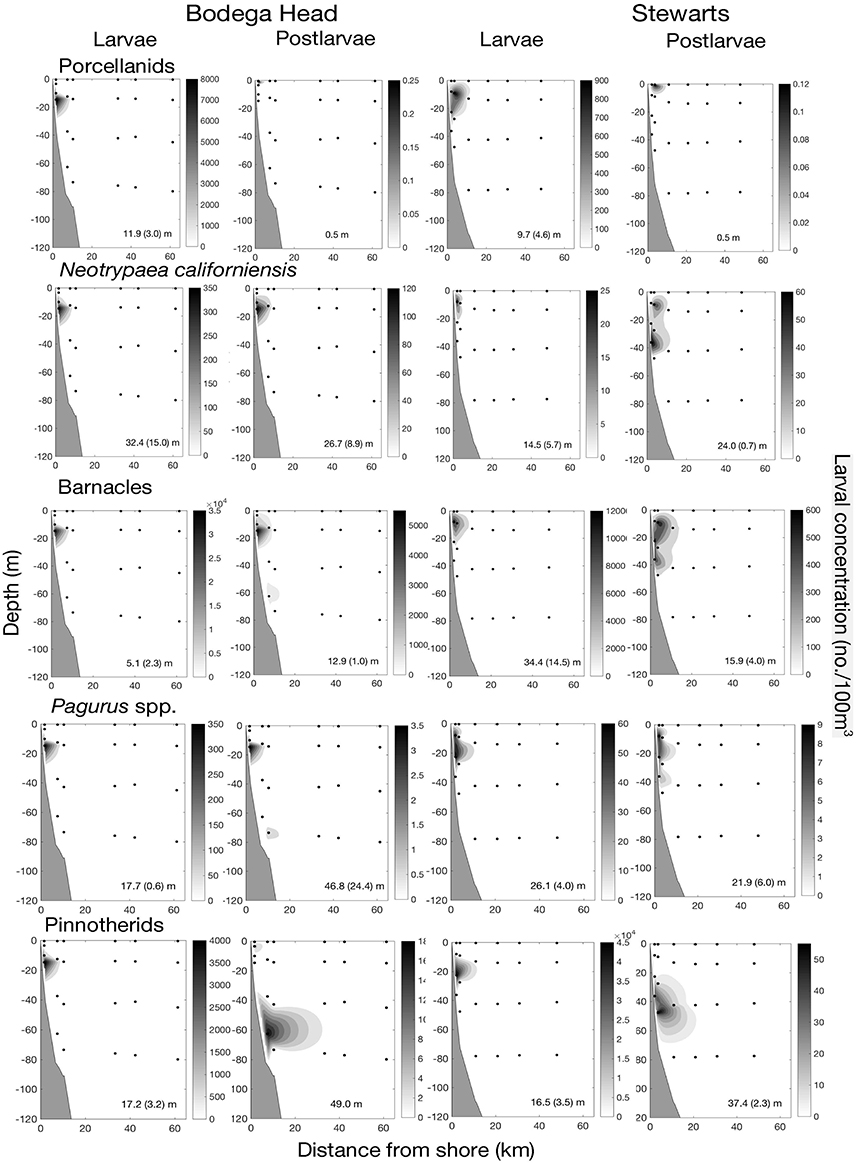
Figure 8. Inner-shelf larval development of 21 species in relation to distance from shore and depth off Bodega Head and Stewarts Point for larvae that largely occurred <6 km. First row of panels for five species of porcelain crabs (Petrolisthes cinctipes, Pet. eriomerus, Pet. manimaculus, Pachycheles rudis, Pac. pubescens); second row of panels for a mud shrimp (Neotrypaea californiensis); third row of panels for five species of barnacles (Balanus crenatus B. glandula, Semibalanus cariosus, Chthamalus dalli/fissus); fourth row of panels for three hermit crabs (Pagurus samuelis, P. hirsutiusculus, P. granosimanus); and fifth row of panels for seven pea crabs (Pinnixa faba, P. tubicola, Pinnotheres pugettensis, Scleroplax granulata, Fabia subquadrata, two unidentified species). The depth center of mass (ZCM; mean with SE in parentheses) is given at the bottom of each panel. ZCM with no variation shown in parentheses indicates species were collected during a single cruise, and separate groups of larvae within panels were collected during different cruises. Missing panel indicates that small larvae passed through nets.
A midshelf cluster of all stages of four species largely occurred <6 km from shore with late stages occurring <32 km at both sites, including the xanthid crab Lophopanopeus bellus, two grapsid crabs (Hemigrapsus oregonensis, H. nudus) and Romaleon (Cancer) antennarius (Figures 7, 9). Off Bodega Head, all stages of these species composed 85.1% of all larvae along the transect contributing 38.10% of the similarity to the cluster. Off Stewarts Point, all stages of these species composed 93.7% of all larvae collected along the transect and contributed 31.16% of the similarity to the cluster (Figure 7). The vertical distributions of L. bellus and the grapsid crabs were similar throughout development (Figure 9). ZCMs of L. bellus off Bodega Head were 14.0 ± 2.4 m for early larvae and 16.0 m for postlarvae (late stages were not collected), and ZCMs off Stewarts Point were 23.8 ± 5.5 m for early larvae, 27.9 m for late larvae and for 27.9 m postlarvae. Off Bodega Head, ZCMs of grapsid larvae were 13.1 ± 3.1 m for early stages, 14.6 ± 2.6 m for late stages and 12.6 m for postlarvae, and off Stewarts Point, ZCMs were 11.1 ± 1.0 m for early stages, 10.8 ± 1.8 m for late stages and postlarvae were not collected (Figure 9). Vertical distributions also were similar throughout development for R. antennarius off Bodega Head (early 23.3 ± 10.9 m, late 19.0 ± 3.0 m, postlarvae 17.8 ± 0.8 m), but there was evidence of a possible OVM off Stewarts Point with larvae occurring shallower (early 14.3 ± 5.5 m, late 15.2 ± 6.8 m) than postlarvae on two cruises (31.8 ± 6.8 m).
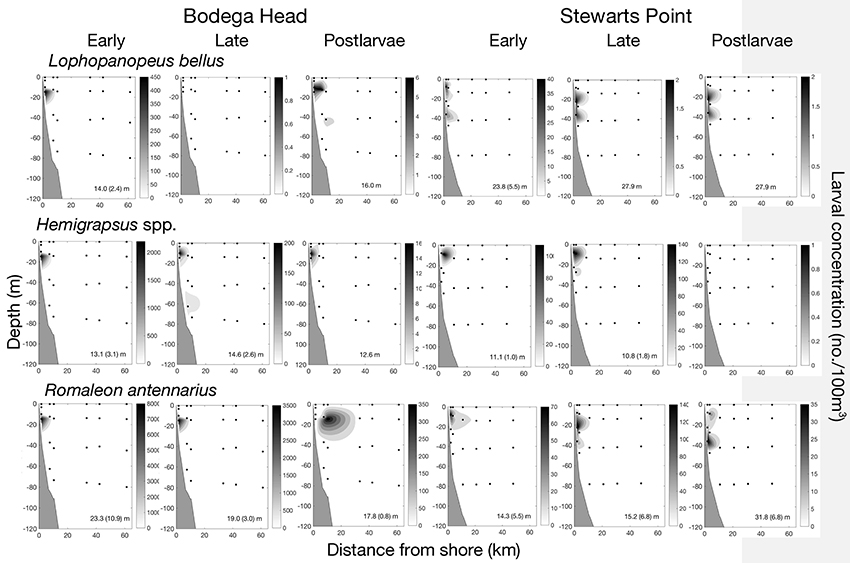
Figure 9. Midshelf larval development of four species in relation to distance from shore and depth off Bodega Head and Stewarts Point where larvae largely occurred <11 km from shore with late stages primarily occurring <32 km. First row of panels for the xanthid crab Lophopanopeus bellus; second row of panels for two grapsid crabs (Hemigrapsus oregonensis, H. nudus); and third row panels for a rock crab Romaleon (Cancer) antennarius. The depth center of mass (ZCM; mean with SE in parentheses) is given at the bottom of each panel. ZCM with no variation shown in parentheses indicates species were collected during a single cruise, and separate groups of larvae within panels were collected during different cruises. Missing panel indicates that small larvae passed through nets.
An offshore cluster composed of 14 species with early larval stages largely occurring <11 km from shore and late stages occurring >30 km from shore (Figures 7, 10). Off Bodega Head, all stages of these species composed 1.9% of all larvae collected along the transect and contributed 49.36% of the similarity to the cluster, and off Stewarts Point, all stages of these species composed 3.4% of all larvae along the transect contributing 65.73% of the similarity to the cluster (Figure 7). Larvae of seven majiids (Pugettia richii, P. producta, P. gracilis, Scyra acutifrons, Mimulus foliatus, Oregonia gracilis, unidentified species) primarily completed development <1l km from shore, whereas larvae of three species of cancrid crabs [Metacarcinus (Cancer) magister, Cancer productus, Glebocarcinus (Cancer) oregonensis], two barnacles (Balanus nubilus, Pollicipes polymerus), Emerita analoga and an unidentified porcellanid occurred in low concentrations beyond 11 km (Figures 7, 10). Larvae of these species commonly occurred in the Ekman layer midshelf and offshore (Figure 10). Cancrid postlarvae even were prevalent in the neuston at Station 3 off Stewarts Point (Figure 10), being reflected as an outlier for the offshore group off Stewarts Point in the dendrogram (Figure 7). The barnacles appeared to undertake an OVM (Figure 10); ZCMs were shallower for late-stage barnacle larvae (Bodega 15.3 ± 0.1 m; Stewarts 15.6 ± 2.8 m) than postlarvae (Bodega 30.5 ± 3.6 m; Stewarts 27.2 ± 4.5 m). Majiids may have undertaken an OVM (Figure 10). Off Bodega Head, ZCMs of majiid larvae were shallower (early 11.7 ± 1.6 m, late 11.1 ± 1.1) than postlarvae (21.9 ± 15.8 m), and off Stewarts Point, early-stage larvae also were shallower (15.8 ± 5.3 m) than late-stage larvae (20.6 ± 13.4) and postlarvae (22.1 ± 2.3 m). Cancrids may have undertaken a ROVM (Figure 10). Cancrid larvae off Bodega Head were deeper (early 12.0 ± 0.4 m) than postlarvae (0.5 m), and this also was the case off Stewarts Point (early 22.2 ± 10.9 m, late 13.8 ± 1.0 m), except during one cruise when postlarvae occurred in low concentrations ~45 m deep resulting in a ZCM of 20.2 ± 13.1 m. Emerita analoga larvae commonly occurred in the surface layer across the shelf (Figure 10) with ZCMs off Bodega Head of 6.7 ± 2.2 m for early larvae and 12.5 m for late larvae and ZCMs off Stewarts Point of 15.0 m for late larvae. Unidentified porcellanid larvae also occurred in the surface layer across the shelf off Stewarts Point with ZCMs of 0.5 m for early larvae and 10.0 m for late larvae, but early stages were deep in the water column during the one cruise that they were collected off Bodega Head with a ZCM of 59.4 m (Figure 10). We did not detect ontogenetic changes in vertical distributions of E. analoga or the unidentified porcellanid because we did not collect postlarvae.
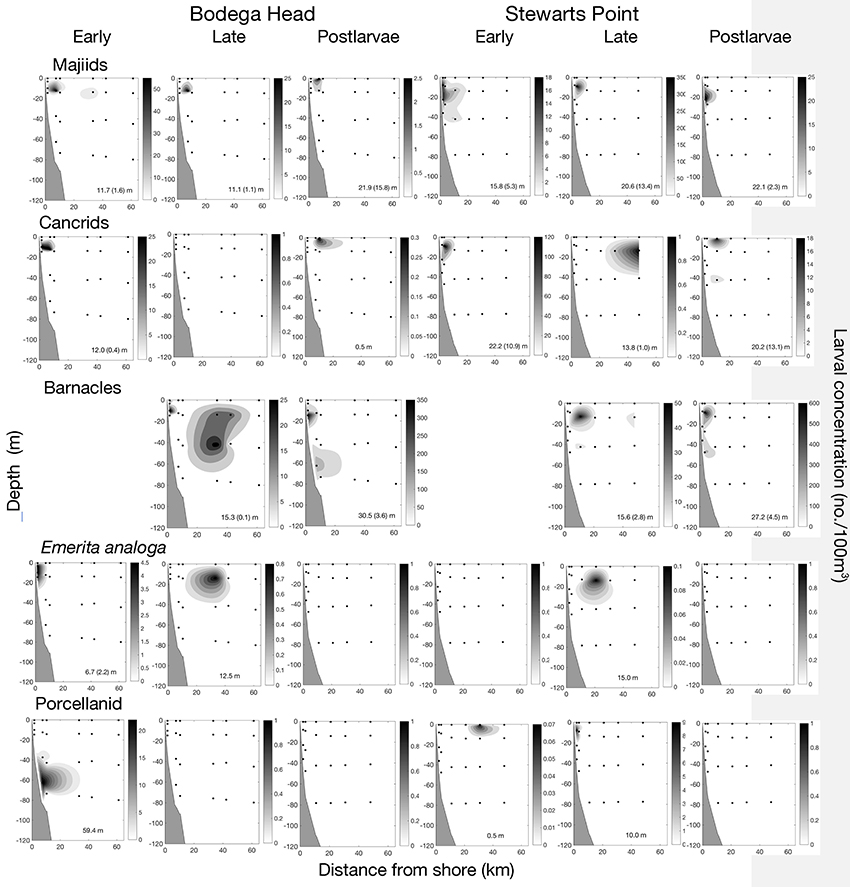
Figure 10. Offshore larval development of 14 species in relation to distance from shore and depth off Bodega Head and Stewarts Point where larvae largely occurred <11 km from shore with late stages occurring >32 km from shore. First row of panels for seven majid crabs (Pugettia richii, Pugettia producta, Pugettia gracilis, Scyra acutifrons, Mimulus foliatus, Oregonia gracilis, unidentified species); second row of panels for three cancrid crabs (C. magister, C. productus, C. oregonensis); third row of panels for two barnacles (Balanus nubilus, Pollipes polymerus); fourth row of panels for the mole crab (Emerita analoga); and fifth row of panels for an unidentified porellanid. The depth center of mass (ZCM; mean with SE in parentheses) is given at the bottom of each panel. ZCM with no variation shown in parentheses indicates species were collected during a single cruise, and separate groups of larvae within panels were collected during different cruises. Missing panel indicates that small larvae passed through nets.
Discussion
We have shown that larvae of nearshore benthic crustaceans are retained nearshore or reliably migrate different distances from shore during the season of peak upwelling and in the core of an upwelling center within the region of the most persistent, strongest upwelling along the Pacific Coast of North America. In this work and previous studies, we have repeatedly sampled larval assemblages during many cruises over multiple locations and years (Morgan et al., 2009b,c; Morgan and Fisher, 2010; Hameed et al., 2018). In this work, we focused on the time and place of maximum upwelling, showing that larvae were not transported farther offshore at this region of strong, persistent upwelling, contrary to widespread expectations. During the present study, we sampled larvae of 42 nearshore benthic crustaceans and 99; l98.7989% of all larvae were collected over the inner shelf, <11 km from shore along both transects. Larvae were most concentrated within a few kilometers of the shoreline, in the coastal boundary layer where offshore Ekman transport and alongshore currents are weak (Lentz and Chapman, 1989; Largier et al., 1993; Kaplan et al., 2005; Kirincich et al., 2005; Roughan et al., 2006; Morgan et al., 2009c; Morgan and Fisher, 2010; Nickols et al., 2012, 2013; Hameed et al., 2018). Not only was this characteristic coastal-boundary-layer circulation (Pettigrew and Murray, 1986; Shanks, 1995a; Largier, 2002, 2003) persistent, but it is expected to contribute to nearshore larval retention of diverse benthic crustaceans in other upwelling regions worldwide (Morgan, 2014).
All larval stages of 21 species occurred in high concentrations primarily in the coastal boundary layer <6 km offshore, including the five species of porcelain crabs, mud shrimp, five species of barnacles, three hermit crabs and seven pea crabs. The three species of barnacles, three species of hermit crabs and six of the seven species of pinnotherids in this nearshore group also completed development in high concentrations <6 km from shore off Bodega Head in our previous studies (Morgan et al., 2009b,c; Morgan and Fisher, 2010; Nickols et al., 2013; Hameed et al., 2018). In our present study, these barnacles and hermit crabs completed development close to shore in five of the six cruises, but in the 28 May cruise off Bodega Head their postlarvae were found in very low concentrations up to ~18 km from shore. This survey was conducted during a relaxation event when a poleward flow from the Gulf of Farallones can transport plankton offshore north of Point Reyes, unlike during prevailing upwelling conditions (Kaplan and Largier, 2006; Morgan et al., 2012). In addition, one of the seven pinnotherids (F. subquadrata) developed farther from shore than the other six species in our previous study off Bodega Head, but we did not distinguish it from the other pinnotherids during the present study.
Of the species that did not complete their larval life nearshore, many occurred in high concentrations <11 km early in development and in lower concentrations farther from shore late in development. The four species that occurred midshelf (L. bellus, Hemigrapsus spp., R. antennarius) or the 14 species that occurred offshore (seven majiids, three cancrids, two barnacles, mole crab, unidentified porcellanid) late in development had similar distributions in our previous studies (Morgan et al., 2009b,c; Morgan and Fisher, 2010; Nickols et al., 2013; Hameed et al., 2018). Postlarvae of all of these species spanned the continental shelf while recruiting onshore. Crustacean larvae had similar cross-shelf distributions off Oregon (Lough, 1974; Fisher et al., 2014), indicating that these interspecific differences are maintained in time and space.
Distinctive vertical distributions of larval stages were related to horizontal distributions likely contributing to maintaining the three cross-shelf patterns. Larvae in the nearshore group occurred deep in a shallow water column early in development and most species either descended (N. californiensis, B. crenatus, B. glandula, Pagurus spp., pinnotherids) or ascended (porcellanids) late in development apparently undertaking an OVM or ROVM, respectively. Similar ontogenetic changes in vertical distributions were evident in our previous study off Bodega Head, and in addition, Chthamalus spp. underwent a ROVM (Morgan et al., 2009c). The subsurface vertical distributions of all these larvae and postlarvae undertaking an OVM (in this and prior studies) placed them in onshore and weak alongshore flow (Largier et al., 1993; Dever et al., 2006; Roughan et al., 2006; Nickols et al., 2012), likely facilitating retention nearshore (Peterson, 1998; Batchelder et al., 2002; Papastephanou et al., 2006; Morgan et al., 2009c; Morgan and Fisher, 2010; Nickols et al., 2013; Hameed et al., 2018). Barnacle and pinnotherid larvae typically stay deep, whereas N. californiensis and Pagurus spp. larvae rise near the surface at night (Morgan and Fisher, 2010) after winds subside and are often onshore reducing transport (Peterson et al., 1979; Hobbs et al., 1991; Peterson, 1998; Batchelder et al., 2002; Poulin et al., 2002; Marta-Almeida et al., 2006; dos Santos et al., 2008; Bonicelli et al., 2016). By undertaking a ROVM, postlarvae of the porcellanids and Chthamalus spp. could be transported shoreward by winds and internal tides in the neuston (Pineda, 1994, 1999; Shanks, 1995a), which occur in our region during relaxation periods in a stratified water column (Rosenfeld, 1990).
Early stages of the rest of the species were transported farther from shore by spending more time in surface currents (Morgan et al., 2009c; Morgan and Fisher, 2010), as do copepods (Peterson et al., 1979; Peterson, 1998; Batchelder et al., 2002; Papastephanou et al., 2006). Ten species (R. antennarius, two barnacles, seven majiids), generally descended into deeper onshore flow late in development during our present or previous study (Morgan et al., 2009c). Onshore transport of late stages limits cross-shelf displacement and returns postlarvae to shore (Peterson et al., 1979; Grantham, 1997; Peterson, 1998; Papastephanou et al., 2006; Morgan et al., 2009c). The three species of cancrids transported offshore returned to shore as postlarvae by ascending to the neuston. Ontogenetic vertical migrations either were not apparent (L. bellus) or indeterminate without all larval stages being collected (E. analoga, unidentified porcellanid) in this or our previous study (Morgan et al., 2009c). Even without ontogenetic changes in vertical distributions, crabs in this region generally rise near the surface at night (Morgan and Fisher, 2010) facilitating onshore transport by internal tides and winds (Jamieson and Phillips, 1988; Shenker, 1988; Hobbs et al., 1991; Shanks, 1995b; Rasmuson and Shanks, 2013).
As we discussed previously (Morgan et al., 2009c; Fisher et al., 2014; Morgan, 2014), passive advection and eddy diffusion alone cannot explain the differences in cross-shelf distributions among so many species, because (1) these larvae are released inshore and encounter the same physical processes, (2) distances larvae migrate from shore are not related to larval durations, (3) larval densities were adjusted to account for increasing depth across the shelf to ensure that high larval concentrations after hatching were not simply reduced by dilution due to vertical mixing as the water column deepened offshore and (4) late stages were as abundant or increased midshelf and offshore instead of decreasing. Thus, behavior is common across larval taxa and many species exhibit behaviors that reduce offshore transport in upwelling regions elsewhere on the Pacific Coast, including in the weaker upwelling off Oregon (Peterson et al., 1979; Shanks and Shearman, 2009; Fisher et al., 2014) and central through southern California (Grantham, 1997; Tapia and Pineda, 2007), as well as worldwide, including Chile (Poulin et al., 2002; Bonicelli et al., 2016) and the Iberian Peninsula (Marta-Almeida et al., 2006; dos Santos et al., 2008; Bartilotti et al., 2014).
If larvae remain close to shore, why are populations recruitment limited in persistent, strong upwelling regions? Presumably, there is a constraint between the larval availability in the plankton over the inner shelf and the settlement of benthic larvae in shoreline habitats. Recent work has shown that the ability of postlarvae to enter the surf zone largely depends on temporal and spatial variation in surfzone hydrodynamics, which is determined by breaking waves interacting with alongshore changes in coastal morphology, yielding a spectrum of surf zones from dissipative to reflective (Wright and Short, 1984). Broad surf zones along mildly sloping shorelines usually have far more larvae and other plankton and much greater settlement onshore (Shanks et al., 2010, 2017, 2018, in press; Morgan et al., 2016, 2017, 2018). These dissipative surfzones have bathymetric rip currents, which concentrate plankton by recirculation in a series of rip currents alongshore, unlike narrow reflective surf zones at steep shores (Shanks et al., 2010, 2017, 2018, in press; Morgan et al., 2016, 2017, 2018). Individual based biophysical models revealed that Stokes drift and benthic boundary layer streaming coupled with downward swimming in response to turbulence from waves facilitated onshore larval delivery beaches (Fujimura et al., 2013, 2014, 2017, 2018). The striking differences in surfzone oceanography at dissipative and reflective shores predictably affect larval recruitment and productivity of inshore communities (Morgan et al., 2016, 2017, 2018; Shanks et al., 2017, in press) and appear to explain recruitment limitation at many sites in California (Morgan et al., 2016, 2017; Shanks et al., 2017; Shanks and Morgan, 2018). Together with the above results on larval distributions in upwelling areas, this suggests a shift in paradigms from the past idea that upwelling necessarily limits recruitment to new ideas that recruitment limitation and population connectivity may be better explained by alongshore differences in surfzone circulation (Shanks et al., 2017; Shanks and Morgan, 2018; Morgan, in press).
Larval retention evidently occurs all along coasts in upwelling regions, rather than primarily occurring in bays and eddies in the lee of headlands. This implies that alongshore transport and the scale of connectivity in benthic metapopulations in upwelling areas is less than previously suggested. Larval transport would be reduced even further if postlarvae effectively navigate onshore in upwelling regimes. Elsewhere, postlarvae can navigate onshore to suitable settlement sites using hierarchies of cues that are effective over different spatial scales, including celestial bodies, polarized light, magnetic and electric fields, waves and tides, and acoustic, chemical and visual cues (Kingsford et al., 2002; Montgomery et al., 2006; Arvedlund and Kavanagh, 2009; Morgan, in press). Adding onshore navigation into individual based biophysical models in upwelling regions (Pfeiffer-Herbert et al., 2007; Carr et al., 2008; Petersen et al., 2010; Domingues et al., 2012; Drake et al., 2013; Nolasco et al., 2013) should reduce the scale of population connectivity even further, but onshore navigation remains to be documented for species in upwelling regimes (Morgan, in press). The ultimate goal is to determine realized population connectivity, reconciling evidence from studies of circulation, plankton, genetics and microchemistry. Next steps should also include incorporating documented reproductive outputs of a metapopulation (Hameed et al., 2016), larval behaviors documented here and previously (Morgan et al., 2009b; Morgan and Fisher, 2010) and larval mortality rates (White et al., 2014) into the numerical circulation model (Regional Ocean Modeling System; Drake et al., 2013). This would provide one of the first reliable estimates of realized population connectivity (Burgess et al., 2014). Clearly, a fresh look at the underlying processes responsible for spatial and temporal patterns of recruitment and population connectivity for diverse taxa and locations across upwelling coasts is needed to better understand the regulation of marine populations and communities (Shanks et al., 2017; Morgan et al., 2018; Shanks and Morgan, 2018).
In conclusion, larval retention is likely commonplace all along upwelling coasts with larvae recruiting closer to home and more abundantly than has been widely appreciated. Here, we demonstrated that this is even true at locations and times when upwelling is strongest, such as in the Point Arena upwelling center. This deeply affects the ecology, evolution and management of species in upwelling regimes. More larval retention should increase coupling between stock and recruitment, local adaptation and local benefits of marine protected areas as well as vulnerability of marine protected areas to overfishing and other disturbances (Strathmann et al., 2002; Sanford and Kelly, 2011; Burgess et al., 2014, 2016).
Author Contributions
SGM: Conceived of the research; SGM and JL: Were awarded funding to conduct the study; SHM, SGM, and MR: Conducted the study; SHM and MR: Processed, analyzed and graphed the data with SGM; SGM: Wrote the ms with input by all.
Conflict of Interest Statement
The authors declare that the research was conducted in the absence of any commercial or financial relationships that could be construed as a potential conflict of interest.
Acknowledgments
We thank the crew of the R/V Mussel Point, and M. Sheridan, S. Hameed, W. White, D. Dann, and J. Herum for assisting with sample collection. We also thank M. Losekoot for the HF Radar plots. This research was funded by California Sea Grant (R/FISH-2018) and is a contribution of the Bodega Marine Laboratory.
References
Arvedlund, M., and Kavanagh, K. (2009). “The senses and environmental cues used by marine larvae of fish and decapod crustaceans to find tropical coastal ecosystems,” in Connectivity Among Tropical Coastal Ecosystems, Ecological Part 2, ed I. N. Kerken (Frederiksberg: Springer Science and Business Media), 135–184.
Barth, J. A., Pierce, S. D., and Smith, R. L. (2000). A separating coastal upwelling jet at Cape Blanco, Oregon and its connection to the California Current System. Deep Sea Res. II Top. Stud. Oceanogr. 47, 783–810. doi: 10.1016/S0967-0645(99)00127-7
Bartilotti, C., dos Santos, A., Castro, M., Peliz, Á., and Santos, A. M. P. (2014). Decapod larval retention within distributional bands in a coastal upwelling ecosystem: a random episode or the rule? Mar. Ecol. Prog. Ser. 507, 233–247. doi: 10.3354/meps10817
Batchelder, H. P., Edwards, C. A., and Powell, T. M. (2002). Individual-based models of copepod populations in coastal upwelling regions: implications of physiologically and environmentally influenced diel vertical migration on demographic success and nearshore retention. Prog. Oceanogr. 53, 307–333. doi: 10.1016/S0079-6611(02)00035-6
Bonicelli, J., Tyburczy, J., Tapia, F. J., Finke, G. R., Parragué, M., Dudas, S., et al. (2016). Diel vertical migration and cross-shore distribution of barnacle and bivalve larvae in the central Chile inner-shelf. J. Exp. Mar. Biol. Ecol. 35, 35–46. doi: 10.1016/j.jembe.2016.08.013
Burgess, S. C., Baskett, M. L., Grosberg, R. K., Morgan, S. G., and Strathmann, R. R. (2016). When is dispersal for dispersal? Unifying marine and terrestrial perspectives. Biol. Rev. 91, 867–882. doi: 10.1111/brv.12198
Burgess, S. C., Nickols, K. J., Griesemer, C. D., Barnett, L. A. K., Dedrick, A. G., Satterthwaite, E., et al. (2014). Beyond larval connectivity: how empirical methods can quantify population persistence to improve marine protected area design. Ecol. Appl. 24, 257–270. doi: 10.1890/13-0710.1
Carr, S. D., Capet, X. J., McWilliams, J. C., Pennington, J. T., and Chavez, F. P. (2008). The influence of diel vertical migration on zooplankton transport and recruitment in an upwelling region: estimates from a coupled behavioral-physical model. Fish. Oceanogr. 17, 1–15. doi: 10.1111/j.1365-2419.2007.00447.x
Dever, E. P., Dorman, C. E., and Largier, J. L. (2006). Surface boundary layer variability off northern California, U. S. A., during upwelling. Deep Sea Res. II Top. Stud. Oceanogr. 53, 2887–2905. doi: 10.1016/j.dsr2.2006.09.001
Domingues, C. P., Nolasco, R., Dubert, J., and Queiroga, H. (2012). Model-derived dispersal pathways from multiple source populations explain variability of invertebrate larval supply. PLoS ONE 7:e35794. doi: 10.1371/journal.pone.0035794
Dorman, C. E., Dever, E. P., and Largier, J. L. (2005). Buoy measured wind, wind stress and curl of the wind stress over the shelf off Bodega Bay, California. Deep Sea Res. II Top. Stud. Oceanogr. 53, 2850–2864. doi: 10.1016/j.dsr2.2006.07.006
dos Santos, A., Santos, M. P., Conway, D. V. P., Bartilotti, C., Lourenco, P., and Queiroga, H. (2008). Diel vertical migration of decapod larvae in the Portuguese coastal upwelling ecosystem: implications for offshore transport. Mar. Ecol. Prog. Ser. 359, 171–183. doi: 10.3354/meps07341
Drake, P. T., Edwards, C. A., Morgan, S. G., and Dever, E. P. (2013). Influence of behavior on larval dispersal and population connectivity in a realistic simulation of the California current system. J. Mar. Res. 34, 317–350. doi: 10.1357/002224013808877099
Epifanio, C. E., and Cohen, J. H. (2016). Behavioral adaptations in larvae of brachyuran crabs: a review. J. Exp. Mar. Biol. Ecol. 482, 85–105. doi: 10.1016/j.jembe.2016.05.006
Epifanio, C. E., and Garvine, R. W. (2001). Larval transport on the Atlantic continental shelf of North America: a review. Estuar. Coast. Shelf Sci. 52, 51–77. doi: 10.1006/ecss.2000.0727
Fisher, J. L., Peterson, W. T., and Morgan, S. G. (2014). Does latitudinal variation in the intensity and persistence of upwelling regulate larval advection and supply? Mar. Ecol. Prog. Ser. 503, 123–137. doi: 10.3354/meps10732
Fujimura, A., Reniers, A. J. H. M , Paris, C. B., Shanks, A. L., MacMahan, J. H., and Morgan, S. G. (2014). Numerical simulations of larval transport into a rip-channeled surf zone. Limnol. Oceanogr. 59, 1434–1447. doi: 10.4319/lo.2014.59.4.1434
Fujimura, A. G., Reniers, A. J. H. M., Paris, C. B., Shanks, A. L., MacMahan, J. H., and Morgan, S. G. (2017). Numerical simulations of onshore transport of larvae and detritus to a steep pocket beach. Mar. Ecol. Prog. Ser. 582, 33–43. doi: 10.3354/meps12331
Fujimura, A. G., Reniers, A. J., Paris, C. B., Shanks, A. L., MacMahan, J. H., and Morgan, S. G. (2018). Mechanisms of Cross-Shore Transport and Spatial Variability of Phytoplankton on a Rip-Channeled Beach. Front. Mar. Sci. 5:183. doi: 10.3389/fmars.2018.00183
Fujimura, A., Reniers, A., Paris, C., Shanks, A., MacMahan, J., and Morgan, S. (2013). Slope-dependent biophysical modeling of surf zone larval transport. Coast. Dynam. 2013, 661–670.
Genin, A., Jaffe, J. S., Reef, R., Richter, C., and Franks, P. J. S. (2005). Swimming against the flow: a mechanism of zooplankton aggregation. Science 308, 860–862. doi: 10.1126/science.1107834
Graham, W. M., and Largier, J. L. (1997). Upwelling shadows as nearshore retention sites: the example of northern Monterey Bay. Cont. Shelf Res. 17, 509–532. doi: 10.1016/S0278-4343(96)00045-3
Grantham, B. A. (1997). Coastal Upwelling, Larval Recruitment, and the Dynamics of Upper Intertidal 9 Barnacle Communities. Dissertation, Stanford University (Palo Alto, CA).
Halle, C. M., and Largier, J. L. (2011). Surface circulation downstream of the Point Arena upwelling center. Cont. Shelf Res. 31, 1260–1272. doi: 10.1016/j.csr.2011.04.007
Hameed, S. O., Elliott, M. L., Morgan, S. G., and Jahncke, J. (2018). Interannual variation and spatial distribution of decapod larvae in a region of persistent coastal upwelling. Mar. Ecol. Prog. Ser. 587, 55–71. doi: 10.3354/meps12408
Hameed, S. O., White, J. W., Miller, S. H., Nickols, K. J., and Morgan, S. G. (2016). Inverse approach to estimating larval dispersal reveals limited population connectivity along 700 km of wave-swept open coast. Proc. R. Soc B 283:20160370. doi: 10.1098/rspb.2016.0370
Hickey, B. (1998). “Coastal oceanography of Western North America from the tip of Baja California to Vancouver Island,” in The Sea: Ideas and Observations on Progress in the Study of the Seas, eds A. Robinson and K. H. Brink (New York, NY: John Wiley & Sons), 10339–10368.
Hobbs, R. C., Botsford, L. W., and Thomas, A. (1991). Influence of hydrographic conditions and wind forcing to the distribution and abundance of dungeness crab, Cancer magister, larvae. Can. J. Fish. Aquat. Sci. 49, 1379–1388. doi: 10.1139/f92-153
Jamieson, G. S., and Phillips, A. C. (1988). Occurrence of Cancer crab (C. magister and C. oregonensis) megalopae off the west coast of Vancouver Island, British Columbia. Fish. Bull. 86, 525–542.
Kaplan, D. M., and Largier, J. L. (2006). HF-radar-derived origin and destination of surface waters off Bodega Bay, California. Deep Sea Res. II Top. Stud. Oceanogr. 53, 2906–2930. doi: 10.1016/j.dsr2.2006.07.012
Kaplan, D. M., Largier, J. L., and Botsford, L. W. (2005). HF radar observations of surface circulation off Bodega Bay northern California, U. S. A. J. Geophys. Res. 110:C10020. doi: 10.1029/2005JC002959
Kingsford, M. J., Leis, J. M., Shanks, A. L., Lindeman, K. C., Morgan, S. G., and Pineda, J. (2002). Sensory environments, larval abilities and local self-recruitment. Bull. Mar. Sci. 70, 309–340.
Kirincich, A. R., Barth, J. A., Grantham, B. A., Menge, B. A., and Lubchenco, J. (2005). Wind-driven inner-shelf circulation off central Oregon during summer. J. Geophys. Res. 110:C10SC03. doi: 10.1029/2004JC002611
Koracin, D., Dorman, C. E., and Dever, E. P. (2004). Coastal perturbations of marine-layer winds, wind stress, and wind stress curl along California and Baja California in June 1999. J. Phys. Oceanogr. 34, 1152–1173. doi: 10.1175/1520-0485(2004)034<1152:CPOMWW>2.0.CO;2
Largier, J. L. (2002). “Linking oceanography and nearshore ecology: perspectives and challenges,” in The Oceanography and Ecology of the Nearshore and Bays in Chile, eds J. C. Castilla and J. L. Largier (Santiago: Ediciones Universidad Catolica de Chile), 207–239.
Largier, J. L. (2003). Considerations in estimating larval dispersal distances from oceanographic data. Ecol. Appl. 13, S71–S89. doi: 10.1890/1051-0761(2003)013[0071:CIELDD]2.0.CO;2
Largier, J. L., Magnell, B. A., and Winant, C. D. (1993). Subtidal circulation over the northern California shelf. J. Geophys. Res. 98, 18147–18179. doi: 10.1029/93JC01074
Lentz, S. J., and Chapman, D. C. (1989). Seasonal differences in the current and temperature variability over the Northern California Shelf during the Coastal Ocean Dynamics Experiment. J. Geophys. Res. 94, 12571–12592. doi: 10.1029/JC094iC09p12571
Lough, R. (1974). Dynamics of Crab Larvae (Anomura, Brachyura) off the Central Oregon Coast, 1969-1971. Dissertation, Oregon State University (Corvallis, OR).
Mace, A. J., and Morgan, S. G. (2006a). Larval accumulation in the lee of a small headland: Implications the design of marine reserves. Mar. Ecol. Prog. Ser. 318, 19–29. doi: 10.3354/meps318019
Mace, A. J., and Morgan, S. G. (2006b). Biological and physical coupling in the lee of a small headland: contrasting larval transport mechanisms in an upwelling region. Mar. Ecol. Prog. Ser. 324, 185–196. doi: 10.3354/meps324185
Marta-Almeida, M., Dubert, J., Peliz, A., and Queiroga, H. (2006). Influence of vertical migration pattern on retention of crab larvae in a seasonal upwelling system. Mar. Ecol. Prog. Ser. 307, 1–19. doi: 10.3354/meps307001
Menge, B. A., and Menge, D. N. L. (2013). Dynamics of coastal meta-ecosystems: the intermittent upwelling hypotehsis and a test in rocky intertidal regions. Ecol. Monogr. 83, 283–310. doi: 10.1890/12-1706.1
Miller, S. H., and Morgan, S. G. (2013). Interspecific differences in depth preference regulate larval transport in an upwelling regime. Mar. Ecol. Prog. Ser. 476, 301–306. doi: 10.3354/meps10150
Montgomery, J. C., Jeffs, A., Simpson, S. D., Meekan, M., and Tindle, C. (2006). Sound as an orientation cue for the pelagic larvae of reef fishes and decapod crustaceans. Adv. Mar. Biol. 51, 143–196. doi: 10.1016/S0065-2881(06)51003-X
Morgan, S. G. (2006). “Larval migration between the Hudson River estuary and New York bight,” in The Hudson River Estuary, eds J. S. Levinton and J. R. Waldman (New York, NY: Cambridge University Press), 157–170.
Morgan, S. G. (2014). Behaviorally mediated larval transport in upwelling systems. Adv. Oceanogr. 2014:364214. doi: 10.1155/2014/364214
Morgan, S. G. (in press). “Dispersal,” in Developmental Biology and Larval Ecology, The Natural History of the Crustacea, Vol. VII, eds K. Anger, S. Harzsch, and M. Thiel (New York, NY: Academic Press).
Morgan, S. G., and Fisher, J. L. (2010). Larval behavior regulates nearshore retention and offshore migration in an upwelling shadow and along the open coast. Mar. Ecol. Prog. Ser. 404, 109–126. doi: 10.3354/meps08476
Morgan, S. G., Fisher, J. L., and Largier, J. L. (2011). Laval retention, entrainment and accumulation in the lee of a small headland: recruitment hotspots along windy coasts. Limnol. Oceanogr. 56, 161–178. doi: 10.4319/lo.2011.56.1.0161
Morgan, S. G., Fisher, J. L., and Mace, A. J. (2009a). Larval recruitment in a region of strong, persistent upwelling and recruitment limitation. Mar. Ecol. Prog. Ser. 394, 79–99. doi: 10.3354/meps08216
Morgan, S. G., Fisher, J. L., Mace, A. J., Akins, L., Slaughter, A. M., and Bollens, S. M. (2009b). Cross-shelf distributions and recruitment of crab postlarvae in a region of strong upwelling. Mar. Ecol. Prog. Ser. 380, 173–185. doi: 10.3354/meps07913
Morgan, S. G., Fisher, J. L., McAfee, S. T., Largier, J. L., and Halle, C. M. (2012). Limited recruitment during relaxation events: larval advection and behavior in an upwelling system. Limnol. Oceanogr. 57, 457–470. doi: 10.4319/lo.2012.57.2.0457
Morgan, S. G., Fisher, J. L., Miller, S. H., McAfee, S. T., and Largier, J. L. (2009c). Nearshore larval retention in a region of strong upwelling and recruitment limitation. Ecology 90, 3489–3502. doi: 10.1890/08-1550.1
Morgan, S. G., Shanks, A. L., MacMahan, J. H., Reniers, A. J. H. M., and Fedderson, F. (2018). Planktonic subsidies to surf zone and intertidal communities. Ann. Rev. Mar. Sci. 10, 345–369. doi: 10.1146/annurev-marine-010816-060514
Morgan, S. G., Shanks, A. L., MacMahan, J. H., Reniers, A. J. H. M., Griesemer, C. D., Jarvis, M., et al. (2016). Surfzone hydrodynamics as a key determinant of spatial variation in marine communities. Proc. R. Soc. B 283:20161017. doi: 10.1098/rspb.2016.1017
Morgan, S. G., Shanks, A. L., MacMahan, J. H., Reniers, A. J. H. M., Griesemer, C. D., Jarvis, M., et al. (2017). Surf zones regulate larval supply and zooplankton subsidies to nearshore communities. Limnol. Oceanogr. 62, 2811–2828. doi: 10.1002/lno.10609
Nickols, K. J., Gaylord, B., and Largier, J. L. (2012). The coastal boundary layer: predictable current structure decreases alongshore transport and alters scales of dispersal. Mar. Ecol. Prog. Ser. 464, 17–35. doi: 10.3354/meps09875
Nickols, K. J., Miller, S. H., Gaylord, B., Morgan, S. G., and Largier, J. L. (2013). Spatial differences in larval supply within the coastal boundary layer impact availability to shoreline habitats. Mar. Ecol. Prog. Ser. 494, 191–203. doi: 10.3354/meps10572
Nolasco, R., Dubert, J., Domingues, C. P., Cordeiro Pires, A., and Queiroga, H. (2013). Model-derived connectivity patterns along the western Iberian Peninsula: asymmetrical larval flow and source-sink cell. Mar. Ecol. Prog. Ser. 48, 123–142. doi: 10.3354/meps10324
Papastephanou, K. M., Bollens, S. M., and Slaughter, A. M. (2006). Cross-shelf distribution of copepods and the role of event-scale winds in a northern California upwelling zone. Deep-Sea Res. II Top. Stud. Oceanogr. 53, 3078–3093. doi: 10.1016/j.dsr2.2006.07.014
Parrish, R. H., Nelson, C. S., and Bakun, A. (1981). Transport mechanisms and the reproductive success of fishes in the California Current. Biol. Oceanogr. 1, 175–203.
Petersen, C. H., Drake, P. T., Edwards, C. A., and Ralston, S. (2010). A numerical study of inferred rockfish (Sebastes spp.) larval dispersal along the central California coast. Fish. Oceanogr. 19, 21–41. doi: 10.1111/j.1365-2419.2009.00526.x
Peterson, W. T. (1998). Life cycle strategies of copepods in coastal upwelling zones. J. Mar. Syst. 15, 313–326. doi: 10.1016/S0924-7963(97)00082-1
Peterson, W. T., Miller, C. B., and Hutchinson, A. (1979). Zonation and maintenance of copepod populations in the Oregon upwelling zone. Deep-Sea Res. A Oceanogr. Res. Papers 26, 467–494. doi: 10.1016/0198-0149(79)90091-8
Pettigrew, N. R., and Murray, S. P. (1986). “The coastal boundary layer and inner shelf,” in Barocline Processes Continental Shelves, ed C. N. K. Mooers (Washington, DC: American Geophysical Union), 95–108.
Pfeiffer-Herbert, A. S., McManus, M. A., Raimondi, P. T., Chao, Y., and Chai, F. (2007). Dispersal of barnacle larvae along the central California coast: a modeling study. Limnol. Oceanogr. 52, 1559–1569. doi: 10.4319/lo.2007.52.4.1559
Pineda, J. (1994). Spatial and temporal patterns in barnacle settlement rate along a southern California rocky shore. Mar. Ecol. Prog. Ser. 107, 125–138. doi: 10.3354/meps107125
Pineda, J. (1999). Circulation and larval distribution in internal tidal bore warm fronts. Limnol. Oceanogr. 44, 1400–1414. doi: 10.4319/lo.1999.44.6.1400
Poulin, E., Palma, A. T., Leiva, G., Narvaez, D., Pacheco, R., Navarette, S. A., et al. (2002). Avoiding offshore transport of competent larvae during upwelling events: the case of the gastropod Concholepas concolepas in Central Chile. Limnol. Oceanogr. 47, 1248–1255. doi: 10.4319/lo.2002.47.4.1248
Queiroga, H., and Blanton, J. (2005). Interactions between behaviour and physical forcing in the control of horizontal transport of decapod Crustacean larvae. Adv. Mar. Biol. 47, 107–214. doi: 10.1016/S0065-2881(04)47002-3
Rasmuson, L. K., and Shanks, A. L. (2013). In situ observations of Dungeness crab megalopae used to estimate transport distances by internal waves. Mar. Ecol. Prog. Ser. 511, 143–152. doi: 10.3354/meps10926
Robart, M. J. (2013). Suppression of Upwelling at the Windward Shore of Point Reyes, California. MS Thesis, University of California Davis. (Davis, CA)
Rosenfeld, L. K. (1990). Baroclinic semidiurnal tidal currents over the continental-shelf off northern California. J. Geophys. Res. 95, 22153–22172. doi: 10.1029/JC095iC12p22153
Roughan, M., Mace, A. J., Largier, J. L., Morgan, S. G., Fisher, J. L., and Carter, M. L. (2005). Subsurface recirculation and larval retention in the lee of a small headland: a variation on the upwelling shadow theme. J. Geophys. Res. Oceans 110, 1–18. doi: 10.1029/2005JC002898
Roughan, M., Garfield, N., Largier, J., Dever, E., Dorman, C., Dorman, D., et al. (2006). Transport and retention in an upwelling region: the role of across-shelf structure. Deep Sea Res. II Top. Stud. Oceanogr. 53, 2931–2955. doi: 10.1016/j.dsr2.2006.07.015
Roughgarden, J., Gaines, S. D., and Possingham, H. P. (1988). Recruitment dynamics in complex life cycles. Science 241, 1460–1466. doi: 10.1126/science.11538249
Ryan, J. P., Harvey, J. B. J., Zhang, Y., and Woodson, C. B. (2014). Distributions of invertebrate larvae and phytoplankton in a coastal upwelling system retention zone and peripheral front. J. Exp. Mar. Biol. Ecol. 459, 51–60. doi: 10.1016/j.jembe.2014.05.017
Sanford, E., and Kelly, M. W. (2011). Local adaptation in marine invertebrates. Ann. Rev. Mar. Sci. 3, 509–535. doi: 10.1146/annurev-marine-120709-142756
Send, U., Beardsley, R. C., and Winant, C. D. (1987). Relaxation from upwelling in the coastal ocean dynamics experiment. J. Geophys.Res. 92, 1683–1698. doi: 10.1029/JC092iC02p01683
Shanks, A. L. (1995a). “Mechanisms of cross-shelf dispersal of larval invertebrates and fishes,” in Marine Community Ecology, eds M. D. Bertness, S. D. Gaines, and M. E. Hay (Sunderland, MA: Sinauer), 323–367.
Shanks, A. L. (1995b). Orientated swimming by megalopae of several Eastern North Pacific crab species and its potential role in their onshore migration. J. Exp. Mar. Biol. Ecol. 186, 1–16.
Shanks, A. L., and Brink, L. (2005). Upwelling, downwelling, and cross-shelf transport of bivalve larvae: test of a hypothesis. Mar. Ecol. Prog. Ser. 302, 1–12. doi: 10.3354/meps302001
Shanks, A. L., MacMahan, J., Morgan, S. G., Reniers, A. J. H. M., Jarvis, M., Brown, J., et al. (2018). Persistent differences in horizontal gradients in phytoplankton concentration maintained by surfzone hydrodynamics. Estuar. Coasts. 41, 58–176. doi: 10.1007/s12237-017-0278-2
Shanks, A. L., MacMahan, J., Morgan, S. G., Reniers, A. J. H. M., Jarvis, M., Brown, J., et al. (in press). Surfzone hydrodynamics controls phytoplankton concentration. Mar. Ecol. Prog. Ser.
Shanks, A. L., and Morgan, S. G. (2018). Testing the intermittent upwelling hypothesis: upwelling, downwelling, and subsidies to the intertidal zone. Ecol. Monogr. 88, 22–35. doi: 10.1002/ecm.1281
Shanks, A. L., Morgan, S. G., MacMahan, J. H., and Reniers, A. J. H. M. (2017). Alongshore variation in barnacle populations is determined by surfzone hydrodynamics. Ecol. Monogr. 87, 508–532. doi: 10.1002/ecm.1265
Shanks, A. L., Morgan, S. G., MacMahan, J., and Reniers, A. J. H. M. (2010). Surf zone physical and morphological regime as determinants of temporal and spatial variation in larval recruitment. J. Exp. Mar. Biol. Ecol. 392, 140–150. doi: 10.1016/j.jembe.2010.04.018
Shanks, A. L., and Shearman, R. K. (2009). Paradigm lost? Cross-shelf distributions of intertidal invertebrate larvae are unaffected by upwelling or downwelling. Mar. Ecol. Prog. Ser. 385, 189–204. doi: 10.3354/meps08043
Shannon, L. V., Nelson, G., and Jury, M. R. (1981). “Hydrological and meteorological aspects of upwelling in the southern Benguela Current,” in Coastal and Estuarine Sciences 1. Coastal Upwelling, ed F. A. Richard (Washington, DC: American Geophysical Union), 146–159.
Shenker, J. M. (1988). Oceanographic associations of neustonic larval and juvenile fishes and Dungeness crab megalopae off Oregon. Fish. Bull. 86, 299–317.
Strathmann, R., Hughes, T., Kuris, A., Lindeman, K., Morgan, S., Pandolfi, J., et al. (2002). Evolution of local recruitment and its consequences for marine populations. Bull. Mar. Sci. 70, 377–396.
Tapia, F. J., and Pineda, J. (2007). Stage-specific distribution of barnacle larvae in nearshore waters: potential for limited dispersal and high mortality rates. Mar. Ecol. Prog. Ser. 342, 177–190. doi: 10.3354/meps342177
Vander Woude, A. J., Largier, J. L., Lucas, A. J., and Kudela, R. M. (2006). Nearshore retention of upwelled waters in northern California embayments—satellite derived patterns of surface temperature and chlorophyll. Deep Sea Res. II Top. Stud Oceanogr. 53, 2851–2998. doi: 10.1016/j.dsr2.2006.07.003
White, J. W., Morgan, S. G., and Fisher, J. L. (2014). Larval mortality rates are lower than widely expected. Ecology 95, 3344–3353. doi: 10.1890/13-2248.1
Winant, C. D., Dorman, C. E., Friehe, C. A., and Beardsley, R. C. (1988). The marine layer off Northern California: An example of supercritical channel flow. J. Atmos. Sci. 45, 3588–3605.
Wing, S. R., Botsford, L., Largier, J. L., and Morgan, L. E. (1995). Spatial variability in settlement of benthic invertebrates in a northern California upwelling system. Mar. Ecol. Prog. Ser. 128, 199–211. doi: 10.3354/meps128199
Wing, S. R., Botsford, L., Morgan, L. E., Diehl, J. M., and Lundquist, C. J. (2003). Inter-annual variability in larval supply to populations of three invertebrate taxa in the northern California Current. Estuar. Coast. Shelf Sci. 57, 859–872. doi: 10.1016/S0272-7714(02)00416-X
Wing, S. R., Botsford, L. W., Ralston, S. V., and Largier, J. L. (1998). Meroplanktonic distribution and circulation in a coastal retention zone of the northern California upwelling system. Limnol. Oceanogr. 43, 1710–1721. doi: 10.4319/lo.1998.43.7.1710
Keywords: larval transport, behavior, population connectivity, recruitment limitation, upwelling
Citation: Morgan SG, Miller SH, Robart MJ and Largier JL (2018) Nearshore Larval Retention and Cross-Shelf Migration of Benthic Crustaceans at an Upwelling Center. Front. Mar. Sci. 5:161. doi: 10.3389/fmars.2018.00161
Received: 24 January 2018; Accepted: 23 April 2018;
Published: 15 May 2018.
Edited by:
Claire Beatrix Paris, University of Miami, United StatesReviewed by:
Jan Marcin Weslawski, Institute of Oceanology (PAN), PolandGorka Bidegain, University of Southern Mississippi, United States
Copyright © 2018 Morgan, Miller, Robart and Largier. This is an open-access article distributed under the terms of the Creative Commons Attribution License (CC BY). The use, distribution or reproduction in other forums is permitted, provided the original author(s) and the copyright owner are credited and that the original publication in this journal is cited, in accordance with accepted academic practice. No use, distribution or reproduction is permitted which does not comply with these terms.
*Correspondence: Steven G. Morgan, c2dtb3JnYW5AdWNkYXZpcy5lZHU=