- 1Agrosphere (IBG-3), Institute of Bio- and Geosciences, Forschungszentrum Jülich, Jülich, Germany
- 2CNRS, Géosciences Rennes - UMR 6118, FranceOSUR, University of Rennes, Rennes, France
- 3Agricultural Catchments Program, Department of Environment, Soils and Landuse, TEAGASC, Johnstown Castle, Ireland
- 4INRA, Agrocampus-Ouest, UMR1069 SAS, Rennes, France
- 5Norwegian Institute of Bioeconomy Research, Ås, Norway
- 6Department of Soil and Environment, Swedish University of Agricultural Sciences, Uppsala, Sweden
- 7Environmental and Biochemical Sciences Group, James Hutton Institute, Aberdeen, Scotland
- 8Environmental Sciences Research Institute, Ulster University, Londonderry, Ireland
- 9Subsurface and Groundwater Quality, Deltares, Delft, Netherlands
- 10Department of Aquatic Ecosystem Analysis, Helmholtz Centre for Environmental Research-UFZ, Magdeburg, Germany
- 11Division of Soil and Water Management, KU Leuven, Leuven, Belgium
In this paper, we outline several recent insights for the priorities and challenges for future research for reducing phosphorus (P) based water eutrophication in the agricultural landscapes of Northwest Europe. We highlight that new research efforts best be focused on headwater catchments as they are a key influence on the initial chemistry of the larger river catchments, and here many management interventions are most effectively made. We emphasize the lack of understanding on how climate change will impact on P losses from agricultural landscapes. Particularly, the capability to disentangle current and future trends in P fluxes, due to climate change itself, from climate driven changes in agricultural management practices and P inputs. Knowing that, future climatic change trajectories for Western Europe will accelerate the release of the most bioavailable soil P. We stress the ambiguities created by the large varieties of sources and storage/transfer processes involved in P emissions in landscapes and the need to develop specific data treatment methods or tracers able to circumvent them, thereby helping catchment managers to identify the ultimate P sources that most contribute to diffuse P emissions. We point out that soil and aqueous P exist not only in various chemical forms, but also in range of less considered physical forms e.g., dissolved, nanoparticulate, colloidal and other particulates, all affected differently by climate as well as other environmental factors, and require bespoke mitigation measures. We support increased high resolution monitoring of headwater catchments, to not only help verify the effectiveness of catchments mitigation strategies, but also add data to further develop new water quality models (e.g., those include Fe-P interactions) which can deal with climate and land use change effects within an uncertainty framework. We finally conclude that there is a crucial need for more integrative research efforts to deal with our incomplete understanding of the mechanisms and processes associated with the identification of critical source areas, P mobilization, delivery and biogeochemical processing, as otherwise even high-intensity and high-resolution research efforts will only reveal an incomplete picture of the full global impact of the terrestrial derived P on downstream aquatic and marine ecosystems.
Introduction
The increasing world-wide issue of the eutrophication of our lakes, reservoirs, rivers and coastal waters has highlighted an urgent need for interdisciplinary action across research fields (e.g., Moss, 2012; Withers et al., 2014; Elmgren et al., 2015; Pinay et al., 2017; Charlton et al., 2018). It is now well documented that anthropogenically derived phosphorus (P) and nitrogen (N) pollution are currently the main drivers of eutrophication, with the excessive inputs of these two nutrients into freshwater and estuarine water bodies being considered as one of the most urgent environmental issues that human societies face (Rockström et al., 2009; Steffen et al., 2015; George et al., 2017). Agricultural activities and urbanization can both deliver excess P and N to aquatic ecosystems, which may cause eutrophication of water courses and which in turn may alter the native ecological communities, degrade ecosystem services and directly or indirectly impact water supply, recreational uses, and human health (e.g., cyanobacteria blooms). Already, in the late 1960s a fundamental change started to occur in most developed countries regarding the type of P input (see Table S1 for P terminology and additionally Haygarth and Sharpley, 2000), namely a very marked decrease of point-source P due to an increased waste water treatment (Persson, 2001; Billen et al., 2007; Grizzetti et al., 2012; Scavia et al., 2014; Minaudo et al., 2015). This decrease has had rapid and marked effects on the P loading and trophic status of downstream water bodies (e.g., a 75% reduction in 25 years of the P flux in the Seine River and Lake Geneva; disappearance of cyanobacteria blooms in some lakes, such as Lake Erie in the USA, Lake du Bourget in France, and Lake Mjosa in Norway; Nesheim et al., 2010; Jacquet et al., 2014; Scavia et al., 2014; Romero et al., 2016). However, this reduction in point sources has been counterbalanced by the stagnation or even the increase of diffuse P emissions from agricultural soils (Scavia et al., 2014; Dupas et al., 2015d; Stoddard et al., 2016). This has happened even despite the fact that overall fertilizer usage in NW Europe since the 1980's has decreased significantly (Schoumans et al., 2015). For example in Sweden, lower P fertilizer use is now accompanied by more or less balanced P inputs and outputs in agriculture (Bergström et al., 2015). Both dissolved/colloidal P, i.e., the most bio-available forms of P for algae (Dupas et al., 2015a; Mellander et al., 2016; Gu et al., 2017), as well as particulate P (Bechmann and Deelstra, 2013) are involved in this increase of P emissions from agricultural soils. For Norway increased delivery of particulate P to surface waters can partly be assigned to higher soil erosion due to climate change effects (i.e., increased runoff) (Deelstra et al., 2011). Whereas, for Sweden a modeling study (Arheimer and Donnelly, 2013) suggested that the total mean load to the Baltic Sea will increase for P, but may decrease for N by 2100 due to climate-induced changes. Enhanced particulate P can be formed in the surface waters due to the discharge of iron (Fe) and calcium (Ca)-bearing groundwater and subsequent precipitation of P-rich minerals (Baken et al., 2013; Van der Grift et al., 2014, 2018). Depending on the receiving water body, most of this particulate P could in the long run become available to algae (e.g., Yang et al., 2016; Yao et al., 2017).
Maintaining high levels or even increasing diffuse P losses from agricultural landscapes hampers any expected improvements in water quality, thereby challenging our society's ability to combat eutrophication. The issue here concerns not only lakes, rivers and estuaries, but also the multitude of small water bodies of natural and artificial origin, some which may serve as reservoirs for drinking water production.
For 30 years or more, much knowledge has been gained on the basic physico-chemical processes by which P is mobilized in soils and transported to rivers, as well as on the chemical forms of the mobilized and transported P. For example, the role of wetting-drying cycles as catalysts for the production and transfer of dissolved and colloidal phosphorus is now well known (e.g., Turner et al., 2003; Butterly et al., 2011; Blackwell et al., 2013; Chen et al., 2016; Gu et al., 2018). In particular, the important role of colloids to serve as carrier of P in soils and waters has been highlighted (e.g., Henderson et al., 2012; Gottselig et al., 2014; Liu et al., 2014; Baken et al., 2016; Jiang et al., 2017; Gu et al., 2018; Missong et al., 2018). Also well-constrained is the capacity of P to bind with manganese (Mn) and iron oxides and the influence the redox state of soils and waters has on P mobility in the environment (Scalenghe et al., 2012; Van der Grift et al., 2014; Jiang et al., 2015a,b; Smolders et al., 2017). Along with these understandings, novel high resolution P water quality monitoring, such as sensor technology, can be used to understand the processes of dissolved and particle-bound substances in waters. For example by using turbidity as a proxy for particulate P we can now reliably achieve high-frequency observations of P losses from agricultural landscapes over multi-annual periods (Jordan et al., 2007; Skarbøvik and Roseth, 2014; Rode et al., 2016; Shore et al., 2017). This information, combined with ever advancing analytical techniques (Kruse et al., 2015) as well as improvements in landscape visualization tool kits, is helping to facilitate efforts to better quantify P dynamics in time and space, from plot to catchment and landscape.
Transforming this knowledge into appropriate cost effective mitigation strategies remains a major challenge (Schoumans et al., 2014, 2015; Dodds and Sharpley, 2015; Kleinman et al., 2015; Sharpley et al., 2015; Withers et al., 2015a,b; Rowe et al., 2016). The degree of integration and knowledge is such today that some of the most recent studies can go as far as integrating the costs of management in the reduction of diffuse phosphorus emissions, in connection with national or local legislation or land property issues (e.g., McDowell et al., 2016; Vinten et al., 2017; Zhang et al., 2017).
The EU Water Framework Directive (WFD) has since its emergence in the beginning of this millennium set the scene for water management in Europe. Its implementation has resulted in massive efforts to classify all water bodies according to their ecological status, within this context monitoring of European waters has, at least tentatively, become more harmonized through the Common Implementation Strategy (CIS) guidelines. Achieving Good Ecological Status of waterbodies across Europe under the Water Framework Directive will require an improved understanding of the link between P concentrations, loads and sources in the soil and recipient freshwater ecosystems within the context of multiple stressors (Whitehead and Crossman, 2012; Crossman et al., 2013). However, in this paper, we will not focus on all the aspects of the WFD or other EU legislation, but only few aspects that are linked to the main foci of our review. These include the types of monitoring that can give new insight into catchment processes, including investigative monitoring and source apportionment.
Furthermore, we are taking into account the considerable amount of papers already published on the topic of P transfer and P management in agricultural landscapes, including several recent review papers (e.g., Kleinman et al., 2011; Chowdhury et al., 2014; Schoumans et al., 2014; Sharpley et al., 2015; Kadlec, 2016; McDowell et al., 2016). As well as related contemporary topical reviews on aspects like scenario analysis and mitigation (Roberts et al., 2012; Schoumans et al., 2014; Dodd and Sharpley, 2016; Ahmad et al., 2017; Liu et al., 2017; Wu et al., 2017), policy and implementation (Christen and Dalgaard, 2013; McDowell et al., 2016), novel modeling approaches and management insights Shepherd et al., 2011; Radcliffe et al., 2015; Xie et al., 2015; Ouyang et al., 2017, water quality modeling (Rode et al., 2010; Wellen et al., 2015; Hashemi et al., 2016), erosion (Panagos et al., 2017) and other more general appraisal of future needs and directions (Fernandez-Mena et al., 2016; Garnache et al., 2016). Hence our intention in this article is not to produce an exhaustive review which also covers the state of knowledge in these related research fields. Rather, our paper is aimed at highlighting certain important knowledge gaps and research challenges on P cycling and transfer processes in agricultural landscapes in Northwest Europe in relation to the continued issue of eutrophication of inland and coastal surface waters. Thereby, implicitly also identifying research needs for these 4 key challenges. These main challenges for us concern: (i) a better understanding of the processes and variables controlling P mobilization and P transfer in headwater catchments, (ii) a better consideration of how the basic mechanisms involved in P mobilization in soils at the small scale aggregate themselves at larger scales, and how this aggregation ultimately control P diffuse emissions in agricultural landscapes, (iii) the resolution of ambiguities in the interpretation of river P dynamics for identification of sources in catchments, and (iv) a better understanding and prediction of the effects of climate change on P fluxes and P physical-chemical forms.
Headwater Catchments: the Right Place to be to Manage and Monitor Diffuse Phosphorus Emissions
Combating eutrophication relies on effective reduction in P losses from land to water through appropriate management of P sources and pathways in the landscape. The key scientific and management questions are: where is the excess P coming from? How are these P sources mobilized and delivered to surface waters? What is their impact on water bodies? For still unknown reasons, some headwater catchments (surface area < 50 km2) in agricultural landscapes appear more resilient (have more buffering capacity) than others with regards to P loading, resulting in huge differences in average annual P concentrations and annual P fluxes, as exemplified by the case of Brittany, one of the most intensively farmed regions of France (Legeay et al., 2015; Abbott et al., 2017). This increased variability of P concentration and P fluxes with decreasing catchment size is as a result of headwater catchments having lower intense cultivation rates. In fact the majority of these Brittany headwater catchments shown in Figure 1 are intensively cultivated (>80% of arable land) throughout (Legeay et al., 2015; Abbott et al., 2017). They were also found to be insensitive to the general decreased particulate P emissions that has been recorded in Brittany since the beginning of the 1990's, as this decrease left the amplitude of particulate P flux variability in headwater catchments unchanged (see Figure 1).
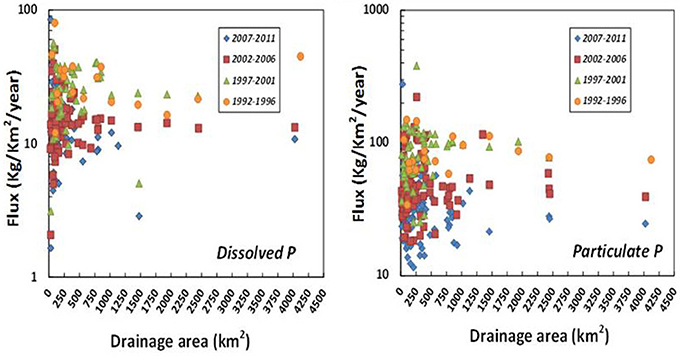
Figure 1. The relationship between dissolved an particulate P fluxes and drainage areas as observed in Brittany rivers, Western France. Fluxes have been calculated as average values for periods of four consecutive years, in order to minimize flux uncertainities. The two plots data from 117 monitoring station. Drainage areas ranges from 11 to 4,000 km2. For both dissolved and particulate P fluxes, an increase variability is observed when moving from large rivers toward headwater catchments, the maximum of variability occurring for headwater catchments of drainage area < 50 km2 (Legeay et al., 2015).
The increased variability of P emissions when moving upstream in headwater catchments is found both for dissolved and particulate P fluxes (Figure 1). Land use, and especially the share of arable land within a catchment, is known to have a strong positive relationship on nutrient losses (Evans et al., 2014). Indeed, plotting median TP (total P) losses against the share of arable land in Swedish catchments included in the monitoring program River outlets and Trend Watercourses results in strong (R2 = 0.79, p < 0.005) and positive relationship (Figure 2). However, long term data from another Swedish water quality program, monitoring program arable catchments, also highlighted an inherent great variability in nutrient delivery among small catchments (< 50 km2) with high portion of the arable land (Figure 2). For these small catchments with high share of arable land, the relationship was not statistically significant at all (R2 = 0.02, p = 0.41). Consequently, measured P concentrations from some small catchments were remarkably low in spite of intensive agricultural production, whereas other catchments were found to be less resilient and much more vulnerable to P losses.
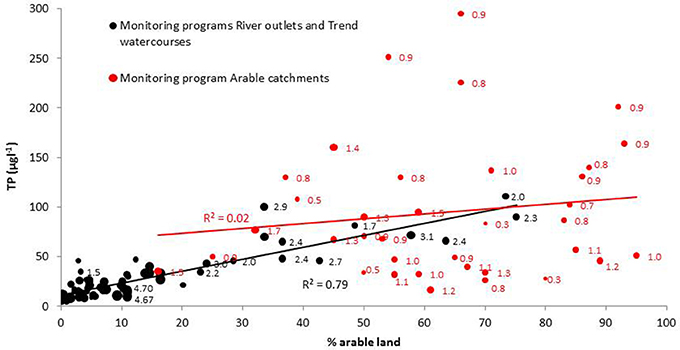
Figure 2. The relationship between the portion of a arable land and median total phosphorus (TP) concentration for the period 2000–2016 included in two Swedish water equality monitoring program: (a) 77 catchments (area 8–50,110 km2). River outlets and trend watercourses (black filled circles) and (b) 36 smaller (area 1.8–54 km2) catchments dominated by agriculture (red filled circles). The size of the filled circles is proportional to the catchment area, and the label value stands for the log10 area of the catchment (km2).
Original source data:
Monitoring programs River outlets and Trend Watercourses: http://miljodata.slu.se/
Monitoring programs Arable catchments:;http://jordbruksvatten.slu.se/.
The high spatial variability observed among headwater catchments appears persistent over time suggesting that occasional synoptic sampling of headwater catchments can provide valuable information for catchment characterization and management with regards to P emissions. This finding also raises the question of the spatial threshold at which the landscape splits into poorly and highly contributing headwater catchments with regards to P emissions, which has been recently referred as to the “landscape grain size” concept (Abbott et al., 2017). There are many potential parameters that could modify the capacity of headwater catchments to release P to river networks, and thus create variations in P emission properties at a specific spatial threshold, or given landscape grain size (see also Dodds and Oakes, 2008; Haygarth et al., 2012). Included are the extent of preferential flow paths in soils and aquifers, which determine residence times in different catchment components, as well as the connectivity between land and water which may strongly vary among headwater catchments (Dahlke et al., 2012; Dupas et al., 2015d, 2016; Mellander et al., 2015). Differences in P applied to or present in soils, the biogeochemical cycling in soils, linked with variations in groundwater dynamics, themselves influenced topography, could be also involved (e.g., Gu et al., 2017). Differences in past land-use history and soil properties will likewise will play a role (Stutter et al., 2015; Gu et al., 2017).
Irrespective of the processes and factors that control the ability of headwater catchments to act as sinks or sources for P, the understanding where P is coming from is important both to target and reduce these sources and also to establish who is responsible for their management according to the polluter pays principle (as defined in the WFD). There is however less clarity in WFD who will pay for the monitoring to obtain this information, in fact, the direct monitoring of point sources is often left to the owner of the waste water treatment plants (Skarbøvik et al., 2014).
Knowing the spatial structure and the typical grain size of source and sink headwater catchments with regards to diffuse P emissions in the landscape could improve site selection for targeted management efforts. Should the difference in resilience properties be of high spatial and temporal stability as found by Legeay et al. (2015) and Abbott et al. (2017), then the intervention in those headwater catchments showing the lowest resilience or highest source properties would potentially yield the largest catchment-level improvements at the lowest cost.
Knowing the landscape typical threshold or grain size could be also helpful for rationalizing water quality monitoring networks. Owing to the temporal stationarity of the spatial distribution of poorly and highly contributing headwater catchments, occasional synoptic sampling of headwater catchments would provide valuable information for identifying high and low contributing headwater catchments in agricultural landscapes. The redistribution of agricultural activity based on the difference in P emission properties of headwater catchments could be a cost effective management strategy for decreasing P loads of larger rivers. This could be implemented even in the absence of a clear understanding of the underlying mechanisms and factors that cause differences in headwater catchment properties. The implementation over the long-term of high frequency monitoring devices at the outlet of larger river basins where the river channel integrates multiple headwater catchments would make it possible to assess the effectiveness of the mitigation strategies thus deployed in the most contributive headwater catchment areas.
Difficulties in Disentangling Point-Source, Diffuse Phosphorus Emissions and Pre-Agricultural Phosphorus Concentration Baselines
Load apportionment models are often used to distinguish between diffuse (mainly from agriculture) and point P sources (sewage treatments, industry sources, fish farming etc.) (Bowes et al., 2008). In many European countries, monitoring networks (statutory monitoring) were established well before 2000 to monitor pollution from point sources and to assess long-term water quality status (Bieroza et al., 2014; Kyllmar et al., 2014), but recently more new emphasis has been put on such networks as part of the obligations and the verification of compliance with EU legislative directives (e.g., WFD). Furthermore, both the location of the sampling points and the monitoring interval are not always suitable to capture the spatial and temporal dynamics of diffuse sources (Jordan and Cassidy, 2011; Bieroza et al., 2014). Thus, targeting diffuse P is difficult, since the sources operate sparsely in both space and time, except for specific locations in the catchment, so-called Critical Source Areas (CSA), and during specific times e.g., rainfall and snowmelt events (Djodjic and Villa, 2015).
Haygarth and Jarvis (1999) stated that having good quantitative knowledge of the baseline P concentrations is necessary when assessing spatiotemporal trends in water quality in agricultural areas. This is also a requirement in the WFD, which states that the environmental goals should deviate only slightly from the background or reference conditions. However, the baseline P concentrations can be expected to vary widely among regions. For example, Phillips and Pitt (2015) demonstrated the differences between European countries of nutrient boundaries used for the WFD. This may be due to natural variations in soil types and topography, but may also be a result of limitations in catchment understanding.
At any rate, such baseline levels should be based on scientific methods, and not on political concerns. Estimations of baseline P is especially difficult in the lower lying areas where the land has been used for agricultural production for centuries, and where such baseline P concentrations must either be modeled or estimated based on limited data. Additionally, those P sources originating from forests and highlands areas (“background runoff”), which are generally poorly quantified themselves, do also contribute to overall baseline P concentrations in the waters of agricultural and urban catchments. Furthermore, the baseline P values may also change, due to large-scale changes such as climate change or the reductions in acid rain. The latter has led to brownification (increased darkening of the water color, due to the increase of dissolved organic carbon content) of Scandinavian waters, which again may have impacted on P losses (Fölster et al., 2014).
Challenges to Monitor and Model Phosphorus Transfer at the Landscape Scale
Monitoring and modeling of P transport pathways in the landscape remains a challenge. This is particularly true for the transfer of particulate P, which is a highly dynamic process, and targeting particulate P sources depends on the exact timing of mitigation measures in relation to plant growth, soil management and soil moisture. Modeling approaches based on detailed measurements of topography (e.g., Lidar) are normally used to target both sources and pathways (e.g., erosion gullies) of sediment-bound P (Thomas et al., 2016). On tile drained soils, significant amounts of particulate P can also be transferred through macropores, which are difficult to monitor and manage (Bechmann et al., 2017a). In addition, elucidating the sources and pathways of dissolved P is also a challenge since it involves understanding of sub-surface pathways (e.g., shallow groundwater) and their connectivity with water bodies as well as its interactions with soil chemistry governing P sorption and release. Shallow sub-surface pathways and hyporheic flow have been found to be important for dissolved P delivery to streams (Bieroza and Heathwaite, 2015; Mellander et al., 2015). Recent advances in high-temporal resolution sampling with in situ analyzers and sensors have yielded information to help delineate potential pathways through hysteresis pattern analysis (Bieroza and Heathwaite, 2015; Dupas et al., 2015b). Furthermore, establishing robust correlations between routinely measured parameters (e.g., turbidity) or smart environmental tracers and P fractions can further help to gain improved understanding of processes and assist to reduce the monitoring cost (Bieroza and Heathwaite, 2016; Minaudo et al., 2017; Stutter et al., 2017).
Statutory monitoring data are used to assess chemical and ecological status of water bodies and to evaluate the impacts of diffuse pollution on stream nutrient concentrations. Despite intensive efforts to reduce these negative impacts, many rural catchments still show increasing long-term P concentration trends (Bechmann et al., 2017b), possibly due to the influence of weather events on local landscape P dynamics, highlighting the continued need for a deeper understanding of these weather-related processes (Bieroza et al., 2014). Seemingly similar catchments (e.g., percentage arable land in a catchment) are known to show opposing nutrient trends, which in turn may indicate a differing resilience to environmental change (Legeay et al., 2015). The observed disconnection between management practices and water quality in many catchments necessitates further efforts to better target P sources and pathways in space and time. The persistence of P pollution also requires more catchment-tailored approaches to mitigate diffuse pollution. Use of high-resolution mapping of CSAs, including soil chemistry, topography and soil type, can help to identify locations in the landscape that will benefit most from the implementation of mitigation measures (Thomas et al., 2016; Djodjic et al., 2017). Using statutory and high-frequency P monitoring in parallel could further help in identifying the most critical time periods and pathways of diffuse pollution (Dupas et al., 2015c). Additionally, new upscaling modeling approaches are needed to link single location patterns to landscape-wide process understanding.
Ambiguities in the Interpretation of River P Dynamics for Identification of Sources in Catchments
In order to limit P transfer and reduce eutrophication, it is important to identify the sources and the mobilization/delivery mechanisms of P into rivers. One commonly used method for source identification is statistical analysis of water quality time series. In particular, load apportionment models (LAMs) based on concentration - discharge (C-Q) relationships (Bowes et al., 2008, 2014, 2015; Greene et al., 2011; Lamba et al., 2015; Crockford et al., 2017; Glendell et al., 2018) or the identification of periods of the year when one source is believed to dominate over other sources, a method is used to disentangle point sources from diffuse sources (Legeay et al., 2015). Equations in LAMs all rely on the assumption that point source emissions are constant in time (leading to negative C-Q relationships due to dilution when discharge increases) while diffuse source emissions increase with discharge (leading to positive C-Q relationships due to mobilization and delivery of P during storm events). Furthermore, analysis of C-Q hysteresis loops during storm events has been used to distinguish proximal (remobilization of stream bed sediments, bank erosion, erosion of riparian area) and distal sources (hillslope erosion, subsurface transfer) (Stutter et al., 2008; Outram et al., 2014, 2016; Bieroza and Heathwaite, 2015; Bowes et al., 2015; Dupas et al., 2015a,b; Perks et al., 2015; Sherriff et al., 2016). Clockwise loops are often interpreted as originating from proximal sources whereas anticlockwise loops are interpreted as originating from distal sources. However the interpretation of P dynamics observed in rivers, and both LAMs and analysis of C-Q hysteresis loops are subject to “ambiguity” problems. Here, the term ambiguity describes situations when several processes (or sources) can lead to the same observed P dynamics in rivers. This can lead to difficulties in inferring the dominant controlling process (or source) from statistical analysis of water quality time series. Jarvie et al. (2012) have shown that remobilization of streambed sediment during winter high flow is often (wrongly) attributed to diffuse source, whereas these sediments may have been enriched by point sources during the summer low flow period, and thus the primary source is predominantly a point source. This ambiguity may lead to overestimation of diffuse sources in LAMs.
The reductive dissolution of Fe oxyhydroxides in sediments of lowland rivers (Smolders et al., 2017) or riparian wetlands in upland systems (Dupas et al., 2017) can lead to soluble reactive phosphorus (SRP) release during summer low flows, increasing summer SRP concentrations in rivers in the same manner as does an undiluted point source. This ambiguity may lead to overestimation of point sources in LAMs. Likewise, ambiguities in river P dynamics have been highlighted in the identification of proximal versus distal sources within hillslopes. Bieroza and Heathwaite (2015) have shown that in the case of two successive storm events, the first event could transport a distal P source to the near-stream zone, which can be remobilized during a second event (exhibiting a clockwise hysteresis loop). Therefore, if only the second event was monitored, there is an ambiguity in the location (proximal/distal) of the primary source, because the primary mobilization of a proximal source can lead to the same observed P dynamics pattern as the secondary mobilization of a distal source (both would exhibit clockwise hysteresis loops). Furthermore, in-situ monitoring of uncultivated soils in the near stream zone have highlighted that these zones represented a significant contribution to annual SRP exports in a temperate agricultural catchment (Gu et al., 2017). However, it is not clear to which extend these zones are primary sources (due to legacy P inputs before their conversion into uncultivated buffers) or secondary sources (as they accumulate and re mobilize P rich eroded soils from cultivated upslope areas). At the scale of the hyporheic zone, Van der Grift et al. (2014, 2018) have shown that SRP via Fe(II) oxidatie at the groundwater-surface water interface can be followed by remobilization of newly formed particulate P. Thus, there can be an ambiguity in the location (proximal/distal) of the source of the particulate P, which can be attributed to a proximal source (the river bank) whereas it may stem from remobilization of a more distal primary source. Another example of ambiguity is the P release pulses observed in summer from river sediments whose ultimate source can be a mixture of several point and diffuse sources, located further upstream (Cooper et al., 2015a; Dupas et al., 2017; Smolders et al., 2017).
Given the important implications of these ambiguities in the identification of P sources and pathways in rural landscapes, several improved methods can be proposed. Firstly, it is possible to use tracers (e.g., stable isotopes and plant-specific biomarkers) and/or complementary water quality parameters in addition to P (Jarvie et al., 2012; Cooper et al., 2015b; Alewell et al., 2016; Glendell et al., 2018) to trace the primary sediment source. For example, Ahlgren et al., 2012 found Barium (Ba) being a promising tracer element, being present in significantly higher amounts in waters affected by agricultural runoff. Whereas Paruch and co-authors developed microbial source-tracking techniques based on advanced DNA methods to distinguish between different sources of microbial contamination (Paruch et al., 2015; Paruch and Paruch, 2017). Furthermore, recent work do suggest that the composition of P carrier colloids could also be a ‘tracer/fingerprint' the source of P (Missong et al., 2018). Secondly, more observational data is needed, combining monitoring at the catchment outlet with in situ observation within functional zones such as riparian wetland or groundwater (Mellander et al., 2016; Gu et al., 2017) to disentangle primary mobilization from secondary delivery/remobilization. Thirdly, interpretation of long term change in water quality dynamics (“trajectory”) in catchments where management changes, such as removal of point sources, decrease in soil P status or installation of buffer zones, have been recorded over several decades (Bieroza et al., 2014) may help to resolve ambiguities in river P dynamics. Therefore, meta-analysis of large catchment datasets may help to identify typical situations where one mechanism (e.g., summer sediment P release versus point source P input) dominates over others. In doing so, “typical” situations can be identified without ambiguity and the P dynamics pattern can be transferred to other, less studied areas as a guide to infer what are the key mechanisms controlling P loads. Finally, consideration of time lags and legacy effects is crucial when communicating results of source identification studies to catchment managers, so that they do not only target secondary proximal sources (short term vision) but also primary more distal sources (long term vision).
Difficulties in Predictions of Dissolved/Colloidal/Particulate Catchment Phosphorus “Hot Spots” and “Hot/Critical” Moments
The difficulty in attributing spatio-temporal variations in P fluxes and P concentrations in streams and rivers draining agricultural landscapes to specific sources or specific mobilization/transfer processes is well known (e.g., Haygarth et al., 2005; Edwards and Withers, 2007; Granger et al., 2010; Trevisan et al., 2012; Hahn et al., 2013; Sharpley et al., 2015). This difficulty mainly arises from the fact that the transfer of P in agricultural landscapes is not often caused by a single process or a single source, rather being the consequence of a series of processes, mobilizing P from several permanent or temporary sources. A good illustration of this difficulty and of the ambiguities it may create in terms of process and source identification was provided by the dissolved/colloidal P release pulses observed in riparian wetland zones. Riparian zones in agricultural catchments are active in transforming poorly mobile particulate P into highly mobile dissolved and colloidal P, which is directly or indirectly bioavailable (Stutter et al., 2009; Dupas et al., 2015a; Gu et al., 2017). Indeed, these P release pulses come from the solubilisation of particulate P eroded from the upland cultivated fields which are temporarily accumulated in wetland zones. This implies that the primary sources of the released P are the upland cultivated fields from which P originates. Quite clearly, such entanglement of processes and existence of temporary sources may lead to ambiguities in the clear identification of the zones and processes contributing most to the risk of P mobilization and P transfer at the catchment scale. Therefore, moving toward full understanding the overall sequence of transfer and retainment of P process chain and how P release capacities vary in space and time is challenging, but it is a knowledge prerequisite to better control and reduce P loss in agricultural landscapes.
In order to better evaluate and quantify how the above mentioned processes control P emissions in catchments, we need to understand how their spatio-temporal occurrence and intensity is controlled by landscape properties, and how the zones where these processes take place are connected with each other and to the river network. Though relevant for particulate P, the issue is particularly relevant for dissolved and colloidal P, which both appear much more significant contributors to P losses from agricultural lands than previously assumed (Kleinman et al., 2011, 2015; Dupas et al., 2015c; Jiang et al., 2015a; Mellander et al., 2015, 2016; Gu et al., 2017), and are also potentially bioavailable P forms. From the perspective of locating dissolved and colloidal P sources in the landscape, the way landscape structures, in interaction with climatic variables, control ground- and surface water dynamics is of fundamental importance. Groundwater level changes in the overall landscape, in response to precipitation and evapotranspiration, influence the location, timing and duration of soil water saturation and wetting-drying episodes that in turn cause reduction of soil Fe-oxyhydroxides. Ground- and surface water dynamics are thus anticipated to be the important determinants of hot spots and hot moment release of dissolved/colloidal P in catchments. An example of such complex control of landscape structure on P release dynamics in catchments has been provided in a recent study conducted on riparian wetlands in a small French catchment (Gu et al., 2017). Large differences have been observed with regards to P release dynamics in the riparian zone, depending on the topography and the control it exerts on the location and timing of biogeochemical processes such as soil Fe-oxyhydroxide reduction or P leaching following soil rewetting. This study has led to the development of a coherent, spatially integrated concept of “landscape biogeochemistry” (see Figure 3) in which the topography, as a first-order control on spatial variation of hydrological conditions (Sørensen et al., 2006), appears to control spatial and temporal distribution of P release processes, the inputs of P from cultivated soils situated upslope (control of topography on soil erosion) and the organic and inorganic nature of released P through indirect control of topography on the rate of soil organic matter mineralization.
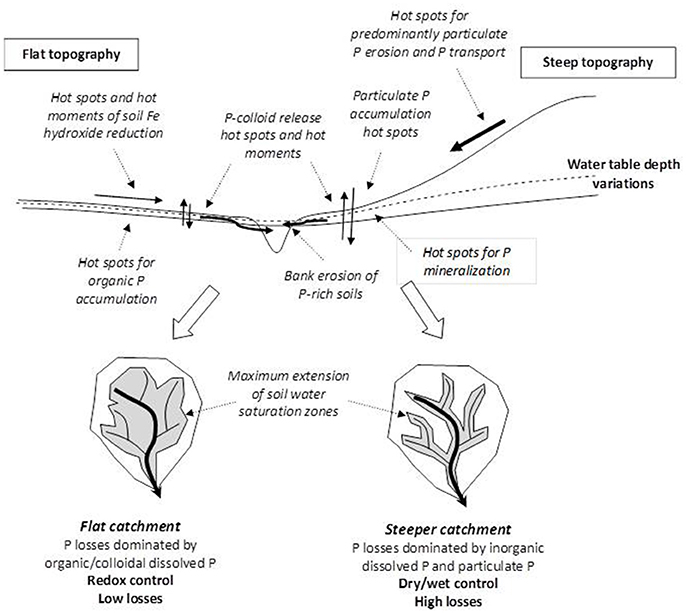
Figure 3. Sketch illustrating the concept of landscape biogeochemistry applied to dissolved, colloidal and particulate P emissions in catchments (adapted from Gu et al., 2017).
The chemistry of P in the soil-water continuum is often controlled through interactions with iron (Fe). Iron is a redox sensitive element and is commonly present in the soil-groundwater-surface water system. Mechanisms such as reduction of soil Fe-oxyhydroxides or rewetting of dried soils are known to trigger the release of P in soils, notably that of dissolved and colloidal P fractions (Turner and Haygarth, 2001; Stutter et al., 2009; Blackwell et al., 2010, 2013; Obour et al., 2011; Scalenghe et al., 2012; Gu et al., 2017; Missong et al., 2018). Redox gradients also result in the precipitation or dissolution of Fe-rich particles, which have a major impact on the fate and bioavailability of P (Jiang et al., 2017). Particulate or colloidal P is formed at the groundwater-surface water interface in groundwater-fed lowland catchments (Van der Grift et al., 2014, 2018; Baken et al., 2015). The formation of Fe hydroxyphosphate precipitates, with molar P/Fe ratio of 0.5 is the main immobilization process of dissolved P during Fe(II) oxidation (Voegelin et al., 2013; Van der Grift et al., 2016) and therefore a major control on the P retention in natural waters that drain anaerobic aquifers. As a consequence, Fe-bound P can be the dominant P fraction in suspended particulate matter under such conditions (Van der Grift, 2017). The Fe(II) oxidation may also lead to formation of Fe(III) bearing colloids. Furthermore, redox mediated P release from river sediments in lowland rivers has been identified as dissolved reactive P (DRP) release mechanism during summer anoxia (Smolders et al., 2017). Reductive dissolution of ferric Fe oxides was found to be associated with mobilization of P to the water column from sediments with a molar P/Fe ratio >0.4. In contrast, no sediment DRP release was found for a lower P/Fe ratio irrespective of temperature and dissolved oxygen treatments. Hence, the P/Fe molar ratio in sediments is an indicator for P mobilization. Clearly, Fe redox dynamics should be taken into account when describing and predicting P transfer from both soils and sediments to surface water.
Particulate P, the dominant P form in many agricultural areas in Northwest European countries like Norway (Bechmann et al., 2008), may be transferred within the headwater catchments adsorbed onto soil particles, but it may also be released from the soil particles when it reaches the recipient waterbody. In addition, some algae can also use particulate P directly. When soil particles do contain high levels of P (high soil P status), these particles may release high amounts of P when diluted in water. Therefore, the importance of particulate (including colloidal) associated phosphorus for algal growth, both on a short and long-term time-scale requires further investigation.
Locating and apportionment of the sources and fluxes of the dissolved vs. collodial P conveyed by rivers in landscapes remains a longstanding and fundamental challenge for catchment scientists (see McClain et al., 2003; Bol et al., 2016; Gottselig et al., 2017b; Missong et al., 2018), with major implications for land management and nutrient pollution mitigation strategies. Colloidal P is characterized as dissolved P in most routine water quality monitoring programs (Gottselig et al., 2017a), however, it is believed to have reduced bioavailability compared to the truly dissolved P (Baken et al., 2014). Furthermore, colloids which consist of organic matter, Fe/Al oxyhydroxides and clay minerals (Jiang et al., 2015a; Missong et al., 2017) are important P carriers in and to surface waters (Gottselig et al., 2014, 2017a,b) and thus should be more explicitly accounted for in P budgets. These interactions between P and colloids need to be understood to better assess and predict P mobility, availability and eutrophication in surface waters, such as homo- and hetero-aggregation behavior of colloidal particles as a controlling factor for P retention. While the mobilization and retention of P cannot be fully understood without understanding its interaction with Fe it is currently omitted from current water quality models and one of their important limitations.
Uncertain Impacts of Weather and Climate Change on P Status
Human influence on the global climate and a range of future climate projections are now well established (Jenkins et al., 2010; IPCC, 2014). However, less information is currently available in the literature on how climate change will impact on current and future P losses from agricultural landscapes (Ockenden et al., 2017). Similarly, how to disentangle current and future trends in P fluxes due to climate change itself from those related to climate driven changes in agricultural management practices and P inputs (Dupas et al., 2016; Bussi et al., 2017). Furthermore, the responses to climate change could vary widely across geographical scales due to localized differences in the sensitivity of catchment P losses to climatic drivers (Mellander et al., 2018), thus highlighting the need to establish climate vulnerability maps for better assessments of the sensitivity of P water quality trends (such as eutrophication potential) to climatic factors. For example, in Western Europe the weather patterns are influenced by both anthropogenic warming and by decadal trends in the North Atlantic Oscillation (NAO) (Hurrell, 1995). Thus long term changes in weather are not spatially uniform and the effects on water quality are expected to vary for different physical and chemical settings, making an area more or less susceptible to P loss (Mellander et al., 2018). We therefore need to better understand both the heterogeneity in large scale weather changes and the influence on small scale processes of P release, retention and transfer pathways within the landscape, as each pathway has a different impact on water quality at the catchment scale. This knowledge is needed both in order to assess current water quality trends (concentrations and loads) and to model future scenarios for specific regions.
As said, while the impacts of projected climate on river hydrological regimes are widely studied, fewer projections of the likely impact on future P pollution in running waters exist (Whitehead et al., 2009; Dunn et al., 2015; Mehdi et al., 2015; Hesse and Krysanova, 2016; Ockenden et al., 2016, 2017). This may be related to the continuing complexity of human activities and its impact on water quality across a range of different spatial scales, including the small headwater catchment scale, while climate scientists traditionally focus their work mostly only at the larger scales (Michalak, 2016). In addition, while it is possible to model the link between precipitation and discharge with reasonable certainty, the relationship between climate change and water quality is subject to uncertainties in our understanding of the potential effects of climate change on the physical and biogeochemical processes along the nutrient source-mobilization-delivery-impact continuum. Furthermore, it is difficult to disentangle direct climate change impacts from the indirect consequences of adaptive land use mediated by climate change (Bussi et al., 2016, 2017). Thus, water quality modeling with respect to climate change impacts is subject to more weaknesses and uncertainties compared to rainfall-runoff modeling (Hesse and Krysanova, 2016). Understanding the impact of climate change on water quality will require an understanding of the link between P concentrations, loads and recipient freshwater ecosystems within the context of multiple stressors (Whitehead and Crossman, 2012; Crossman et al., 2013), including point source and diffuse pollution sources and water abstraction.
Across NW Europe, national scale climate projections vary from relatively straightforward patterns of drier summers and wetter winters in the Atlantic climate across the British Isles (Jenkins et al., 2010), to more complicated regional patterns and higher uncertainty related to both the direction and magnitude of change in precipitation in the more continental climatic regime of Germany (e.g., Van der Linden and Mitchell, 2009; Gädeke et al., 2017). Meanwhile, in Scandinavian countries (Norway and Sweden), climate projections have indicated greater warming in the northern latitudes than in southern latitudes, particularly during winter. The precipitation in Sweden is expected to increase throughout the country but more moderately in summer (Lind and Kjellström, 2008), while in Norway precipitation is expected to increase across the country with the highest increase expected during spring in Mid-Norway (Hanssen-Bauer et al., 2009). Air temperatures affect soil P mineralization, therefore increased temperatures in summer will likely lead to a buildup of labile P pool in the soil (Turner and Haygarth, 2001), ready for transfer to waterbodies by the autumn/winter rains. Thus, areas where both summer temperatures and winter rainfall are projected to increase are likely to become more prone to P mobilization and thus vulnerable to P loss to water (Jordan et al., 2012) (Figure 4). Soil rewetting after a dry summer will mobilize P of mostly microbial origin, largely controlled by the soil P status (Dupas et al., 2015a), while prolonged wet periods due to more rain or more frequent rain may further enhance P release due to reductive dissolution of Fe (hydr)oxides (Van der Grift et al., 2016; Gu et al., 2017). In both cases, the mobilized P consists mainly of dissolved (both organic and inorganic) P forms, i.e., of P forms which are potentially highly bioavailable to algae.
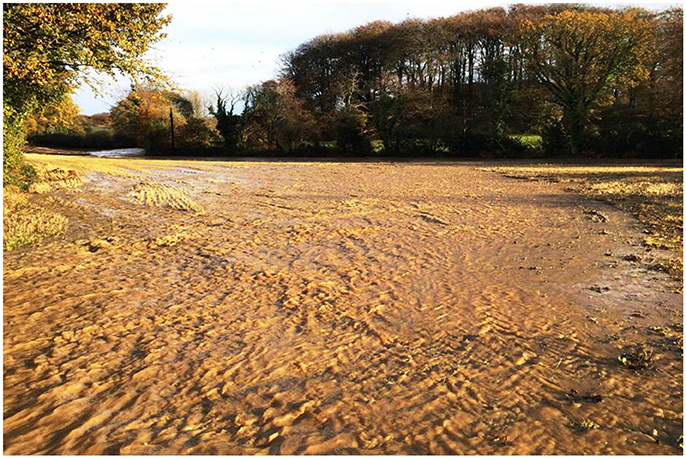
Figure 4. Overland flow on an arable field with well drained soils (country Wexford, Ireland), following heavy rainfall in November 2014 [photo: Michael Fleming].
Drying-rewetting and freezing-thawing episodes of soils are two of the most common forms of abiotic soil perturbation, resulting in solubilisation and release of P (Blackwell et al., 2009). In response to climate change it is likely that the frequency of such episodes and/or the duration of the dry and frozen periods will change differently in different areas. Furthermore, soil freezing-thawing events can increase the rates of P losses due to freezing of plant material (e.g., Bechmann et al., 2005). In Northern European countries, such as Sweden, projected warmer temperatures will likely shorten the period of persistent snowpack and cause more soil freezing-thawing episodes (Mellander et al., 2007) with increased erosion and loss of particulate P. In German lower mountain ranges snowmelt events are often an important source of sediment and P loss (Ollesch et al., 2005, 2006). Increasing temperatures may therefore shorten periods with snow cover, but could in contrast to Northern Europe also lower surface runoff due to smaller number of snowmelt events (Anis and Rode, 2015). These may mobilize both the dissolved and particulate P. In Norway, the trend in the number of freeze-thaw events depends on altitude, with an increasing number observed in the mountains (Hanssen-Bauer et al., 2009) and a decrease observed in the lower lying agricultural areas along the coast (Bechmann and Eggestad, 2016).
Catchments at high risk of P transfer are particularly responsive to changes in climate. For example, in a hydrologically flashy agricultural catchment in Ireland the total reactive phosphorus (TRP) loss was threefold that of a more hydrologically buffered catchment, despite similar soil P status (Mellander et al., 2015). The inter-annual variability in P loss in the flashy catchment was larger than the difference between the two catchments, highlighting the catchment dependence of P transfer risk and sensitivity to more rain, or more frequent rain, to catchment different hydrological flow paths. In another example from Norway, soil erosion was found to be the main process of P transfer in agricultural catchments with arable land and a flashy hydrology. In these catchments the soil P status appeared to have an influence on the catchment P loss (Bechmann et al., 2008). Thus changing rainfall patterns and rainfall intensity under climate change may affect rainfall erosivity and therefore particulate P mobilization and transfer to watercourses (Panagos et al., 2017; Poggio et al., 2018).
An understanding of the magnitude of different hydrological flow paths, their response to climate change and their potential effects on nutrient and soil loss processes will be necessary for choosing the right mitigation measures under future climate scenarios. While the amount of P lost from catchments increases with runoff, several mitigation measures, such as sedimentation ponds, have been implemented to reduce P loading from agricultural catchments, with an effective annual retention of 8–35% of TP (average 18%) (Blankenberg et al., 2013). However, a recent study found that current mitigation efforts will not be sufficient to combat the effects of climate change on P losses, particularly in areas where increased winter runoff will lead to an overriding increase in P loads (Ockenden et al., 2017).
The importance of concentrations vs. loads and seasonality of climate change impacts (i.e., summer vs. winter) will vary with the type of receptor waterbodies (e.g., headwater catchments vs. downstream river reaches and lakes) and will have different impacts on freshwater ecology. For example, increased winter river flows may lead to increased total annual P loads (Ockenden et al., 2017), leading to negative impacts on standing waters such as lakes and reservoirs during the ecologically active period of spring and summer (Stamm et al., 2014). Conversely, increased P concentration during reduced summer flows (Bussi et al., 2017), could lead to negative impacts on river ecosystems (Stamm et al., 2014). These likely differential seasonal and water-body effects will be important to consider when modeling the impact of climate change scenarios on aquatic ecology. Thus improvement of our mechanistic understanding at multiple scales along with development of novel methods for accommodating rigorous error analysis are the imperative challenges for the future of integrated water quality modeling (Rode et al., 2010). Meanwhile, modeling the complex interactions between climate change impacts, social and economic adaptation and land use change is not a trivial task and whilst it is critical for future adaptation policies, to our knowledge, only limited examples of such integrated analysis are available to date (Dunn et al., 2012; Mehdi et al., 2015; Sample et al., 2016).
Conclusion for Future Research
Independent of the variety of issues highlighted in the present paper which do highlight the fact that the reduction of phosphorus (P) based water eutrophication in the agricultural landscapes of Northwest Europe is neither simple nor straightforward. We like to conclude that there is a crucial need for more integrative research efforts to deal with our incomplete understanding of the mechanisms and processes associated with the identification of critical source areas, P mobilization, delivery and biogeochemical processing, as otherwise even high-intensity and high-resolution research efforts will likely only reveal an incomplete picture of the full global impact of the role of terrestrial derived P on downstream aquatic and marine ecosystems.
Author Contributions
All authors listed have made a substantial, direct and intellectual contribution to the work, and approved it for publication.
Conflict of Interest Statement
The authors declare that the research was conducted in the absence of any commercial or financial relationships that could be construed as a potential conflict of interest.
Acknowledgments
The INSU-CNRS Earth Sciences and Astronomy Observatory of Rennes (OSUR), the Loire-Brittany Water Agency (AELB), and the French Catchment Network Observatory (SOERE Réseau des Bassins Versants) are thanked for their financial support to the international workshop meeting which was held in Rennes (France) between 24th and 26th October 2017 and whose outputs and discussions have served as a basis for constructing this paper.
Supplementary Material
The Supplementary Material for this article can be found online at: https://www.frontiersin.org/articles/10.3389/fmars.2018.00276/full#supplementary-material
References
Abbott, B. W., Gruau, G., Zarnetske, J. P., Moatar, F., Barbe, L., Thomas, Z., et al. (2017). Unexpected spatial stability of water chemistry in headwater stream networks. Ecol. Lett. 21, 296–308. doi: 10.1111/ele.12897
Ahlgren, J., Djodjic, F., and Wallin, M. (2012). Barium as a potential indicator of phosphorus in agricultural runoff. J. Environ. Qual. 41, 208–216. doi: 10.2134/jeq2011.0220
Ahmad, Z. U., Sanin, M., Lian, Q., Zappi, M., and Gang, D. D. (2017). Nonpoint source pollution. Water Environ. Res. 89, 1580–1602. doi: 10.2175/106143017X15023776270593
Alewell, C., Birkholz, A., Meusburger, K., Schindler Wildhaber, Y., and Mabit, L. (2016). Quantitative sediment source attribution with compound-specific isotope analysis in a C3 plant-dominated catchment (central Switzerland). Biogeoscience 13, 1587–1596. doi: 10.5194/bg-13-1587-2016
Anis, M. R., and Rode, M. (2015). Effect of climate change on runoff components using high resolution rainfall-runoff modelling. Hydrol. Process. 29, 2478–2490. doi: 10.1002/hyp.10381
Arheimer, B. C., and Donnelly, Strömqvist, J. (2013). Large-scale effects of climate change on water resources in Sweden and Europe. J. Water Man. Res. 69, 201–207.
Baken, S., Moens, C., Van der Grift, B., and Smolders, E. (2016). Phosphate binding by natural irion-rich colloids in streams. Water Res. 98, 326–333. doi: 10.1016/j.watres.2016.04.032
Baken, S., Nawara, S., Van Moorleghem, C., and Smolders, E. (2014). Iron colloids reduce the bioavailability of phosphorus to the green alga Raphidocelis subcapitata. Water Res. 59, 198–206. doi: 10.1016/j.watres.2014.04.010
Baken, S., Salaets, P., Desmet, N., Seuntjens, P., Vanlierde, E., and Smolders, E. (2015). Oxidation of iron causes removal of phosphorus and arsenic from streamwater in groundwater-fed lowland catchments. Environ. Sci. Technol. 49, 2886–2894. doi: 10.1021/es505834y
Baken, S., Sjöstedt, C., Gustafsson, J. P., Seuntjens, P., Desmet, N., De Schutter, J., et al. (2013). Characterisation of hydrous ferric oxides derived from iron-rich groundwaters and their contribution to the suspended sediment of streams. Appl. Geochem. 39, 59–68. doi: 10.1016/j.apgeochem.2013.09.013
Bechmann, M., and Deelstra, J. (2013). Agriculture and Environment-Long Term Monitoring in Norway. Trondheim: Akademika.
Bechmann, M., Deelstra, J., Stålnacke, P., Eggestad, H. O., Øygarden, L., and Pengerud, A. (2008). Monitoring catchment scale agricultural pollution in Norway – policy instruments, implementation of mitigation methods and trends in nutrient and sediment losses. Environ. Sci. Policy 11, 102–114. doi: 10.1016/j.envsci.2007.10.005
Bechmann, M., and Eggestad, H. O. (2016). Temperaturendringer, Plantevekst og Avrenning. NIBIO popular articles 2, 1–4 (In Norwegian).
Bechmann, M., Starkloff, T., Kværnø, S., and Eklo, O. M. (2017a). Plot Study. Soil and Nutrient Losses through Surface and Subsurface Pathways with Different Soil Tillage. NIBIO report 3, 1–48 (In Norwegian).
Bechmann, M., Stenrød, M., Greipsland, I., Hauken, M., Deelstra, J., Eggestad, H. O., et al. (2017b). Erosion and Loss of Nutrients and Pesticides from Agricultural Dominated Catchments. NIBIO report 3, 1–104 (In Norwegian).
Bechmann, M. E., Kleinman, P. J., Sharpley, A. N., and Saporito, L. S. (2005). Freeze-thaw effects on phosphorus loss in runoff from manured and catch-cropped soils. J. Environ. Qual. 34. 2301–2309. doi: 10.2134/jeq2004.0415
Bergström, L. Kirchmann, H., Djodjic, F., Kyllmar, K., Ulén, B., Liu, J., et al. (2015). Turnover and losses of phosphorus in Swedish agricultural soils: long-term changes, leaching trends, and mitigation measures. J. Environ. Qual. 44, 512–523. doi: 10.2134/jeq2014.04.0165
Bieroza, M. Z., and Heathwaite, A. L. (2015). Seasonal variation in phosphorus concentration–discharge hysteresis inferred from high-frequency in situ monitoring. J. Hydrol. 524, 333–347. doi: 10.1016/j.jhydrol.2015.02.036
Bieroza, M. Z., and Heathwaite, A. L. (2016). Unravelling organic matter and nutrient biogeochemistry in groundwater-fed rivers und er baseflow conditions: uncertainty in in situ high-frequency analysis. Sci. Total Environ. 572, 1520–1533. doi: 10.1016/j.scitotenv.2016.02.046
Bieroza, M. Z., Heathwaite, A. L., Mullinger, N. J., and Keenan, P. O. (2014). Understanding nutrient biogeochemistry in agricultural catchments: the challenge of appropriate monitoring frequencies. Environ. Sci. Proc. Imp. 16, 1676–1691. doi: 10.1039/C4EM00100A
Billen, G., Garnier, J., Némery, J., Sebilo, M., Ferratore, A., Barles, S., et al. (2007). A long-term view of nutrient transfers through the Seine river continuum. Sci. Total Environ. 375, 80–97. doi: 10.1016/j.scitotenv.2006.12.005
Blackwell, M. S. A., Brookes, P. C., de la Fuente-Martinez, N., Gordon, H., Murray, P. J., Snars, K. E., et al. (2010). Phosphorus solubilization and potential transfer to surface waters from the soil microbial biomass following drying-rewetting and freezing-thawing. Adv. Agron. 106, 1–35. doi: 10.1016/S0065-2113(10)06001-3
Blackwell, M. S. A., Brookes, P. C., de la Fuente-Martinez, N., Murray, P. J., Snars, K. E., Williams, J. K., et al. (2009). Effects of soil drying and rate of re-wetting on concentrations and forms of phosphorus in leachate. Biol. Fert. Soils 45, 635–643. doi: 10.1007/s00374-009-0375-x
Blackwell, M. S. A., Carswell, A. M., and Bol, R. (2013). Variations in concentrations of N and P forms in leachates from dried soils rewetted at different rates. Biol. Fertil. Soils 49, 79–87. doi: 10.1007/s00374-012-0700-7
Blankenberg, A. G. B., Deelstra, J., Øgaard, A. F., and Pedersen, R. (2013). “Phosphorus and sediment retention in a constructed wetland,” in Agriculture and Environment- Long Term Monitoring in Norway. Trondheim: Akademika.
Bol, R., Julich, D., Brödlin, D., Siemens, J., Dippold, M. A., Spielvogel, S., et al. (2016). Dissolved and colloidal phosphorus fluxes in forest ecosystems - an almost blind spot in ecosystem research. J. Plant Nutr. Soil Sci. 179, 425–438. doi: 10.1002/jpln.201600079
Bowes, M. J., Jarvie, H. P., Halliday, S. J., Skeffington, R. A., Wade, A. J., Loewenthal, M., et al. (2015). Characterising phosphorus and nitrate inputs to a rural river using high-frequency concentration-flow relationships. Sci. Total Environ. 511, 608–620. doi: 10.1016/j.scitotenv.2014.12.086
Bowes, M. J., Jarvie, H. P., Naden, P. S., Old, G. H., Scarlett, P. M., Roberts, C., et al. (2014). Identifying priorities for nutrient mitigation using river concentration-flow relationships: the Thames basin, UK. J. Hydrol. 517, 1–12. doi: 10.1016/j.jhydrol.2014.03.063
Bowes, M. J., Smith, J. T., Jarvie, H. P., and Neal, C. (2008). Modelling of phosphorus inputs to rivers from diffuse and point sources. Sci. Total Environ. 395, 125–138. doi: 10.1016/j.scitotenv.2008.01.054
Bussi, G., Janes, V., Whitehead, P. G., Dadson, S. J., and Holman, I. P. (2017). Dynamic response of land use and river nutrient concentration to long-term climatic changes. Sci. Total Environ. 590–591, 818–831. doi: 10.1016/j.scitotenv.2017.03.069
Bussi, G., Whitehead, P. G., Bowes, M. J., Read, D. S., Prudhomme, C., and Dadson, S. J. (2016). Impacts of climate change, land-use change and phosphorus reduction on phytoplankton in the river Thames (UK). Sci. Total Environ. 572, 1507–1519. doi: 10.1016/j.scitotenv.2016.02.109
Butterly, C. R., McNeill, A. M., Baldock, J. A., and Marschner, P. (2011). Rapid changes in carbon and phosphorus after rewetting of dry soil. Biol. Fertil. Soils 47, 41–50. doi: 10.1007/s00374-010-0500-x
Charlton, M. B., Bowes, M. J., Hutchins, M. G., Orr, H. G., Soley, R., and Davison, P. (2018). Mapping eutrophication risk from climate change: future phosphorus concentrations in English rivers. Sci. Total Environ. 613, 1510–1526. doi: 10.1016/j.scitotenv.2017.07.218
Chen, H., Lai, L., Zhao, X., Li, G., and Lin, Q. (2016). Soil microbial biomass carbon and phosphorus as affected by frequent drying-rewetting. Soil Res. 54, 321–327. doi: 10.1071/SR14299
Chowdhury, R. B., Moore, G. A., and Weatherley, A. J. (2014). A review of recent substance flow analyses of phosphorus to identify priority management areas at different geographical scales. Res. Conserv. Recycl. 83, 213– 228. doi: 10.1016/j.resconrec.2013.10.014
Christen, B., and Dalgaard, T. (2013). Buffers for biomass production in temperate European agriculture: a review and synthesis on function, ecosystem services and implementation. Biomass Bioenergy 55, 53–67. doi: 10.1016/j.biombioe.2012.09.053
Cooper, R. J., Pedentchouk, N., Hiscock, K. M., Disdle, P., Krueger, T., and Rawlins, B. G. (2015b). Apportioning sources of organic matter in streambed sediments: an integrated molecular and compound-specific stable isotope approach. Sci. Total Environ. 520, 187–197. doi: 10.1016/j.scitotenv.2015.03.058
Cooper, R. J., Rawlins, B. G., Krueger, T., Lézé, B., Hiscock, K. M., and Pedentchouk, N. (2015a). Contrasting controls on the phosphorus concentration of suspended particulate matter under baseflow and storm event conditions in agricultural headwater streams. Sci. Total Environ. 533, 49–59. doi: 10.1016/j.scitotenv.2015.06.113
Crockford, L., O'Riordain, S., Taylor, D., Melland, A. R., Shortle, G., and Jordan, P. (2017). The application of high temporal resolution data in river catchment modelling and management strategies. Environ. Monit. Assess. 189:461. doi: 10.1007/s10661-017-6174-1
Crossman, J., Whitehead, P. G., Futter, M. N., Jin, L., Shahgedanova, M., Castellazzi, M., et al. (2013). The interactive responses of water quality and hydrology to changes in multiple stressors, and implications for the long-term effective management of phosphorus. Sci. Total Environ. 454–455, 230–244. doi: 10.1016/j.scitotenv.2013.02.033
Dahlke, H. E., Easton, Z. M., Lyon, S. W., Walter, M. T., Destouni, G., and Steenhuis, T. S. (2012). Dissecting the variable source area concept – subsurface flow pathways and water mixing processes in a hillslope. J. Hydrol. 420–421, 125–141. doi: 10.1016/j.jhydrol.2011.11.052
Deelstra, J., Øygarden, L., Blankenberg, A. G. B., and Eggestad, H. O. (2011). Climate change and runoff from agricultural catchments in Norway. Int. J. Clim. Change Strateg. Manag. 3, 345–360. doi: 10.1108/17568691111175641
Djodjic, F., Elmquist, H., and Collentine, D. (2017). Targeting critical source areas for phosphorus losses: evaluation with soil testing, farmers' assessment and modelling. Ambio 47, 45–56. doi: 10.1007/s13280-017-0935-5
Djodjic, F., and Villa, A. (2015). Distributed, high-resolution modelling of critical source areas for erosion and phosphorus losses. Ambio 44, S241–S251. doi: 10.1007/s13280-014-0618-4
Dodd, R. J., and Sharpley, A. N. (2016). Conservation practice effectiveness and adoption: unintended consequences and implications for sustainable phosphorus management. Nutr. Cycle Agroecosyst. 104, 373–392. doi: 10.1007/s10705-015-9748-8
Dodds, R. J., and Sharpley, A. N. (2015). Recognizing the role of soil organic phosphorus in soil fertility and water quality. Res. Conserv. Recycl. 105, 282–293. doi: 10.1016/j.resconrec.2015.10.001
Dodds, W. K., and Oakes, R. M. (2008). Headwater influences on downstream water quality. Environ. Manag. 41, 367–377. doi: 10.1007/s00267-007-9033-y
Dunn, S. M., Brown, I., Sample, J., and Post, H. (2012). Relationships between climate, water resources, land use and diffuse pollution and the significance of uncertainty in climate change. J. Hydrol. 434–435, 19–35. doi: 10.1016/j.jhydrol.2012.02.039
Dunn, S. M., Towers, W., Dawson, J. J. C., Sample, J., and McDonald, J. (2015). A pragmatic methodology for horizon scanning of water quality linked to future climate and land use scenarios. Land Use Policy 44, 131–144. doi: 10.1016/j.landusepol.2014.12.007
Dupas, R., Delmas, M., Dorioz, J. M., Granier, J., Moatar, F., and Gascuel-Odoux, C. (2015d). Assessing the impact of agricultural pressures on N and P loads and eutrophication risks. Ecol. Indic. 48, 396–407. doi: 10.1016/j.ecolind.2014.08.007
Dupas, R., Gascuel-Odoux, C., Gillet, N., Grimaldi, C., and Gruau, G. (2015c). Distinct export dynamics for dissolved and particulate phosphorus reveal independent transport mechanisms in an arable headwater catchment. Hydrol. Proces. 29, 3162–3178. doi: 10.1002/hyp.10432
Dupas, R., Gruau, G., Gu, S., Guillaume, H., Jaffrézic, A., and Gascuel-Odoux, C. (2015a). Groundwater control of biogeochemical processes causing phosphorus release from riparian wetlands. Water Res. 84, 307–314. doi: 10.1016/j.watres.2015.07.048
Dupas, R., Jomaa, S., Musolff, A., Borchardt, D., and Rode, M. (2016). Disentangling the influence of hydroclimatic patterns and agricultural management on river nitrate dynamics from sub-hourly to decadal time scales. Sci. Total Environ. 571, 791–800. doi: 10.1016/j.scitotenv.2016.07.053
Dupas, R., Musolff, A., Jawitz, J. W., Suresh, P., Rao, C., Jäger, C. G., et al. (2017). Carbon and nutrient export regimes from headwater catchments to downstream reaches. Biogeosciences 14, 4391–4407. doi: 10.5194/bg-14-4391-2017
Dupas, R., Tavenard, R., Fovet, O., Gilliet, N., Grimaldi, C., and Gascuel-Odoux, C. (2015b). Identifying seasonal patterns of phosphorus storm dynamics with dynamic time warping. Water Resour. Res. 51, 8868–8882. doi: 10.1002/2015WR017338
Edwards, A. C., and Withers, P. J. A. (2007). Linking phosphorus sources to impacts in different types of water body. Soil Use Manag. 23, 133–143. doi: 10.1111/j.1475-2743.2007.00110.x
Elmgren, R., Blenckner, T., and Andersson, A. (2015). Baltic sea management: successes and failures. Ambio 44, S335–S344. doi: 10.1007/s13280-015-0653-9
Evans, D. M., Schoenholtz, S. H., Griffith, S. M., and Floyd, W. C. (2014). Spatial and temporal patterns of dissolved nitrogen and phosphorus in surface waters of a multi-land use basin. Environ. Monit. Assess. 186, 873–887 doi: 10.1007/s10661-013-3428-4
Fernandez-Mena, H., Nesme, T., and Pellerin, S. (2016). Towards an agro-industrial ecology: a review of nutrient flow modelling and assessment tools in agro-food systems at the local scale. Sci. Total Environ. 543, 467–479. doi: 10.1016/j.scitotenv.2015.11.032
Fölster, J., Johnson, R. K., Futter, M. N., and Wilander, A. (2014). The swedish monitoring of surface waters: 50 years of adaptive monitoring. Ambio 43, 3–18. doi: 10.1007/s13280-014-0558-z
Gädeke, A., Pohle, I., Koch, H., and Grünewald, U. (2017). Trend analysis for integrated regional climate change impact assessments in the Lusatian river catchments (North-eastern Germany). Reg. Environ. Change 17, 1751–1762. doi: 10.1007/s10113-017-1138-0
Garnache, C., Swinton, S. M., Herriges, J. A., Lupi, F., and Stevenson, R. J. (2016). Solving the phosphorus pollution puzzle: synthesis and directions for future research. Am. J. Agric. Econ. 98, 1334–1359. doi: 10.1093/ajae/aaw027
George, T. S., Giles, C. D., Menezes-Blackburn, D., Condron, L. M., Gama-Rodrigues, A. C., Jaisi, D., et al. (2017). Organic phosphorus in the terrestrial environment: a perspective on the state of the art and future priorities. Plant Soil 427, 191–208. doi: 10.1007/s11104-017-3391-x
Glendell, M., Jones, R., Dungait, J. A. J., Meusburger, K., Schwendel, A. C., Barclay, R., et al. (2018). Tracing of particulate organic C sources across the terrestrial-aquatic continuum, a case study at the catchment scale (Carminowe Creek, southwest England). Sci. Total Environ. 616–617, 1077–1088. doi: 10.1016/j.scitotenv.2017.10.211
Gottselig, N., Amelung, W., Bol, R., Nischwitz, V., Siemens, J., Kirchner, J., et al. (2017b). Natural nanoparticles and colloids in European forest stream waters and their role for phosphorus transport. Glob. Biogeochem. Cycl. 31, 1592–1607.
Gottselig, N., Bol, R., Nischwitz, V., Vereecken, H., Amelung, W., and Klumpp, E. (2014). Distribution of phosphorus-containing fine colloids and nanoparticles in stream water of a forest catchment. Vad. Zone J. 13, 1–11. doi: 10.2136/vzj2014.01.0005
Gottselig, N., Nischwitz, V., Meyn, T., Amelung, W., Bol, R., Halle, C., et al. (2017a). Phosphorus binding to nanoparticles and colloids in forest stream waters. Vad. Zone J. 16, 1–12. doi: 10.2136/vzj2016.07.0064
Granger, S. J., Hawkins, J. M. B., Bol, R., Anthony, S., White, S. M., Owens, P., et al. (2010). Towards a holistic classification of diffuse agricultural water pollution from intensively managed grasslands on heavy soils. Adv. Agron. 105, 83–115. doi: 10.1016/S0065-2113(10)05003-0
Greene, S., Taylor, D., McElarney, Y. R., Foy, R. H., and Jordan, P. (2011). An evaluation of catchment-scale phosphorus mitigation using load apportionment modelling. Sci. Total Environ. 409, 2211–2221. doi: 10.1016/j.scitotenv.2011.02.016
Grizzetti, B., Bouraoui, F., and Aloe, A. (2012). Changes of nitrogen and phosphorus loads to European seas. Glob. Change Biol. 18, 769–782. doi: 10.1111/j.1365-2486.2011.02576.x
Gu, S., Gruau, G., Dupas, R., Rumpel, C., Crème, A., Fovet, O., et al. (2017). Release of dissolved phosphorus from riparian wetlands: evidence for complex interactions among hydroclimate variablilty, topography and soil properties. Sci. Total Environ. 598, 421–431. doi: 10.1016/j.scitotenv.2017.04.028
Gu, S., Gruau, G., Malique, F., Dupas, R., Petitjean, P., Gascuel-Odoux, C., et al. (2018). Drying/rewetting cycles stimulate release of colloid-bound phosphorus in riparian soils. Geoderma 321, 32–41. doi: 10.1016/j.geoderma.2018.01.015
Hahn, C., Prasuhn, V., Stamm, C., Lazzarotto, P., Evangelou, M. W. H., and Schulin, R. (2013). Prediction of dissolved reactive phosphorus losses from small agricultural catchments: calibration and validation of a parsimonious model. Hydrol. Earth Syst. Sci. 17, 3679–3693. doi: 10.5194/hess-17-3679-2013
Hanssen-Bauer, I., Drange, H., Førland, E. J., Roald, L. A., Børsheim, K. Y., Hisdal, H., et al. (2009). Klima I Norge 2100. Bakgrunnsmateriale til NOU Klimatilplassing, Norsk klimasenter, Oslo.
Hashemi, F., Olesen, J. E., Dalgaard, T., and Børgesen, C. D. (2016). Review of scenario analyses to reduce agricultural nitrogen and phosphorus loading to the aquatic environment. Sci. Total Environ. 573, 608–626. doi: 10.1016/j.scitotenv.2016.08.141
Haygarth, P. M., Condron, L. M., Heathwaite, A. L., Turner, B. L., and Harris, G. P. (2005). The phosphorus transfer continuum: linking source to impact with an interdisciplinary and multi-scaled approach. Sci. Total Environ. 344, 5–14. doi: 10.1016/j.scitotenv.2005.02.001
Haygarth, P. M., and Jarvis, S. C. (1999). Transfer of phosphorus from agricultural soil. Adv. Agron. 66, 195–249. doi: 10.1016/S0065-2113(08)60428-9
Haygarth, P. M., Page, T. J. C., Beven, K. J., Freer, J., Joynes, A., Butler, P., et al. (2012). Lancaster Environment Centre, Scaling up the phosphorus signal from soil hillslopes to headwater catchments. Freshw. Biol. 57, S7–25. doi: 10.1111/j.1365-2427.2012.02748.x
Haygarth, P. M., and Sharpley, A. N. (2000). Terminology for phosphorus transfer. JEQ 29, 10–15. doi: 10.2134/jeq2000.00472425002900010002x
Henderson, R., Kabengi, N., Mantripragada, N., Cabrera, M., Hassan, S., and Thompson, A. (2012). Anoxia-induced release of colloid- and nanoparticle-bound phosphorus in grassland soils. Environ. Sci. Technol. 46, 11727–11734. doi: 10.1021/es302395r
Hesse, C., and Krysanova, V. (2016). Modeling climate and management change impacts on water quality and in-stream processes in the Elbe River Basin. Water 8:40. doi: 10.3390/w8020040
Hurrell, J. W. (1995). Decadal trends in the North Atlantic oscillation: regional temperatures and precipitation. Science 269, 676–679. doi: 10.1126/science.269.5224.676
IPCC (2014). Climate Change 2014: Synthesis Report. Contribution of Working Groups, I., II and III to the Fifth Assessment Report of the Intergovernmental Panel on Climate Change, eds Core Writing Team, R. K. Pachauri and L. A. Meyer (Geneva: IPCC).
Jacquet, S., Kerimoglu, O., Rimet, F., and Paolini, G. (2014). Cyanobacterial bloom termination: the disappearance of Planktothrix rubescens from Lake Bourget (France) after restoration. Freshw. Biol. 59, 2472–2487. doi: 10.1111/fwb.12444
Jarvie, H. P., Sharpley, A. N., Scott, J. T., Haggard, B. E., Bowes, M. J., and Massey, L. B. (2012). Within-river phosphorus retention: accounting for a missing piece in the watershed phosphorus puzzle. Environ. Sci. Technol. 46, 13284–13292. doi: 10.1021/es303562y
Jenkins, G. J., Murphy, J. M., Sexton, D. M. H., Lowe, J. A., Jones, P., and Kilsby, C. G. (2010). UK Climate Projections: Briefing Report. Version 2, December 2010. Available online at: http://ukclimateprojections.metoffice.gov.uk/22536
Jiang, X., Bol, R., Nischwitz, V., Siebers, N., Willbold, S., Vereecken, H., et al. (2015a). Phosphorus containing water dispersible nanoparticles in arable soil. J. Environ. Qual. 44, 1772–1778. doi: 10.2134/jeq2015.02.0085
Jiang, X.-Q., Bol, R., Willbold, S., Vereecken, H., and Klumpp, E. (2015b). Speciation and distribution of P associated with Fe and Al oxides in aggregate-sized fraction of an arable soil. Biogeosciences 12, 6443–6452. doi: 10.5194/bg-12-6443-2015
Jiang, X. -Q., Bol, R., Cade-Menun, B. J., Nischwitz, V., Willbold, S., Bauke, S. L., et al. (2017). Colloid-bound and dissolved phosphorus species in topsoil water extracts along a grassland transect from Cambisol to Stagnosol. Biogeosciences 14, 1153–1164. doi: 10.5194/bg-14-1153-2017
Jordan, P., Arnscheidt, J., McGrogan, H., and McCormick, S. (2007). Characterising phosphorus transfers in rural catchments using a continuous bank-side analyser. Hydrol. Earth Syst. Sci. 11, 372–381. doi: 10.5194/hess-11-372-2007
Jordan, P., and Cassidy, R. (2011). Technical note: assessing a 24/7 solution for monitoring water quality loads in small river catchments. Hydrol. Earth Syst. Sci. 15, 3093–3100. doi: 10.5194/hess-15-3093-2011
Jordan, P., Melland, A. R., Mellander, P. E., Shortle, G., and Wall, D. (2012). The seasonality of phosphorus transfers from land to water: implications for trophic impacts and policy evaluation. Sci. Total Environ. 434, 101–109. doi: 10.1016/j.scitotenv.2011.12.070
Kadlec (2016). Large constructed wetlands for phosphorus control: a review. Water 8:243. doi: 10.3390/w8060243
Kleinman, P. J., Sharpley, A. N., Withers, P. J., Bergström, L., Johnson, L. T., and Doody, D. G. (2015). Implementing agricultural phosphorus science and management to combat eutrophication. Ambio 44, 297–310. doi: 10.1007/s13280-015-0631-2
Kleinman, P. J. A., Sharpley, A. N., McDowell, R. W., Flaten, D. N., Buda, A. R., Tao, L., et al. (2011). Managing agricultural phosphorus for water quality protection: principles for progress. Plant Soil 349, 169–182. doi: 10.1007/s11104-011-0832-9
Kruse, J., Abraham, M., Amelung, W., Baum, C., Bol, R., Kühn, O., et al. (2015). Innovative methods in soil phosphorus research: a review. J. Plant Nutr. Soil Sci. 178, 43–88. doi: 10.1002/jpln.201400327
Kyllmar, K., Bechmann, M., Deelstra, J., Iital, A., Blicher-Mathiesen, G., Jansons, V., et al. (2014). Long-term monitoring of nutrient losses from agricultural catchments in the Nordic–Baltic region – a discussion of methods, uncertainties and future needs. Agric. Ecosyst. Environ. 198, 4–12. doi: 10.1016/j.agee.2014.07.005
Lamba, J., Karthikeyan, K. G., and Thompson, A. M. (2015). Using radiometric fingerprinting and phosphorus to elucidate sediment transport dynamics in an agricultural watershed. Hydrol. Proc. 29, 2681–2693. doi: 10.1002/hyp.10396
Legeay, P. L., Gruau, G., and Moatar, F. (2015). Une Analyse de la Variabilité Spatio-temporelle des Flux et Des Sources Du Phosphore Dans Les Cours D'eau Bretons., Agence de l'Eau. Loire, Bretagne, 1–104 (in French).
Lind, P., and Kjellström, E. (2008). Temperature and Precipitation Changes in Sweden, a Wide Range of Model-Based Projections for the 21st Century. SMHI Reports Meteorology and Climatology.
Liu, J., Yang, J., Liang, X., Zhao, Y., Cade-Menun, B., and Hu, Y. (2014). Molecular speciation of phosphorus present in readily dispersible colloids from agricultural soils. Soil Sci. Soc. Am. J. 78, 47–53. doi: 10.2136/sssaj.2013.05.0159
Liu, Y., Engel, B. A., Flanagan, D. C., Gitaua, M. W., McMillan, S. K., and Chaubey, I. (2017). A review on effectiveness of best management practices in improving hydrology and water quality: needs and opportunities. Sci. Total Environ. 601–602, 580–593. doi: 10.1016/j.scitotenv.2017.05.212
McClain, M. E., Boyer, E. W., Dent, L., Gergel, S. E., Grimm, N. B., Groffman, P. M., et al. (2003). Biogeochemical hot spots and hot moments at the interface of terrestrial and aquatic ecosystems. Ecosystems 3, 301–312. doi: 10.1007/s10021-003-0161-9
McDowell, R. W., Dils, R. M., Collins, A. L., Flahive, K. A., Sharpley, A. N., and Quinn, J. (2016). A review of the policies and implementation of practices to decrease water quality impairment by phosphorus in New Zealand, the UK, and the US. Nutr. Cycl. Agroecosyst. 104, 289–305. doi: 10.1007/s10705-015-9727-0
Mehdi, B., Ludwig, R., and Lehner, B. (2015). Regional Studies Evaluating the impacts of climate change and crop land use change on streamflow, nitrates and phosphorus: a modelling study in Bavaria. J. Hydrol. 4, 60–90. doi: 10.1016/j.ejrh.2015.04.009
Mellander, P.-E., Jordan, P., Shore, M., Melland, A. R., and Shortle, G. (2015). Flow paths and phosphorus transfer pathways in two agricultural streams with contrasting flow controls. Hydrol. Process. 29, 3504–3518. doi: 10.1002/hyp.10415
Mellander, P.-E., Ottosson Löfvenius, M., and Laudon, H. (2007). Climate change impact on snow and soil temperature in boreal scots pine stands. Clim. Change 85, 179–193. doi: 10.1007/s10584-007-9254-3
Mellander, P. E., Jordan, P., Bechmann, M., Fovet, O., Shore, M. M., McDonald, N. T., et al. (2018). Integrated climate-chemical indicators of diffuse pollution from land to water. Sci. Rep. 8:944. doi: 10.1038/s41598-018-19143-1
Mellander, P. E., Jordan, P., Shore, M., McDonald, N. T., Wall, D. P., Shortle, G., et al. (2016). Identifying contrasting influences and surface water signals for specific groundwater phosphorus vulnerability. Sci. Total Environ. 541, 292–302. doi: 10.1016/j.scitotenv.2015.09.082
Michalak, A. (2016). Study role of climate change in extreme threats to water quality. Nature 535, 349–350. doi: 10.1038/535349a
Minaudo, C., Dupas, R., Gascuel-Odoux, C., Fovet, O., Mellander, P.-E., Jordan, P., et al. (2017). Nonlinear empirical modeling to estimate phosphorus exports using continuous records of turbidity and discharge. Water Resour. Res. 53, 7590–7606. doi: 10.1002/2017WR020590
Minaudo, C., Meybeck, M., Moatar, F., Gassama, N., and Curie, F. (2015). Eutrophication mitigation in rivers: 30 Years of trends in spatial and seasonal patterns of biogeochemistry of the Loire River (1980-2012). Biogeoscience 12, 2549–2563. doi: 10.5194/bg-12-2549-2015
Missong, A., Bol, R., Nischwitz, V., Siemens, J., Krüger, J., and, Lang, F., et al. (2017). Phosphorus in water dispersible colloids of forest soil profiles. Plant Soil 427, 71–86. doi: 10.1007/s11104-017-3430-7
Missong, A., Holzmann, S., Bol, R., Nischwitz, V., Puhlmann, H., v. Wilpert, K., et al. (2018). Leaching of natural colloids from forest topsoils and their relevance for phosphorus transfer. Sci. Total Environ. 634, 305–315. doi: 10.1016/j.scitotenv.2018.03.265
Moss, B. (2012). Cogs in the endless machine: lakes, climate change and nutrient cycles: a review. Sci. Total Environ. 434, 130–142. doi: 10.1016/j.scitotenv.2011.07.069
Nesheim, I., Stålnacke, P., Nagothu, U. S., Skarbøvik, E., Barkved, L. J., and Thaulow, H. (2010). “IWRM status in the Glomma River basin,” in Integrating Water Resources Management. Interdisciplinary Methodologies and Strategies, eds G. D. PracticGooch, A. Rieu-Clarke, and P. Stålnacke (London: IWA-Publishing), 13–23.
Obour, A. K., Silveira, M., Vendraminin, J. M. B., Sollenberger, L. E., and O'Connor, G. A. (2011). Fluctuating water table effect on phosphorus release and availability from a Florida Spodsol. Nutr. Cycl. Agroecosyst. 91, 207–217. doi: 10.1007/s10705-011-9456-y
Ockenden, M. C., Deasy, C. E., Benskin, C. M. H., Beven, K. J., Burke, S., Collins, A. L., et al. (2016). Changing climate and nutrient transfers: evidence from high temporal resolution concentration-flow dynamics in headwater catchments. Sci. Total Environ. 548–549, 325–339. doi: 10.1016/j.scitotenv.2015.12.086
Ockenden, M. C., Hollaway, M. J., Beven, K. J., Collins, A. L., Evans, R., Falloon, P. D., et al. (2017). Major agricultural changes required to mitigate phosphorus losses under climate change. Nat. Commun. 8:161. doi: 10.1038/s41467-017-00232-0
Ollesch, G., Kistner, I., Meissner, R., and Lindenschmidt, K. E. (2006). Modelling of snowmelt erosion and sediment yield in a small low-mountain catchment in Germany. Catena 68, 161–176. doi: 10.1016/j.catena.2006.04.005
Ollesch, G., Sukhanovski, Y., Kistner, I., Rode, M., and Meissner, R. (2005). Characterisation and modelling of the spatial heterogeneity of snowmelt erosion. Earth Surf. Process. Land. 30, 197–211. doi: 10.1002/esp.1175
Outram, F. N., Cooper, R. J., Sünnenberg, G., Hiscock, K. M., and Lovett, A. A. (2016). Antecedent conditions, hydrological connectivity and anthropogenic inputs: factors affecting nitrate and phosphorus transfers to agricultural headwater streams. Sci. Total Environ. 545–546, 184–199. doi: 10.1016/j.scitotenv.2015.12.025
Outram, F. N., Lloyd, C. E. M., Jonczyk, J., Benskin, C. M. H., Grant, F., Perks, M. T., et al. (2014). High-frequency monitoring of nitrogen and phosphorus response in three rural catchments to the end of the 2011-2012 drought in England. Hydrol. Earth Syst. Sci. 18, 3429–3448. doi: 10.5194/hess-18-3429-2014
Ouyang, W., Gao, X., Wei, P., Gao, B., Lin, C. Y., and Hao, F. H. (2017). A review of diffuse pollution modelling and associated implications for watershed management in China. J. Soils Sediments 17, 1527–1536. doi: 10.1007/s11368-017-1688-2
Panagos, P., Ballabio, C., Meusburger, K., Spinoni, J., Alewell, C., and Borrelli, P. (2017). Towards estimates of future rainfall erosivity in Europe based on REDES and worldclim datasets. J. Hydrol. 548, 251–262 doi: 10.1016/j.jhydrol.2017.03.006
Paruch, A. M., Blankenberg, A. G. B., Bechmann, M., and Mæhlum, T. (2015). Application of host-specific genetic markers for microbial source tracking of faecal water contamination in an agricultural catchment. Acta Agric. Scand. 65, 164–172. doi: 10.1080/09064710.2014.941392
Paruch, L., and Paruch, A. M. (2017). The importance of melting curve analysis in discriminating faecal and environmental Bacteroidales bacteria. Microbiology 86, 536–538. doi: 10.1134/S0026261717040117
Perks, M. T., Owen, G. J., Benskin, C. M., Jonczyk, J., Deasy, C., Burke, S., et al. (2015). Dominant mechanisms for the delivery of fine sediment and phosphorus to fluvial networks draining grassland dominated headwater catchments. Sci. Total Environ. 523, 178–190. doi: 10.1016/j.scitotenv.2015.03.008
Persson, G. (2001). Phosphorus in tributaries to lake Mälaren, Sweden: analytical fractions, anthropogenic contribution and bioavailability. Ambio 30, 486–495. doi: 10.1579/0044-7447-30.8.486
Phillips, G., and Pitt, J. A. A. (2015). Comparison of European Freshwater Nutrient Boundaries Used for the Water Framework Directive: A Report to ECOSTATP. 1–176.
Pinay, G., Gascuel, C., Ménesguen, A., Souchon, Y., Le Moal, M., Levain, A., et al. (2017). L'eutrophisation: Manifestations, Causes,Conséquences et Prédictibilité. Synthèse de l'Expertise Scientifique Collective CNRS - Ifremer - INRA -Irstea (France), 1–148.
Poggio, L., Simonettia, E., and Gimona, A. (2018). Enhancing the worldclim data set for national and regional applications. Sci. Total Environ. 625, 1628–1643. doi: 10.1016/j.scitotenv.2017.12.258
Radcliffe, D. E., Reid, K. D., Blombäck, K., Bolster, C. H., Collick, A. S., Easton, Z. M., et al. (2015). Applicability of models to predict phosphorus losses in drained fields: a review. J. Environ. Qual. 44, 614–628. doi: 10.2134/jeq2014.05.0220
Roberts, W. M., Stutter, M. I., and Haygarth, P. M. (2012). Phosphorus retention and remobilization in vegetated buffer strips: a review. J. Environ. Qual. 41, 389–399. doi: 10.2134/jeq2010.0543
Rockström, J., Steffen, W., Noone, K., Persson, Å., Chapin, F. S., Lambin, E., et al. (2009). Planetary boundaries: exploring the safe operating space for humanity. Ecol. Soc. 14:32. doi: 10.5751/ES-03180-140232
Rode, M., Arhonditsis, G., Balin, D., Kebede, T., Krysanova, V., van Griensven, A., et al. (2010). New challenges in integrated water quality modelling. Hydrol. Process. 24, 3447–3461. doi: 10.1002/hyp.7766
Rode, M., Wade, A. J., Cohen, M. J., Hensley, R. T., Bowes, M. J., Kirchner, J. W., et al. (2016). Sensors in the stream: the high-frequency wave of the present. Environ. Sci. Technol. 50, 10297–10307. doi: 10.1021/acs.est.6b02155
Romero, E., Le Gendre, R., Garnier, J., Billen, G., Fisson, C., Silvestre, M., et al. (2016). Long-term water quality in the lower seine: lessons learned over 4 decades of monitoring. Environ. Sci. Pol. 58, 141–154. doi: 10.1016/j.envsci.2016.01.016
Rowe, H., Withers, P. J. A., Baas, P., Chan, N. I., Doody, D., Holiman, J., et al. (2016). Integrating legacy soil phosphorus into sustainable nutrient management strategies for future food, bioenergy and water security. Nutr. Cycl. Agroecosyst. 104, 393–412. doi: 10.1007/s10705-015-9726-1
Sample, J. E., Baber, I., and Badger, R. (2016). A spatially distributed risk screening tool to assess climate and land use change impacts on water-related ecosystem services. Environ. Model. Softw. 83, 12–26. doi: 10.1016/j.envsoft.2016.05.011
Scalenghe, R., Edwards, A. C., Barberis, E., and Ajmone-Marsan, F. (2012). Release of phosphorus under reducing and simulated open drainage conditions from overfertilised soils. Chemosphere 95, 289–294. doi: 10.1016/j.chemosphere.2013.09.016
Scavia, D., Allan, J. D., Arend, K. K., Bartell, S., Beletsky, D., Bosch, N. S., et al. (2014). Assessing and addressing the re-eutrophication of Lake Erie: central basin hypoxia. J. Great Lakes Res. 40, 226–246. doi: 10.1016/j.jglr.2014.02.004
Schoumans, O. F., Bouraoui, F., Kabbe, C., Oenema, O., and van Dijk, K. C. (2015). Phosphorus management in Europe in a changing world. Ambio 44, 180–192. doi: 10.1007/s13280-014-0613-9
Schoumans, O. F., Chardon, W. J., Bechmann, M. E., Gascuel-Odoux, C., Hofman, G., Kronvang, B., et al. (2014). Mitigation options to reduce phosphorus losses from the agricultural sector and improve surface water quality: a review. Sci. Total Environ. 468–469, 1255–1266. doi: 10.1016/j.scitotenv.2013.08.061
Sharpley, A. N., Bergström, L., Aronsson, H., Bechmann, M., Bolster, C. H., Börling, K., et al. (2015). Future agriculture with minimized phosphorus losses to waters: research needs and direction. Ambio 44, 163–179. doi: 10.1007/s13280-014-0612-x
Shepherd, A., Wu, L. H., Chadwick, D., and Bol, R. (2011). A review of quantitative tools for assessing the diffuse pollution response to farmer adaptions and mitigation methods under climate change. Adv. Agron. 112, 1–54. doi: 10.1016/B978-0-12-385538-1.00001-9
Sherriff, S. C., Rowan, J. S., Fenton, O., Jordan, P., Melland, A. R., Mellander, P. E., et al. (2016). Storm event suspended sediment-discharge hysteresis and controls in agricultural watersheds: implications for watershed scale sediment management. Environ. Sci. Technol. 50, 1769–1778. doi: 10.1021/acs.est.5b04573
Shore, M., Murphy, S., Mellander, P.-E., Short, G., Melland, A. R., Crockford, L. (2017) Influence of storm flow base flow phosphorus pressures on stream ecology in agricultural catchments. Sci. Total Environ. 590–591, 469–483. doi: 10.1016/j.scitotenv.2017.02.100, et al.
Skarbøvik, E., and Roseth, R. (2014). Use of sensor data for turbidity, pH and conductivity as an alternative to conventional water quality monitoring in four Norwegian case studies. Acta Agric. Scand. Sect. B Soil Plant Sci. 65, 63–73.doi: 10.1080/09064710.2014.966751
Skarbøvik, E., Stålnacke, P., Kaste, Ø., and Austnes, K. (2014). Trends in nutrients and metals in Norwegian rivers and point sources 1990–2009. Hydrol. Res. 45, 441–454. doi: 10.2166/nh.2013.233
Smolders, E., Baetens, E., Verbeeck, M., Nawara, S., Diels, J., Verdievel, M., et al. (2017). Internal loading and redox cycling of sediment iron explain reactive phosphorus concentrations in lowland rivers. Environ. Sci. Technol. 51, 2584–2592. doi: 10.1021/acs.est.6b04337
Sørensen, R., Zinko, U., and Seibert, J. (2006). On the calculation of the topographic wetness index: evaluation of different methods based on field observations. Hydrol. Earth Syst. Sci. 10, 101–112. doi: 10.5194/hess-10-101-2006
Stamm, C., Jarvie, H. P., and Scott, T. (2014). What's more important for managing phosphorus: loads, concentrations or both? Environ. Sci. Technol. 48, 23–24. doi: 10.1021/es405148c
Steffen, W., Richardson, K., Rockström, J., Cornell, S. E., Fetzer, I., Bennett, E. M., et al. (2015). Planetary boundaries: guiding human development on a changing planet. Science 347:1259855. doi: 10.1126/science.1259855
Stoddard, J. L., Van Sickle, J., Herlihy, A. T., Brahney, J., Paulsen, S., Peck, D. V., et al. (2016). Continental-Scale Increase in lake and stream phosphorus: are oligotrophic systems disappearing in the United States? Environ. Sci. Technol. 50, 3409–3415. doi: 10.1021/acs.est.5b05950
Stutter, M., Dawson, J. J. C., Glendell, M., Napier, F., Potts, J. M., Sample, J., et al. (2017). Evaluating the use of in-situ turbidity measurements to quantify fluvial sediment and phosphorus concentrations and fluxes in agricultural streams. Sci. Total Environ. 607–608, 391–402. doi: 10.1016/j.scitotenv.2017.07.013
Stutter, M. I., Langan, S. J., and Cooper, R. J. (2008). Spatial contributions of diffuse inputs and within-channel processes to the form of stream water phosphorus over storm events. J. Hydrol. 350, 203–214. doi: 10.1016/j.jhydrol.2007.10.045
Stutter, M. I., Langan, S. J., and Lumsdon, D. (2009). Vegetated buffer strips can lead to increased release of phosphorus to waters: a biogechemical assessment of the mechanisms. Environ. Sci. Technol. 43, 1858–1863. doi: 10.1021/es8030193
Stutter, M. I., Shand, C. A., George, T. S., Blackwell, M. S. A., Dixon, L., Bol, R., et al. (2015). Land use and soil factors affecting accumulation of phosphorus species in temperate soils. Geoderma 257–258, 29–39. doi: 10.1016/j.geoderma.2015.03.020
Thomas, I. A., Jordan, P., Mellander, P. E., Fenton, O., Shine, O., Creamer, R., et al. (2016). Improving the identification of hydrologically sensitive areas using LiDAR DEMs for the delineation and mitigation of critical source areas of diffuse pollution. Sci. Total Environ. 556, 276–290. doi: 10.1016/j.scitotenv.2016.02.183
Trevisan, D., Quétin, P., Barbet, D., and Dorioz, J. M. (2012). POPEYE: a river-load oriented model to evaluate the efficiency of environmental policy measures for reducing phosphorus losses. J. Hydrol. 450–451, 254–266. doi: 10.1016/j.jhydrol.2012.05.001
Turner, B. L., Dressen, J. P., Haygarth, P. M., and Mckelvie, I. D. (2003). Potential contribution of lysed bacterial celles to phosphorus solubilisation in two rewetted Australian pasture soils. Soil Biol. Biochem. 35, 187–189. doi: 10.1016/S0038-0717(02)00244-4
Turner, B. L., and Haygarth, P. M. (2001). Biogeochemistry: phosphorus solubilisation in rewetted soils. Nature 411:258. doi: 10.1038/35077146
Van der Grift, B. (2017). Geochemical and Hydrodynamic Phosphorus Retention Mechanisms in Lowland Catchments. Ph.D. thesis, Universiteit Utrecht.
Van der Grift, B., Behrends, T., Osté, L. A., Schot, P. P., Wassen, M. J., and Griffioen, J. (2016). Fe hydroxyphosphate precipitation and Fe(II) oxidation kinetics upon aeration of Fe(II) and phosphate-containing synthetic and natural solutions. Geochim. Cosmochim. Acta 186, 71–90. doi: 10.1016/j.gca.2016.04.035
Van der Grift, B., Osté, L., Schot, P. P., Kratz, A., Van Popta, E., Wassen, M., et al. (2018). Forms of phosphorus in suspended particulate matter in agriculture-dominated lowland catchments: iron as phosphorus carrier. Sci. Total Environ. 631–632, 115–129. doi: 10.1016/j.scitotenv.2018.02.266
Van der Grift, B., Rozemeijer, J. C., Griffioen, J., and van der Velde, Y. (2014). Iron oxidation kinetics and phosphate immobilization along the flow-path from groundwater into surface water. Hydrol. Earth Syst. Sci. 18, 4687–4702. doi: 10.5194/hess-18-4687-2014
Van der Linden, P., and Mitchell, J. F. (2009). ENSEMBLES Climate, Change, and Its Impacts: Summary of Research and Results from the, ENSEMBLES Project. Exeter: Met Office Hadley Centre, 1–160.
Vinten, A., Sample, J., Ibiyemi, A., Abdul-Salama, Y., and Stutter, M. (2017). A tool for cost-effectiveness analysis of field scale sediment-bound phosphorus mitigation measures and application to analysis of spatial and temporal targeting in the Lunan Water catchment, Scotland. Sci. Total Environ. 586, 631–641. doi: 10.1016/j.scitotenv.2017.02.034
Voegelin, A., Senn, A. C., Kaegi, R., Hug, S. J., and Mangold, S. (2013). Dynamic Fe-precipitate formation induced by Fe(II) oxidation in aerated phosphate-containing water. Geochim. Cosmochim. Acta 117, 216–231. doi: 10.1016/j.gca.2013.04.022
Wellen, C., Kamran-Disfani, A. R., and Arhonditsis, G. B. (2015). Evaluation of the current state of distributed watershed nutrient water quality modeling. Environ. Sci. Technol. 49, 3278–3290. doi: 10.1021/es5049557
Whitehead, P. G., and Crossman, J. (2012). Macronutrient cycles and climate change: key science areas and an international perspective. Sci. Total Environ. 434, 13–17. doi: 10.1016/j.scitotenv.2011.08.046
Whitehead, P. G., Wade, A. J., and Butterfield, D. (2009). Potential impacts of climate change on water quality and ecology in six UK rivers. Hydrol. Res. 40, 113–122. doi: 10.2166/nh.2009.078
Withers, P. J., van Dijk, K. C., Neset, T. S., Nesme, T., Oenema, O., Rubæk, G. H., et al. (2015b). Stewardship to tackle global phosphorus inefficiency: the case of Europe. Ambio 44, 193–206. doi: 10.1007/s13280-014-0614-8
Withers, P. J. A., Elser, J. J., Hilton, J., Ohtake, H., Schippere, W. J., and van Dijk, K. C. (2015a). Greening the global phosphorus cycle: how green chemistry can help achieve planetary P sustainability. Green Chem. 17, 2087–2099. doi: 10.1039/C4GC02445A
Withers, P. J. A., Neal, C., Jarvie, H. P., and Doody, D. G. (2014). Agriculture and eutrophication: where do we go from here? Sustainability 6, 5853–5875. doi: 10.3390/su6095853
Wu, Y., Liu, J., Shena, R., and Fub, B. (2017). Mitigation of nonpoint source pollution in rural areas: from control to synergies of multi ecosystem services. Sci. Total Environ. 607–608, 1376–1380. doi: 10.1016/j.scitotenv.2017.07.105
Xie, H., Chen, L., and Shen, Z. (2015). Assessment of agricultural best management practices using models: current issues and future perspectives. Water 7, 1088–1108. doi: 10.3390/w7031088
Yang, B., Liu, S. M., and Zhang, J. (2016). Phosphorus speciation and availability in sediments off the eastern coast of Hainan Island, South China Sea. Cont. Shelf Res. 118, 111–127. doi: 10.1016/j.csr.2016.03.003
Yao, Q. Z., Du, J. T., Chen, H. T., and Yu, Z. G. (2017). Particle-size distribution and phosphorus forms as a function of hydrological forcing in the Yellow River. Environ. Sci. Poll. Res. 23, 3385–3398. doi: 10.1007/s11356-015-5567-3
Keywords: phosphorus, cycling, soil, eutrophication, climate change, colloidal and particulate, water quality
Citation: Bol R, Gruau G, Mellander P-E, Dupas R, Bechmann M, Skarbøvik E, Bieroza M, Djodjic F, Glendell M, Jordan P, Van der Grift B, Rode M, Smolders E, Verbeeck M, Gu S, Klumpp E, Pohle I, Fresne M and Gascuel-Odoux C (2018) Challenges of Reducing Phosphorus Based Water Eutrophication in the Agricultural Landscapes of Northwest Europe. Front. Mar. Sci. 5:276. doi: 10.3389/fmars.2018.00276
Received: 26 March 2018; Accepted: 20 July 2018;
Published: 23 August 2018.
Edited by:
Rosalind Jane Dodd, Lincoln University, New ZealandReviewed by:
Sönke Hohn, Leibniz Centre for Tropical Marine Research (LG), GermanyVerena Pfahler, Rothamsted Research (BBSRC), United Kingdom
Copyright © 2018 Bol, Gruau, Mellander, Dupas, Bechmann, Skarbøvik, Bieroza, Djodjic, Glendell, Jordan, Van der Grift, Rode, Smolders, Verbeeck, Gu, Klumpp, Pohle, Fresne and Gascuel-Odoux. This is an open-access article distributed under the terms of the Creative Commons Attribution License (CC BY). The use, distribution or reproduction in other forums is permitted, provided the original author(s) and the copyright owner(s) are credited and that the original publication in this journal is cited, in accordance with accepted academic practice. No use, distribution or reproduction is permitted which does not comply with these terms.
*Correspondence: Roland Bol, ci5ib2xAZnotanVlbGljaC5kZQ==