- 1Department of Marine Sciences, University of Connecticut, Groton, CT, United States
- 2Key Laboratory of Tropical Marine Bio-Resources and Ecology, South China Sea Institute of Oceanology, Chinese Academy of Science, Guangzhou, China
Strombidium rassoulzadegani is a planktonic ciliate that retains chloroplasts from its food and uses them to obtain a nutritional supplement from photosynthesis. Unlike most members of the Oligotrichia, it is not difficult to grow in culture and thus it can serve as an experimental model for this kind of mixotrophy. We report here on its distribution, seasonal pattern of occurrence in the western North Atlantic, and on experiments to elucidate patterns of encystment and excystment, preferred food algae, and heterotrophic growth. Among ten different microalgae, including members of the Dinophyceae, Chlorophyceae, Haptophyceae, Cryptophyceae, and Bacillariophyceae, only four could support growth for more than 1 week, and only the chlorophyte Tetraselmis chui (PLY 429) could consistently support sustained growth in the dark. Of the four algae that supported growth, three also resulted in longer survival when the ciliate was subsequently starved in the light, compared to the dark, suggesting that all of them provided some photosynthetic benefit to the ciliate. The dinoflagellate Prorocentrum minimum (JA) supported similar survival in the light and dark and likely does not undergo chloroplast retention in the ciliate.
Introduction
The widespread application of fluorescence microscopy in plankton studies during the 1980s resulted in the observation of chloroplasts in many marine ciliates, a group that had previously been considered wholly heterotrophic (the sole exception being the remarkably unique Mesodinium rubrum) (Laval-Peuto et al., 1986; McManus and Fuhrman, 1986; Stoecker et al., 1987). Plastids appeared to be “arranged” around the periphery of the cell in some cases, and could be distinguished clearly from ingested food algae in vacuoles. Some observations of chloroplasts in ciliates had been made earlier (e.g., Blackbourn et al., 1973), but these came before it was widely appreciated that ciliates are abundant and ecologically important members of marine plankton communities. Subsequent lab and field work indicated that chloroplast retention by ciliates was widespread, comprising up to half of the assemblage, and that the chloroplasts were physiologically functional within the ciliates (Stoecker and Silver, 1987; Stoecker et al., 1987; Putt, 1990).
The capacity of some ciliates to both eat and photosynthesize with retained chloroplasts has been referred to as a kind of mixotrophy. The overall prevalence of mixotrophy in the protist plankton has come to be better appreciated in recent years. Phytoplankton that can eat and microzooplankton that can photosynthesize are probably the rule rather than the exception, and efforts are underway to quantify mixotrophy and incorporate it into models of the ocean's food web (Flynn and Mitra, 2009). To this end, a new lexicon of terms describing mixotrophy has been created to categorize organisms according to whether they have permanent photosynthetic capability (constitutive mixotrophs) or acquire this capacity from other organisms, either through symbiosis or retention of chloroplasts from algal food, and further, whether the mixotrophy is acquired from only one or multiple algal species (Flynn et al., 2013; Mitra et al., 2016). In this context, the organism that is the subject of this paper, Strombidium rassoulzadegani, is a “non-constitutive generalist” mixotrophic ciliate that can use plastids from multiple prey (Schoener and McManus, 2012; see also McManus et al., 2004, reported as S. stylifer). Other terms for this form of mixotrophy include “kleptoplastidy/kleptoplasty” and “chloroplast symbiosis”, as reviewed in Stoecker (1998), Stoecker et al. (2009), and Johnson (2011).
We first observed S. rassoulzadegani by its DNA signature as a member of a cryptic species complex related to S. oculatum. The latter had long been studied from tide pools in Europe, where it apparently has a tidal period of encystment/excystment (Faure-Fremiet, 1948; Jonsson, 1994; Montagnes et al., 2002b). In 2003, we isolated S. rassoulzadegani from a tide pool in Connecticut, USA, brought it into culture, and described it as a new species (McManus et al., 2010). Since that time, we have isolated it more than a dozen times and maintained individual isolates for up to 6 years. A number of papers have described its growth, photosynthesis, and nutrient utilization (McManus et al., 2012; Schoener and McManus, 2012, 2017). Its transcriptome was obtained recently through the Marine Microbial Eukaryote Transcriptome Sequencing Project (Santoferrara et al., 2014). The practice of retaining chloroplasts from ingested food appears to be widespread in the ciliate subclass Oligotrichia, of which the Strombidiidae comprise a family, but progress in understanding the physiology and ecological importance of this phenomenon has been hampered by the difficulty of cultivating these ciliates (cf. Montagnes et al., 1996). Because S. rassoulzadegani is an easily-cultivated exception to this rule, it can serve as a good model organism for studying chloroplast retention, in particular the costs and benefits of this nutritional mode. The purpose of the present paper is to discuss what we have learned from field and culture studies about the global distribution of S. rassoulzadegani, its encystment/excystment patterns, utilization of different algal foods, and heterotrophic growth, and to recommend procedures for its isolation and maintenance in culture.
Methods
Distribution
We previously designed primers specific to S. rassouzadegani for the ITS region of the ribosomal operon (ITS1-5.8S-ITS2; GenBank DQ241747), and tested them for specificity against DNAs from tide pools where multiple members of the cryptic species cluster were known to be present (McManus et al., 2010). Using this primer set (F1: TGCTAATCCAATCCAACTCAACCA; R1: GAGCCCAGATACGATTCCAAAGT), we documented presence/absence of S. rassoulzadegani in tidepool samples from the North and South Atlantic and Pacific Oceans (Table S1), and in a time series of samples collected weekly or biweekly over an annual cycle from two shore sites in Connecticut, USA (March 2009–May 2010). Samples of 60 ml were collected by us or colleagues, filtered through cellulose nitrate filters (Whatman, 3.0 μm pore-size) and stored in lysis buffer prior to extraction and amplification. PCR methods were as described in Katz et al. (2005), including both positive and negative controls. Cycling conditions were: initial 95°C for 2 min, followed by 35 cycles of 95°C for 0.5 min, 55°C for 0.5 min, 72°C for 1.5 min, then a final 72°C for 2 min and hold at 4°C. Presence on a gel of the appropriate-sized PCR product was interpreted as presence of the ciliate. Ten percent of positive results were cut from gels and sequenced for verification.
Isolation and Cultivation
S. rassoulzadegani is readily found in tide pools at mid-latitudes in the North Atlantic. It is often abundant enough to pick directly from unconcentrated water samples using a drawn capillary and exploiting its strong positive phototaxis, or it can be harvested a few days later from samples enriched with the green alga Tetraselmis chui (PLY 429). Although we have sometimes picked its congener S. apolatum from the same samples (verified by ITS sequence), we have never been able to successfully keep the latter in culture. All experiments reported here were performed using an isolate from Appledore Island, ME, USA, collected in August 2016.
Cultures of S. rassoulzadegani are routinely maintained in the lab at 20°C, salinity 28, on a 12:12 L:D photoperiod at c. 70–100 μmol m−2 s−1. They grow best on the green alga Tetraselmis chui (PLY 429), and under those conditions, growth saturates at a food concentration of about 2.5 × 104 cells ml−1 and light at 50 μmol m−2 s−1 (McManus et al., 2012). We have had the most success growing cultures in f/2 medium (Guillard and Ryther, 1962) in six-well plastic culture plates, though larger cultures can be successful when more material is needed for molecular or physiological studies and more dilute medium (f/20) is sometimes used to slow down the growth of the food algae (Schoener and McManus, 2017). Culture maintenance and temperature-controlled experiments were done in a reach-in incubator (Thermo Scientific) with controlled temperature and light (using 60 watt cool-white fluorescent bulbs).
Microscopy
Light microscope images were taken with bright-field or differential interference contrast illumination on an Olympus BX50 compound microscope, using either an Olympus Magna-Fire (color) or Hammamatsu C11440 (monochrome) digital camera. For transmission electron microscopy, cultures of S. rassoulzadegani (approximately 100 ml) were concentrated to 1 ml over a 25 mm diameter 0.45 μm pore size filter. Concentrated samples were fixed for 30 min by mixing 1:1 with 2.5% glutaraldehyde and 2.5% paraformaldehyde buffered with 0.1 M Na cacodylate (pH 7.4) in sterile seawater. Fixed samples were pelleted by centrifugation and prepared for electron microscopy as described previously (Stanton et al., 1981). Ultrathin sections were examined and photographed using a FEI Biotwin (LaB6, 80 kv) transmission electron microscope.
Sizes of plastids in light- and dark-grown cells were measured on live cells suspended in a drop of 0.4 M sucrose (isotonic to the chloroplasts) and squashed between cover slip and slide to release the plastids. Plastid dimensions in digital images were measured with NIS Elements software and volumes calculated assuming either spherical or prolate spheroid shape.
Encystment/Excystment
New ciliate cultures generally produce cysts during the first few weeks of cultivation, but long-term cultures seem to lose this habit, possibly due to selection for only active trophic cells during culture transfers because the cysts adhere to the culture well bottoms. Encystment experiments were conducted in six-well plates in triplicate, with triplicate controls, using relatively new cultures from which cells had recently excysted. For salinity shock experiments, cells were suspended in f/2 medium at salinities of 5, 10, 15, 20, 30, 45, or 60 (practical salinity scale), with controls at the standard culture salinity of 28. Other treatments included presence/absence of predators (copepods), evaporation to dryness, cold (4°C) and darkness, and starvation. We also compared cyst formation in control cultures (20°C 12:12 L:D) with those exposed to shortened photoperiod (12:12 L:D to 9.5:14.5 over 12 days), gradual temperature reduction (20°−10°C over 12 days), or both photoperiod and temperature reduction at the same time. Comparison of treatments was done by t-test.
To attempt to induce excystment, culture wells containing cysts adhering to the bottom were treated with additions of dried macroalgae (Ulva sp.), filtered medium in which T. chui had been grown, additions of S. rassoulzadegani trophic cells from other cultures, or rinses of fresh autoclaved f/2 medium. The cysts are very sticky and cannot be picked up by drawn capillary, the way trophic cells can, so we just waited until enough cysts had accumulated in the culture wells to start an experiment by removing all trophic cells. Although we did not quantify them precisely, there were hundreds of cysts per well and they accumulated in the cultures over several weeks. Thus, we did not test for emergence from cysts that had been encysted for months or years. However, given the very short duration of encystment in congener S. oculatum (tidal cycle of encystment/excystment) we considered that short-term experiments were appropriate.
Food Preference Experiments
Ten different algal strains were tested for the ability to support survival and growth in light (12:12 L:D cycle at 70 μmol m−2 s−1) or dark (24 D, inside an insulated styrofoam container) conditions (Table 1). S. rassoulzadegani cells were picked from cultures growing on PLY 429 and placed, 20 per well, into six-well plates containing the different food algae and f/2 medium. Because no quantitative statistical results were produced in these initial tests, the treatments were not replicated. All algae were obtained from the National Marine Fisheries Service/NOAA Laboratory collection in Milford CT, except for ISO SP. The latter was isolated by us from our ciliate cultures. Using universal primers (Medlin et al., 1988), we sequenced the small-subunit (SSU) and used BLAST at the NCBI website to identify it as 99% similar to Isochrysis sp. strain CCAP 927/14 (NCBI accession number DQ079859). The latter is synonymous with the TISO strain, according to the culture data in the National Center for Marine Algae. The amount of food algae was judged by eye and adjusted each day to avoid over- or under-feeding (growth is saturated above 104 cells ml−1; McManus et al., 2004). Initially, wells were observed every day for 6 days (short-term experiments). After 6 days, the wells with 100% mortality were emptied and those treatments were restarted with the same algae and 20 new S. rassouladegani cells. Those, and the wells in which ciliates were still alive, were followed for a further 4 weeks, with growth and survival monitored in the same way (long-term experiments).
Heterotrophic vs. Mixotrophic Growth
After we had established that S. rassoulzadegani could be grown heterotrophically in darkness if sufficient care was given to ensure saturating concentrations of fresh PLY 429 were added daily, we performed 5 separate experiments to measure growth under light (12:12 L:D) or dark (24 D) conditions. Cultures were started in f/2 medium in well-plates with 10–30 ciliates per triplicated well and fed abundant PLY 429. Plates were either kept in darkness (24 D, inside an insulated styrofoam container) or light (12:12 L:D at 70 μmol m−2 s−1). Light, or lack thereof, was measured with an Apogee model MQ-200 quantum light meter. After 5–8 days, well contents were preserved with lugols iodine solution and ciliates were counted with an inverted microscope. Growth rates were calculated assuming exponential growth, in units of d−1, as μ = ln(Nt/N0)/Δt, where N0 and Nt are ciliate abundances at the beginning and end of the experiment, respectively, and Δt is the experimental duration in days.
Starvation Experiments
Ciliates grown out in each of the four most suitable algal foods (PLY 429, RHODO, JA, and ISO SP) were rinsed and placed, 20 per well, in triplicate wells in 12-well plates with 4 ml of filtered, autoclaved f/2 medium and no food. Plates were placed in light (12:12 L:D) or darkness and monitored for survivors until all were dead (3–8 d, depending on treatment).
Results
Distribution
We have isolated cultures of S. rassoulzadegani from tide pools in Dunbar, Scotland, the northeast USA (Maine and Connecticut), and from open water nearshore in Sao Sebastiao, Brazil (CEBIMAR marine laboratory, U. Sao Paolo). We have found it reliably during summer in the relatively pristine tide pools of Shoals Marine Lab (Appledore Island, Maine), and have sometimes observed it co-occurring with its close congener S. apolatum, though we have never succeeded in keeping the latter under cultivation for more than a day or so. Based on finding its ITS sequence in filtered water samples, we conclude that it is globally distributed (Figure 1; Table S1).
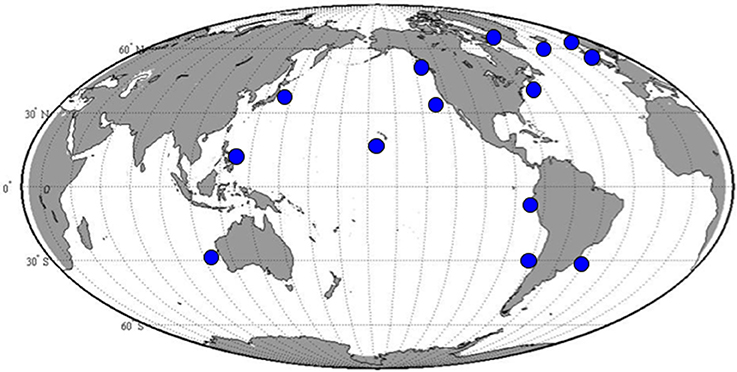
Figure 1. Positive results for amplification with S. rassoulzadegani species-specific ITS-region PCR primers.
At two shore sites on Long Island Sound, USA, we sampled 41 (Groton, CT) and 32 (Madison, CT) times over a 14-month period, collecting water from three different tide pools each time. At Groton, S. rassoulzadegani rDNA was present in at least one pool on all sampling dates from May through October and on a few dates in March and April. It was never detected from November through February. In Madison, which is 45 km closer to the eutrophic western end of the Sound, S. rassoulzadegani rDNA signature was seen in at least one pool in every month except December.
Isolation and Cultivation
Although it was initially cultured on “swarmer cells” (zoospores or gametes) of a green macroalga, Ulva sp. (McManus et al., 2004), we have subsequently isolated and maintained S. rassoulzadegani primarily on the green microalga Tetraselmis chui (strain PLY 429), a common food in aquaculture applications. In culture, the cells are grass-green, with a prominent reddish eyespot consisting of a cluster of pigment granules that appear to originate in the food alga. They show strong positive phototaxis, swimming in a helical pattern toward the light, which can help to concentrate them from natural populations for isolation. In culture, they can achieve densities of >103 cells/ml.
Encystment/Excystment
S. rassoulzadegani produces a typical oligotrich cyst, consisting of a roughly spherical body, with a short neck and a frothy-looking plug (Montagnes et al., 2002a; Figure 2). The cysts appear to be surrounded with a sticky organic layer to which bacteria and algae in the cultures adhere. It does not appear to encyst/excyst on a tidal cycle, as described for its tidepool congener S. oculatum. We did not observe any other distinct periodicity to the cyst cycle. Trophic cells did not encyst in response to sudden changes in salinity (range 5–60, with no survival at the highest and lowest salinities), the presence of predators (copepods), evaporation of the medium to dryness, starvation, or cold/dark (4°C) conditions. A gradual reduction in temperature, from 20° to 10°C over 12 days under constant light conditions (12:12 photoperiod at c. 70 μmol m−2 s−1) did not induce encystment. Neither did a gradual decrease in the light period (from 12:12 to 9.5:14.5) at constant 20°C. However, simultaneous reductions in temperature and light did result in significantly more cysts, compared to controls at 20°C and 12:12 (Figure 3A).
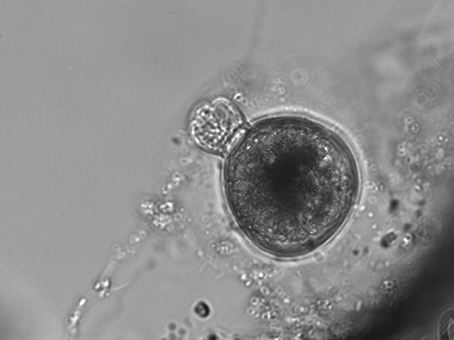
Figure 2. Typical S. rassoulzadegani cyst, with “frothy plug” at top left, and adhering organic layer. Cyst diameter is c. 40 μm.
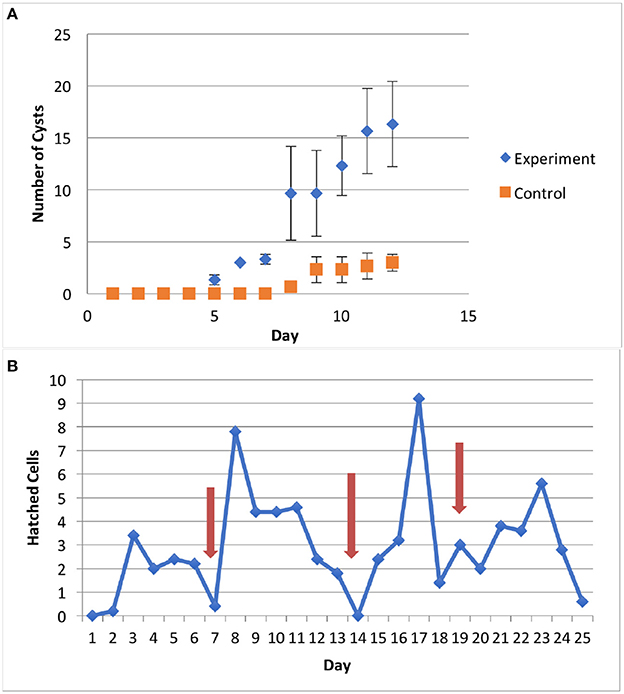
Figure 3. (A) Results of encystment experiment. Shortened photoperiod (12:12–9.5:14.5) and decrease in temperature (20°-10°C) over 12 days induced formation of more cysts, compared to controls (12:12, 20°C); error bars are one standard deviation (n = 3). (B) Excystment was stimulated by rinsing cysts with fresh medium (arrows).
Excystment was not induced by additions of algal exudates (T. chui spent medium), dried Ulva sp., or trophic cells of S. rassoulzadegani. Rinsing cysts with fresh medium (filtered, autoclaved f/2 seawater) was the only treatment with which we successfully stimulated excystment (Figure 3B).
Microscopy
Plastids within the ciliate appear to be free in the cytoplasm (not surrounded by ciliate vacuolar membrane), and are found in various shapes and sizes, even when derived from a single food alga (Figures 4A,B). Under the light microscope, some plastids are smaller, rounder, and darker (color changes from green to dark green), possibly with age (Figure 5). When ciliates were grown on Tetraselmis chui (PLY 429) in light (12:12) or dark conditions, the cells still contained abundant chloroplasts, but the plastids were much smaller in dark-grown cells (mean volume per plastid of 107 vs. 37 μm3 in light vs. dark, respectively; P < 0.001 t-test).
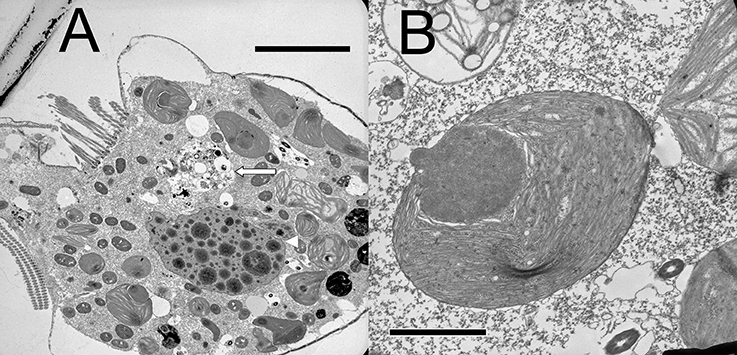
Figure 4. (A) Lower magnification thin section of S. rassoulzadegani cell with chloroplasts in varying shapes and sizes, digestive vacuole (long arrow), and the ciliate macronucleus (arrowhead); scale bar = 10 μm. (B) Individual Tetraselmis chui chloroplast retained within S. rassoulzadegani; scale bar = 2 μm.
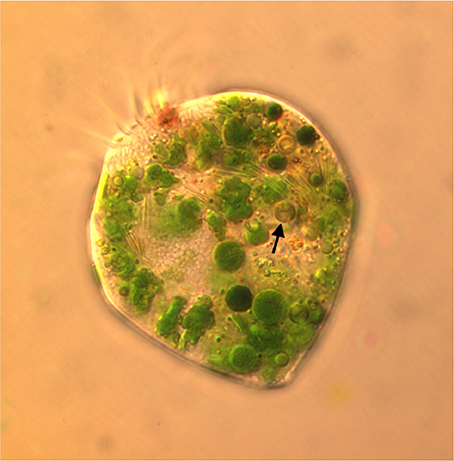
Figure 5. Micrograph of S. razoulzadegani fed Tetraselmis chui (PLY 429), showing variety of shapes and sizes of retained chloroplasts, including smaller dark green and brownish ones (arrow) that may be older than the larger “grass-green” ones.
Food Preference and Heterotrophic Growth Experiments
In short-term experiments (6 d), S. rassoulzadegani achieved positive growth when fed Tetraselmis chui (PLY 429), Rhodomonas lens (RHODO), Isochrysis sp. (ISO SP), and Prorocentrum minimum (JA) (Figure 6). On all other foods (the diatom T. weissflogii, the haptophyte I. galbana TISO, and the dinoflagellates P. minimum EXUV, A. carterae, A. minutum, and S. lachrymosa; Table 1) the ciliates were all dead by the end of the experiment. In each case of successful growth, cells that were grown on PLY 429 and switched to another food replaced the PLY 429 chloroplasts almost completely by the end of the experiment. As with PLY 429, the chloroplasts of the other algae appeared under light microscopy to be free in the cytoplasm (Figures 6A–C). A caveat is that this can only be verified with electron microscopy, whereas we have only observed PLY 429 and RHODO (data not shown) by transmission electron microscopy. In the dinoflagellate JA, on the other hand, the algae appear to be digested in vacuoles (Figure 6D). When held in darkness for 6 d with ample food, only Tetraselmis chui (PLY 429) and Isochrysis galbana (TISO) supported net positive growth in the ciliate, though we have observed short-term positive growth in both light and darkness on Rhodomonas lens (RHODO) and Prorocentrum minimum (EXUV) as well (unpublished data and McManus et al., 2012).
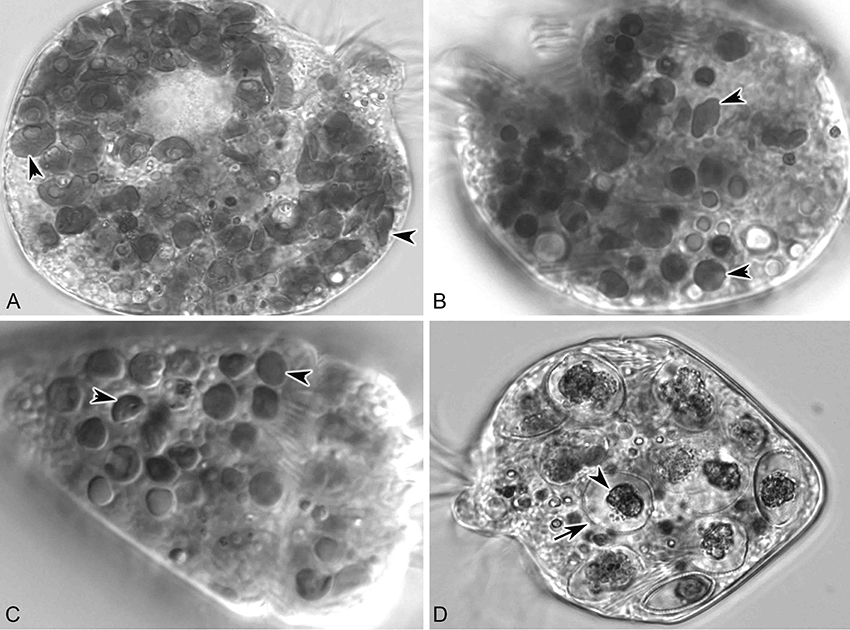
Figure 6. S. rassoulzadegani fed four food algae that supported positive growth in the light (12:12 L:D). (A) S. rassoulzadegani fed T. chui PLY429; arrowheads indicate different shapes and sizes of retained chloroplasts; (B) S. rassoulzadegani fed R. lens RHODO; arrowheads as in A; (C) S. rassoulzadegani fed Isochrysis sp. ISO SP; arrowheads as in A; (D) S. rassoulzadegani fed P. minimum JA; note that no retained plastids appear in the cell. Arrow marks the rigid wall of the algal cell and arrowhead marks the partially-digested algal cytoplasm.
In longer-term experiments (5 weeks), PLY 429, RHODO, ISO SP, and JA supported survival of the ciliate under 12:12 L:D conditions. We had noticed that in RHODO, ISO SP, and JA treatments, the algae sometimes overgrew the ciliates, causing cultures to crash, so sustained growth could only be obtained by carefully controlling the algal abundance. Only PLY 429 supported long-term growth even at very high algal densities, and only PLY 429 supported long-term growth (several months) in darkness. Even after 4 weeks of cultivation in darkness on PLY 429, the ciliates continued to retain some chloroplasts from their food (Figures 7A,D). The ciliates died within 3 weeks in the dark in all other food treatments. One issue we faced in trying to grow the mixotrophic ciliate on these foods even for PLY 429 in the dark is that fresh algal food had to be added daily or every second day because the algae appeared to become less motile in the dark, possibly declining in nutritional value. This may have been a factor leading to the earlier conclusion that mixotrophy was “obligate” (no growth in darkness even with abundant food) in plastid-retaining ciliates (McManus et al., 2004, reported as S. stylifer).
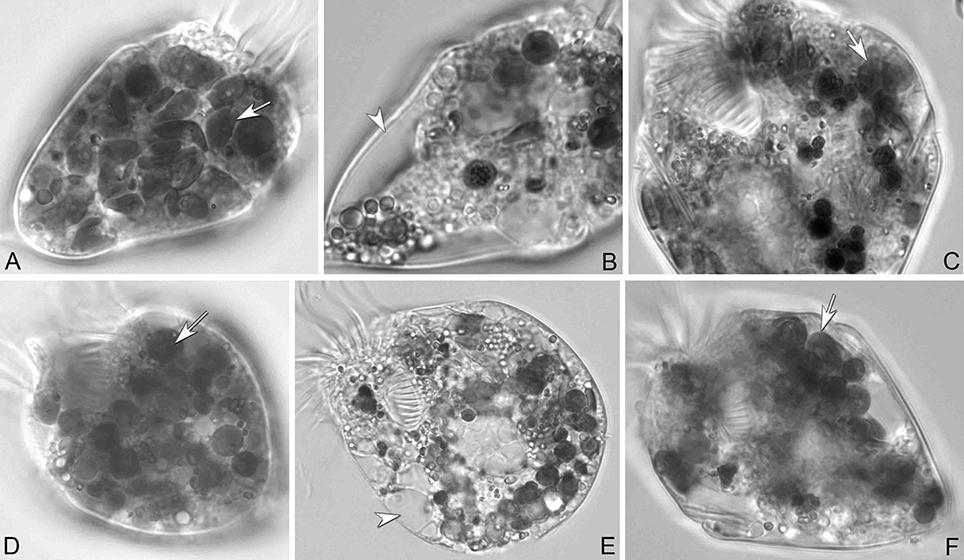
Figure 7. S. rassoulzadegani from cultures grown on T. chui PLY429 at 12:12 L:D (A–C) or 24 D (D–F). (A,D) Cells with adequate algae food (arrows). (B,E) Cells starved for 72 h; arrowheads mark empty vacuoles. (C,F) Algae were taken up again (arrows) after new algal food was added. Even in complete darkness (F), fed cells retain some chloroplasts.
When fed with fresh PLY 429 every day at saturating concentrations, S. rassoulzadegani could achieve growth rates 55–78% as great as those in the light (Figure 8). In five short-term experiments (5–8 day), growth on PLY 429 in the light (12:12 L:D at c. 70 μmol m−2 s−1) ranged from 0.75 to 1.19 d−1; that in the dark ranged from 0.58 to 0.82 d−1 (Figure 8).
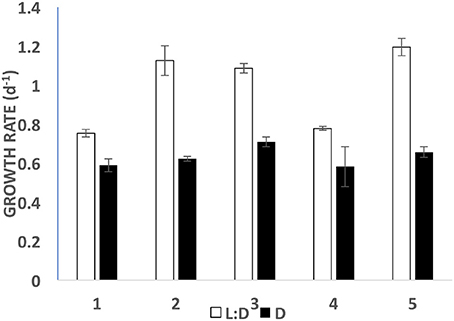
Figure 8. Growth rates in light (open bars; 12:12 photoperiod at c. 70 μmol m−2 s−1) vs. dark (filled bars) for five short term (5–8 day) experiments. Error bars are standard deviations (n = 3).
Ciliates fed any of the four food algae that supported net positive growth in the 6 day experiments (PLY 429, RHODO, ISO SP, or JA) survived longer in the light than the dark when subsequently starved, with the exception of JA, where survival was nearly identical in light and dark (Figure 9). Live observation on ciliates fed PLY 429 showed that the chloroplasts declined in size and number when cell were starved under both 12:12 L:D and 24 D (Figures 7B,E). After algal food was added to starved cultures, new chloroplasts were quickly retained as with non-starved cultures (Figures 7C,F).
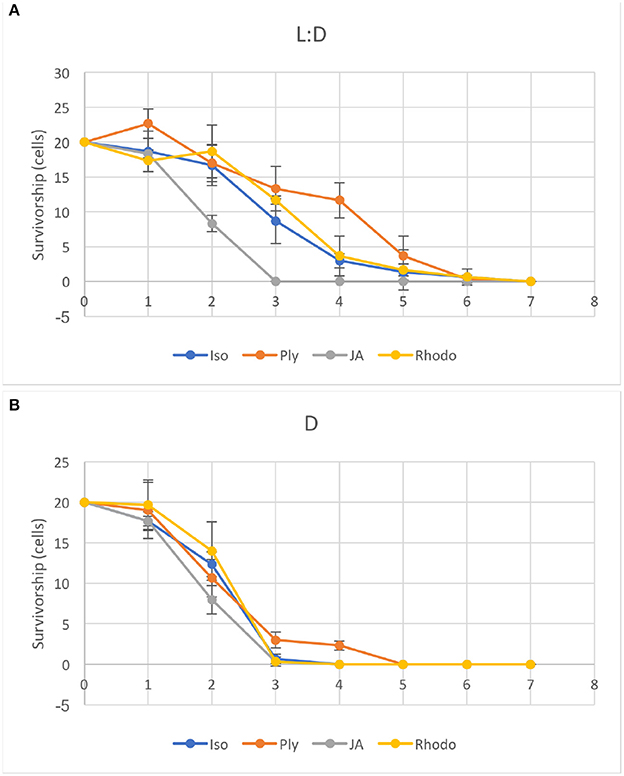
Figure 9. Ciliates were starved under light (A, 12:12 L:D photoperiod at c. 70 μmol m−2s−1) or dark (B) conditions after growth on four different food algae (see Table 1 for algal abbreviations). Error bars are standard deviations (n = 3).
Discussion
We have found S. rassoulzadegani by its rDNA signature nearly everywhere we have looked for it, including shore samples from Greenland, Iceland, Ireland, Scotland, Norway, the east and west coasts of North and South America, Hawaii, Japan, the Philippines, and western Australia. In northwest Atlantic tide pools, it is detectable almost all year, except for winter. Its apparent disappearance in winter may be related to the encystment pattern we observed, which is related to seasonal changes in light and temperature, if the cysts are in the benthos or otherwise unrepresented in our DNA samples from the tide pools. Even when it is low in abundance, the ciliate can easily be concentrated by placing a sample in a darkened volumetric flask and placing a light near the top of the neck, where the ciliates will aggregate by phototaxis (cf. Faure-Fremiet, 1948 for S. oculatum). Thus, this ciliate should be available for study almost anywhere in the world and could become a model for mixotrophs, and ciliates in general, in aquaculture or ecological studies.
Although we often observed cysts in culture, we never observed the full processes of encystment or excystment. The cysts are clear enough to allow observation of the developing trophic cells tumbling about inside (Figure 2), and we have seen the frothy plug being pushed out, but were not able to document emergence. Likewise, although we could cause relatively new cysts (weeks old) to hatch in response to flushing with fresh growth medium, older cysts stored >6 months in darkness at 4°C could not be induced to excyst. Thus, we have not developed any methods for longer-term storage of the resting stage, and we do not know how long the cysts may remain viable in nature. The only treatment we found that successfully stimulated encystment was simultaneous stepdown in temperature and light to levels the ciliates would experience during Autumn at mid-latitudes. This suggests that encystment is a seasonal response in this ciliate. Although our time series samples were collected from surface water in the tide pools, whereas cysts would be expected to be at the bottom or attached to macroalgae or other substrates (cf. Montagnes et al., 2002b), we cannot rule out that some of the positive results for presence of S. rassoulzadegani may have been due to cysts, especially in winter.
The apparent loss of the encystment habit in older cultures is interesting. It may be that long-term cultivation in abundant food and light at constant temperature places the mechanism for encystment in an inactive state. Since most of our cultures have been started from several individuals, we cannot rule out that selection for rapidly-growing non-encysting cell lines during culture transfers is responsible for the loss of the encystment habit. Further experimental work or transcriptomic studies on encysting/excysting cells may shed light on this in the future.
Between the present and previous work (McManus et al., 2012), we have tested 15 different strains of microalgae as food for S. rassoulzadegani. Although results have sometimes been inconsistent, a few general conclusions can be made. First, the best food giving the most consistent positive growth is Tetraselmis chui (PLY 429). In the light, exponential growth on PLY 429 was consistently about 1 d−1 (doubling time of 0.7 day; Figure 8). Growth in the light on Rhodomonas lens (RHODO) was about the same, but care had to be taken not to overfeed with RHODO, whereas the ciliates still grew well even at PLY 429 concentrations well above 105 cells ml−1. Poor growth at high algal concentrations is likely due to the accumulation of metabolites or other changes in the medium (e.g., pH; Pedersen and Hansen, 2003) from the microalgae. Growth was never successful with diatoms as the diet, while some dinoflagellates supported growth and others did not. Two haptophytes, Isochrysis galbana (TISO) and an Isochrysis sp. (ISO SP) that is 99% similar to TISO by SSU sequence but notably different in pigmentation and some culture habits, were able to support growth if cell concentrations were carefully controlled (e.g., the failure of TISO to support growth in the light in our experiments was probably due to overgrowth of the algae in the light treatment). To date, we have found no green alga besides PLY 429 that supports growth. It should be noted that absence of growth on a given food is not proof that growth is not possible, as slight changes in culture conditions can lead to success in some cases. Also, combinations of different unsuitable foods may themselves be suitable, though we have not found a mixed food that supports growth without one of its components being PLY 429 (cf. McManus et al., 2012).
We previously succeeded in achieving positive growth rates in light and dark for several weeks on the dinoflagellate Prorocentrum minimum (EXUV; McManus et al., 2012). In the present study, EXUV did not support growth in light or dark, whereas a different strain of P.minimum, JA, only supported growth in the light, including long-term growth (5 weeks). In darkness or light, cultures eventually died out when feeding on P. minimum (both strains). Given the vagaries of establishing and maintaining ciliate cultures, we think the only supportable conclusion is that heterotrophic growth in S. rassoulzadegani is possible on a variety of foods, but difficult to maintain due to possible overgrowth or food quality issues with the algae.
In contrast to earlier results, in the present study we were able to sustain growth in darkness on T. chui (PLY 429) by adding fresh algae several times per week. We observed that in dark-grown ciliate cultures, these small flagellates became less motile, possibly also declining in food quality. Prey properties including swimming motility and nutritional quality are regarded as having important effects on grazing selectivity of ciliates (Chen et al., 2010; Yang et al., 2015). Thus, previous failure of cultures to grow in darkness may have been due to food issues rather than the metabolism of the ciliate itself.
When ciliates are grown in the dark, chloroplasts are still retained from food, though they are distinctly smaller and seem darker than when they are grown in the light. Chloroplasts decline in size and number but are still present when ciliates are starved as well, suggesting that there is some process whereby chloroplasts are partly or wholly “immunized” from digestion by the ciliate. It is still not known whether plastids are egested from the ciliate when no longer functional or digested by them. As they appear to be free in the cytoplasm of the ciliate, it would require autophagous vacuoles to digest them. The latter are not seen in mixotrophic ciliates (Laval-Peuto and Febvre, 1986), so it may be difficult for the ciliates to eliminate the plastids and this may impose a cost on growth in the dark. Others have speculated that space taken up by retained chloroplasts imposes a “cost” on digestion and hence growth in the dark (Raven, 1997), but we found that growth in the dark, while significantly slower than in the light, was still in the range of 0.5–0.6 d−1, so the cost may not be as great as previously thought (Figure 8).
The only available evidence on functioning of chloroplasts in S. rassoulzadegani comes from 14CO2 uptake observations. Schoener and McManus (2017) showed net incorporation of 14C into organic matter in the light, and found that this gave this mixotrophic ciliate a growth efficiency advantage in comparison to a strict heterotroph, primarily at growth-limiting food concentrations. Our light vs. dark starvation experiment showed that survival was enhanced in the light for three of the four microalgae (ISO SP, PLY 429, and RHODO) that were capable of supporting short-term growth in the dark. This suggests that retained chloroplasts from ISO SP and RHODO remained functional as well. Only the dinoflagellate Prorocentrum minimum (JA) had equivalent survival in light and dark, supporting earlier conjectures that growth by S. rassoulzadegani on dinoflagellates is wholly heterotrophic. Possibly, digestion of the cellulosic cell wall of these armored dinoflagellates makes it difficult preserve and retain the chloroplasts, or dinoflagellate chloroplasts may not have a sufficient genome to function independently inside the ciliate.
The new observations on culture conditions and food preferences from this study raise questions about the nature of mixotrophy in S. rassoulzadegani. Although they always grow best in light, it is now clearly established that they can grow wholly heterotrophically in the dark over at least several months. We also found that multiple algal species can support mixotrophic growth in this species, though the green Tetraselmis chui was clearly the best food. The ability to grow on diverse foods and potentially to use multiple different chloroplasts for mixotrophy indicates a level of nutritional plasticity that may contribute to their widespread distribution in the ocean. Future work on how food quality affects mixotrophic metabolism should shed more light on this.
Given that plastids are retained from food even in darkness and that they endure within the cells when starved, it is important to know how photosynthetic performance varies over time and whether the ciliate exerts any genetic control over the plastids. Host nucleus-encoded genes that target retained chloroplasts have been found in Dinophysis acuminata, a mixotrophic dinoflagellate that uses plastids retained from the ciliate Mesodinium rubrum, which itself retains plastids from cryptophyte algae (Wisecaver and Hackett, 2010). We have not found similar transcripts in S. rassoulzadegani so far (Santoferrara et al., 2014), but new methods in transcriptomics are making an experimental approach to mixotrophy more tractable. In the remarkable mixotrophic ciliate Mesodinium rubrum, both nuclei and plastids of prey are retained (Johnson et al., 2007) and trancriptomics has been used to elucidate how the ciliate/prey chimera functions as an integrated organism (Lasek-Nesselquist et al., 2015; Kim et al., 2016).
Conclusion
S. rassoulzadegani is a widely-distributed, easily cultivable mixotrophic oligotrich ciliate. It should be isolated from more regions of the world and used as a model for mixotrophs, and oligotrichs in general, in ecological, aquaculture, and physiological studies. In particular, its ability to grow on a variety of foods and to survive under light and dark conditions raises the possibility that it can provide insight into the costs and benefits of kleptoplastic mixotrophy in marine ciliates.
Author Contributions
All authors listed have made a substantial, direct and intellectual contribution to the work, and approved it for publication.
Conflict of Interest Statement
The authors declare that the research was conducted in the absence of any commercial or financial relationships that could be construed as a potential conflict of interest.
The handling Editor declared a past co-authorship with one of the authors, GM.
Acknowledgments
We thank Gary Wikfors (NOAA National Marine Fisheries Service Laboratory, Milford CT) for algal cultures; Laura Katz (Smith College) for help with sequencing and primer design; Wen Song, Susan Smith, and Luciana Santoferrara for help with experiments; and Paul Renaud, Leo Blanco-Bernal, Barbara Costas, Andrew Payson, and Joseph Eslao for sample collection. We thank Sueann Mentone, Department of Cellular and Molecular Physiology, Yale School of Medicine for technical help with the electron microscopy. Support was provided by the US National Science Foundation (grant OCE 1435515), the Natural Science Foundation of China (No. 41576124), Shoals Marine Lab, and the University of Connecticut.
Supplementary Material
The Supplementary Material for this article can be found online at: https://www.frontiersin.org/articles/10.3389/fmars.2018.00205/full#supplementary-material
References
Blackbourn, D. J., Taylor, F. J. R., and Blackbourn, J. (1973). Foreign organelle retention by ciliates. J. Protozool. 20, 286–288. doi: 10.1111/j.1550-7408.1973.tb00877.x
Chen, B., Liu, H., and Lau, M. T. S. (2010). Grazing and growth responses of a marine oligotrichous ciliate fed with two nanoplankton: does food quality matter for micrograzers? Aquatic Ecol. 44, 113–119. doi: 10.1007/s10452-009-9264-5
Faure-Fremiet, E. (1948). Le rythme de maree du S. oculatum Gruber. Bull. Biol. France Belgique 82, 3–23.
Flynn, K. J., and Mitra, A. (2009). Building the “perfect beast”: modelling mixotrophic plankton. J. Plankton Res. 31, 965–992. doi: 10.1093/plankt/fbp044
Flynn, K. J., Stoecker, D. K., Mitra, A., Raven, J. A., Glibert, P. M., Hansen, P. J., et al. (2013). Misuse of the phytoplankton–zooplankton dichotomy: the need to assign organisms as mixotrophs within plankton functional types. J. Plankton Res. 35, 3–11. doi: 10.1093/plankt/fbs062
Guillard, R. R., and Ryther, J. H. (1962). Studies of marine planktonic diatoms. I. Cyclotella nana Hustedt, and Detonula confervacea (cleve). Gran. Can. J. Microbiol. 8, 229–239. doi: 10.1139/m62-029
Johnson, M. D. (2011). Acquired phototrophy in ciliates: a review of cellular interactions and structural adaptations. J. Eukaryot. Microbiol. 58, 185–195. doi: 10.1111/j.1550-7408.2011.00545.x
Johnson, M. D., Oldach, D., Delwiche, C. F., and Stoecker, D. K. (2007). Retention of transcriptionally active cryptophyte nuclei by the ciliate Myrionecta rubra. Nature 445, 426–428. doi: 10.1038/nature05496
Jonsson, P. R. (1994). Tidal rhythm of cyst formation in the rock pool ciliate Strombidium-oculatum Gruber (Ciliophora, Oligotrichida) - a description of the functional biology and an analysis of the tidal synchronization of encystment. J. Exp. Mar. Biol. Ecol. 175, 77–103. doi: 10.1016/0022-0981(94)90177-5
Katz, L. A., McManus, G. B., Snoeyenbos-West, O. L. O., Griffin, A., Pirog, K., Costas, B., et al. (2005). Reframing the “Everything is everywhere” debate: evidence for high gene flow and diversity in ciliate morphospecies. Aquat. Microbial Ecol. 41, 55–65. doi: 10.3354/ame041055
Kim, G. H., Han, J. H., Kim, B., Han, J. W., Nam, S. W., Shin, W., et al. (2016). Cryptophyte gene regulation in the kleptoplastidic, karyokleptic ciliate Mesodinium rubrum. Harmful Algae 52, 23–33. doi: 10.1016/j.hal.2015.12.004
Lasek-Nesselquist, E., Wisecaver, J. H., Hackett, J. D., and Johnson, M. D. (2015). Insights into transcriptional changes that accompany organelle sequestration from the stolen nucleus of Mesodinium rubrum. BMC Genomics 16:805. doi: 10.1186/s12864-015-2052-9
Laval-Peuto, M., and Febvre, M. (1986). On plastid symbiosis in Tontonia appendiculariformis (Ciliophora, Oligotrichina). Biosystems 19, 137–158. doi: 10.1016/0303-2647(86)90026-2
Laval-Peuto, M., Salvano, P., Gayol, P., and Greuet, C. (1986). Mixotrophy in marine planktonic ciliates: ultrastructural study of Tontonia appendiculariformis (Ciliophora, Oligotrichina). Mar. Microb. Food Webs. 1, 81–104.
McManus, G. B., and Fuhrman, J. A. (1986). Photosynthetic pigments in the ciliate Laboea strobila from Long Island Sound, USA. J. Plankton Res. 8, 317–327. doi: 10.1093/plankt/8.2.317
McManus, G. B., Schoener, D. M., and Haberlandt, K. (2012). Chloroplast symbiosis in a marine ciliate: ecophysiology and the risks and rewards of hosting foreign organelles. Front. Microbiol. 3:321. doi: 10.3389/fmicb.2012.00321
McManus, G. B., Xu, D., Costas, B. A., and Katz, L. A. (2010). Genetic identities of cryptic species in the Strombidium stylifer/apolatum/oculatum cluster, including a description of Strombidium rassoulzadegani n. sp. J. Euk. Microbiol. 57, 369–378. doi: 10.1111/j.1550-7408.2010.00485.x
McManus, G. B., Zhang, H., and Lin, S. (2004). Marine planktonic ciliates that prey on macroalgae and enslave their chloroplasts. Limnol. Oceanogr. 49, 308–313. doi: 10.4319/lo.2004.49.1.0308
Medlin, L., Elwood, H. J., Stickel, S., and Sogin, M. L. (1988). The characterization of enzymatically amplified eukaryotic 16S-like rRNA-coding regions. Gene 71, 491–499. doi: 10.1016/0378-1119(88)90066-2
Mitra, A., Flynn, K. J., Tillmann, U., Raven, J. A., Caron, D., Stoecker, D. K., et al. (2016). Defining planktonic protist functional groups on mechanisms for energy and nutrient acquisition: incorporation of diverse mixotrophic strategies. Protist 167, 106–120. doi: 10.1016/j.protis.2016.01.003
Montagnes, D. J. S., Berger, J. D., and Taylor, F. J. R. (1996). Growth rate of the marine planktonic ciliate Strombidinopsis cheshiri Snyder and Ohman as a function of food concentration and interclonal variability. J. Exp. Mar. Biol. Ecol. 206, 121–132. doi: 10.1016/S0022-0981(96)02626-3
Montagnes, D. J., Lowe, C. D., Poultonb, A., and Jonsson, P. R. (2002a). Redescription of Strombidium oculatum Gruber 1884 (Ciliophora, Oligotrichia). J. Eukaryot. Microbiol. 49, 329–337. doi: 10.1111/j.1550-7408.2002.tb00379.x
Montagnes, D. J. S., Wilson, D., Brooks, S. J., Lowe, C., and Campey, M. (2002b). Cyclical behaviour of the tide-pool ciliate Strombidium oculatum. Aquat. Microbial. Ecol. 28, 55–68. doi: 10.3354/ame028055
Pedersen, M. F., and Hansen, P. J. (2003). Effects of high pH on the growth and survival of six marine heterotrophic protists. Mar. Ecol. Prog. Ser. 260, 33–41. doi: 10.3354/meps260033
Putt, M. (1990). Abundance, chlorophyll content and photosynthetic rates of ciliates inthe Nordic Seas during summer. Deep Sea Res. 37, 1713–1731. doi: 10.1016/0198-0149(90)90073-5
Raven, J. A. (1997). Phagotrophy in phototrophs. Limnol. Oceanogr. 42, 198–205. doi: 10.4319/lo.1997.42.1.0198
Santoferrara, L. F., Guida, S., Zhang, H., and McManus, G. B. (2014). De Novo transcriptomes of a mixotrophic and a heterotrophic ciliate from marine plankton. PLoS ONE 9:e101418. doi: 10.1371/journal.pone.0101418
Schoener, D. M., and McManus, G. B. (2012). Plastid retention, use, and replacement in a kleptoplastidic ciliate. Aquat. Microb. Ecol. 67, 177–187. doi: 10.3354/ame01601
Schoener, D. M., and McManus, G. B. (2017). Growth, grazing, and inorganic C and N uptake in a mixotrophic and a heterotrophic ciliate. J. Plankton Res. 39, 379–391. doi: 10.1093/plankt/fbx014
Stanton, B. A., Biemesderfer, D., Wade, J. B., and Giebisch, G. (1981). Structural and functional study of the rat distal nephron: effects of potassium adaptation and depletion. Kidney Int. 19, 36–48. doi: 10.1038/ki.1981.5
Stoecker, D. K. (1998). Conceptual models of mixotrophy in planktonic protists and some ecological and evolutionary implications. Eur. J. Protistol. 34, 281–290. doi: 10.1016/S0932-4739(98)80055-2
Stoecker, D. K., and Silver, M. W. (1987). Chloroplast retention by marine planktonic ciliates. Ann. N. Y. Acad. Sci. 503, 562–565. doi: 10.1111/j.1749-6632.1987.tb40646.x
Stoecker, D. K., Johnson, M. D., de Vargas, C., and Not, F. (2009). Acquired phototrophy in aquatic protists. Aquat. Microbial Ecol. 57, 279–310. doi: 10.3354/ame01340
Stoecker, D. K., Michaels, A. E., and Davis, L. H. (1987). Large proportion of marine planktonic ciliates found to contain functional chloroplasts. Nature 326, 790–792. doi: 10.1038/326790a0
Wisecaver, J. H., and Hackett, J. D. (2010). Transcriptome analysis reveals nuclear-encoded proteins for the maintenance of temporary plastids in the dinoflagellate Dinophysis acuminata. BMC Genomics 11:366. doi: 10.1186/1471-2164-11-366
Keywords: mixotrophy, kleptoplasty, oligotrich, encystment, chloroplast
Citation: McManus GB, Liu W, Cole RA, Biemesderfer D and Mydosh JL (2018) Strombidium rassoulzadegani: A Model Species for Chloroplast Retention in Oligotrich Ciliates. Front. Mar. Sci. 5:205. doi: 10.3389/fmars.2018.00205
Received: 28 March 2018; Accepted: 25 May 2018;
Published: 12 June 2018.
Edited by:
Matthew D. Johnson, Woods Hole Oceanographic Institution, United StatesReviewed by:
Sai Elangovan S, National Institute of Oceanography (CSIR), IndiaMiran Kim, Chonnam National University, South Korea
Copyright © 2018 McManus, Liu, Cole, Biemesderfer and Mydosh. This is an open-access article distributed under the terms of the Creative Commons Attribution License (CC BY). The use, distribution or reproduction in other forums is permitted, provided the original author(s) and the copyright owner are credited and that the original publication in this journal is cited, in accordance with accepted academic practice. No use, distribution or reproduction is permitted which does not comply with these terms.
*Correspondence: George B. McManus, Z2VvcmdlLm1jbWFudXNAdWNvbm4uZWR1