- 1Département de Biologie Marine, Centre Scientifique de Monaco, Monaco City, Monaco
- 2Sorbonne Universités, University Paris, Paris, France
- 3UMR ENTROPIE (UMR250), Laboratoire d'Excellence “CORAIL”, New Caledonia, France
- 4Institut National Pour la Recherche Agronomique, UMR CARRTEL, Thonon les Bains, France
Environmental conditions are known to influence corals and their associated communities of microorganisms. However, our insights into the impacts of seasonal changes in ultraviolet radiation (UVR) on both coral physiology and microbiome remain very limited. To address this challenge, we maintained the coral Acropora muricata shaded from UVR or under ambient UVR levels during two contrasting seasons, i.e. summer and winter, and assessed the impact of UVR on the coral holobiont at each season. To this end, we analyzed the physiology (e.g., calcification, protein content, photosynthesis-related parameters) and coral microbiota composition, as well as the abundance and composition of the microbial communities and organic matter contents of the surrounding seawater. Our results show major seasonal effects on coral phenotype: (1) a lower host biomass and photosynthesizing, but fast calcifying phenotype in summer, and (2) a higher host biomass and photosynthesizing, but slow calcifying phenotype in winter. UVR had only a significant impact on Symbiodinium functioning. Specifically, high UVR levels reduced photosynthesis efficiency in summer, but an increase in chlorophyll a content may have compensated for this effect. The coral microbiota, which was variable but generally dominated by Endozoicomonas, was not affected by UVR, but its composition differed between seasons. In contrast, UVR had a major, but differential impact on the seawater microbial communities at both seasons. Particularly in summer, bacteria from the Alteromonadaceae were significantly more abundant (15-fold; up to 75%) in seawater under ambient UVR levels. Overall, our study suggests that UVR has only a limited impact on coral holobiont composition and functioning, despite major fluctuations in the surrounding seawater microbiome; seasonal changes in the holobiont are thus mostly driven by other environmental factors.
Introduction
Population dynamics and reproductive cycles of many species are influenced by seasonal variations in environmental factors (Clarke, 1988; Gooday et al., 1990; Coma et al., 2000), leading to a long-standing interest in the way organisms react to the changing of seasons. Although tropical corals live in a relatively stable environment, they experience seasonal fluctuations in environmental conditions, such as seawater temperatures (up to 9°C, Dandan et al., 2015) or photosynthetically active radiation (PAR; up to 5 times, Browne et al., 2015). Nutrient levels and water quality can also vary by a factor 5 between seasons, through for example, sediment loading, or metal and nutrient pollution (Browne et al., 2015; Watson et al., 2017). Seasonality is reflected both in the coral physiology (Crossland, 1984; Warner et al., 2002; Ulstrup et al., 2008) and in the composition of coral-associated microbial communities (Brown et al., 1999; Fitt et al., 2000; Littman et al., 2010; Li et al., 2014). The symbiotic association of the coral animal with its diverse assemblages of microorganisms consisting in bacteria, archaea, fungi, viruses, and protists, including the dinoflagellate algae Symbiodinium (Kelly et al., 2014; Apprill et al., 2016), is termed the coral holobiont (Rohwer et al., 2002).
Most studies, which investigated seasonal changes in the physiology and composition of the coral holobiont, have focused on the effect of PAR intensities on Symbiodinium density (Fitt et al., 2000) and photosynthetic capacities (Edmunds and Davies, 1986; Warner et al., 2002; Ulstrup et al., 2008). The effect of seawater temperature variations on coral physiology has also received a lot of attention; in particular because episodes of temperature extremes can induce coral bleaching (loss in symbionts and photosynthetic pigments), resulting in decreased photosynthesis and calcification rates (Hoegh-Guldberg, 1999; Hoegh-Guldberg et al., 2017; Hughes et al., 2017; Wolff et al., 2018), as well as shifts in the composition of coral-associated microbial communities (Littman et al., 2010, 2011; Ziegler et al., 2017). However, little is known about the response of coral holobionts to seasonal changes in ultraviolet radiation (UVR) levels. UVR is an important component of the sunlight received by corals in shallow waters, where high intensities prevail (Madronich et al., 1998). UVR levels actually show depth and seasonal variations (Smith and Baker, 1981; Torres et al., 2007; Zepp et al., 2008), which may contribute to the seasonal changes that have been observed in coral physiology. For example, variations in tissue content of mycosporine-like amino acids (MAAs), which protect corals from the deleterious effects of UVR (Shick et al., 1996; Shick and Dunlap, 2002) has been attributed to changes in UV exposure (Lesser and Lewis, 1996; Lesser, 2000), and severe bleaching has been linked to the concomitant increase in seawater temperature and UVR levels (Lesser et al., 1990). Despite these few studies, the seasonal changes in UVR levels on Symbiodinium functioning, coral calcification, organic matter release and coral microbiota need to be further studied. UVR also affects the growth, metabolism, and viability of seawater microorganisms (Korbee et al., 2010; Llabrés et al., 2010; Carrillo et al., 2015), but the seasonal effect of UVR exposure on reef bacterioplankton has been poorly studied (Lyons et al., 1998; Conan et al., 2008; Häder et al., 2015). Changes in the composition of bacterioplankton surrounding coral colonies can affect the composition of coral-associated bacterial communities (Wear and Thurber, 2015 and references therein); the contrary may be true as well, as corals release microbes into the environment, for example via mucus excretion (Allers et al., 2008; Garren and Azam, 2012; Nelson et al., 2013; Taniguchi et al., 2015).
To better understand the impact of UV radiation on coral holobiont functioning, we investigated the physiology and microbiota of the holobiont of the scleractinian coral Acropora muricata and its surrounding seawater during two seasons (summer and winter) under ambient and reduced UVR levels. A. muricata is a dominant coral species in New Caledonia, whose populations have severely suffered from a mass bleaching events in 2016. Such knowledge provides novel insights into how environmental conditions influence the status of the coral holobiont and its interactions with seawater microbial communities.
Experimental Procedures
Coral Collection and Experimental Setup
Twenty 3 cm-long nubbins of Acropora muricata were collected from the 10 different parent colonies at 3 m depth on the reef of Îlot Maître in the Nouméa lagoon (22°19′S, 166°24′E) in January 2016 (Austral summer - before the mass bleaching event of February 2016) (permit number: APA-NCPS-2016-001). In June 2016 (Austral winter), nubbins were collected from the same parental colonies. At both seasons, nubbins were evenly distributed in four 100 L outdoor experimental tanks continuously supplied with lagoon water at a rate of 72 L h−1. Corals were fed once a week with Artemia salina nauplii. Photosynthetically active radiation (PAR) in the experimental tanks was maintained at the same level as in situ using shade cloths, and measured using a LI-1000 data logger connected to a LI-193 spherical quantum sensor (LI-COR, Lincoln, NE, USA). UVR levels were measured using an ILT1400 portable radiometer connected to SEL033/UVA/TD UVA and SEL240/UVB-1/TD UVB detectors (International Light Technologies, Peabody, MA, USA). In summer, the maximal irradiance obtained at midday on a sunny day was ~900 μmole quanta m−2 s−1 PAR, ~20 W m−2 ultraviolet A (UVA, 315–400 nm) and ~1.2 W m−2 of ultraviolet B (UVB, 280–315 nm) radiation. In winter, irradiance levels on a sunny day at mid-day were ~500 μmole quanta m−2 s−1 of PAR, ~9 W m2 of UVA, ~0.7 W m2 of UVB. Two out of the four tanks were shielded from UVR (condition hereafter called “no UV”) using specific UVR filters (226 Lee U.V. filters), while the two other tanks received the ambient dose of UVR (condition hereafter called “UV”). Following collection, coral nubbins were maintained under experimental UVR conditions for 1.5 months prior to the assay incubations (described below) in summer and winter. At both seasonal time points, five nubbins from each condition (UV and no UV), were randomly sampled from the two tanks per condition, and used to assess tissue parameters (symbiont density, chlorophyll and protein concentrations), calcification rates and photosynthetic efficiency (assay details described below). Following experimental incubations, nubbins were frozen at −20°C until further analyses. The remaining five nubbins per condition were used to assess coral-associated bacterial communities (see details below).
All incubations were performed under the same temperature (29.3 ± 0.9°C in summer and 23.1 ± 0.6°C in winter) and light regime as the experimental tanks. Symbiont density, chlorophyll a and protein content, net and gross photosynthesis, respiration and calcification rates were normalized to the nubbin surface area (cm2) measured using the wax technique (Stimson and Kinzie, 1991).
Photochemical Efficiency
Five nubbins per condition were incubated in the dark for 10 min before the relative electron transport rate (rETR) versus irradiance, or rapid light curves, were generated using a Pulse Amplitude Modulation (PAM) fluorometer (Diving-PAM, Walz, Germany) according to Ralph and Gademann (2005). For this purpose, nubbins were illuminated for 10 s with seven different light intensities (from 0 to 900 μmole quanta m−2 s−1) and the rETRmax was deduced from the rapid light curves.
Calcification Rates
Nubbins were incubated 2 h in individual 100 mL beakers filled with 0.45 μm filtered seawater, continuously stirred with stirring bars and hermetically closed to avoid any oxygen exchange with the ambient air. The incubation seawater in each beaker was filtered through 0.2 μm and stored at 4°C for the subsequent determination of the calcification rates using the alkalinity anomaly method (Smith and Key, 1975). The measurement was performed using a Titralab TIM865 titration manager (Hach, Loveland, CO, USA). Data were expressed in μmol CaCO3 cm−2 d−1 and corrected against a blank (filtered seawater incubated for the same period without nubbin).
Organic Matter and Planktonic Microbe Concentrations in Seawater
Nubbins were incubated for 2 h in 200 mL of 0.45 μm filtered seawater under each experimental condition. The total organic carbon (TOC) and total nitrogen (TN) concentration was assessed by sampling 20 mL seawater in triplicate from each beaker with sterile syringes at the end of the incubation. Samples were transferred to pre-combusted (450°C, 5 h) glass vials, acidified with phosphoric acid (20%, 250 μL) and kept frozen until subsequent analysis. For dissolved organic carbon (DOC) and dissolved nitrogen (DN) concentration, 20 mL subsamples were taken from each beaker in triplicate and filtered through 0.22 μm pore size filter (Millipore, Burlington, MA, USA). Samples were analyzed using a TOC-L analyzer (Shimadzu, Japan) and data were expressed in μg L−1. To determine the concentrations of planktonic microbes, 4.8 mL seawater were sampled at the end of the incubation, fixed with 0.2 mL of glutaraldehyde (25%) for 30 min in the dark and then stored at −80°C. Samples were analyzed by flow cytometry as described by Jacquet et al. (2013).
Symbiont, Chlorophyll a, and Protein Content
Five coral nubbins maintained under each experimental condition were incubated in 1L of 0.45 μm filtered seawater, continuously stirred with stirring bars, for 2 h, as described above. After the incubations, nubbins were frozen at −20°C for the subsequent determination of tissue parameters. Nubbin tissue was airbrushed in filtrated seawater and homogenized with a Potter-Elvejhem tissue grinder. A sub-sample was taken for the determination of the symbiont density of each sample using a Neubauer cell, on five replicated counts. A second sub-sample was used to assess the protein content according to Hoogenboom et al. (2010), using BCA assay kit (Interchim Protein Quantification Kit). For chl a measurements, another subsample was centrifuged at 5,000 × g for 10 min at 4°C to separate the symbionts (in the pellet) from the host tissue. The pellet was then re-suspended in 10 mL acetone and kept in the dark at 4°C for 24 h prior to measurements. Samples were then centrifuged for 15 min at 10,000 × g and the absorbance was measured at 630, 663, and 750 nm using an EVOLUTION 201 UV-Visible spectrophotometer (Thermo Fisher Scientific). Chlorophyll concentrations were computed using the equations of Jeffrey and Humphrey's (1975).
Statistical Analysis
Effects of UVR and season on coral tissue parameters, Symbiodinium photophysiology, and seawater organic matter content and microbial abundances were assessed with two-way ANOVAs using UVR and season as factors. Normality of the residuals and variance homoscedasticity were tested using Shapiro and Bartlett tests, respectively. When needed, data were log transformed in order to fulfill those criteria. A Tukey's High Significance Difference post hoc test was performed when results of the ANOVAs were significant, with the a priori decision to determine only the effects of UVR in winter and summer, as well as the effects of season under ambient conditions (i.e., under UVR).
Bacterial Community Analysis
To assess the bacterial diversity, seawater from each experimental condition was filtered sequentially through 10, 3, and 0.2 μm Whatman Nuclepore Track-Etched filters (Sigma-Aldrich) and the last filter was kept in RNAlater (ThermoFisher Scientific) at −20°C. Five nubbins from each tank condition were preserved in RNAlater at 4°C for 24 h before being stored at −20°C. Reference samples of seawater and coral preserved directly after fragment collection from the lagoon had not been collected, and it should therefore be taken into account that the microbiota in aquaria may differ slightly from the natural conditions as previously observed (Kooperman et al., 2007; Mohamed et al., 2008; Pratte et al., 2015; Röthig et al., 2017). RNAlater-preserved coral nubbins were crushed frozen in liquid nitrogen. DNA was extracted from crushed coral and from the filters using the Genomic DNA Buffer Set and Genomic-tip 20/G columns (QIAGEN, Hilden, Germany) according to the manufacturer's protocol. DNA concentrations were determined for each sample and samples were sent to Molecular Research Laboratory (MR DNA, Shallowater, Texas, USA) for 16S rRNA gene amplicon library generation using the 515F/806R primer pair, which targets the V4 region of the 16S rRNA gene, and paired-end (2 × 300 bp) sequencing on the Illumina MiSeq platform.
16S rRNA Gene Amplicon Data Analysis
The quality (.qual) and reads (.fasta) files were obtained from MR DNA and contained a total of 5,163,731 reads. The QIIME v1.9 pipeline (Caporaso et al., 2010) was used to process the data as detailed in van de Water et al. (2018b). Briefly, low quality (Phred <20) sequences, and reads <200 bp in length were removed and each read was assigned to its respective sample using the split_libraries.py script. Chimeric sequences were identified and removed using the UCHIME algorithm (Edgar et al., 2011) and the SILVA v123 database (Quast et al., 2013) as reference. The quality filtered sequence file contained 4,582,530 reads, with an average of 114.563 reads per sample (range 55,679–170,361). Operational Taxonomic Units (OTUs) were defined at the level of 97% similarity. Taxonomic identities were assigned using the UCLUST algorithm (Edgar, 2010), implemented in the assign_taxonomy.py script, against the SILVA reference database (version 123). Singletons, and OTUs classified as chloroplast or mitochondria were subsequently removed from the dataset, resulting in an average of 98,189 reads per sample (range 43,353–158,135). Alpha and beta diversity metrics were generated from OTU tables rarified to 43.353 reads per sample using the QIIME pipeline. OTU tables, sample metadata and representative sequences of each OTU are provided in the Supplementary Data. Raw sequences were deposited in the NCBI Sequence Read Archive (SRA) under BioProject accession number PRJNA408048.
The phyloseq package (McMurdie and Holmes, 2013) integrated in R was used to generate statistically relevant graphical presentations of the microbiome data obtained, including (1) abundance plots of relevant taxa, and (2) a non-metric dimensional scaling (nMDS) on Bray-Curtis dissimilarity matrices, to visualize differences in the beta diversity of seawater and coral-associated bacterial communities under the different experimental conditions. Permutational Analysis of Variance (permANOVA) performed under Type III partial sums of squares and 9,999 permutations under the reduced model was used to statistically assess differences in bacterial community diversity using PRIMER 6 & PERMANOVA+ (PRIMER-E Ltd, Auckland, New Zealand) (Clarke and Gorley, 2006; Anderson et al., 2008). Negative binomial modeling (likelihood ratio tests for the effect of experimental factors and Wald tests for pair-wise comparisons) implemented in the DESeq2 package in R (Love et al., 2014), was used for differential abundance analysis followed by Benjamini-Hochberg False Discovery Rate corrections to test which bacterial OTUs were impacted by UV radiation and/or season. Overall effects of UV radiation and season on the seawater and coral-associated bacterial communities, and potential differences in dispersion among samples within treatment groups, were investigated using the ADONIS and betadisper functions in the R-package vegan, respectively (Oksanen et al., 2011).
Results
Impact of Season and UVR on Coral Holobiont Physiology
Under ambient UVR exposure (reef conditions), there was no difference in symbiont density and chlorophyll a content in the tissues of A. muricata between seasons (Table S1, Figures 1A,B,C), but the maximal relative electron transport rate (rETRmax), which is a proxy for the photosynthetic efficiency of the symbionts, was 54% lower in summer compared to winter (Figure 1D). UVR shading significantly decreased the rETRmax by 29% in winter (Table S1); however, it was increased by 48% in summer, despite a lower chl a content in the tissues and per Symbiodinium cell.
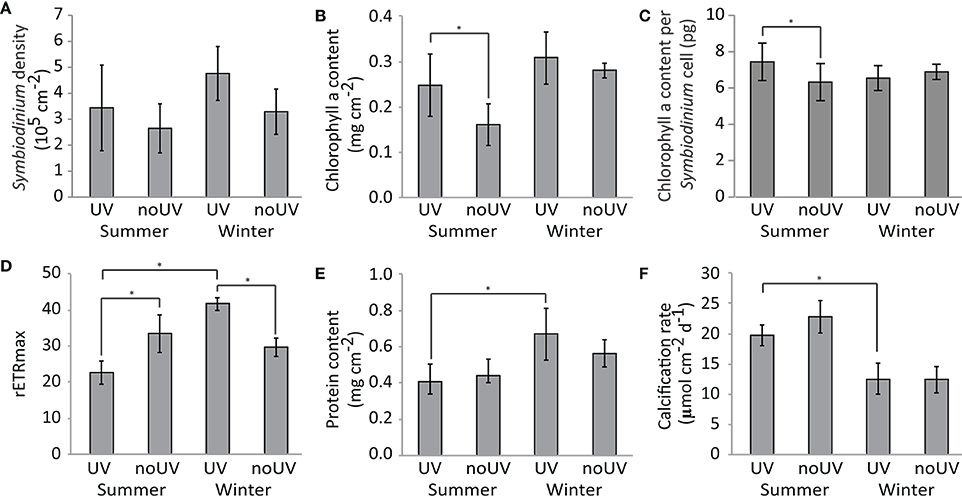
Figure 1. Changes in the physiological parameters of the A. muricata holobiont [coral and Symbiodinium: (A) Sybiodinium density, (B) Chlorophyll a content, (C) Chlorophyll content per Symbiodinium cell, (D) maximum relative Electron Transport Rate, (E) Protein content, and (F) Calcification rate] under ambient or no UV radiation at two contrasting seasons (summer and winter). Data are the mean and standard deviations of five biological replicates. Comparisons indicated with *are significantly different (Tukey's Honest Significant Difference-corrected p < 0.05).
Protein content of coral tissue was 32% lower in summer compared to winter, while calcification rate was 57% higher (Figures 1E,F, Table S1). UVR did not have an effect on these coral physiological parameters at either season.
Impact of Season and UVR on Seawater Organic Matter
Significant differences in the concentrations of total organic carbon (TOC) and total nitrogen (TN) as well as dissolved organic carbon (DOC) and dissolved nitrogen (DN) were found between summer and winter regardless of UVR level (Figure 2; Table S1). Under ambient UVR levels, TOC and DOC were 33 and 43% higher in winter compared to summer, respectively. Contrary, levels of TN were 62% higher in summer compared to winter, while DN levels were 65% higher.
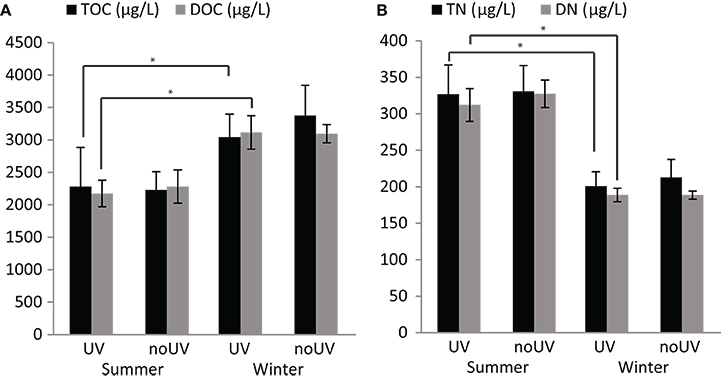
Figure 2. Concentrations of organic matter in seawater. Differences in the concentrations of (A) total and dissolved organic carbon (TOC and DOC, respectively), and (B) total and dissolved nitrogen (TN and DN, respectively) in the seawater. Data are the mean and standard deviations of five biological replicates. Comparisons indicated with *are significantly different (Tukey's Honest Significant Difference-corrected p-value < 0.05).
Impact of Season and UVR on Coral-Associated Bacterial Communities
The bacterial communities associated with Acropora muricata were highly distinct from those present in the surrounding seawater (p < 0.001; Figure 3, File S1). The coral microbiota was consistently dominated by members of the Hahellaceae genus Endozoicomonas and to a lesser extent by Alteromonadaceae and Flammeovirgaceae (Figure 4). These assemblages were not impacted by UVR, but did change between the seasons (Figure 3; Table S2; p = 0.0079). Alpha diversity and richness were similarly impacted, but the evenness remained the same between summer and winter (Table 1; Table S3; p = 0.0142). The relative contribution of Rhodospirillaceae was high in a few winter samples, while members of the Rhodobacteraceae were increased in a few summer samples (Figure 4), which caused a reduction in Endozoicomonas abundances in these samples. Despite the apparent variability in microbiota composition between samples, no differences in sample dispersion were observed between the UVR and no UVR conditions at either season. To assess which OTUs were primarily responsible for the seasonal shift in microbiome composition, differential abundance analysis was performed (output can be found in File S1). Results showed significant effects on various bacteria, particularly 25 OTUs varied significantly in abundance between summer and winter (Figure 5, Table S4, and Figure S1). Overall, the abundance of four Endozoicomonas OTUs, one Thalassospira (Rhodospirillaceae) OTU, one cyanobacterium OTU and two Unassigned OTUs were significantly reduced in summer compared to winter. In contrast, four Cyanobacterium OTUs and two Thalassotalea (Alteromonadales) OTUs along with OTUs belonging to a range of bacterial taxa [e.g., Candidatus Amoebophilus (Flammeovirgaceae), LWSR-14 (Rickettsiales), Paracoccus (Rhodobacteraceae)] were significantly more abundant in summer.
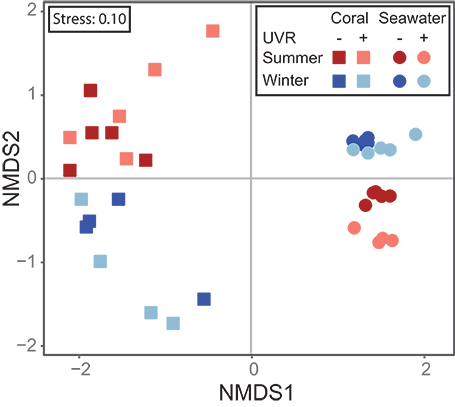
Figure 3. Non-Metric Dimensional Scaling (nMDS) plot showing the (1) seasonal differences in the beta diversity of the coral microbiota, (2) seasonal changes in the beta diversity in seawater microbial communities, and (3) the effect of UVR on the beta diversity in seawater microbial communities at both seasons.
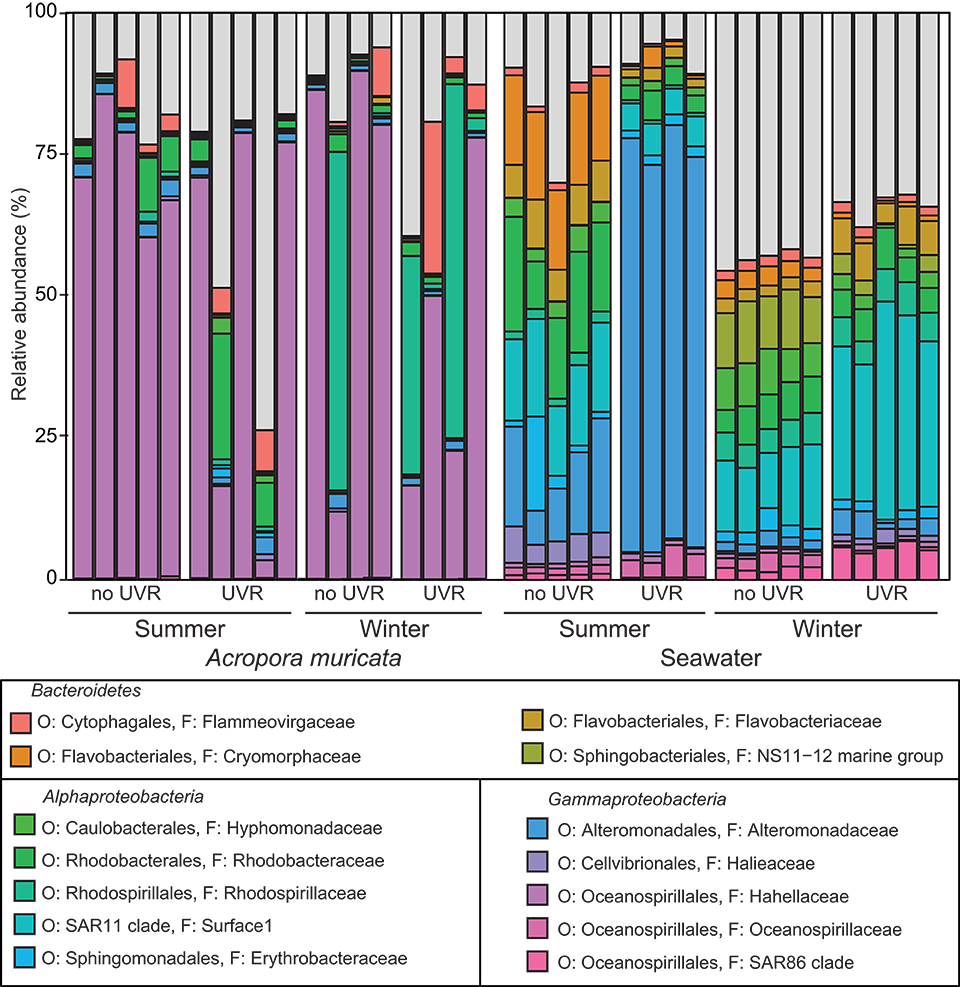
Figure 4. Relative abundance of the major bacterial families present in the A. muricata holobiont and the surrounding seawater (>1% of the total community) under ambient or no UV radiation at two contrasting seasons (summer and winter).
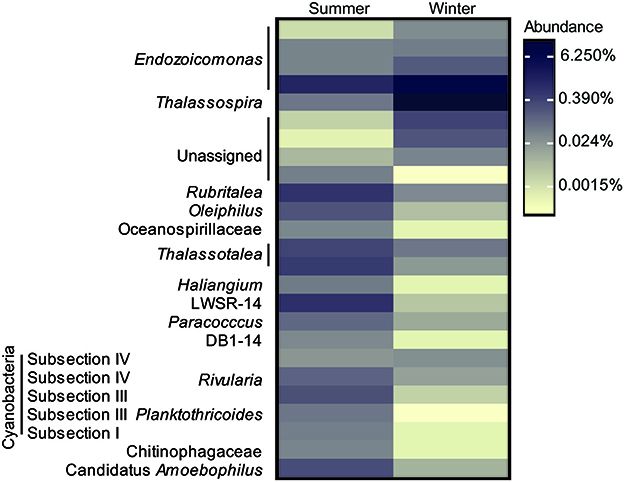
Figure 5. Heatmap overview of bacterial OTUs associated with A. muricata that are differentially abundant between the summer and winter season under ambient UVR conditions.
Impact of Season and UVR on Seawater Microbial Communities
Flow cytometry analyses of the seawater surrounding the coral fragments revealed that the abundances of algae and virus-like particles (VLP) were significantly increased in summer compared to winter, but no effect of UVR level was observed (Figures 6A,B; Table S1). Bacterial abundances showed a seasonal trend, increasing in summer, but our condition comparisons did not show this effect under ambient UVR conditions (Figure 6C; Table S1). The ratio between VLPs and bacteria was approximately 2.7-fold higher in summer (Figure 6D; Table S1).
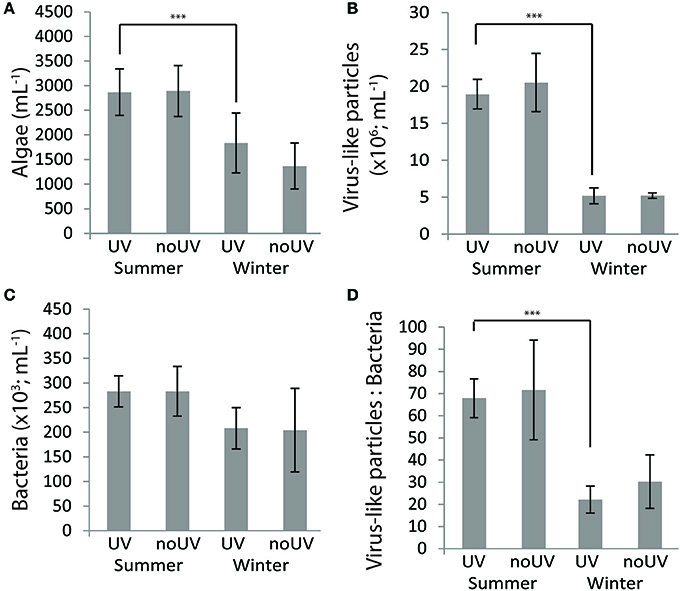
Figure 6. Concentrations of (A) algae, (B) viruse-like particles (VLP) and (C) bacteria and (D) the ratio VLP:bacteria in the seawater under ambient and no UVR conditions at two contrasting seasons (summer and winter). Data are the mean and standard deviations of five biological replicates. Comparisons indicated with ***are significantly different (p < 0.05).
The diversity of the seawater microbial communities (Table 1 – alpha diversity metrics), however, was highly influenced by both season and UVR level, showing interactive effects of these two factors (Figure 3; Table S5—beta diversity: p = 0.0001; Table S6—alpha diversity: p = 0.0001). Interestingly, alpha diversity (richness, evenness and diversity indices) was significantly reduced by UVR in summer (Table S6; p = 0.0017), but increased in winter (Table S6; p = 0.0002). Similarly, the alpha diversity under natural UVR conditions was reduced in summer compared to winter (Table S6; p = 0.0001). No significant differences in the dispersion of samples within treatment groups were observed between the UVR and no UVR conditions at either season. Several bacterial taxa made up the vast majority of the community in both seasons and under UVR treatments (Figure 4), but obvious changes were observed. Differential abundance analysis was used to assess which taxa contributed significantly to these differences in seawater community structure between (1) UVR and no UVR exposure in winter, (2) UVR and no UVR exposure in summer, and (3) UVR exposure in winter and summer (output can be found in Table S7 and File S1). In winter, UVR exposure increased members of the Flavobacteriales and SAR11 clade, while reducing Bdellovibrionales, Alteromonadales, Caulobacterales, Cytophagales and DB1-14 (Figure 4; Figure S2). Interestingly, the abundance of various Oceanospirillales, Rhodospirillales, Rhodobacterales, and Rickettsiales OTUs were inversely impacted by UVR [i.e., the abundance of some OTUs was reduced under UVR, resulting in an increase in relative abundance of other OTUs (Figure S2)]. In summer, members of the order Alteromonadales (particularly genera Marinobacter and Alteromonas) were highly abundant under UVR, which resulted in a significant reduction in the relative abundance of SAR11, Rhodobacterales, Pseudomonadales, Flavobacteriales, Cytophagales, Phycisphaerales, and Oceanospirillales (Figure 4; Figure S3). Differences between seawater bacterial communities under natural UVR levels in summer (high UVR) and winter (low UVR) (Figure 4; Figure S4), showed highly similar patterns as ambient UVR and no UVR exposure treatments, respectively (Figure 4; Figures S2, S3).
Discussion
In this study, we assessed the impact of UV radiation on the physiology and the microbiome of the scleractinian coral A. muricata at two contrasting seasons. Our findings show that seasonality in the coral holobiont composition and functioning is to some extend driven by UV radiation but primarily by other environmental factors. On the contrary, large seasonal and UVR-induced variations were observed in the seawater microbial communities, which was overall dominated by bacterial taxa known to degrade and/or grow on organic matter.
Seasonality Has Higher Impact on Coral Holobiont Than UV Radiation
In the Acropora muricata holobiont, the coral host and associated bacterial communities were only affected by seasonal changes, whereas the algal endosymbiont Symbiodinium was also impacted by UVR exposure. The effect of UVR exposure on Symbiodinium was, however, in part seasonally dependent and limited to changes in the cellular concentration of chlorophyll a and in the photosynthetic efficiency (rETRmax). In winter, exposure to naturally moderate levels of UVR enhanced the rETRmax of A. muricata. Utilization of moderate doses of UVR (mostly UV-A) as a source of energy for photosynthesis has been previously observed in phytoplankton species (Gao et al., 2007; Wu et al., 2009), and our results suggest this may also be applicable to Symbiodinium in corals. On the contrary, in summer, exposure to naturally high UVR levels led to a significant reduction in Symbiodinium photosynthetic efficiency, which may have been compensated for through an increase in chlorophyll content, as previously observed (Cardini et al., 2015). Previous studies have also shown that the lowest photosynthetic yield (Fv/Fm) or rETRmax values of diverse scleractinian coral species coincide with the highest irradiance and temperature levels on the reef, and are caused by damage to the photosystems (Fitt et al., 2000; Warner et al., 2002; Winter et al., 2016). The impairment of the photosynthetic capacities of Symbiodinium, and thereby energy acquisition by A. muricata in summer, may explain the lower coral tissue biomass (i.e., protein concentration) observed compared to winter. However, this effect may also have been exacerbated by enhanced respiratory metabolism at high temperatures as previously observed in various coral species (Fitt et al., 2000).
Despite having reduced energy reserves, calcification rates were enhanced in A. muricata in summer, which corresponds with reports on a positive linear relation between coral calcification and seawater temperature between 23° and 28°C (Lough and Barnes, 2000; Silverman et al., 2007). Although UVR has been shown to decrease calcification rates in several coral species (Jokiel and York, 1982; Gleason and Wellington, 1993; Torres-Pérez and Armstrong, 2012), calcification rates of Acropora muricata in this study as well as Acropora validata and Porites compressa in previous studies (Glynn et al., 1993; Kuffner, 2002) were not impacted by UVR. The different effects of UVR on coral skeletal growth may be linked to the amount of energy acquired by the coral via its algal endosymbiont Symbiodinium, and dedicated to calcification (Courtial et al., 2017). Overall, our data suggest that A. muricata has two seasonally driven phenotypes: thin tissue holobionts with faster calcification rates in summer, and thicker tissue but slower calcifying holobionts in winter.
Seasonal effects were also observed in the coral-associated bacterial communities, but these were not impacted by UVR exposure. The presence of UVR-filtering “sunscreen” molecules (e.g., mycosporine-like amino acids (MAAs), fluorescent proteins) in coral tissue and/or mucus (Shick and Dunlap, 2002) may protect the microbial community from the damaging effects of UVR. However, we cannot exclude that the bacteria were affected in their physiology, as observed in the algal symbiont Symbiodinium. Few studies have assessed seasonal changes in coral-associated microbial communities thus far, but have generally reported highly different profiles between winter and summer in scleractinian corals (Hong et al., 2009; Ceh et al., 2011; Chen et al., 2011; Kimes et al., 2013; Sharp et al., 2017; Cai et al., 2018), but not in octocorals (van de Water et al., 2018a). Changes in the coral microbiota may be caused by fluctuations in seawater temperature, rainfall and sunlight intensity (Chen et al., 2011) as well as nutrient availability in seawater (Hernández-Zulueta et al., 2016). A recent study that investigated the effects of temperature, nutrient pollution and algal cover on the microbiomes of three coral species, however, found that temperature variation explained differences in microbial community structure over time better than other measured seasonal parameters (Zaneveld et al., 2016). In summer, A. muricata harbored a significantly higher abundance of several Cyanobacterium OTUs, which might play a role in providing new nitrogen to the coral holobiont (Bednarz et al., 2017), since many cyanobacteria are capable of fixing nitrogen (i.e., diazotrophs). Fixation of dinitrogen by these bacteria is an important functional process for corals thriving in oligotrophic tropical environments as they can provide up to 15% of new nitrogen to corals (Bednarz et al., 2017). Increases in the abundance and diversity of nitrogen-fixing bacteria in coral tissue has been observed previously under elevated temperatures (Santos et al., 2014; Cardini et al., 2015, 2016) and between winter and summer (Cai et al., 2018). We also observed a higher abundance of a Candidatus Amoebophilus bacterium in summer compared to winter. Previously, bacteria belonging to this taxon were reported to be part of the core microbiome of 3 coral species (Apprill et al., 2016). This bacterium is a Bacteroidetes-affiliated intracellular symbionts of amoebae and may be an endosymbiont of a protist host within coral tissues (Apprill et al., 2016).
In summer, the coral-associated microbial community also contained a higher abundance of bacteria belonging to the genus Thalassotalea (previously Thalassomonas), which have previously been found in increased numbers in diseased and stressed corals (Sunagawa et al., 2009; Weynberg et al., 2016). In fact, T. loyana has been identified as the causative agent of a white plague-like disease (Thompson, 2006). Such a shift toward a potentially more pathogenic coral microbiota in summer may affect coral health and increase the susceptibility of the host to disease (Bourne and Munn, 2005; Ritchie, 2006; Bourne et al., 2008). Higher temperatures may directly affect bacterial growth and metabolism, or indirectly alter bacterial antibiotic production and bacteria–bacteria interactions (Rypien et al., 2010). Elevated temperatures may also impair a coral's defense capability (van de Water et al., 2015), leading to a reduced ability of the coral host to regulate its microbiome, or require the coral to invest in its innate immune system (van de Water et al., 2018a) and protective surface mucosal layer (Pratte and Richardson, 2014) to maintain a healthy microbiome, thereby potentially depleting its energy stores. As the immunocompetence of an animal largely depends on its energy reserves, the higher occurrence of potentially pathogenic OTUs in A. muricata in summer may be linked to a reduced fitness of the holobiont, as evidenced by the lower tissue biomass (proteins), and Symbiodinium photosynthetic efficiency compared to winter. In summer, we also observed a lower abundance of Thalassospira, known as potential symbionts of ctenophores (Hao, 2014), and four OTUs of Endozoicomonas, a genus commonly found in healthy corals (Bayer et al., 2013a,b; Neave et al., 2017; van de Water et al., 2017, 2018b), as well as in a wide range of other marine invertebrates (Forget and Juniper, 2013; Fiore et al., 2015; Katharios et al., 2015). Although their exact functions are unknown, both Thalassospira and Endozoicomonas may be involved in host health through nutrient acquisition and provision, and in structuring of the host microbial community (Hao, 2014; reviewed by Neave et al., 2016). Our results on the abundance of Endozoicomonas, and the observed changes in host physiology between summer and winter, however, do not appear to provide additional insights into the potential role of Endozoicomonas in corals.
Seawater Bacterial Communities Are Impacted by Both Season and UVR Exposure
Most of the organic carbon and nitrogen in seawater of Nouméa lagoon was in the dissolved form, attesting the oligotrophic nature of these tropical waters, with few large size suspended particles in the water (Biddanda et al., 2001). However, increased rainfall as well as higher diazotroph abundance and activity (Berthelot et al., 2015) may have been responsible for the higher amount of dissolved nitrogen in seawater in summer. Combined with the increased seawater temperatures, this may have driven the increased microbial abundances observed in summer (Moriarty et al., 1985). For example, when more dissolved inorganic nitrogen (DIN) is available, autotrophs tend to retain the photosynthetically-acquired carbon for their growth and proliferation, rather than releasing it in seawater as organic carbon (Naumann et al., 2010; Mueller et al., 2016). However, these DIN-repleted autotrophs may have released excess nitrogen (Naumann et al., 2010; Wymore et al., 2015). In addition, high temperatures and microbial abundances may have increased microbial respiration rates, leading to lower DOC levels in the water in summer. Taken together, these observations may explain the observed inverse relationship between DOC and DN in seawater. Only the abundances of bacteria didn't significantly change between summer and winter. As the number of VLPs, and concomitantly the ratio VLP:bacteria, was also higher in summer and most viruses in seawater are bacteriophages, our results suggest ongoing lytic infections of bacterioplankton and likely higher temporal dynamics within seawater microbial communities in summer (Parikka et al., 2017). Higher levels of UVR and seawater temperatures have previously been found to induce VLP production (42 and 33% of the cases examined, respectively) (Jiang and Paul, 1996). As we did not find an effect of UVR on VLP abundances or the ratio VLP:bacteria, this suggests that the seasonal differences observed here are likely related to seawater temperatures. VLPs have indeed been coupled to the dynamics of the coral reef bacterioplankton community on spatial scales (Seymour et al., 2005) and viral predation has been shown to remove 24–367% of the bacterial standing stock on coral reefs every day, releasing up to 62 micrograms of organic carbon per liter of seawater (Payet et al., 2014). As such, viral lytic infections may also explain the lower organic carbon content of the seawater in summer, and reflects the high abundant and dynamic microbial community within coral reefs.
Seawater bacterial communities were dominated by bacterial taxa known to degrade and/or grow on dissolved and particulate organic matter, or transparent exopolymeric particles released by phytoplankton, including Flavobacteriales and Rhodobacterales (Taylor et al., 2014), as well as SAR11, Alteromonadales and Oceanospirillales (Bergauer et al., 2018). It should, however, be noted that SAR11 may have been underrepresented in our dataset because of the 806r reverse primer used to construct the sequencing libraries, rather than the 806rb primer which better reflects SAR11 abundances (Apprill et al., 2015). The higher nitrogen levels and temperatures in summer may have in part driven the differences in seawater bacterial community diversity between the two seasons. Interestingly, however, the effect of UVR level was different between the seasons. This observation suggests that seawater bacterioplankton communities is very dynamic and may change within a few hours depending on the environmental conditions. Overall, UVR significantly reduced the bacterial community diversity, possibly due to UVR-induced inhibition of bacterial growth (Llabrés and Agustí, 2010; Korbee et al., 2012; Carrillo et al., 2015) linked to DNA photodamage (Jeffrey et al., 2000; Alonso-Sáez et al., 2006), However, patterns appeared to be relatively consistent, showing various bacterial taxa consistently increase or decrease in relative abundance under UVR exposure conditions at both seasons as well as between summer (high UVR levels) and winter (low UVR levels). Although the abundance of most of the main bacterial groups decreased in response to UVR, members of the order Alteromonadales (genera Marinobacter and Alteromonas) were significantly higher, particularly in summer, which may explain the reduced community diversity and suggests that these bacteria may be more resistant to the high summer UVR levels on the reef than other species. UVR resistance of Altermonadales bacteria has indeed been shown in experimental sterilization trails, showing rapid recolonization of the seawater and surfaces by bacteria from this order (particularly Marinobacter) after UVR sterilization treatment (Hess-Erga et al., 2010). As such, this may explain why Altermonadales dominated the seawater microbial community under natural summer UVR levels, while other main groups such as Flavobacteriales, Rhodobacterales and SAR11, increased in abundance, and likely outcompeted Alteromonadales, when UVR levels were reduced experimentally or in winter. Alphaproteobacteria (Rhodobacterales and SAR11) are indeed known to be relatively sensitive to UVR, even in temperate environments (Alonso-Sáez et al., 2006) where UVR levels are lower than in tropical environments. In winter, however, the abundance of SAR11 was higher under ambient UVR conditions. This additional light in winter may be required for the activity of the ATP-generating proton pump proteorhodopsin in SAR11 (Lami and Kirchman, 2014), and may provide these bacteria an energetic advantage under moderate/low UVR levels. In general, our results confirm significant seasonal differences in the abundances and composition of the bacterioplankton communities of seawater, and that both UVR and organic matter concentrations are important determinants of the community structure.
Concluding Remarks
In this study, we provide an overview of the seasonal impact of UVR on the physiology and microbiome of the scleractinian coral Acropora muricata. In general, we observed two seasonal coral holobiont phenotypes: (1) a fast calcifying, but lower photosynthesizing and animal tissue biomass phenotype in summer, and (2) a slow calcifying, but higher photosynthesizing and biomass phenotype in winter. The impact of ambient UVR levels on the coral holobiont was limited, however, and affected only the photosynthetic process in Symbiodinium, particularly in summer. Although the coral microbiota showed seasonal differences, it was shielded from UVR impacts, unlike the microbial communities in the seawater surrounding the coral. Overall, our study suggests that UVR has a limited impact on coral holobiont composition and functioning, and that seasonal changes in the holobiont are thus mostly driven by other environmental factors.
Author Contributions
LC, FH, and CF-P designed the experiment. LC and FH conducted the experiment. LC processed the samples and performed physiological analyses. SJ performed the flow cytometry analyses. JvdW analyzed the bacterial community data. All authors contributed to the writing and editing of the manuscript.
Conflict of Interest Statement
The authors declare that the research was conducted in the absence of any commercial or financial relationships that could be construed as a potential conflict of interest.
Acknowledgments
The authors would like to thank R. Rodolfo Metalpa and C. Rottier for laboratory assistance, C. Payri, director of the ENTROPIE UMR, for scientific support and Mr. R. Farman and the technical staff of the Aquarium des lagons (Nouméa, New Caledonia), for hosting the tanks and providing technical help during the experimental setup. We also would like to thank N. Wabete and M. Le Gall (Institut Français de Recherche pour l'Exploitation de la Mer) for their collaboration in lab analysis. JvdW would like to thank the Paul Hamel Foundation for financial support.
Supplementary Material
The Supplementary Material for this article can be found online at: https://www.frontiersin.org/articles/10.3389/fmars.2018.00275/full#supplementary-material
References
Allers, E., Niesner, C., Wild, C., and Pernthaler, J. (2008). Microbes enriched in seawater after addition of coral mucus. Appl. Environ. Microbiol. 74, 3274–3278. doi: 10.1128/AEM.01870-07
Alonso-Sáez, L., Gasol, J. M., Lefort, T., Hofer, J., and Sommaruga, R. (2006). Effect of natural sunlight on bacterial activity and differential sensitivity of natural bacterioplankton groups in Northwestern Mediterranean coastal waters. Appl. Environ. Microbiol. 72, 5806–5813. doi: 10.1128/AEM.00597-06
Anderson, M., Gorley, R. N., and Clarke, R. K. (2008). Permanova+ for Primer: Guide to Software and Statistical Methods Google Scholar. Plymouth: PRIMER-E.
Apprill, A., McNally, S., Parsons, R., and Weber, L. (2015). Minor revision to V4 region SSU rRNA 806R gene primer greatly increases detection of SAR11 bacterioplankton. Aquat. Microb. Ecol. 75, 129–137. doi: 10.3354/ame01753
Apprill, A., Weber, L. G., and Santoro, E. (2016). Distinguishing between microbial habitats unravels ecological complexity in coral microbiomes. mSystems 1:e00143-16. doi: 10.1128/mSystems.00143-16
Bayer, T., Arif, C., Ferrier-Pagès, C., Zoccola, D., Aranda, M., and Voolstra, C. R. (2013a). Bacteria of the genus Endozoicomonas dominate the microbiome of the Mediterranean gorgonian coral Eunicella cavolini. Mar. Ecol. Prog. Ser. 479, 75–84. doi: 10.3354/meps10197
Bayer, T., Neave, M. J., Alsheikh-Hussain, A., Aranda, M., Yum, L. K., Mincer, T., et al. (2013b). The microbiome of the Red Sea coral Stylophora pistillata is dominated by tissue-associated Endozoicomonas bacteria. Appl. Environ. Microbiol. 79, 4759–4762. doi: 10.1128/AEM.00695-13
Bednarz, V. N., Grover, R., Maguer, J.-F., Fine, M., and Ferrier-Pagès, C. (2017). The assimilation of diazotroph-derived nitrogen by scleractinian corals depends on their metabolic status. MBio 8:e02058-16. doi: 10.1128/mBio.02058-16
Bergauer, K., Fernandez-Guerra, A., Garcia, J. A. L., Sprenger, R. R., Stepanauskas, R., Pachiadaki, M. G., et al. (2018). Organic matter processing by microbial communities throughout the Atlantic water column as revealed by metaproteomics. Proc. Natl. Acad. Sci. U.S.A. 115, E400–E408. doi: 10.1073/pnas.1708779115
Berthelot, H., Moutin, T., L'Helguen, S., Leblanc, K., Hélias, S., Grosso, O., et al. (2015). Dinitrogen fixation and dissolved organic nitrogen fueled primary production and particulate export during the VAHINE mesocosm experiment (New Caledonia lagoon). Biogeosciences 12, 4099–4112. doi: 10.5194/bg-12-4099-2015
Biddanda, B., Ogdahl, M., and Cotner, J. (2001). Dominance of bacterial metabolism in oligotrophic relative to eutrophic waters. Limnol. Oceanogr. 46, 730–739. doi: 10.4319/lo.2001.46.3.0730
Bourne, D. G., and Munn, C. B. (2005). Diversity of bacteria associated with the coral Pocillopora damicornis from the Great Barrier Reef. Environ. Microbiol. 7, 1162–1174. doi: 10.1111/j.1462-2920.2005.00793.x
Bourne, D., Iida, Y., Uthicke, S., and Smith-Keune, C. (2008). Changes in coral-associated microbial communities during a bleaching event. ISME J. 2, 350–363. doi: 10.1038/ismej.2007.112
Brown, B. E., Dunne, R. P., Ambarsari, I., Le Tissier, M. D. A., and Satapoomin, U. (1999). Seasonal fluctuations in environmental factors and variations in symbiotic algae and chlorophyll pigments in four Indo-Pacific coral species. Mar. Ecol. Prog. Ser. 191, 53–69.
Browne, N. K., Tay, J. K. L., Low, J., Larson, O., and Todd, P. A. (2015). Fluctuations in coral health of four common inshore reef corals in response to seasonal and anthropogenic changes in water quality. Mar. Environ. Res. 105, 39–52. doi: 10.1016/j.marenvres.2015.02.002
Cai, L., Zhou, G., Tong, H., Tian, R.-M., Zhang, W., Ding, W., et al. (2018). Season structures prokaryotic partners but not algal symbionts in subtropical hard corals. Appl. Microbiol. Biotechnol. 102, 4963–4973. doi: 10.1007/s00253-018-8909-5
Caporaso, J. G., Kuczynski, J., Stombaugh, J., Bittinger, K., Bushman, F. D., Costello, E. K., et al. (2010). QIIME allows analysis of high-throughput community sequencing data. Nat. Methods 7, 335–336. doi: 10.1038/nmeth.f.303
Cardini, U., Bednarz, V. N., Naumann, M. S., van Hoytema, N., Rix, L., Foster, R. A., et al. (2015). Functional significance of dinitrogen fixation in sustaining coral productivity under oligotrophic conditions. Proc. Biol. Sci. 282:20152257. doi: 10.1098/rspb.2015.2257
Cardini, U., van Hoytema, N., Bednarz, V. N., Rix, L., Foster, R. A., Al-Rshaidat, M. M., et al. (2016). Microbial dinitrogen fixation in coral holobionts exposed to thermal stress and bleaching. Environ. Microbiol. 18, 2620–2633. doi: 10.1111/1462-2920.13385
Carrillo, P., Medina-Sánchez, J. M., Herrera, G., Durán, C., Segovia, M., Cortés, D., et al. (2015). Interactive effect of UVR and phosphorus on the coastal phytoplankton community of the Western Mediterranean Sea: unravelling eco-physiological mechanisms. PLoS ONE 10:e0142987. doi: 10.1371/journal.pone.0142987
Ceh, J., Van Keulen, M., and Bourne, D. G. (2011). Coral-associated bacterial communities on Ningaloo Reef, Western Australia. FEMS Microbiol. Ecol. 75, 134–144. doi: 10.1111/j.1574-6941.2010.00986.x
Chen, C.-P., Tseng, C.-H., Chen, C. A., and Tang, S.-L. (2011). The dynamics of microbial partnerships in the coral Isopora palifera. ISME J. 5, 728–740. doi: 10.1038/ismej.2010.151
Clarke, A. (1988). Seasonality in the Antarctic marine environment. Comp. Biochem. Physiol. B. 90, 461–473.
Clarke, K. R., and Gorley, R. N. (2006). Primer v6: User Manual/Tutorial. Plymouth: Primer-E Plymouth Marine Laboratory.
Coma, R., Ribes, M., Gili, J.-M., and Zabala, M. (2000). Seasonality in coastal benthic ecosystems. Trends Ecol. Evol. 15, 448–453. doi: 10.1016/S0169-5347(00)01970-4
Conan, P., Joux, F., Torréton, J., Pujo-Pay, M., Douki, T., Rochelle-Newall, E., et al. (2008). Effect of solar ultraviolet radiation on bacterio- and phytoplankton activity in a large coral reef lagoon (southwest New Caledonia). Aquat. Microb. Ecol. 52, 83–98. doi: 10.3354/ame01204
Courtial, L., Roberty, S., Shick, J. M., Houlbrèque, F., and Ferrier-Pagès, C. (2017). Interactive effects of ultraviolet radiation and thermal stress on two reef-building corals. Limnol. Oceanogr. 62, 1000–1013. doi: 10.1002/lno.10481
Crossland, C. J. (1984). Seasonal variations in the rates of calcification and productivity in the coral Acropora formosa on a high-latitude reef. Mar. Ecol. Prog. Ser. Oldend. 15, 135–140.
Dandan, S. S., Falter, J. L., Lowe, R. J., and McCulloch, M. T. (2015). Resilience of coral calcification to extreme temperature variations in the Kimberley region, northwest Australia. Coral Reefs 34, 1151–1163. doi: 10.1007/s00338-015-1335-6
Edgar, R. C. (2010). Search and clustering orders of magnitude faster than BLAST. Bioinformatics 26, 2460–2461. doi: 10.1093/bioinformatics/btq461
Edgar, R. C., Haas, B. J., Clemente, J. C., Quince, C., and Knight, R. (2011). UCHIME improves sensitivity and speed of chimera detection. Bioinformatics 27, 2194–2200. doi: 10.1093/bioinformatics/btr381
Edmunds, P. J., and Davies, P. S. (1986). An energy budget for Porites porites (Scleractinia). Mar. Biol. 92, 339–347. doi: 10.1007/BF00304690
Fiore, C. L., Labrie, M., Jarett, J. K., and Lesser, M. P. (2015). Transcriptional activity of the giant barrel sponge, Xestospongia muta Holobiont: molecular evidence for metabolic interchange. Front. Microbiol. 6:364. doi: 10.3389/fmicb.2015.00364
Fitt, W. K., McFarland, F. K., Warner, M. E., and Chilcoat, G. C. (2000). Seasonal patterns of tissue biomass and densities of symbiotic dinoflagellates in reef corals and relation to coral bleaching. Limnol. Oceanogr. 3, 677–685. doi: 10.4319/lo.2000.45.3.0677
Forget, N. L., and Juniper, S. (2013). Free-living bacterial communities associated with tubeworm (Ridgeia piscesae) aggregations in contrasting diffuse flow hydrothermal vent habitats at the Main Endeavour Field, Juan de Fuca Ridge. Microbiology Open 2, 259–275.
Gao, K., Wu, Y., Li, G., Wu, H., Villafane, V. E., and Helbling, E. W. (2007). Solar UV radiation drives CO2 fixation in marine phytoplankton: a double-edged sword. Plant Physiol. 144, 54–59. doi: 10.1104/pp.107.098491
Garren, M., and Azam, F. (2012). Corals shed bacteria as a potential mechanism of resilience to organic matter enrichment. ISME J. 6, 1159–1165. doi: 10.1038/ismej.2011.180
Gleason, D. F., and Wellington, G. M. (1993). Ultraviolet radiation and coral bleaching. Nature 365, 836–838.
Glynn, P. W., Imai, R., Sakai, K., and Nakano, Y. Y. K. (1993). “Experimental responses of Okinawan (Ryukyu Island, Japan) reef corals to high seawater temperature and UV radiation,” in Proceedings of Seventh International Coral Reef Symposium, ed R. RH (Mangilao: University of Guam Press), 27–37.
Gooday, A. J., Turley, C. M., and Allen, J. A. (1990). Responses by benthic organisms to inputs of organic material to the ocean floor: a review and discussion. Philos. Trans. R. Soc. London A Math. Phys. Eng. Sci. 331, 119–138.
Häder, D.-P., Williamson, C. E., Wängberg, S.-Å., Rautio, M., Rose, K. C., Gao, K., et al. (2015). Effects of UV radiation on aquatic ecosystems and interactions with other environmental factors. Photochem. Photobiol. Sci. 14, 108–126. doi: 10.1039/c4pp90035a
Hernández-Zulueta, J., Araya, R., Vargas-Ponce, O., Díaz-Pérez, L., Rodríguez-Troncoso, A. P., Ceh, J., et al. (2016). First deep screening of bacterial assemblages associated with corals of the Tropical Eastern Pacific. FEMS Microbiol. Ecol. 92:fiw196. doi: 10.1093/femsec/fiw196
Hess-Erga, O.-K., Blomvågnes-Bakke, B., and Vadstein, O. (2010). Recolonization by heterotrophic bacteria after UV irradiation or ozonation of seawater; a simulation of ballast water treatment. Water Res. 44, 5439–5449. doi: 10.1016/j.watres.2010.06.059
Hoegh-Guldberg, O. (1999). Climate change, coral bleaching and the future of the world's coral reefs. Mar. Freshw. Res. 50, 839–866. doi: 10.1071/MF99078
Hoegh-Guldberg, O., Poloczanska, E. S., Skirving, W., and Dove, S. (2017). Coral reef ecosystems under climate change and ocean acidification. Front. Mar. Sci. 4:158. doi: 10.3389/fmars.2017.00158
Hong, M.-J., Yu, Y.-T., Chen, C. A., Chiang, P.-W., and Tang, S.-L. (2009). Influence of species specificity and other factors on bacteria associated with the coral Stylophora pistillata in Taiwan. Appl. Environ. Microbiol. 75, 7797–7806. doi: 10.1128/AEM.01418-09
Hoogenboom, M., Beraud, E., and Ferrier-Pagès, C. (2010). Relationship between symbiont density and photosynthetic carbon acquisition in the temperate coral Cladocora caespitosa. Coral Reefs 29, 21–29. doi: 10.1007/s00338-009-0558-9
Hughes, T. P., Kerry, J., Álvarez-Noriega, M., Álvarez-Romero, J., Anderson, K., Baird, A., et al. (2017). Global warming and recurrent mass bleaching of corals. Nature 543, 373–377. doi: 10.1038/nature21707
Jacquet, S., Dorigo, U., and Personnic, S. (2013). Flow Cytometry: Principles, Methodology and Applications. Hauppauge, NY: Nova Science Publishers.
Jeffrey, S. W., and Humphrey, G. F. (1975). New spectrophotometric equations for determining chlorophylls a, b, c1 and c2 in higher plants, algae and natural phytoplankton. Biochem. Physiol. Pflanz 167, 191–194. doi: 10.1016/0022-2860(75)85046-0
Jeffrey, W. H., Kase, J. P., and Wilhelm, S. W. (2000). “UV radiation effects on heterotrophic bacterioplankton and viruses in marine ecosystems,” in The Effects of UV Radiation in the Marine Environment, eds S. De Mora, S. Demers and M. Vernet (Cambridge: Cambridge University Press).
Jiang, S., and Paul, J. (1996). Occurrence of lysogenic bacteria in marine microbial communities as determined by prophage induction. Mar. Ecol. Prog. Ser. 142, 27–38. doi: 10.3354/meps142027
Jokiel, P. L., and York, H. Jr. (1982). Solar ultraviolet photobiology of the reef coral Pocillopora damicornis and symbiotic zooxanthellae. Bull. Mar. Sci. 32, 301–315.
Katharios, P., Seth-Smith, H. M. B., Fehr, A., Mateos, J. M., Qi, W., Richter, D., et al. (2015). Environmental marine pathogen isolation using mesocosm culture of sharpsnout seabream: striking genomic and morphological features of novel Endozoicomonas sp. Sci. Rep. 5:17609. doi: 10.1038/srep17609
Kelly, L. W., Williams, G. J., Barott, K. L., Carlson, C. A., Dinsdale, E. A., Edwards, R. A., et al. (2014). Local genomic adaptation of coral reef-associated microbiomes to gradients of natural variability and anthropogenic stressors. Proc. Natl. Acad. Sci. U.S.A. 111, 10227–10232. doi: 10.1073/pnas.1403319111
Kimes, N. E., Johnson, W. R., Torralba, M., Nelson, K. E., Weil, E., and Morris, P. J. (2013). The Montastraea faveolata microbiome: ecological and temporal influences on a Caribbean reef-building coral in decline. Environ. Microbiol. 15, 2082–2094. doi: 10.1111/1462-2920.12130
Kooperman, N., Ben-Dov, E., Kramarsky-Winter, E., Barak, Z., and Kushmaro, A. (2007). Coral mucus-associated bacterial communities from natural and aquarium environments. FEMS Microbiol. Lett. 276, 106–113. doi: 10.1111/j.1574-6968.2007.00921.x
Korbee, N., Carrillo, P., Mata, M. T., Rosillo, S., Medina-Sánchez, J. M., and Figueroa, F. L. (2012). Effects of ultraviolet radiation and nutrients on the structure–function of phytoplankton in a high mountain lake. Photochem. Photobiol. Sci. 11, 1087–1098. doi: 10.1039/c2pp05336e
Korbee, N., Teresa Mata, M., and Figueroa, F. L. (2010). Photoprotection mechanisms against ultraviolet radiation in Heterocapsa sp.(Dinophyceae) are influenced by nitrogen availability: mycosporine-like amino acids vs. xanthophyll cycle. Limnol. Oceanogr. 55, 899–908. doi: 10.4319/lo.2010.55.2.0899
Kuffner, I. B. (2002). Effects of ultraviolet radiation and water motion on the reef coral, Porites compressa Dana: a transplantation experiment. J. Exp. Mar. Bio. Ecol. 270, 147–169. doi: 10.1016/S0022-0981(02)00031-X
Lami, R., and Kirchman, D. (2014). Diurnal expression of SAR11 proteorhodopsin and 16S rRNA genes in coastal North Atlantic waters. Aquat. Microb. Ecol. 73, 185–194. doi: 10.3354/ame01716
Lesser, M. P. (2000). Depth-dependent photoacclimatization to solar ultraviolet radiation in the Caribbean coral Montastraea faveolata. Mar. Ecol. Prog. Ser. 192, 137–151. doi: 10.3354/meps192137
Lesser, M. P., and Lewis, S. (1996). Action spectrum for the effects of UV radiation on photosynthesis in the hermatypic coral Pocillopora damicornis. Mar. Ecol. Prog. Ser. 134, 171–177.
Lesser, M. P., Stochaj, W. R., Tapley, D. W., and Shick, J. M. (1990). Coral Reefs effects of irradiance, ultraviolet radiation, and temperature. Coral Reefs 8, 225–232. doi: 10.1007/BF00265015
Li, J., Chen, Q., Long, L.-J., Dong, J.-D., Yang, J., and Zhang, S. (2014). Bacterial dynamics within the mucus, tissue and skeleton of the coral Porites lutea during different seasons. Sci. Rep. 4:7320. doi: 10.1038/srep07320
Littman, R. A., Bourne, D. G., and Willis, B. L. (2010). Responses of coral associated bacterial communities to heat stress differ with Symbiodinium type on the same coral host. Mol. Ecol. 19, 1978–1990. doi: 10.1111/j.1365-294X.2010.04620.x
Littman, R., Willis, B. L., and Bourne, D. G. (2011). Metagenomic analysis of the coral holobiont during a natural bleaching event on the Great Barrier Reef. Environ. Microbiol. Rep. 3, 651–660. doi: 10.1111/j.1758-2229.2010.00234.x
Llabrés, M., Agustí, S., Alonso-Laita, P., and Herndl, G. J. (2010). Synechococcus and Prochlorococcus cell death induced by UV radiation and the penetration of lethal UVR in the Mediterranean Sea. Mar. Ecol. Prog. Ser. 399, 27–37. doi: 10.3354/meps08332
Llabrés, M., and Agustí, S. (2010). Effects of ultraviolet radiation on growth, cell death and the standing stock of Antarctic phytoplankton. Aquat. Microb. Ecol. 59, 151–160. doi: 10.3354/ame01392
Lough, J. M., and Barnes, D. J. (2000). Environmental controls on growth of the massive coral Porites. J. Exp. Mar. Bio. Ecol. 245, 225–243. doi: 10.1016/S0022-0981(99)00168-9
Love, M. I., Huber, W., and Anders, S. (2014). Moderated estimation of fold change and dispersion for RNA-seq data with DESeq2. Genome Biol. 15:550. doi: 10.1186/s13059-014-0550-8
Lyons, M. M., Aas, P., Pakulski, J. D., Van Waasbergen, L., Miller, R. V., Mitchell, D. L., et al. (1998). DNA damage induced by ultraviolet radiation in coral-reef microbial communities. Mar. Biol. 130, 537–543. doi: 10.1007/s002270050274
Madronich, S., McKenzie, R. L., Björn, L. O., and Caldwell, M. M. (1998). Changes in biologically active ultraviolet radiation reaching the Earth's surface. J. Photochem. Photobiol. B Biol. 46, 5–19. doi: 10.1016/S1011-1344(98)00182-1
McMurdie, P. J., and Holmes, S. (2013). phyloseq: an R package for reproducible interactive analysis and graphics of microbiome census data. PLoS ONE 8:e61217. doi: 10.1371/journal.pone.0061217
Mohamed, N. M., Rao, V., Hamann, M. T., Kelly, M., and Hill, R. T. (2008). Monitoring bacterial diversity of the marine sponge Ircinia strobilina upon transfer into aquaculture. Appl. Environ. Microbiol. 74, 4133–4143. doi: 10.1128/AEM.00454-08
Moriarty, D. J. W., Pollard, P. C., and Hunt, W. G. (1985). Temporal and spatial variation in bacterial production in the water column over a coral reef. Mar. Biol. 85, 285–292. doi: 10.1007/BF00393249
Mueller, B., den Haan, J., Visser, P. M., Vermeij, M. J. A., and van Duyl, F. C. (2016). Effect of light and nutrient availability on the release of dissolved organic carbon (DOC) by Caribbean turf algae. Sci. Rep. 6:23248. doi: 10.1038/srep23248
Naumann, M. S., Haas, A., Struck, U., Mayr, C., El-Zibdah, M., and Wild, C. (2010). Organic matter release by dominant hermatypic corals of the Northern Red Sea. Coral Reefs 29, 649–659. doi: 10.1007/s00338-010-0612-7
Neave, M. J., Apprill, A., Ferrier-Pagès, C., and Voolstra, C. R. (2016). Diversity and function of prevalent symbiotic marine bacteria in the genus Endozoicomonas. Appl. Microbiol. Biotechnol. 100, 8315–8324. doi: 10.1007/s00253-016-7777-0
Neave, M. J., Rachmawati, R., Xun, L., Michell, C. T., Bourne, D. G., Apprill, A., et al. (2017). Differential specificity between closely related corals and abundant Endozoicomonas endosymbionts across global scales. ISME J. 11, 186–200. doi: 10.1038/ismej.2016.95
Nelson, C. E., Goldberg, S. J., Kelly, L. W., Haas, A. F., Smith, J. E., Rohwer, F., et al. (2013). Coral and macroalgal exudates vary in neutral sugar composition and differentially enrich reef bacterioplankton lineages. ISME J. 7, 962–979. doi: 10.1038/ismej.2012.161
Oksanen, J., Blanchet, F. G., Kindt, R., Legendre, P., Minchin, P. R., O'hara, R. B., et al. (2011). vegan: Community Ecology Package. R Package version. Available online at: https://cran.r-project.org/web/packages/vegan/vegan.pdf
Parikka, K. J., Le Romancer, M., Wauters, N., and Jacquet, S. (2017). Deciphering the virus-to-prokaryote ratio (VPR): insights into virus-host relationships in a variety of ecosystems. Biol. Rev. 92, 1081–1100. doi: 10.1111/brv.12271
Payet, J. P., McMinds, R., Burkepile, D. E., and Vega Thurber, R. L. (2014). Unprecedented evidence for high viral abundance and lytic activity in coral reef waters of the South Pacific Ocean. Front. Microbiol. 5:493. doi: 10.3389/fmicb.2014.00493
Pratte, Z. A., and Richardson, L. L. (2014). Impacts of temperature increase and acidification on thickness of the surface mucopolysaccharide layer of the Caribbean coral Diploria spp. Coral Reefs 33, 487–496. doi: 10.1007/s00338-013-1115-0
Pratte, Z. A., Richardson, L. L., and Mills, D. K. (2015). Microbiota shifts in the surface mucopolysaccharide layer of corals transferred from natural to aquaria settings. J. Invertebr. Pathol. 125, 42–44. doi: 10.1016/j.jip.2014.12.009
Quast, C., Pruesse, E., Yilmaz, P., Gerken, J., Schweer, T., Yarza, P., et al. (2013). The SILVA ribosomal RNA gene database project: improved data processing and web-based tools. Nucleic Acids Res. 41, D590–D596. doi: 10.1093/nar/gks1219
Ralph, P. J., and Gademann, R. (2005). Rapid light curves: a powerful tool to assess photosynthetic activity. Aquat. Bot. 82, 222–237. doi: 10.1016/j.aquabot.2005.02.006
Ritchie, K. B. (2006). Regulation of microbial populations by coral surface mucus and mucus associated bacteria. 322, 1–14. doi: 10.3354/meps322001
Rohwer, F., Seguritan, V., Azam, F., and Knowlton, N. (2002). Diversity and distribution of coral-associated bacteria. Mar. Ecol. Prog. Ser. 243, 1–10. doi: 10.3354/meps243001
Röthig, T., Roik, A., Yum, L. K., and Voolstra, C. R. (2017). Distinct bacterial microbiomes associate with the deep-sea coral Eguchipsammia fistula from the Red Sea and from aquaria settings. Front. Mar. Sci. 4:259. doi: 10.3389/fmars.2017.00259
Rypien, K. L., Ward, J. R., and Azam, F. (2010). Antagonistic interactions among coral-associated bacteria. Environ. Microbiol. 12, 28–39. doi: 10.1111/j.1462-2920.2009.02027
Santos, H. F., Carmo, F. L., Duarte, G., Dini-Andreote, F., Castro, C. B., Rosado, A. S., et al. (2014). Climate change affects key nitrogen-fixing bacterial populations on coral reefs. ISME J. 8, 2272–2279. doi: 10.1038/ismej.2014.70
Seymour, J. R., Patten, N., Bourne, D. G., and Mitchell, J. G. (2005). Spatial dynamics of virus-like particles and heterotrophic bacteria within a shallow coral reef system. Mar. Ecol. Prog. Ser. 288, 1–8. doi: 10.3354/meps288001
Sharp, K. H., Pratte, Z. A., Kerwin, A. H., Rotjan, R. D., and Stewart, F. J. (2017). Season, but not symbiont state, drives microbiome structure in the temperate coral Astrangia poculata. Microbiome 5:120. doi: 10.1186/s40168-017-0329-8
Shick, J. M., and Dunlap, W. C. (2002). Mycosporine-like amino acids and related gadusols: biosynthesis, accumulation, and UV-protective functions in aquatic organisms. Annu. Rev. Physiol. 64, 223–262. doi: 10.1146/annurev.physiol.64.081501.155802
Shick, J., Lesser, M. P., and Jokiel, P. L. (1996). Effects of ultraviolet radiation on corals and other coral reef organisms. Glob. Chang. Biol. 2, 527–545.
Silverman, J., Lazar, B., and Erez, J. (2007). Effect of aragonite saturation, temperature, and nutrients on the community calcification rate of a coral reef. J. Geophys. Res. Ocean. 112:C05004. doi: 10.1029/2006JC003770
Smith, R. C., and Baker, K. S. (1981). Optical properties of the clearest natural waters (200–800 nm). Appl. Opt. 20, 177–184. doi: 10.1364/AO.20.000177
Smith, S. V., and Key, G. S. (1975). Carbon dioxide and metabolism in marine environments. Limnol. Oceanogr. 20, 493–495. doi: 10.4319/lo.1975.20.3.0493
Stimson, J., and Kinzie, R. A. (1991). The temporal pattern and rate of release of zooxanthellae from the reef coral Pocillopora damicornis (Linnaeus) under nitrogen-enrichment and control conditions. J. Exp. Mar. Bio. Ecol. 153, 63–74. doi: 10.1016/S0022-0981(05)80006-1
Sunagawa, S., DeSantis, T. Z., Piceno, Y. M., Brodie, E. L., DeSalvo, M. K., Voolstra, C. R., et al. (2009). Bacterial diversity and White Plague Disease-associated community changes in the Caribbean coral Montastraea faveolata. ISME J. 3, 512–521. doi: 10.1038/ismej.2008.131
Taniguchi, A., Yoshida, T., Hibino, K., and Eguchi, M. (2015). Community structures of actively growing bacteria stimulated by coral mucus. J. Exp. Mar. Bio. Ecol. 469, 105–112. doi: 10.1016/j.jembe.2015.04.020
Taylor, J. D., Cottingham, S. D., Billinge, J., and Cunliffe, M. (2014). Seasonal microbial community dynamics correlate with phytoplankton-derived polysaccharides in surface coastal waters. ISME J. 8, 245–248. doi: 10.1038/ismej.2013.178
Thompson, F. L. (2006). Thalassomonas loyana sp. nov., a causative agent of the white plague-like disease of corals on the Eilat coral reef. Int. J. Syst. Evol. Microbiol. 56, 365–368. doi: 10.1099/ijs.0.63800-0
Torres, O., Tanskanen, A., Veihelmann, B., Ahn, C., Braak, R., Bhartia, P. K., et al. (2007). Aerosols and surface UV products from Ozone Monitoring Instrument observations: an overview. J. Geophys. Res. Atmos. 112:D24S47. doi: 10.1029/2007JD00880
Torres-Pérez, J. L., and Armstrong, R. A. (2012). Effects of UV radiation on the growth, photosynthetic and photoprotective components, and reproduction of the Caribbean shallow-water coral Porites furcata. Coral Reefs 31, 1077–1091. doi: 10.1007/s00338-012-0927-7
Ulstrup, K. E., Hill, R., Van Oppen, M. J. H., Larkum, A. W. D., and Ralph, P. J. (2008). Seasonal variation in the photo-physiology of homogeneous and heterogeneous Symbiodinium consortia in two scleractinian corals. Mar. Ecol. Prog. Ser. 361, 139–150. doi: 10.3354/meps07360
van de Water, J. A. J. M., Chaib De Mares, M., Dixon, G. B., Raina, J., Willis, B. L., Bourne, D. G., et al. (2018a). Antimicrobial and stress responses to increased temperature and bacterial pathogen challenge in the holobiont of a reef-building coral. Mol. Ecol. 27, 1065–1080 doi: 10.1111/mec.14489
van de Water, J. A. J. M., Melkonian, R., Voolstra, C. R., Junca, H., Beraud, E., Allemand, D., et al. (2017). Comparative assessment of Mediterranean gorgonian-associated microbial communities reveals conserved core and locally variant bacteria. Microb. Ecol. 73, 466–478 doi: 10.1007/s00248-016-0858-x
van de Water, J. A. J. M., Voolstra, C. R., Rottier, C., Cocito, S., Peirano, A., Allemand, D., et al. (2018b). Seasonal stability in the microbiomes of temperate gorgonians and the red coral Corallium rubrum across the Mediterranean Sea. Microb. Ecol. 75, 274–288. doi: 10.1007/s00248-017-1006-y
van de Water, J. A. J. M., Lamb, J. B., van Oppen, M. J. H., Willis, B. L., and Bourne, D. G. (2015). Comparative immune responses of corals to stressors associated with offshore reef-based tourist platforms. Conserv. Physiol. 3:cov032. doi: 10.1093/conphys/cov032
Warner, M., Chilcoat, G., McFarland, F., and Fitt, W. (2002). Seasonal fluctuations in the photosynthetic capacity of photosystem II in symbiotic dinoflagellates in the Caribbean reef-building coral Montastraea. Mar. Biol. 141, 31–38. doi: 10.1007/s00227-002-0807-8
Watson, J. R., Krömer, J. O., Degnan, B. M., and Degnan, S. M. (2017). Seasonal changes in environmental nutrient availability and biomass composition in a coral reef sponge. Mar. Biol. 164:135. doi: 10.1007/s00227-017-3167-0
Wear, S. L., and Thurber, R. V. (2015). Sewage pollution: mitigation is key for coral reef stewardship. Ann. N. Y. Acad. Sci. 1355, 15–30. doi: 10.1111/nyas.12785
Weynberg, K. D., Voolstra, C. R., Neave, M. J., Buerger, P., and van Oppen, M. J. H. (2016). From cholera to corals: viruses as drivers of virulence in a major coral bacterial pathogen. Sci. Rep. 5:17889. doi: 10.1038/srep17889
Winter, A. P. M., Chaloub, R. M., and Duarte, G. A. S. (2016). Photosynthetic responses of corals Mussismilia harttii (Verrill, 1867) from turbid waters to changes in temperature and presence/absence of light. Brazilian J. Oceanogr. 64, 203–216. doi: 10.1590/S1679-87592016080806403
Wolff, N. H., Mumby, P. J., Devlin, M., and Anthony, K. R. N. (2018). Vulnerability of the Great Barrier Reef to climate change and local pressures. Glob. Chang. Biol. 24, 1978–1991. doi: 10.1111/gcb.14043
Wu, H., Gao, K., and Wu, H. (2009). Responses of a marine red tide alga Skeletonema costatum (Bacillariophyceae) to long-term UV radiation exposures. J. Photochem. Photobiol. B Biol. 94, 82–86. doi: 10.1016/j.jphotobiol.2008
Wymore, A. S., Rodríguez-Cardona, B., and McDowell, W. H. (2015). Direct response of dissolved organic nitrogen to nitrate availability in headwater streams. Biogeochemistry 126, 1–10. doi: 10.1007/s10533-015-0153-9
Zaneveld, J. R., Burkepile, D. E., Shantz, A. A., Pritchard, C. E., McMinds, R., Payet, J. P., et al. (2016). Overfishing and nutrient pollution interact with temperature to disrupt coral reefs down to microbial scales. Nat. Commun. 7:11833. doi: 10.1038/ncomms11833
Zepp, R. G., Shank, G. C., Stabenau, E., Patterson, K. W., Cyterski, M., Fisher, W., et al. (2008). Spatial and temporal variability of solar ultraviolet exposure of coral assemblages in the Florida Keys: importance of colored dissolved organic matter. Limnol. Oceanogr. 53, 1909–1922. doi: 10.4319/lo.2008.53.5.1909
Keywords: coral, holobiont, symbiosis, ultra-violet radiation, seasonality, bacteria, symbiodinium, physiology
Citation: van de Water JAJM, Courtial L, Houlbrèque F, Jacquet S and Ferrier-Pagès C (2018) Ultra-Violet Radiation Has a Limited Impact on Seasonal Differences in the Acropora Muricata Holobiont. Front. Mar. Sci. 5:275. doi: 10.3389/fmars.2018.00275
Received: 03 April 2018; Accepted: 20 July 2018;
Published: 10 August 2018.
Edited by:
Michael Sweet, University of Derby, United KingdomReviewed by:
Christina A. Kellogg, United States Geological Survey, United StatesJesse Robert Zaneveld, University of Washington Bothell, United States
Cheong Xin Chan, The University of Queensland, Australia
Copyright © 2018 van de Water, Courtial, Houlbrèque, Jacquet and Ferrier-Pagès. This is an open-access article distributed under the terms of the Creative Commons Attribution License (CC BY). The use, distribution or reproduction in other forums is permitted, provided the original author(s) and the copyright owner(s) are credited and that the original publication in this journal is cited, in accordance with accepted academic practice. No use, distribution or reproduction is permitted which does not comply with these terms.
*Correspondence: Jeroen A. J. M. van de Water, anZkZXdhdGVyQGNlbnRyZXNjaWVudGlmaXF1ZS5tYw==
†These authors have contributed equally to this work