- 1National Institute of Water and Atmospheric Research, Wellington, New Zealand
- 2National Institute of Water and Atmospheric Research, Hamilton, New Zealand
- 3Institute of Marine Science, University of Auckland, Auckland, New Zealand
- 4Tvärminne Zoological Station, University of Helsinki, Helsinki, Finland
- 5Baltic Sea Centre, Stockholm University, Stockholm, Sweden
Understanding the functionality of marine benthic ecosystems, and how they are influenced by their physical environment, is fundamental to realistically predicting effects of future environmental change. The Antarctic faces multiple environmental pressures associated with greenhouse gas emissions, emphasizing the need for baseline information on biodiversity and the bio-physical processes that influence biodiversity. We describe a survey of shallow water benthic communities at eight Ross Sea locations with a range of environmental characteristics. Our analyses link coastal benthic community composition to seafloor habitat and sedimentary parameters and broader scale features, at locations encompassing considerable spatial extent and variation in environmental characteristics (e.g., seafloor habitat, sea ice conditions, hydrodynamic regime, light). Our aims were to: (i) document existing benthic communities, habitats and environmental conditions against which to assess future change, (ii) investigate the relationships between environmental and habitat characteristics and benthic community structure and function, and (iii) determine whether these relationships were dependent on spatial extent. A very high percentage (>95%) of the between-location variability in macro- or epifaunal community composition was explainable using multi-scale environmental variables. The explanatory power varied depending on the scale of influence of the environmental variables measured (fine and medium-scale habitat, broad scale), and with community type. However, the inclusion of parameters at all scales produced the most powerful model for both communities. Ice duration, ice thickness and snow cover were important broad scale variables identified that directly relate to climate change. Even when using only habitat-scale variables, extending the spatial scale of the study from three locations covering 32 km to eight locations covering ~340 km increased the degree of explanatory power from 18–32 to 64–78%. The increase in explanatory power with spatial extent lends weight to the possibility of using an indirect “space for time” substitution approach for future predictions of the effects of change on these coastal marine ecosystems. Given the multiple and interacting drivers of change in Antarctic coastal ecosystems a multidisciplinary, long term, repeated observation approach will be vital to both improve and test predictions of how coastal communities will respond to environmental change.
Introduction
Seafloor community composition is the product of numerous biological and physical processes acting across multiple spatial and temporal scales (e.g., Ricklefs, 1987; Kolasa and Pickett, 1991). Such communities act as sentinels of change, integrating many processes occurring in the surrounding ocean. Understanding the complex functionality of benthic ecosystems, and how they are influenced by their physical environment, is fundamental to realistically predicting future effects of environmental change. Key to these predictions are the need for baseline information on biodiversity and the role of bio-physical processes in shaping existing marine ecosystems, as well as predictions of change at appropriate space and time scales (Dayton and Tegner, 1984; Wiens, 1989).
Antarctic marine environments are amid dramatic change, with significant air and ocean warming already observed (Meredith and King, 2005; Martinson et al., 2008; Turner et al., 2009; IPCC, 2013) and meta-analyses of multiple global climate models predicting that several more degrees of warming could occur in Antarctica over the coming decades (Walsh, 2009; Mayewski et al., 2015). Warming sea temperatures can, as well as directly affecting fauna (Peck et al., 2004; Peck, 2011), combine with changing wind patterns and oceanographic conditions to manifest further impacts through altered sea ice dynamics (e.g., Ducklow et al., 2013). The importance of changes in sea ice extent and persistence have been well highlighted, particularly because changes are anticipated across large areas of the Southern Ocean (Stammerjohn et al., 2008; Gutt et al., 2015, 2016). To date, sea temperatures have increased in the Antarctic Circumpolar Current, both at the surface and at depth (e.g., Aoki et al., 2003; Boning et al., 2008). The Western Antarctic Peninsula (WAP) and Potters Cove have exhibited the greatest increases in sea temperature, by ~1°C since 1950 (Meredith and King, 2005; Schloss et al., 2012). The changes in sea ice dynamics that have accompanied warming in the WAP region have cascaded through trophic levels to affect a range of organisms (Clarke et al., 2007; Ducklow et al., 2007; Grange and Smith, 2013). Although such changes have not been detected in the Ross Sea region of Antarctica, variations in sea ice characteristics and productivity (multiyear, annual, polynya influence) are known to have a major influence on marine communities in this area (e.g., Dayton and Oliver, 1977; Thrush et al., 2006; Norkko et al., 2007; Smith et al., 2012a,b).
A recent review, conducted by Convey et al. (2014), found that ice cover, ice scour, salinity and productivity were the most important physical and biological drivers of Antarctic biodiversity, and that there was not one key factor acting Antarctic-wide. Ice-shelf collapse and iceberg calving have been shown to affect multiple ecosystem components through interruptions to “usual” sea ice patterns and productivity (e.g., Arrigo et al., 2002, Ainley et al., 2006; Kooyman et al., 2007; Siniff et al., 2008; Gutt et al., 2011, 2013; Thrush and Cummings, 2011). Considerable environmental change is expected in Antarctic marine ecosystems in the coming decades (e.g., Stammerjohn et al., 2008, 2012; Jacobs and Giulivi, 2010; Smith et al., 2014), including changes in ocean chemistry (Orr et al., 2005; McNeil and Matear, 2008). The complexity of the direct and indirect influences of this potential change on marine communities cannot be understated (see Gutt et al., 2015). We are not currently in a good position to understand how these unique ecosystems will respond.
Nearshore communities, important to higher trophic levels (Grange and Smith, 2013) and often rich in primary production, are at the boundary between terrestrial and open ocean systems. Significant inputs of water (freshening), sediments, and associated minerals are observed in these areas due to their proximity to ice shelves and glaciers and the associated runoff with melt (Dierssen et al., 2002; Moline et al., 2008; Grange and Smith, 2013; Pasotti et al., 2015; Dayton et al., 2016; Monien et al., 2017). Consequently, impacts of warming could be expected to be greater in the coastal regions, at least in the short term (e.g., Gutt et al., 2015). For this reason, a good understanding of the environmental drivers of patterns in benthic community composition, and the potential resilience of these coastal communities is important.
In this study, we focus on shallow water benthic communities in the coastal Ross Sea. Our analyses link coastal benthic community composition to seafloor habitat and sedimentary parameters and broader scale environmental characteristics, at eight locations along the Ross Sea coastline (Figure 1). This spatially extends a study by Cummings et al. (2006) to encompass greater variation in environmental characteristics (e.g., seafloor habitat, sea ice conditions, hydrodynamic regime, light climate). Some of these characteristics can be considered aligned along a natural gradient of factors that will influence benthic community structure and function, and which are anticipated to change in future (e.g., sea ice persistence).
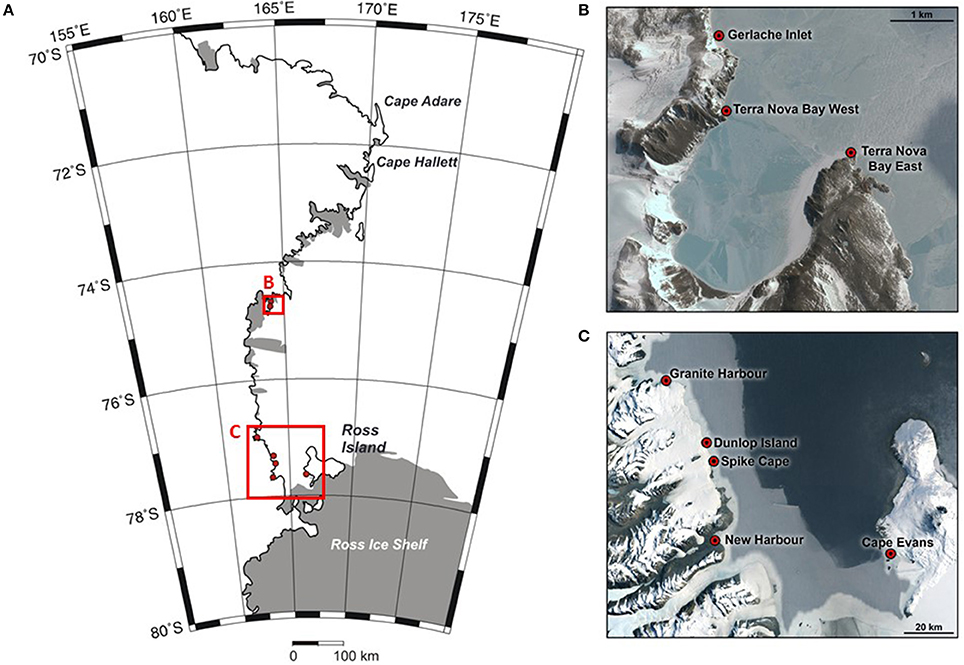
Figure 1. Map of the Ross Sea region (A), showing the sampling locations at Terra Nova Bay (B), and the southern Ross Sea (C). Three sites were sampled at each location. Credits for inset map data: (B) Google Earth, Digital Globe; (C) Google, LandSat/Copernicus.
Our aims were three-fold: (i) to document existing benthic communities, habitats and environmental conditions against which to assess future change, (ii) to investigate the relationships between environmental and habitat characteristics and benthic community structure and function, and (iii) to determine whether the conclusions of Cummings et al. (2006) hold as the spatial and environmental extent of the data increases and whether explanatory power also increases. If the same characteristics are found to be important and if the explanatory power remains the same or increases then it is likely that natural gradients can be used as an indirect space for time (e.g., Pickett, 1989; Fukami and Wardle, 2005) substitution that might help predict the effects of their changes on these coastal marine ecosystems in the coming decades.
Materials and Methods
Eight coastal Ross Sea locations were chosen, spanning ~340 km of coastline (Figure 1) and considerable variability in seafloor habitat and sea ice conditions. At each location (Cape Evans, New Harbor, Dunlop Island, Spike Cape, Granite Harbor, Tethys Bay west, Tethys Bay south and Gerlache Inlet), three sites, separated by at least 50 m, were chosen within the 15–25 m depth range (Table 1). These locations were sampled from 2001 to 2007, in October/November (except for Tethys Bay west, which was sampled in January; see Table 1 for exact dates). While two of these locations were situated in Tethys Bay, they were ~1.6 km apart, and the ice persists for approximately 1 month longer each season at Tethys Bay west than at Tethys Bay south (Table 1). Each site was accessed through a hole drilled in the sea ice and sampling was conducted by SCUBA divers.
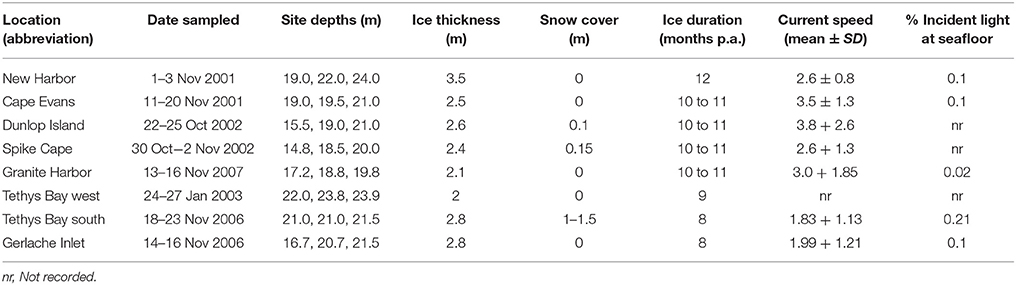
Table 1. Depths, dates of sampling, and broad scale environmental characteristics at the eight coastal Ross Sea locations.
The methodologies used for sample collection and processing, and their rationale, are provided in detail in Cummings et al. (2006), along with our analysis of the three southernmost locations (New Harbor, Dunlop Island, Spike Cape; spanning ~32 km of coastline). These methods are briefly reiterated below.
Transect Based, Nested Sampling
Benthic macro-infaunal communities (hereafter “macrofauna”), epifaunal communities, and fine and medium scale habitat features, were quantified at each site using a nested sampling design. At each site, a 20 m long transect line was laid on the seafloor oriented parallel to the shoreline. Five marker pegs were inserted into the sediment at random points along the length of the transect, which was then videoed by SCUBA divers at fixed heights of 70 and 40 cm above the seafloor. These two heights enabled us to categorize habitat features of different densities between sites, and the information was later scaled to numbers or percent cover m2 (Cummings et al., 2006). Epifaunal taxa and habitat features were quantified from 1.5 m lengths of video frame grabs, each centerd on the location of a sediment core collected to quantify macrofauna (Cummings et al., 2006). Five medium-scale habitat features were quantified from the video frame grabs: % cover of sand, rock, pebble, cobble, and coarse sediments with detritus (Cummings et al., 2006).
Sediment cores were collected adjacent to each marker, to quantify macrofaunal communities (70 mm diameter, 100 mm deep core), and fine-scale sediment characteristics (26 mm diameter, 50 mm deep core). The large core samples were sieved (500 μm mesh), and then preserved in 70% isopropanol in seawater, before macrofauna were separated out, and identified to the lowest taxonomic level possible. Sediment from each small core was homogenized and sub-sampled for analysis of chlorophyll a (Chl a), phaeophytin, particle size and organic content. Chl a and phaeophytin were extracted from freeze dried sediments by boiling in 90% ethanol. The extract was measured spectrophotometrically, and an acidification step was included to separate degradation products (phaeophytin) from Chl a (Sartory, 1982). Sediment for particle size analysis was digested in 6% hydrogen peroxide for 48 h to remove organic matter, and dispersed using Calgon. A Galai particle analyser (Galai Cis−100; Galai Productions Ltd, Midgal Haemek, Israel) was then used to calculate percentage volumes for the coarse, medium and fine sand, silt and clay fractions. Organic content was determined by drying the sediment at 60°C for 48 h, followed by combustion at 400°C for 5.5 h.
Broad Scale Environmental Variables
At each location we collected information on current velocity, water temperature (°C), light transmission to the seafloor, the thickness of sea ice (m), and snow cover (m). Current velocities (cm s−1) and water temperatures were measured by an S4 or Anderra current meter deployed 4 m above the seabed for the duration of our visit (at least 3 days). Light transmission, measured as percentage incident light, was calculated from five replicate measurements of photosynthetically available radiation (PAR) made both above the sea ice and at the seafloor, using a LiCor Li190SA quantum sensor for incident irradiance (background irradiance above the ice) and a Li192SB for underwater irradiance attached to a Li-1000 logger. Ice cover duration (i.e., number of months per year that sea ice covered the site) was also estimated using personal observations and satellite imagery. Current, temperature and irradiance were not measured at Tethys Bay west.
Functional Traits of Macrofauna
To identify the relative predominance of macrofauna with particular functional characteristics, the taxon list was examined and traits assigned using a combination of literature information, advice from experts and our own observations. Three trait categories were characterized for each taxon: feeding traits—suspension feeders, deposit feeder-grazers or predator-scavengers; motility traits—sedentary, limited motility, freely motile; and living position—surface dwellers, or shallow (<2 cm) or deep (>2 cm) sediment dwellers. These traits were chosen for their potential influence on ecosystem functions, and the expectation that differences in traits may be closely linked to habitat (Hewitt et al., 2008). When a taxon exhibited more than one trait within a particular category, “fuzzy coding” was applied to reflect the relative importance of the multiple traits (sensu Chevenet et al., 1994). The fuzzy probability was then multiplied by the abundance of each taxon to provide an abundance weighted measure of each trait. Results are presented as relative proportions per trait within each category.
Statistical Analyses
Community Descriptions
Diversity indices [number of individuals, number of taxa, and species evenness (J′)] were calculated for both the epifauna and macrofauna using the DIVERSE procedure within PRIMER (Clarke and Gorley, 2001). To assess the relative variability in abundance of the three epifaunal taxa common across locations, the scallop Adamussium colbecki, the seastar Odontaster validus and the sea urchin Sterechinus neumayeri. Coefficients of variation (CoV; SD/mean) were calculated, using the average abundance values (m−2) for each of the three sites per location.
The relationship within and between locations for (a) macrofaunal community composition (determined from the core data), and (b) epifaunal community composition (determined from the video) were investigated using non-metric multidimensional scaling ordination (MDS; Clarke and Gorley, 2001) on untransformed data, and averages for each site. Patterns in macrofaunal community functional traits were also investigated using MDS. SIMPER (PRIMER Clarke, 1993), with a cutoff of 90%, was used to determine average similarities within locations, along with the taxa that characterized the communities at each location.
Linking Benthic Community Composition and Environmental Characteristics
The degree to which variations within and among locations in benthic community composition (macrofauna, epifauna) could be explained by broad scale environmental features and/or medium to fine-scale habitat features was investigated. The scales were assigned to the variables based on the spatial grain at which they were collected (Table 2). Habitat scale features were those specific to the habitat surrounding the macro- and/or epifauna. Those collected using small cores immediately adjacent to the macrofauna cores were considered to represent fine scale habitat structure pertaining to macrofauna; those collected using video along the 20 m transect represented the habitat loosely pertaining to epifauna. Our measures of fine- and medium-scale habitat features also encompassed variation at their respective scales through multiple measurements: (i) cores were taken at 5 random positions along each 20 m transect, (ii) medium scale habitat features were also assessed at 5 random positions, each of which encompassed a 1.5 m long section of transect. Factors such as currents, light, and ice conditions were considered broad scale because these were generally more consistent at the scale of the locations within which the transect and core data were collected. We note that some of these broad scale factors will vary within locations.
Broad scale environmental variables included in these analyses were ice thickness (m), ice cover duration (mo), snow cover (m), light transmission, current speed (minimum, maximum, average), maximum water temperature, and depth. Latitude and longitude were also included in this category. Habitat scale features included medium-scale data determined from the transect video (% cover m−2 sand, rock, pebble, cobble, coarse sediments with detritus), and the fine-scale information from the core samples [sediment particle size (% composition of gravel, medium sand, fine sand, mud), % organic content, and chlorophyll a, phaeophytin (μg g−1)].
We used Canonical Correspondence Analysis (CCA; ter Braak, 1986), on an untransformed average for each location and down weighting of rare species, with forward selection to link changes in benthic communities to local and broad-scale environmental drivers. The relationship between functional traits of macroinfauna and the multiscale suite of environmental characteristics was investigated using the same procedure.
Results
Communities
Epifauna
Numbers of epifaunal species per site ranged from 2 to 5 m−2, with abundances lowest at Spike Cape (Site 2, 10.0 ± 3.6 m−2) and highest at Cape Evans (Site 1, 219.8 ± 31.9 m−2) (Table 3). The extremely high numbers at the latter site are due mostly to the echinoderm Sterechinus neumayeri. Numbers of individuals were generally lowest at the southernmost locations, New Harbor, Spike Cape and Dunlop Island, and highest at Tethys Bay south (Table 3). Species evenness (J′) is generally moderate (values of 0.4–0.6; Table 3), although at a few sites, where only one or two species are found, J′ is consequently elevated (i.e., 1.63 at Tethys Bay west Site 3; and 0.8–1.0 at Gerlache Inlet, Dunlop Island Site 3, New Harbor Site 1 and Cape Evans Site 2; Table 3).
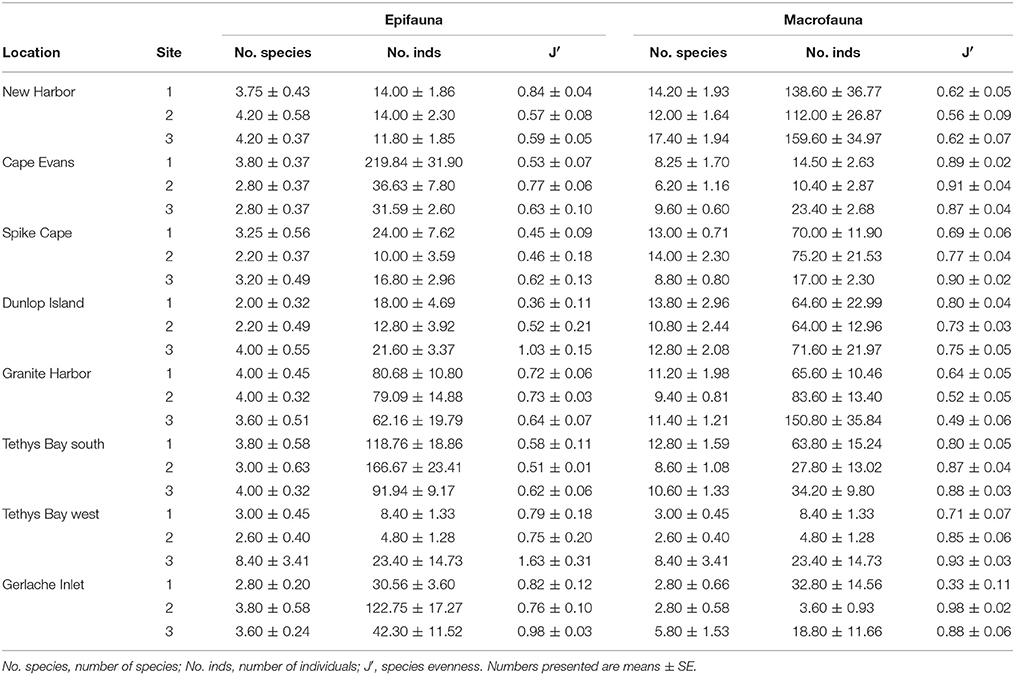
Table 3. Summary diversity indices at each site and location for the epifauna determined using video, and the macrofauna determined using cores.
Across locations, the epifaunal communities were dominated by one of five taxa: the echinoderms S. neumayeri, Odontaster validus, and Ophionotus victoriae, the bivalves Adamussium colbecki and Laternula elliptica, and the sponge Homaxinella balforensis; Table 4). At four locations (Cape Evans, Spike Cape, Tethys Bay south and Tethys Bay west) the first and second most abundant taxa were S. neumayeri and O. validus, respectively. Within location similarity was greatest at New Harbor and Tethys Bay south (~74%; Table 4). The MDS of epifaunal community composition shows generally clear separation and tight clustering in ordination space of sites within Dunlop Island, Spike Cape and New Harbor (Figure 2A). Community compositions at the Gerlache Inlet and Cape Evans sites are the most variable (Figure 2A).
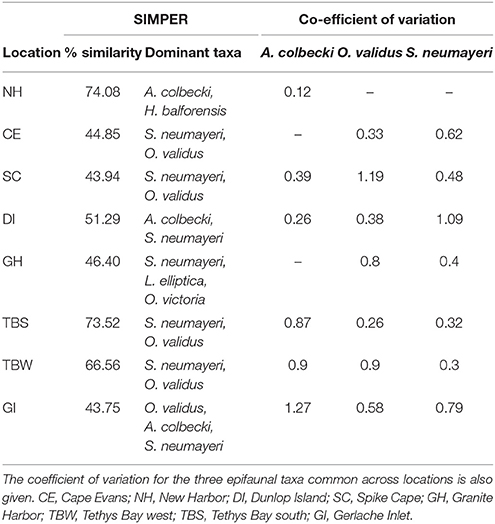
Table 4. The percentage similarity in epifaunal community composition at each location, and the dominant taxa contributing to 90% of the variability (from SIMPER analysis of mean abundance m−2 determined from video analysis).
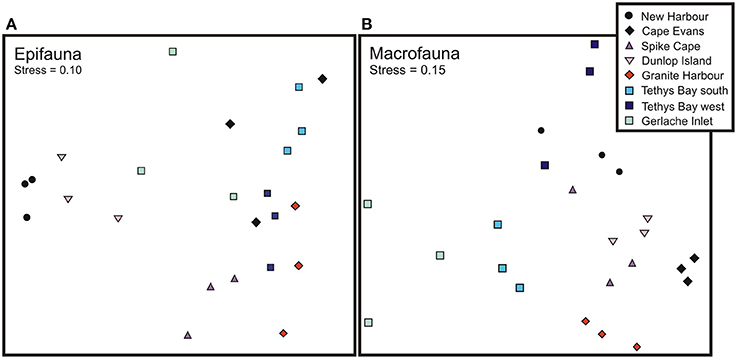
Figure 2. Multidimensional Scaling ordination plot of (A) epifaunal and (B) macrofaunal community composition.
The relative within-location variability in abundance of the three common epifaunal taxa, assessed using the CoV, indicated that A. colbecki was distributed in similar numbers across sites at New Harbor (0.12), but was considerably patchier at Gerlache Inlet (1.27) (Table 4). Distribution of O. validus was similar across sites at Cape Evans, Dunlop Island, Tethys Bay south (0.26–0.38), and most variable at Granite Harbor, Tethys Bay west and Spike Cape (0.8–1.19). S. neumayeri distributions were similar at Tethys Bay west, Tethys Bay south, Granite Harbor (0.3–0.4) and relatively patchy at Dunlop Island (1.09).
Macrofauna
Numbers of macrofauna species ranged from 2.6 ± 0.4 core−1 at Tethys Bay west Site 2, to 17.4 ± 1.94 core−1 at Cape Evans Site 3 (Table 3). Numbers of individuals were also variable, ranging from 3.6 ± 0.93 core−1 at Gerlache Inlet Site 2 to around 138.6 ± 36.8 core−1 at Cape Evans. Evenness was high (>0.6) at most sites (Table 3), indicating the dominance of 1–2 species. The exception is all three of the Gerlache Inlet sites, where evenness was relatively low (0.33–0.49; Table 3). Dominant taxa in the macrofaunal communities varied with location. The burrowing anemone Edwardsia sp. was common at New Harbor, Spike Cape, Dunlop Island and Granite Harbor, and the polychaete Polygordius antarcticus at five of the eight locations (Table 5). Polychaetes featured at all locations except Granite Harbor and Tethys Bay north, and one (Ophryotrocha ?notialis) was the dominant taxa at Gerlache Inlet (Table 5).
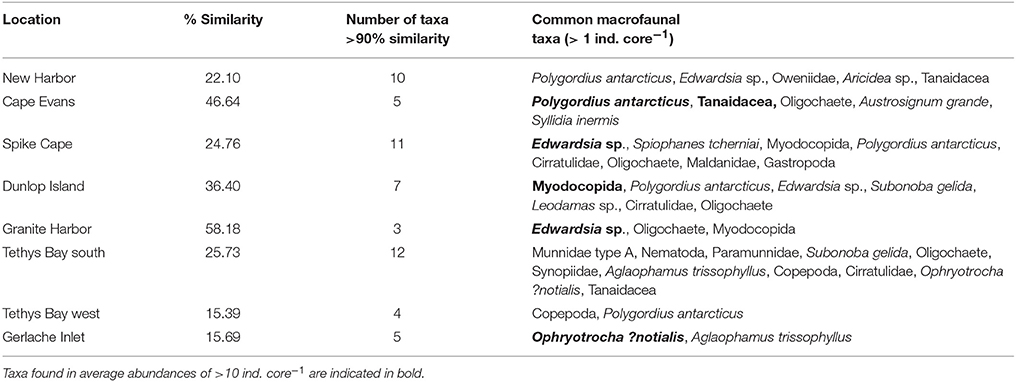
Table 5. The percentage average similarity in macrofaunal community composition at each location, the number of taxa contributing to 90% of the similarity, and the taxa found in average abundances of >1 ind. core−1.
The within location similarity was low compared to that of the epifauna, reaching a maximum at Cape Evans and Granite Harbor (47 and 58%, respectively), and a low of ~15% at Tethys Bay west and Gerlache Inlet (Table 5). The MDS of macrofaunal community composition shows generally clear distinctions in ordination space of sites within a location, with little overlap between locations (Figure 2B). The exceptions to this are New Harbor and Tethys Bay north, and Dunlop Island and Spike Cape (Figure 2B).
For all three of the functional characteristics investigated here (i.e., feeding type, motility, living position), the Granite Harbor macrofaunal communities were clearly distinguishable from those of all other sites (Supplementary Figure 1). Specifically, these communities were dominated by suspension feeders with limited mobility that dwell in the near-surface sediment layer (i.e., <2 cm; Supplementary Figure 1). The macrofaunal community traits at the three Terra Nova Bay locations (Tethys Bay west, Tethys Bay south, Gerlache Inlet) tended to be similar to one another, with mostly freely motile, surface dwelling predator/scavengers (Supplementary Figure 1). While the remaining (more southerly) locations showed variable trait characteristics, macrofauna at New Harbor, Cape Evans and Dunlop Island were most commonly freely motile deposit-grazers dwelling in the top 2 cm (Supplementary Figure 1).
Environmental Characteristics
The largest distinction between locations in broad scale environmental characteristics was in sea ice cover. Annual sea ice was present at all locations except New Harbor (where multi-year ice is the norm), but varies in the number of months it persists each year (Table 1), in thickness (range from 2.0 m at Tethys Bay west to 3.5 m at New Harbor at the time of sampling), and in the amount of snow cover on the ice (Table 1). Note that the latter measure of snow cover can also vary considerably from year to year at the same location (authors' pers. obs.). Currents measured at the sites at the time of sampling were fastest at Granite Harbor (3.0 ± 1.85 cm sec−1) and slowest at Tethys Bay south (1.83 ± 1.13 cm sec−1). At each location, <1% of the above ice incident light is transmitted to the seafloor below (Table 1).
The video transect-habitat analyses revealed simple habitat structure at all three of the New Harbor sites (100% sand) and the three Dunlop Island sites (from 77.33 ± 8.62 to 92.50 ± 1.89% sand; Supplementary Table 1), and the most complex at Granite Harbor and Gerlache Inlet (mixed habitat type; Supplementary Table 1). The proportion of the seafloor containing sediments available to be cored was low at several sites (i.e., coarse sediment/sand categories Supplementary Table 1). At this finer core-habitat scale, the soft sediments were comprised predominantly of coarse sand at Cape Evans (~80%; Supplementary Table 2), New Harbor (52–60%; Supplementary Table 2), Tethys Bay south (50–64%; Supplementary Table 2) and Tethys Bay west (62–75%; Supplementary Table 2). Dunlop Island sediments also contained a significant portion of coarse sand (38.26 ± 1.66 to 41.30 ± 5.20%), with considerable percentages of gravel, fine and medium sand. Granite Harbor sediments were more variable, with one site dominated by coarse sand (Site 1, 43.05 ± 6.05%), and Sites 2 and 3 with similar percentages of medium sand (40.20 ± 0.40 and 42.46 ± 1.18%, respectively) and equal amounts of coarse and fine sand (28.30 ± 2.17 and 27.12 ± 2.87%). The sediments at Spike Cape were of mixed particle sizes. Sediment organic content was very low at all locations, only exceeding 1% at Granite Harbor Site 1 (1.31 ± 0.48%). Sediment associated chlorophyll a was also highest at this Site (6.69 ± 2.77 μg g−1). Concentrations of phaeophytin were variable, ranging from 1.60 ± 0.36 μg g−1 at New Harbor Site 1, to 26.09 ± 9.66 μg g−1 at Tethys Bay west Site 3 (Supplementary Table 2).
Linking Benthic Community Composition and Environmental Characteristics
In the multi-scale CCA, six variables explained 97% of the variability in epifaunal community composition between the eight locations (Figure 3A). These include maximum current and longitude (aligned with CCA Axis 1), and ice thickness, % composition of silt, % cover of sand, and phaeophytin. The direction of the vectors for the latter two variables are aligned with the communities at New Harbor, Dunlop Island and Gerlache Inlet (% sand), and Spike Cape, Tethys Bay west, Tethys Bay south, and Cape Evans (phaeophytin) (Figure 3). Maximum current speed is the variable important in separating the Granite Harbor community from those at the remaining locations (Figure 3A).
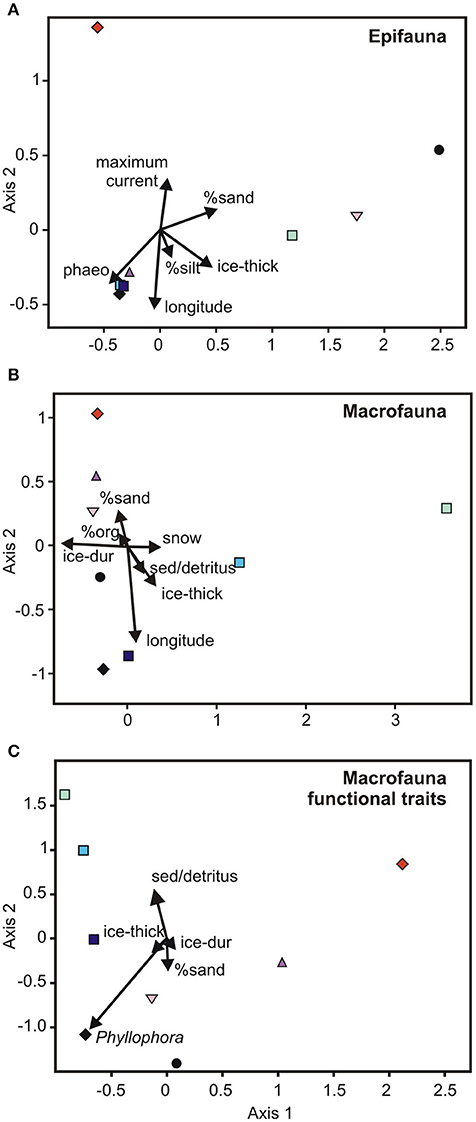
Figure 3. Canonical Correspondence Analysis ordinations of the relationship between habitat and broad scale environmental variables and (A) epifaunal and (B) macrofaunal community composition, and (C) macroinfaunal functional traits. The variation explained by the variables was 97, 95, and 97%, respectively. The locations are represented by different colored symbols as defined in Figure 2. Phaeo, phaeophytin; ice-thick, ice thickness; ice-dur, ice duration; sed/detritus, sediment associated detritus; org, organic content.
A mixture of seven broad scale and habitat scale variables combined to explain 95% of the variability in macrofaunal community composition (i.e., longitude, ice duration, ice thickness, % cover of sand, snow cover, % cover of sediment detritus, % sediment organic content; Figure 3B). The south-western McMurdo Sound locations tend to be arranged along Axis 1, with the Terra Nova Bay sites distributed along Axis 2 (Figure 3B). Cape Evans and Tethys Bay west are close together, near the bottom left of the CCA (Figure 3B). The variables closely associated with these axes are longitude and % cover of sand (Axis 1) and ice duration and snow cover (Axis 2).
When comparing the percentage variation explained at the different scales of observation, the multi-scale CCA (described above) explained the most variability in community composition for both epifauna and macrofauna (97 and 95%, respectively), with a similar number of variables in each case (Table 6; Figures 3A,B). For epifauna, the analysis incorporating only broad scale parameters also explained a high amount of community variation (91%), while that using parameters measured at only the habitat scale explained the least (64%) (Table 6). For macrofaunal communities, the variation explained by the separate habitat and broad scale CCAs was similar (i.e., 78 and 73%, respectively; Table 6).
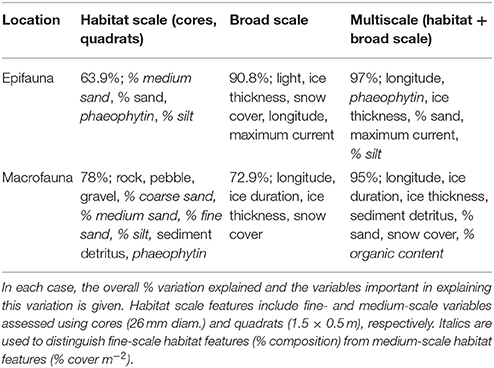
Table 6. Summary of results of the Canonical Correspondence Analysis (CCA) conducted to link benthic community composition to habitat scale and broad scale characteristics, separately and together (multiscale).
At the multi-scale, a very high percentage of variation in functional traits of macrofauna (97%) was explained by a combination of five broad and habitat-scale parameters (i.e., ice duration and thickness, % cover of sand, sediment detritus and Phyllophora; Figure 3C). Interestingly, none of the habitat-scale variables identified as important were those measured at the finest scale (i.e., sediment cores). Axis 2 distinguished New Harbor and Dunlop Island, both of which had abundant sand and no coarse sediment detritus, and the three Terra Nova Bay locations, with the shortest ice duration (8–9 months) and motile, surface dwelling predator/scavengers, were located in the top left-hand side of the ordination. Cape Evans was distinguished by its relatively high amount of Phyllophora (Figure 3C and Supplementary Table 1) and was grouped with Dunlop Island and New Harbor, reflecting the similarity in functional traits amongst these sites (i.e., freely motile near-surface dwelling deposit-grazers).
Discussion
This paper has described the benthic communities and environments at eight coastal Ross Sea locations. Information was collected using a nested sampling design that had previously been implemented at the three southernmost locations. This new description has provided further quantitative, baseline information on each location, as a step toward assessing future change. We describe how the epifaunal and macrofaunal communities, and the functional traits of the latter, vary between locations. A major aim was to investigate whether community characteristics are predictable based on the surrounding environmental factors, and whether the explanatory power of these factors increases with spatial scale of observation. The original analysis of only three locations (~32 km spatial extent) concluded that low or simple habitat structure did not necessarily result in low diversity of benthic fauna; a conclusion that was supported following the addition of five more locations to the north and east (~340 km total spatial extent). Even when using only habitat-scale variables, we could explain considerably more of the variation in community composition across our eight, more spatially extensive, sampling locations (epifauna 64%; macrofauna 78% explained; Table 6) than in our previous analysis of only the three southernmost locations (32 and 18%, respectively; Cummings et al., 2006). In the present study, the combination of habitat and broad scale variables explained ≥95% of the variability across all eight locations, indicating the importance of capturing environmental and biological variability across a larger scale in documenting and understanding community patterns.
Communities
Across all eight locations, epifaunal communities were dominated by one of three invertebrate taxa (S. neumayeri, A. colbecki, and O. validus; Table 4), with only 2-5 species in total. Overall abundances were generally lowest at the south-western most locations, New Harbor, Spike Cape and Dunlop Island, but there were no obvious patterns in species evenness (Table 3). These three locations receive relatively food-poor water that flows northwards along the coastline after exiting the Ross Ice Shelf (Barry and Dayton, 1988; Thrush et al., 2006; Figure 1). When the distribution of the three main taxa within locations was examined using CoV, all showed patchy distributions at some locations, and more even distributions at others (Table 4). This patchiness was not highly correlated with diversity in seafloor habitat type (as characterized from the transect-video; cf. Table 4 and Supplementary Table 1). The most dominant species in the macrofaunal communities were Polygordius antarcticus, Ophryotrocha ?notialis, Edwardsia sp., Munnidae type A, and copepods. Although, like the epifauna, one or two species tended to dominate these communities, many more species also featured in reasonable abundances (Table 5). Surface dwelling, freely motile macrofaunal taxa characterized the communities at the three Terra Nova Bay locations; at Gerlache Inlet and Tethys Bay south these were predator/scavengers, and at Tethys Bay west they were deposit feeder/grazers (Supplementary Figure 1). No patterns were apparent for the other locations (Supplementary Figure 1).
Linking Communities to Environment
Despite the obvious differences in environmental characteristics between some of the locations in this study—for example, New Harbor, where sea ice can persist for a decade and the seafloor is predominantly sandy, vs. Gerlache Inlet, where the ice breaks out annually and the seafloor habitat is heterogeneous—similarities in community composition exist that are often greater than those at locations with more similar environmental characteristics. In this example, epifaunal communities at both locations have high numbers of suspension feeding A. colbecki (Table 4), and the macrofaunal communities are dominated by polychaetes (Table 5). Surface dwelling freely motile macrofauna were the most common at both locations, however feeding traits differed (deposit-grazers at New Harbor, predator/scavengers at Gerlache Inlet; Supplementary Figure 1).
Formally examining benthic community composition and its relationship to environmental characteristics did, however, reveal some interesting patterns. In the epifaunal MDS and CCAs, the three locations with A. colbecki amongst their most dominant taxa, New Harbor, Dunlop Island and Gerlache Inlet, were located together in ordination space (Figures 2A, 3A). Of the environmental variables important in this separation, ice thickness and % cover of sand aligned with these communities in the CCA (Figure 3A). Maximum current speed is the variable important in separating the Granite Harbor epifaunal community (dominated by S. neumayeri, L. elliptica, and O. validus) from those at the remaining locations (Figure 3A). A total of six variables explained 97% of the variation in epifaunal community composition across the eight locations (longitude, phaeophytin, ice thickness, % cover of sand, maximum current, % composition of silt; Figure 3A). A similarly high percentage of the variation was explained for macrofaunal community composition (95%; longitude, ice duration, ice thickness, % cover of sediment detritus and sand, snow cover, % organic content; Figure 3B), and macrofaunal functional traits (97%; ice duration and thickness, sand, sediment detritus, Phyllophora; Figure 3C). While this explanatory power is extremely high, for both the epifaunal and macrofaunal community analyses, one of these variables was longitude, a likely surrogate for an environmental factor (or factors) not included in this analysis.
One of our aims in describing the functional traits of macrofaunal communities was to identify patterns that may reflect specific site characteristics. For example, an abundance of benthic suspension feeders could reflect a food-rich water column, more exposed/disturbed sites may be characterized by motile species. However, such simple relationships were not always apparent: while the low abundance of macrofaunal suspension feeders at water column food-poor New Harbor supports this idea, suspension feeders did not predominate at Gerlache Inlet, a location adjacent to the phytoplankton-rich Terra Nova Bay polynya (Supplementary Figure 1). Potentially, the general nature of the trait categories we used (e.g., predator/scavenger; deposit/grazer) has made identification of clear correlations difficult. Alternatively, and perhaps more likely, it could be due to the generally facultative or omnivorous nature of Antarctic fauna (e.g., Norkko et al., 2007; Wing et al., 2012). In the latter case, the expression of traits by a particular species may differ between locations, or along environmental gradients, perhaps in response to differential food availability or competition with other fauna, thus confusing the signal (e.g., Concepción et al., 2017, Gianuca et al., 2017). Indeed, the plasticity of feeding strategies of epifauna found at some of these same locations has already been noted (Norkko et al., 2007). A clearer understanding of function at the species and community scale is needed to characterise and predict the capacity for functional redundancy and therefore the inherent response diversity to help predict their likely resilience to environmental change. Incorporating other types of natural history information which have not been considered here, such as competitive interactions within and between species, will also be important in determining and understanding community patterns (e.g., Dayton, 1989).
Which Environmental Factors, and at Which Scale?
Broad scale environmental variables were more important drivers of community composition for epifauna, while habitat scale variables are most important for macroinfauna (Table 6). However, in both community types, environmental information at multiple scales (habitat + broad scale parameters combined) explained more variability in community composition than when either habitat or broad scale parameters were considered on their own (Table 6). This improvement in explanatory power with increasing scale was considerably greater for macrofauna than for epifauna (Table 6). Nevertheless, even for epifauna, multiscale factors explained 6% more than was explained by broad scale variables alone, and three of the six important variables in the multiscale analysis were habitat scale variables (phaeophytin, % composition of silt, % cover of sand; Table 6). For both macro- and epifaunal communities, the high number and variety of variables identified as influencing community composition illustrates the complexity of the system, and the inherent difficulty of predicting community composition based on simple (few factors) environmental information (e.g., Gutt, 2007 and references therein). These results further highlight the influence of environmental factors across multiple scales on structuring benthic communities.
The structuring influence of sea ice conditions and their control of light availability and productivity to benthic community structure and function is paramount and indisputable in this region (e.g., Thrush and Cummings, 2011; Fountain et al., 2016). Environmental factors reflective of sea ice conditions were important variables explaining variation in community composition and functional traits (i.e., ice thickness for epifauna; ice thickness and duration and snow cover for macrofauna; ice thickness and duration for macrofaunal functional traits; see Figure 3). This suggests that an indirect space-for-time substitution type approach, using gradients in these factors which are anticipated to change with warming, could be successfully used to predict ecosystem consequences under future scenarios. Nevertheless, the large number of other biophysical variables, processes and interactions contributing to structuring these communities signifies the complexity of the processes acting across this region. While we detected clear differences in environmental conditions between New Harbor and the considerably more productive and habitat diverse Gerlache Inlet, as already discussed there were also strong similarities in their epifaunal communities. More information is required to (i) understand interactions between structuring variables (including those not measured here), and (ii) incorporate temporal variability in these factors in the analysis.
Future Variables to Consider
There are several broad scale variables not considered in this analysis for which longitude could be a placeholder: these include disturbance by ice (Dayton, 1989; Gutt, 2001; Smale, 2007) and by wind and wave induced disturbance likely to be experienced during the (relatively short) ice free periods in summer. Characterizing hydrodynamic conditions at different scales (site, location, bay; coastal, and open ocean connectivity; e.g., Gutt et al., 2018) will be crucial to understanding potential sources of food and larvae, freshening and ice formation processes going forward (e.g., Hauquier et al., 2015). Associated with circulation, proximity to productive polynyas, phytoplankton blooms and their influences on supply of food are also not considered here, but will be very important in sustaining benthic communities (Dayton and Oliver, 1977; Thrush et al., 2010), and may underlie similarities in community patterns between locations. Although we have “estimated” productivity via sediment-associated measures of benthic chlorophyll a and phaeophytin, and light availability via PAR, other measures are needed to more explicitly link productivity sources from the sea ice and the water column to the benthos. Information on biogeochemical characteristics of the water column are also necessary, particularly as ocean acidification is anticipated to be one of the most important and widespread changes facing Antarctic waters (e.g., Fabry et al., 2009; Constable et al., 2014; Gutt et al., 2015). Freshening, from melt of ice shelves and glaciers and elevated inputs from terrestrial stream flows (e.g., Grange and Smith, 2013; Sahade et al., 2015; Dayton et al., 2016) can also be expected to influence biogeochemistry, particularly in these coastal regions. More data is needed on variability in these key factors across multiple spatial and temporal scales to elucidate the relative importance of the various factors from a community and/or population perspective (cf. Emslie et al., 2007).
Temporal Variation
The data presented here represent a snapshot in time of the epi- and macrofaunal benthic communities at each of our study locations. The influence of variation in environmental factors over time (seasonal, annual, and greater-than annual scales), particularly for ice conditions and productivity, have not been incorporated in our analyses, yet would be expected to have a strong influence on the benthic communities we documented at each sampling point (e.g., Dayton, 1989; Thrush and Cummings, 2011). While our study has explained virtually all the variability in community composition using our suite of environmental drivers, rapid or unexpected change to one (or several) of these parameters could deconstruct these relationships. For example, settlement and growth of a large hexactinellid sponge, absent in the preceding 22 years, was noted in correlation with a region-wide shift in phytoplankton productivity associated with the calving of a massive iceberg (Dayton et al., 2013). Such a change in community dominance resulting from episodic recruitment may be a good indicator of some environmental disruption having occurred. Although biological process amongst Antarctic benthos is considered slow, this same study, where observations were made over a 40 year time period, suggests that population increases and decreases may occur over decade rather than century time periods, emphasizing the need to re-evaluate ideas of slow processes and stability over century time scales (Dayton et al., 2013), and, likewise, their response to change. As climate change will result in direct and indirect effects across the food chain through alteration of bottom up and top down processes (e.g., Constable et al., 2014), more detailed natural history information on species and communities of interest is needed to predict likely change and sensitivities (e.g., Ricklefs, 2012). The importance of repeated and consistently made observations of biological communities, and biologically relevant environmental parameters (at appropriate space and time scales) in understanding ecosystem structure and function cannot be overstated, particularly when the aim is to detect responses to and predict implications of, future change (e.g., Ducklow et al., 2013; Sahade et al., 2015; Obryk et al., 2016; Barnes, 2017).
Concluding Comments
We consider that the data presented here can act as a valuable baseline against which future patterns can be assessed, and that it will be useful in the choice of best/most representative sites in the coastal Ross Sea for longer term studies. However, our aims in this analysis were not to merely describe existing benthic communities, habitats, and environmental conditions. Instead we hoped to define variables important for driving community and habitat change and to determine whether, once these were established, natural gradients in key parameters could be used to predict future changes associated with climate warming. Our results suggest that this indirect space for time surrogacy approach incorporating broad scale variables likely to be affected in future, may well be able to be utilized, but that the role of small-scale habitat features would also need to be incorporated for such predictions to be truly useful. When long term, consistent and repeated observations of coastal benthic communities are combined with measures of multiscale environmental characteristics likely to be important in influencing functioning and in structuring distributions of species, populations and communities, these observations become immensely powerful in understanding natural variability, detecting responses to and attributing causes of change.
Author Contributions
VC participated in all Antarctic sampling events, led the 2003–2007 field programmes, coordinated sample processing, analyses (sample identification, statistics) and planned and wrote this manuscript. AN led the 2001–2002 field programmes, and ST and PM were key participants in all field events. JH conducted the statistical analyses. PM analyzed the video transect footage, while NH contributed with sample identifications and figure preparation. All authors contributed to the design of the sampling protocols and the writing of the paper.
Funding
This research was funded by the New Zealand Ministry of Fisheries (ZBD2001/02, ZBD2002/01, ZBD2006/03), the New Zealand Ministry for Business, Innovation and Employment (COX01707), and the National Institute of Water and Atmospheric Research (NSOF, COMS1406).
Conflict of Interest Statement
The authors declare that the research was conducted in the absence of any commercial or financial relationships that could be construed as a potential conflict of interest.
Acknowledgments
We thank the many scientists and divers involved in the field components of this research (Neil Andrew, Rod Budd, Scott Edhouse, Greig Funnell, Ian Hawes, Drew Lohrer, Steve Mercer, Peter Notman, Anne-Marie Schwarz), NIWA Hamilton laboratory staff for sample processing, particularly Greig Funnell for video analysis and identifications, and Geoff Read for polychaete identifications. We are very grateful to Antarctica New Zealand for their excellent logistics support, without which this work would not be possible. Programma Nazionale Di Ricerche in Antartide are thanked for their support in Terra Nova Bay. Finally, we thank the reviewers for their constructive comments on this manuscript.
Supplementary Material
The Supplementary Material for this article can be found online at: https://www.frontiersin.org/articles/10.3389/fmars.2018.00232/full#supplementary-material
Supplementary Figure 1. The proportion of the macrofaunal community exhibiting different types of functional traits at each site and location. (A) Feeding mode, (B) motility, (C) living position.
Supplementary Table 1. Habitat characteristics at the transect-video scale (mean ± SE). The coarse sediment category also contained detrital organic material.
Supplementary Table 2. Habitat characteristics at the sediment core scale (mean ± SE). CE, Cape Evans; NH, New Harbor; DI, Dunlop Island; SC, Spike Cape; GH, Granite Harbor, GH; TBW, Tethys Bay west; TBS, Tethys Bay south; GI, Gerlache Inlet.
References
Ainley, D. G., Ballard, G., and Dugger, K. M. (2006). Competition among penguins and cetaceans revewals trophic cascades in the Western Ross Sea Antarctica. Ecology 87, 2080–2093. doi: 10.1890/0012-9658(2006)87[2080:CAPACR]2.0.CO;2
Aoki, S., Yoritaka, M., and Masuyama, A. (2003). Multidecadal warming of subsurface temperature in the Indian sector of the Southern Ocean. J. Geophys. Res. 108:8081. doi: 10.1029/2000JC000307
Arrigo, K. R., Van Dijken, G. L., Ainley, D. G., Fahnestock, M. A., and Markus, T. (2002). The impact of the B-15 iceberg on productivity and penguin breeding success in the Ross Sea, Antarctica. Geophys. Res. Lett. 29, 8-1–8-4. doi: 10.1029/2001GL014160
Barnes, D. K. A. (2017). Iceberg killing fields limit huge potential for benthic blue carbon in Antarctic shallows. Glob. Chang. Biol. 23, 2649–2659. doi: 10.1111/gcb.13523
Barry, J. P., and Dayton, P. K. (1988). Current patterns in McMurdo Sound, Antarctica and their relationship to biological production of local biotic communities. Polar Biol. 8, 367–376. doi: 10.1007/BF00442028
Boning, C. W., Dispert, A., Visbeck, M., Rintoul, S. R., and Schwarzkopf, F. U. (2008). The response of the Antarctic circumpolar current to recent climate change. Nat. Geosci. 1, 864–869. doi: 10.1038/ngeo362
Chevenet, F., Doledec, S., and Chessel, D. (1994). A fuzzy coding approach for the analysis of long-term ecological data. Freshw. Biol. 31, 295–309. doi: 10.1111/j.1365-2427.1994.tb01742.x
Clarke, K. R. (1993). Non-parametric multivariate analyses of changes in community structure. Austral. J. Ecol. 18, 117–143.
Clarke, A., Murphy, E. J., Meredith, M. P., King, J. C., Peck, L. S., Barnes, D. K., et al. (2007). Climate change and the marine ecosystem of the western Antarctic Peninsula. Philos. Trans. R. Soc. B. 362, 149–166. doi: 10.1098/rstb.2006.1958
Concepción, E. D., Götzenberger, L., Nobis, M. P., de Bello, F., Obrist, M. K., and Moretti, M. (2017). Contrasting trait assembly patterns in plant and bird communities along environmental and human-induced land-use gradients. Ecography 40, 753–763. doi: 10.1111/ecog.02121
Constable, A. J., Melbourne-Thomas, J., Corney, S. P., Arrigo, K. R., Barbraud, C., Barnes, D. K., et al. (2014). Climate change and Southern Ocean ecosystems I: how changes in physical habitats directly affect marine biota. Glob. Chang. Biol. 20, 3004–3025. doi: 10.1111/gcb.12623
Convey, P., Chown, S. L., Clarke, A., Barnes, D. K., Bokhorst, S., Cummings, V., et al. (2014). The spatial structure of Antarctic biodiversity. Ecol. Monogr. 84, 203–244. doi: 10.1890/12-2216.1
Cummings, V. J., Thrush, S. F., Norkko, A., Andrew, N. L., Hewitt, J. E., Funnell, G. A., et al. (2006). Accounting for local scale variability in benthos: implications for future assessments of latitudinal trends in the coastal Ross Sea. Antarc. Sci. 18, 633–644. doi: 10.1017/S0954102006000666
Dayton, P. K. (1989). Interdecadal variation in an Antarctic sponge and its predators from oceanographic climate shifts. Science 245, 1484–1486. doi: 10.1126/science.245.4925.1484
Dayton, P. K., Kim, S., Jarrell, S. C., Oliver, J. S., Hammerstrom, K., Fisher, J. L., et al. (2013). Recruitment, growth and mortality of an Antarctic hexactinellid sponge, Anoxycalyx joubini. PLoS ONE 8:e56939. doi: 10.1371/journal.pone.0056939
Dayton, P. K., Hammerstrom, K., Jarrell, S. C., Kim, S., Nordhausen, W., Osborne, D. J., et al. (2016). Coastal impacts of an unusual flood in Salmon Valley, McMurdo Sound, Antarctica. Antarc. Sci. 26, 6105–6122. doi: 10.1017/S0954102016000171
Dayton, P. K., and Oliver, J. S. (1977). Antarctic soft-bottom benthos in oligotrophic and eutrophic environments. Science 197, 55–58. doi: 10.1126/science.197.4298.55
Dayton, P. K., and Tegner, M. J. (1984). “The importance of scale in community ecology: a kelp forest example with terrestrial analogs,” in A New Ecology. Novel Approaches to Interactive Systems, eds P. W. Price, C. N. Slobodchikoff, and W. S. Gaud (New York, NY: John Wiley & Sons), 457–481.
Dierssen, H. M., Smith, R. C., and Vernet, M. (2002). Glacial meltwater dynamics in coastal waters West of the Antarctic Peninsula. Proc. Natl. Acad. Sci. U.S.A. 99, 1790–1795. doi: 10.1073/pnas.032206999
Ducklow, H. W., Baker, K., Martin, D. G., Quetin, L. B., Ross, R. M., Smith, R. C., et al. (2007). Marine pelagic ecosystems: the West Antarctic Peninsula. Philos. Trans. R. Soc. B. 362, 67–94. doi: 10.1098/rstb.2006.1955
Ducklow, H. W., Fraser, W. R., Meredith, M. P., Stammerjohn, S. E., Doney, S. C., Martinson, D. G., et al. (2013). West Antarctic Peninsula: an ice-dependent coastal marine ecosystem in transition. Oceanography 26, 190–203. doi: 10.5670/oceanog.2013.62
Emslie, S. D., Coats, L., and Licht, K. (2007). A 45,000 yr record of Adélie penguins and climate change in the Ross Sea, Antarctica. Geology 35, 61–64. doi: 10.1130/G23011A.1
Fabry, V. J., McClintock, J. B., Mathis, J. T., and Grebmeier, J. M. (2009). Ocean acidification at high latitudes: the bellweather. Oceanography 22, 160–171. doi: 10.5670/oceanog.2009.105
Fountain, A. G., Saba, G., Adams, B., Doran, P., Fraser, W., Gooseff, M., et al. (2016). The impact of a large-scale climate events on Antarctic ecosystem processes. Bioscience 66, 848–863. doi: 10.1093/biosci/biw110
Fukami, T., and Wardle, D. A. (2005). Long-term ecological dynamics: reciprocal insights from natural and anthropogenic gradients. Proc. R. Soc. B Biol. Sci. 272, 2105–2115. doi: 10.1098/rspb.2005.3277
Gianuca, A. T., Declerck, S. A. J., Cadotte, M. W., Souffreau, C., De Bie, T., and De Meester, L. (2017). Integrating trait and phylogenetic distances to assess scale-dependent community assembly processes. Ecography 40, 742–752. doi: 10.1111/ecog.02263
Grange, L. J., and Smith, C. R. (2013). Megafaunal communities in rapidly warming fjords along the West Antarctic Peninsula: hotspots of abundance and beta diversity. PLoS ONE 8:e77917. doi: 10.1371/journal.pone.0077917
Gutt, J. (2001). On the direct impact of ice on marine benthic communities, a review. Polar Biol. 24, 553–564. doi: 10.1007/s003000100262
Gutt, J. (2007). Antarctic macro-zoobenthic communities: a review and an ecological classification. Antarc. Sci. 19, 165–182. doi: 10.1017/S0954102007000247
Gutt, J., Barratt, I., Domack, E., d'Udekem d'Acoz, C., Dimmler, W., Grémare, A., et al. (2011). Biodiversity change after climate-induced ice-shelf collapse in the Antarctic. Deep Sea Res. II 58, 74–83. doi: 10.1016/j.dsr2.2010.05.024
Gutt, J., Bertler, N., Bracegirdle, T. J., Buschmann, A., Comiso, J., Hosie, G., et al. (2015). The Southern Ocean ecosystem under multiple climate change stresses - an integrated circumpolar assessment. Glob. Change Biol. 21, 1434–1453. doi: 10.1111/gcb.12794
Gutt, J., Cape, M., Dimmler, W., Fillinger, L., Isla, E., Lieb, V., et al. (2013). Shifts in Antarctic megabenthic structure after ice-shelf disintegration in the Larsen area east of the Antarctic Peninsula. Polar Biol. 36, 895–906. doi: 10.1007/s00300-013-1315-7
Gutt, J., Cummings, V., Dayton, P. K., Isla, E., Jentsch, A., and Schiaparelli, S. (2016). “Antarctic marine animal forests-three-dimensional communities in Southern Ocean ecosystems,” in Marine Animal Forests: The Ecology of Benthic Biodiversity Hotspots, eds S. Rossi, L. Bramanti, A. Gori, and C. Orejas Saco del Valle (Springer International Publishing), 315–344.
Gutt, J., Isla, E., Bertler, N., Bodeker, G., Bracegirdle, T., Comiso, J., et al. (2018). Cross-disciplinarity in the advance of Antarctic ecosystem research. Mar. Genomics 37, 1–17. doi: 10.1016/j.margen.2017.09.006
Hauquier, F., Durán Suja, L., Gutt, J., Veit-Köhler, G., and Vanreusel, A. (2015). Different oceanographic regimes in the vicinity of the Antarctic Peninsula reflected in benthic nematode communities. PLoS ONE 10:e0137527. doi: 10.1371/journal.pone.0137527
Hewitt, J. E., Thrush, S. F., and Dayton, P. K. (2008). Habitat variation, species diversity and ecological functioning in a marine system. J. Exp. Mar. Biol. Ecol. 366, 116–122. doi: 10.1016/j.jembe.2008.07.016
IPCC (2013). “Climate change 2013: the physical science basis,” in Contribution of Working Group I to the Fifth Assessment Report of the Intergovernmental Panel on Climate Change, eds T. F. Stocker, D. Qin, G. K. Plattner, M. Tignor, S. K. Allen, J. Boschung, A. Nauels, Y. Xia, V. Bex, and P. M. Midgley (Cambridge, UK; New York, NY), 1535.
Jacobs, S. S., and Giulivi, C. F. (2010). Large multidecadal salinity trends near the Pacific-Antarctic Continental Margin. J. Climate 23, 4508–4524. doi: 10.1175/2010JCLI3284.1
Kolasa, J., and Pickett, S. T. A. (eds). (1991). Ecological Heterogeneity. Ecological Studies (Analysis and Synthesis), Vol. 86. New York, NY: Springer.
Kooyman, G. L., Ainley, D. G., Ballard, G., and Pongannis, P. J. (2007). Effects of giant icebergs on two emperor penguin colonies in the Ross Sea, Antarctica. Antarc. Sci. 19, 31–38. doi: 10.1017/S0954102007000065
Martinson, D. G., Stammerjohn, S. E., Iannuzzi, R. A., Smith, R. C., and Vernet, M. (2008). Western Antarctic Peninsula physical oceanography and spatio-temporal variability. Deep Sea Res. Part II 55, 1964–1987. doi: 10.1016/j.dsr2.2008.04.038
Mayewski, P. A., Bracegirdle, T., Goodwin, I., Schneider, D., Bertler, N. A. N., Birkel, S., et al. (2015). Potential for Southern Hemisphere surprises. J. Q. Sci. 20, 391–395. doi: 10.1002/jqs.2794
McNeil, B. I., and Matear, R. J. (2008). Southern Ocean acidification: a tipping point at 450-ppm atmospheric CO2. Proc. Natl. Acad. Sci. U.S.A. 105, 18860–18864. doi: 10.1073/pnas.0806318105
Meredith, M. P., and King, J. C. (2005). Rapid climate change in the ocean west of the Antarctic Peninsula during the second half of the 20th century. Geophys. Res. Lett. 32:L19604. doi: 10.1029/2005GL024042
Moline, M. A., Karnovsky, N. J., Brown, Z., Divoky, G. J., Frazer, T. K., Jacoby, C. A., et al. (2008). “High latitude changes in ice dynamics and their impact on polar marine ecosystems,” in Year in Ecology and Conservation Biology 2008. Annals of the New York Academy of Sciences. Vol. 1134, eds R. S. Ostfeld and W. H. Schlesinger (Hoboken, NJ: John Wiley & Sons, Inc.), 267–319.
Monien, D., Monien, P., Brünjes, R., Widmer, T., Kappenberg, A., Silva Busso, A., et al. (2017). Meltwater as a source of potentially bioavailable iron to Antarctica waters. Antarct. Sci. 29, 277–291. doi: 10.1017/S095410201600064X
Norkko, A., Thrush, S. F., Cummings, V. J., Gibbs, M. M., Andrew, N. L., Norkko, J., et al. (2007). Trophic structure of coastal Antarctic food webs associated with changes in food supply and sea ice extent. Ecology 88, 2810–2820. doi: 10.1890/06-1396.1
Obryk, M. K., Doran, P. T., Friedlaender, A. S., Gooseff, M., Priscu, J. C., Stammerjohn, S. E., et al. (2016). Responses of Antarctic marine and freshwater ecosystems to changing ice conditions. Bioscience 66, 864–879. doi: 10.1093/biosci/biw109
Orr, J. C., Fabry, V. J., Aumont, O., Bopp, L., Doney, S. C., Feely, R. A., et al. (2005). Anthropogenic ocean acidification over the twenty-first century and its impact on calcifying organisms. Nature 437, 681–686. doi: 10.1038/nature04095
Pasotti, F., Saravia, L. A., De Troch, M., Tarantelli, M. S., Sahade, R., and Vanreusel, A. (2015). Benthic trophic interactions in an Antarctic shallow water ecosystem affected by recent glacier retreat. PLoS ONE 10:e0141742. doi: 10.1371/journal.pone.0141742
Peck, L. S. (2011). Organisms and responses to environmental change. Mar. Genomics 4, 237–243. doi: 10.1016/j.margen.2011.07.001
Peck, L. S., Webb, K., and Bailey, D. (2004). Extreme sensitivity of biological function to temperature in Antarctic marine species. Funct. Ecol. 18, 625–630. doi: 10.1111/j.0269-8463.2004.00903.x
Pickett, S. T. A. (1989). “Space-for-time substitution as an alternative to long-term studies,” in Long-Term Studies in Ecology, ed G. E. Likens (New York, NY: Springer), 110–135.
Ricklefs, R. E. (1987). Community diversity: relative roles of local and regional processes. Science 235, 167–171. doi: 10.1126/science.235.4785.167
Ricklefs, R. E. (2012). Naturalists, natural history, and the nature of biological diversity. Am. Nat. 179, 423–435. doi: 10.1086/664622
Sahade, R., Lagger, C., Torre, L., Momo, F., Monien, P., Schloss, I., et al. (2015). Climate change and glacier retreat drive shifts in an Antarctic benthic ecosystem. Sci. Adv. 1:e1500050. doi: 10.1126/sciadv.1500050
Sartory, D. P. (1982). Spectrophotometric Analysis of Chlorophyll a in Freshwater Phytoplankton. Report No. TR 115. Hydrological Research Institute, Department of Environment Affairs, Pretoria, 163.
Schloss, I. R., Abele, D., Moreau, S., Demers, S., Bers, A. V., González, O., et al. (2012). Response of phytoplankton dynamics to 19-year (1991–2009) climate trends in Potter Cove (Antarctica). J. Mar. Syst. 92, 53–66. doi: 10.1016/j.jmarsys.2011.10.006
Siniff, D. B., Garrott, R. A., Rotella, J. J., Fraser, W. R., and Ainley, D. G. (2008). Projecting the effects of environmental change on Antarctic seals. Antarct. Sci. 20, 425–435. doi: 10.1017/S0954102008001351
Smale, D. A. (2007). Ice disturbance intensity structures benthic communities in nearshore Antarctic waters. Mar. Ecol. Prog. Ser. 349, 89–102. doi: 10.3354/meps07104
Smith, W. O., Ainley, D., Cattaneo-Vietti, R., and Hofmann, E. (2012a). “The Ross Sea continental shelf: regional biogeochemical cycles, trophic interactions, and potential future changes,” in Chapter 7 in Antarctic Ecosystems: An Extreme Environment in a Changing World, eds A. D. Rogers, N. M. Johnston, E. J. Murphy, and A. Clarke (New Jersey, NJ: Blackwell Publishing Ltd), 213–235.
Smith, W. O., Ainley, D. G., Arrigo, K. R., and Dinniman, M. S. (2014). The oceanography and ecology of the Ross Sea. Ann. Rev. Mar. Sci. 6, 469–487. doi: 10.1146/annurev-marine-010213-135114
Smith, W. O., Sedwick, P. N., Arrigo, K. R., Ainley, D. G., and Orsi, A. H. (2012b). The Ross Sea in a sea of change. Oceanography 25, 90–103. doi: 10.5670/oceanog.2012.80
Stammerjohn, S. E., Martinson, D. G., Smith, R. C., Yuan, X., and Rind, D. (2008). Trends in Antarctic annual sea ice retreat and advance and their relation to El Niño–Southern Oscillation and Southern Annular mode variability. J. Geophys. Res. 113:C03S90. doi: 10.1029/2007JC004269
Stammerjohn, S. E., Massom, R., Rind, D., and Martinson, D. G. (2012). Regions of rapid sea ice change: an interhemispheric seasonal comparison. Geophys. Res. Lett. 39:L06501. doi: 10.1029/2012GL050874
ter Braak, C. J. F. (1986). Canonical correspondence analysis: a new eigenvector technique for multivariate direct gradient analysis. Ecology 67, 1167–1179.
Thrush, S. F., and Cummings, V. J. (2011). Massive icebergs, alteration in primary food resources and change in benthic communities at Cape Evans, Antarctica. Mar. Ecol. 32, 289–299. doi: 10.1111/j.1439-0485.2011.00462.x
Thrush, S. F., Dayton, P. K., Cattaneo-Vietti, R., Chiantore, M., Cummings, V. J., Andrew, N. L., et al. (2006). Broad-scale factors influencing the biodiversity of coastal benthic communities of the Ross Sea. Deep Sea Res. II 53, 959–971. doi: 10.1016/j.dsr2.2006.02.006
Thrush, S. F., Hewitt, J. E., Cummings, V. J., Norkko, A., and Chiantore, M. (2010). β-diversity and species accumulation in Antarctic coastal benthic communities: the role of habitat, distance and productivity on ecological connectivity. PLoS ONE 5:e11899. doi: 10.1371/journal.pone.0011899
Turner, J. R., Bindschadler, A., Convey, P., Di Prisco, G., Fahrbach, E., Gutt, J., et al. (2009). Scientific Committee on Antarctic Research. Antarctic Climate Change and the Environment, 526.
Walsh, J. E. (2009). A comparison of Arctic and Antarctic climate change, present and future. Antarc. Sci. 21, 179–188. doi: 10.1017/S0954102009001874
Keywords: Antarctica, benthos, coast, environmental change, multi-scale observations, functional traits
Citation: Cummings VJ, Hewitt JE, Thrush SF, Marriott PM, Halliday NJ and Norkko A (2018) Linking Ross Sea Coastal Benthic Communities to Environmental Conditions: Documenting Baselines in a Spatially Variable and Changing World. Front. Mar. Sci. 5:232. doi: 10.3389/fmars.2018.00232
Received: 04 March 2018; Accepted: 15 June 2018;
Published: 09 July 2018.
Edited by:
Huw James Griffiths, British Antarctic Survey (BAS), United KingdomReviewed by:
Jan Marcin Weslawski, Institute of Oceanology (PAN), PolandJeroen Ingels, Florida State University, United States
Copyright © 2018 Cummings, Hewitt, Thrush, Marriott, Halliday and Norkko. This is an open-access article distributed under the terms of the Creative Commons Attribution License (CC BY). The use, distribution or reproduction in other forums is permitted, provided the original author(s) and the copyright owner(s) are credited and that the original publication in this journal is cited, in accordance with accepted academic practice. No use, distribution or reproduction is permitted which does not comply with these terms.
*Correspondence: Vonda J. Cummings, dm9uZGEuY3VtbWluZ3NAbml3YS5jby5ueg==