- 1School of Geographical and Earth Sciences, University of Glasgow, Glasgow, United Kingdom
- 2School of GeoSciences, University of Edinburgh, Edinburgh, United Kingdom
Mass coral bleaching events during the last 20 years have caused major concern over the future of coral reefs worldwide. Despite damage to key ecosystem engineers, little is known about bleaching frequency prior to 1979 when regular modern systematic scientific observations began on the Great Barrier Reef (GBR). To understand the longer-term relevance of current bleaching trajectories, the likelihood of future coral acclimatization and adaptation, and thus persistence of corals, records, and drivers of natural pre-industrial bleaching frequency and prevalence are needed. Here, we use linear extensions from 44 overlapping GBR coral cores to extend the observational bleaching record by reconstructing temperature-induced bleaching patterns over 381 years spanning 1620–2001. Porites spp. corals exhibited variable bleaching patterns with bleaching frequency (number of bleaching years per decade) increasing (1620–1753), decreasing (1754–1820), and increasing (1821–2001) again. Bleaching prevalence (the proportion of cores exhibiting bleaching) fell (1670–1774) before increasing by 10% since the late 1790s concurrent with positive temperature anomalies, placing recently observed increases in GBR coral bleaching into a wider context. Spatial inconsistency along with historically diverging patterns of bleaching frequency and prevalence provide queries over the capacity for holobiont (the coral host, the symbiotic microalgae and associated microorganisms) acclimatization and adaptation via bleaching, but reconstructed increases in bleaching frequency and prevalence, may suggest coral populations are reaching an upper bleaching threshold, a “tipping point” beyond which coral survival is uncertain.
Introduction
Mass coral bleaching events during the last 20 years have caused major concern over the future of coral reefs worldwide (Hughes et al., 2017). Despite these mass bleachings, little is known about bleaching frequency prior to 1979 when regular modern systematic scientific observations on the Great Barrier Reef (GBR, Figure 1) began. The underlying mechanisms of contemporary coral bleaching are known to cause a breakdown of the coral-algae symbiosis (Glynn, 1991; Hoegh-Guldberg, 1999), but the contribution of specific mechanisms are still debated (Weis, 2008). Stressors such as thermal increases/decreases, irradiance extremes, disease and freshwater runoff, along with their combined effects, can cause coral bleaching with an associated reduction in coral growth (Hendy et al., 2003; Fabricius, 2005; Wiedenmann et al., 2013). However, warm sea surface temperatures (SSTs), such as those driven by El Niño Southern Oscillation are often correlated with mass bleaching (Clark et al., 2014) and have been well documented in conjunction with major widespread bleaching events in 1998 and 2002 (Cantin and Lough, 2014). The relevance of temperature is such that, using spatial thermal histories, coral reefs at risk of bleaching can now be forecast (Liu et al., 2006; Logan et al., 2014; NOAA, 2018). Forecasting of these events by NOAA Coral Reef Watch (NOAA's monitoring for coral reef ecosystems) is set to become more advanced with the inclusion of other key factors such as light stress through the Light Stress Damage (LSD) product suite, which accounts for rapid changes in light availability (e.g., going from cloudy to clear days in conjunction with rising temperatures) and the acclimatization time of the coral to such changes (Skirving et al. 2018). As the photoacclimatory response of the Symbiodinium is integral to the holobiont (the coral host, the symbiotic microalgae and associated microorganisms; Rosenberg et al., 2007; Mieog et al., 2009) and susceptibility to thermal stress (Bay et al., 2017; Scheufen et al., 2017; Skirving et al., 2018), further research and modeling of the interplay between temperature and light stress, and how this leads to bleaching is crucial. While bleaching events may include bleaching leading to mortality, sub-lethal bleaching, where the coral bleaches but recovers, may act as a “safety valve” allowing coral hosts to survive briefer periods of thermal stress in warmer waters (Suggett and Smith, 2011) providing a possible mechanism for coral hologenome acclimatization and adaptation (Kenkel and Matz, 2016). Additionally, there is evidence that thermal acclimation by corals can occur with minimal symbiont clade replacement (Palumbi et al., 2014).
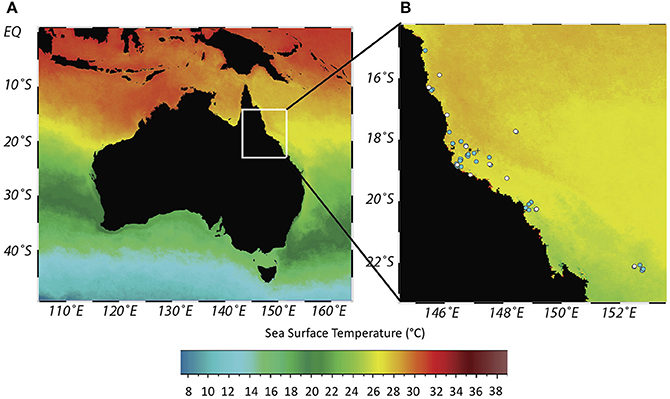
Figure 1. Sea surface temperature (SST) chart of Australia (A) and location of coral cores from Great Barrier Reef (GBR) (B). Dot shading indicates locations where coral cores bleached (white dot)/did not bleach (blue dot) in synchrony with either ENSO, PDO or SOI extremes over a 503 (ENSO, PDO) or 138 (SOI) year period depending on core length. Rib and Kelso reefs denoted by * and + respectively. Constructed using Ocean Data View. Core locations detailed in Table SM1. Overlaid Sea Surface Temperature (Celsius) at 4 km resolution from Aqua MODIS 11 μ Daytime 2016 annual composite (NASA Goddard Space Flight Center Ocean Biology Processing Group, 2017). Overlaid annual composite is indicative to show the presence of a thermal gradient across the GBR.
Advances in reconstructing bleaching beyond the observational record have highlighted that there may have been at least 2 mass bleaching events since the last glacial maximum which occurred ~20 kyr BP and prior to the industrial revolution (Dishon et al., 2015). Additionally, coral deaths during 1865-1979 in the South China Sea may have been caused by El Niño-associated warming (Yu et al., 2006, 2012), and also, death of branching Acropora sp. on the GBR over the last century has increased (Clark et al., 2017). However, as studies reconstruct past coral mortality, it is still not possible to accurately assess if corals will survive in the warmer oceans projected for the end of the century (Hoegh-Guldberg, 1999; Hoegh-Guldberg et al., 2007; Van Hooidonk et al., 2013; Bay et al., 2017) as we do not understand their ability to bleach and survive over centennial time scales through generational-scale acclimatization and adaptation processes. Bleaching reconstructions have also been attempted from cores using carbon and oxygen isotopes (Heikoop et al., 2000; Suzuki et al., 2003; Hetzinger et al., 2016) as well as Sr/Ca ratios (Marshall and Mcculloch, 2002), however, the balance between kinetic (e.g., simultaneous depletion/enrichment of two isotopes due to non-bleaching drivers), metabolic and environmental factors or the influence of stress on carbonate mineralogy complicates the use of these as proxies.
At present, observational records of coral bleaching extend back a few decades, and while these data have been valuable, they only provide us with a short time window within which to place recent rapid bleaching increases (Bellwood et al., 2004) into a longer temporal context. Thus, to understand corals' ability to bleach and survive over centennial time scales, continuous historic records of widespread coral bleaching at centennial time scales are needed. The use of coral cores in assessing biogeochemical markers of past environmental conditions (Chalker et al., 1985; Lough and Barnes, 2000; Mcculloch et al., 2003; Crueger et al., 2009; Lough, 2011; Zinke et al., 2014a,b; Tierney et al., 2015), contemporary biological events (De'ath et al., 2009; Cantin and Lough, 2014) and growth rates (Carilli et al., 2010b, 2012; Carricart-Ganivet et al., 2012; Hetzinger et al., 2016; D'Olivo and Mcculloch, 2017) are well documented and provide a mechanism to reconstruct historic GBR bleaching. Skeletal linear extension rates in coral cores can be affected by various localized and widespread stressors, such as river runoff, high turbidity, changes in nutrients and in particular temperature-induced bleaching (Goreau and Macfarlane, 1990; Leder et al., 1991; Lough and Barnes, 2000; Hendy et al., 2003; Fabricius, 2005; Carilli et al., 2012; Carricart-Ganivet et al., 2012; Wiedenmann et al., 2013; Cantin and Lough, 2014; Hetzinger et al., 2016; D'Olivo and Mcculloch, 2017). We reconstruct centennial-scale bleaching frequency and prevalence using changes in linear extension to consider recent bleaching trends in an environmentally relevant context, through comparison of pre- and post-industrialisation (late 1800s) bleaching during a period of rapid environmental change (IPCC, 2013). To identify the reductions in linear extension rate that correspond to potential temperate-induced bleaching events over the past four centuries, we use multiple selection criteria; (1) we set minimum linear extension change “thresholds” by calibrating changes in coral linear extension rates to observational temperature records, (2) we assessed cores over large spatial regions, and (3) we imposed a “bleaching event” threshold, where we only identify a bleaching event as one where a certain number of coral cores met all threshold conditions. Through such an approach, potential impacts of localized events become secondary to larger, reef-scale environmental processes. This temperature-induced bleaching reconstruction does not indicate a severity of bleaching in individual coral colonies, and is reliant upon a bleaching event being severe enough to reduce coral growth past a calibrated threshold. Consequently, mild and isolated bleaching events are not reconstructed, and bleaching events severe enough to cause mortality would not be recorded, as coral cores used here were alive at time of collection.
Methods
To infer and quantify the frequency and prevalence of bleaching events 1575–2001, we use historical changes in linear extension rates indicative of bleaching (Carilli et al., 2012; Cantin and Lough, 2014; Hetzinger et al., 2016; D'Olivo and Mcculloch, 2017) from GBR (Figure 1) Porites spp. coral cores [as per Veron (2000) may include Porites lobata, Porites australiensis, Porites lutea, Porites evermanni, Porites solida, Porites myrmidonensis, Porites mayeri]. Cores used in this study were previously used to identify decreases in calcification on the GBR (De'ath et al., 2009), as seawater temperature proxies (Lough and Barnes, 1997, 2000) and for past environmental interrogation (Chalker et al., 1985). It is important to note that skeletal linear extension rates in individual cores can be affected by various localized effects, such as river runoff, high turbidity, or changes in nutrients (Hendy et al., 2003; Fabricius, 2005; Wiedenmann et al., 2013) so comparisons are conducted both on individual cores and GBR wide. Sampling followed the workflow summarized in Figure 2 and detailed here.
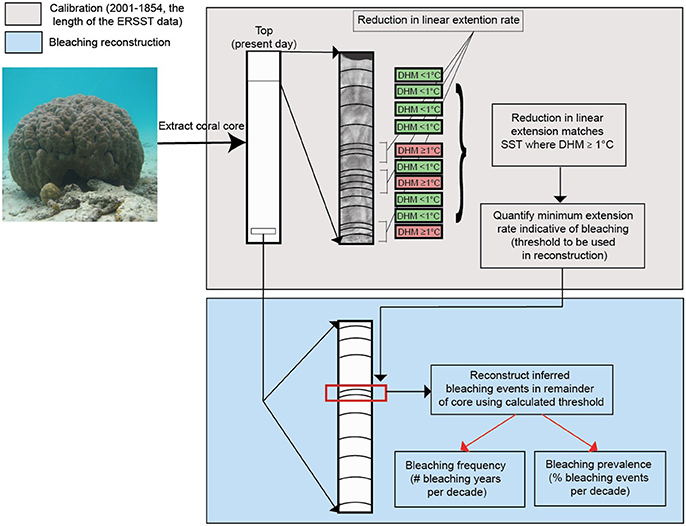
Figure 2. Methodology workflow. Coral linear extension obtained from databases as detailed in methods. The upper gray box illustrates reconstructed bleaching calibration process using ERSST data, DHM (°C) indicates degree heating months. The lower blue box illustrates reconstruction of bleaching.
Coral growth: Porites spp. annual extension length from the GBR were obtained from The Great Barrier Reef Coral Growth Database (Pages WDCA., 2015) with submissions by Chalker et al. (1985), Lough and Barnes (1997, 2000), and De'ath et al. (2009). Fifty-nine coral cores were initially assessed with 44 coral cores finally used (see below), covering the period 1572–2001 with the individual cores ranging from 32 to 416 years in length. Only coral core linear extension data were used with no interpolation of data between sites where coral cores were unavailable.
Bleaching Threshold, Degree Heating Months and Environmental Data
Site specific monthly SSTs were calculated for each coral using NOAA extended reconstructed SST (ERSST) from ship and buoy data (1854–2013) (Smith et al., 2008). ERSST data were from different dataset cells for offshore and inshore reefs [e.g., Abraham (152E 22S) vs. N Molle Is (150E 20S)]. Degree heating months (DHM, °C month−1 Donner et al., 2005) experienced by each coral, and the bleaching threshold (Glynn and D'croz, 1990) were calculated following Donner et al. (2005) where a DHM is equal to 1 month of SST that is 1°C above the maximum mean monthly (MMM) for that grid cell. A DHM of 1°C is equivalent to a degree heating week (DHW) of 4°C, at which a lower intensity bleaching threshold is reached (Donner et al., 2005). DHWs, as a measure of accumulated heat stress have been significant proven indicators of bleaching (Eakin et al., 2010), while on their own DHWs do not predict the severity of bleaching exhibited by individual coral colonies.
Bleaching and Coral Extension
Corals have distinct annual growth bands (Knutson et al., 1972) visible in X-rays; in massive Porites spp. bleaching events cause a reduction in annual extension length (Carilli et al., 2012; Cantin and Lough, 2014). Coral skeletons are thus capable of providing a history of bleaching events assuming the absolute age of the coral is known, there are accompanying environmental data, and multiple corals/sites are used (Cantin and Lough, 2014). In addition, as massive coral species (e.g., Porites spp.) are more resilient to bleaching (Marshall and Baird, 2000; Loya et al., 2001), they are more likely to have survived historic bleaching events while recording evidence of them in their skeletons. As a consequence of this, bleaching reconstruction based upon massive Porites spp. will be conservative relative to the bleaching that may have been evident in other coral species.
Calibration of Years Indicative of Bleaching
This was conducted over the period of coral growth that coincided with 1854–2001, the length of the ERSST data. For each Porites spp. core individually, extension length in years where a site-specific DHM ≥1°C month−1 was experienced by the coral was used as the extension length attainable during a bleaching year by that specific coral. In most cases, corals experienced 2–5 such years over the calibration period. In those cases, the minimum growth rate attained in any of the DHM ≥1°C years was used as the threshold extension attainable during a bleaching year. Critically, coral extension rates attained during DHM ≥1°C years were lower than those during DHM <1°C years, validating the calculated extension threshold for each coral. Cores that did not have years of growth which coincided with site-specific DHM ≥1°C years (for example because recorded DHM ≥1°C years only occurred after they were cored) were excluded as they could not be calibrated (this reduced the cores available from 59 to 44).
Reconstruction
1) Annually resolved coral extension length time series were detrended using linear regression allowing assessment of historic bleaching events that may have been obscured by (a) ontogenetic, (b) temperature (to remove the impact of historically lower temperatures prior to our calibration which would have artificially inflated historic bleaching frequency and prevalence), and (c) latitudinal dependent growth trends (Lough and Barnes, 2000; Ridd et al., 2013). ERSSTs were not detrended as this would have artificially inflated historic bleaching events by bringing historic ERSST closer to the bleaching threshold (ERSST has natural trend while contemporary bleaching threshold does not). In addition, coral extension was not directly compared with ERSST but rather coral extension was compared with modern bleaching thresholds and then bleaching threshold was compared with ERSST.
2) Over the calibration period, years were assigned as possible bleaching years when DHM ≥1°C.
3) Before the calibration period when no ERSST data are available, for each coral, when extension rate fell below the extension threshold calculated during the calibration, that year was assigned as a possible bleaching year. To account for slow recovery of individual corals after reconstructed bleaching and prevent a reduction in growth caused by single bleaching event (Carilli et al., 2012; Cantin and Lough, 2014; D'Olivo and Mcculloch, 2017) being counted multiple times, potential bleaching events in individual corals present in consecutive years (up to 3 years were observed) were only counted as a single event. Subsequent reference to “evidence of bleaching” is used here to describe reductions in coral growth below the calibrated DHM threshold. Additionally, this process prevented us from spuriously attributing bleaching to years with low growth caused by low temperatures; this is because by using the DHM growth threshold, corals would be unlikely to persist in temperatures so low that their growth was consistently below growth generated during bleached years. Thus, as we only used corals that survived bleaching, our analysis resulted in conservative values of bleaching, as lethal bleaching (bleaching resulting in mortality) will have removed corals from the population before they were sampled. Similarly, only cores with complete banding were used to remove any influence of growth anomalies associated with partial coral mortality. Our reconstruction is unlikely to be influenced by GBR sea level changes over the last 4 centuries as GBR sea levels have been constant for the past 6000 years and even average increases of 1.2 mm yr−1 since 1959 around Townsville are within the 3 mm yr−1 corals can accommodate without influences on reef growth (GBRMPA, 2018).
Widespread Coral Bleaching Threshold
Here, we only considered bleaching events that were evidenced in ≥20% of corals bleaching in any individual year across all cores assessed. This was to (1) ensure spatiotemporally isolated bleaching events in particular cores did not overinflate decadal bleaching frequency/prevalence (e.g., one bleaching event in each of 10 years with each in a different core) and (2) to remove the weight of spatially restricted bleaching (e.g., from flood plumes, or from cores experiencing high light stress in relation to other cores from the same region) not affecting the whole GBR. While at present, there is not an agreed prevalence “threshold” to define a widespread bleaching event, we chose 20%, as 21% is the lowest threshold that was classified as a widespread bleaching event on the GBR (during 1997/8) (AIMS, 2014).
Proxy Validation: Comparison of Reconstructed Bleaching With Other Records
Observational percentage bleaching time series for only Porites spp. corals are not available for large spatial areas of the GBR, thus preventing direct comparison of reconstructed and observed bleaching over long time scales. To validate our bleaching proxy we used 3 approaches:
Comparison of Reconstructed Bleaching With Reconstructed SST
Our bleaching reconstruction was compared to a SST reconstruction for the western Pacific (Tierney et al., 2015) which included the GBR. The SST reconstruction is generated from coral cores, with only one site (Abraham reef) providing SST on the GBR (using a different core to this study); the majority of data come from further north in the western Pacific region which covers 25°N−25°S, 110–155°E. Data provided by the GBR core are SST derived from δ18O and not growth band inferred temperature, thus making our bleaching reconstruction independent of the SST reconstruction. We correlated reconstructed SST (Tierney et al., 2015) and reconstructed bleaching prevalence (defined below) in 10 yr bins (to allow for the influence of non-thermal bleaching) between 1700 and 1980, the time scale over which 3 cores or more were available and thus core number did does not drive bleaching (for this validation, logistic regression was conducted on the bleaching and core number time series by pooling all GBR corals; Carilli et al., 2010a). The reconstructed SST record (Tierney et al., 2015) only included SST from years during which environmental temperature was the unambiguous driver of growth in the sample corals; this meant errors in δ18O -temperature driven by bleaching of those corals (Rodrigues and Grottoli, 2006) were not included in their record avoiding non-independence between SST and reconstructed bleaching. In addition, bleaching was compared to reconstructed Southern hemisphere surface temperature (Mann et al., 2008) which were generated using “composite plus scale” methodology using corals, marine and lacustrine series, tree-rings, speleothems, ice core, and historical documentary series. While this reconstruction is hemispheric, including terrestrial proxies, it is the longest reconstruction available and provides a contextual trend. It should be noted that the reconstruction also included coral extension rates so is thus not fully independent of our reconstruction, we therefore do not compare the two statistically. Similarly, as we use ERSST to reconstruct bleaching, we do not compare bleaching and ERSST statistically.
Years With Larger Than Average Bleaching Prevalence
We compared reconstructed bleaching prevalence (at annual resolution) to the observational bleaching record for the GBR between 1979 and 2001 (the overlap period between the two records). Most GBR observational data available post 1982 have a wide GBR spatial coverage (Oliver et al., 2009) however, as observational data are for all coral species and not effort adjusted this comparison demonstrates which year shows the highest percentage bleaching in both datasets but is not correlative. When 7 or more coral cores were present, there was no effect of coral number on the percentage of corals showing bleaching at annual resolution, so only the period 1979–2000 was considered [Linear model: F(2, 19) = 2.458, p = 0.112].
Comparison With Other Porites spp. Reconstructed Bleaching Datasets
We compared evidence of bleaching (bleaching presence/absence) during the 1998 mass bleaching event in our reconstructed bleaching record with independent bleaching evidence generated from coral extension records from Rib and Kelso Reef short cores (Cantin and Lough, 2014).
GBR-Wide Bleaching Patterns
While other variables are known to reduce growth/cause bleaching (e.g., disease, competition, flood plumes), temperature is the most common trigger that induces sudden, widespread, bleaching events across the whole GBR (Hoegh-Guldberg, 1999). All of these variables, which may act as “multiple stressors” involved in bleaching events will vary spatially and temporally (Berkelmans et al., 2004). To ensure any patterns we observed were driven by thermal bleaching; (1) we averaged across all corals spanning the whole GBR so spatial and temporal variability in bleaching forcing was minimized next to the potentially larger impact of temperature perturbations. (2) We introduced a 20% threshold (see above) where we consider environmentally-driven bleaching events as occurring in a meaningful proportion of corals across the GBR, rather than isolated individuals in different locations. (3) We decadally binned reconstructed bleaching frequency and prevalence to allow for missed growth years and growth band misidentification in the coral cores. This is because individual corals may bleach for varying reasons (e.g., disease, genotypic susceptibility, flood plumes) and not represent wider, environmentally-driven bleaching. Thus, any growth irregularities or sampling bias in individual cores will have little influence on regional scale, decadal observations. Our approach meets the criteria suggested by Cantin and Lough (2014). In this context the degree of GBR wide bleaching is demonstrated by the bleaching prevalence statistic (% of corals bleached); this gives an indication of the % corals bleached on the GBR but clumping of those locations may be present due the location of available cores in any decade.
Bleaching Frequency and Prevalence
The percentage of corals whose extension length indicated possible bleaching were determined. Percentages were not weighted for the number of corals available as this would artificially inflate possible bleaching prevalence. Rather, (1) only years with bleaching above the 20% threshold were considered and (2) decades with less than two cores (for frequency analysis) or three cores (for prevalence analysis) were not considered in subsequent analyses.
Frequency
Within each 10-year bin, the number of years in which at least 20% of the corals showed evidence of possible bleaching were calculated.
Prevalence
The percentage of coral growth bands that showed evidence of bleaching within each 10-year bin were calculated (excluding those that demonstrated slow recovery post individual bleaching events). Only those above the 20% bleaching threshold were considered.
Determining the Influence of Core Numbers on Frequency and Prevalence Time Series
To determine if the number of cores available drove the reconstructed bleaching frequency and prevalence patterns, logistic regression was conducted on the bleaching and core number time series by pooling all GBR corals. The number of cores were not significant in driving the observed patterns when greater than two cores (for decadal frequency analysis) or three cores (for decadal prevalence analysis) were available (frequency: Z2 (df), 39 (total df) = −0.77, p = 0.442, prevalence: Z2, 37 = −1.26, p = 0.205). Thus, when considering changes in bleaching, only years were used where at least two cores (for frequency) or three cores (for prevalence) were available.
Detecting Changes in Bleaching Over Time
Changes in bleaching trajectories (breakpoints) were used to detect the timing of changes in bleaching frequency (1620–2001) and prevalence (1640–2001) only during time periods over which at least two cores (for frequency) or three cores (for prevalence) were available. Breakpoints were detected using segmented regression as bleaching patterns were not constant temporally.
Site-Specific Bleaching Patterns
We used generalized linear mixed models fit with Laplace approximation to determine if each coral's annually resolved bleaching time series (time as random variable) was driven by three large scale climatic processes: (1) El Niño Southern Oscillation (ENSO) (Li et al., 2011) (up to 503 years depending on core length), (2) Pacific Decadal Oscillation (PDO) (Mann et al., 2009) (up to 503 years depending on core length), and (3) Southern Oscillation Index (SOI) (Können et al., 1998) (up to 138 years depending on core length). All climatic index data were obtained from the NOAA National Climatic Data Center (https://www.ncdc.noaa.gov).
For this approach, individual coral bleaching histories were used as coral locations covered the whole GBR (Figure 1, Table SM1) which has a spatial thermal gradient (De'ath et al., 2009), so spatial responses may vary. Additionally, temperature alone was not compared to bleaching in individual coral colonies as site-specific annual resolution coral bleaching may be driven by factors other than temperature, e.g., flood plumes which vary spatially (Berkelmans et al., 2004), or changes in light intensity (Scheufen et al., 2017) through spatiotemporal changes in cloud cover or water turbidity. Large scale climatic processes integrate such variables along with temperature and allow for a more robust assessment of the drivers of historic coral bleaching.
Statistical Analysis
All analyses were conducted in Rv3.1 (RRCT., 2014) using R Studio (Rstudio, 2014). Segmented analysis was conducted using the Segmented package (Muggeo, 2008) and generalized linear mixed models with the lme4 package (Bates et al., 2014).
Results
Validation of Bleaching Reconstruction
Reconstructed bleaching shows decadal correlation (r = 0.621, p < 0.001) with reconstructed SST for the western Pacific (Figure 3). Both our reconstructed and the observational GBR bleaching records show maximum bleaching during 1998 across the whole GBR (Figure 3B). In addition, at the individual reef scale (Rib and Kelso Reefs) both our and the Cantin and Lough (2014) bleaching reconstruction show no bleaching during 1998 despite overall widespread GBR bleaching.
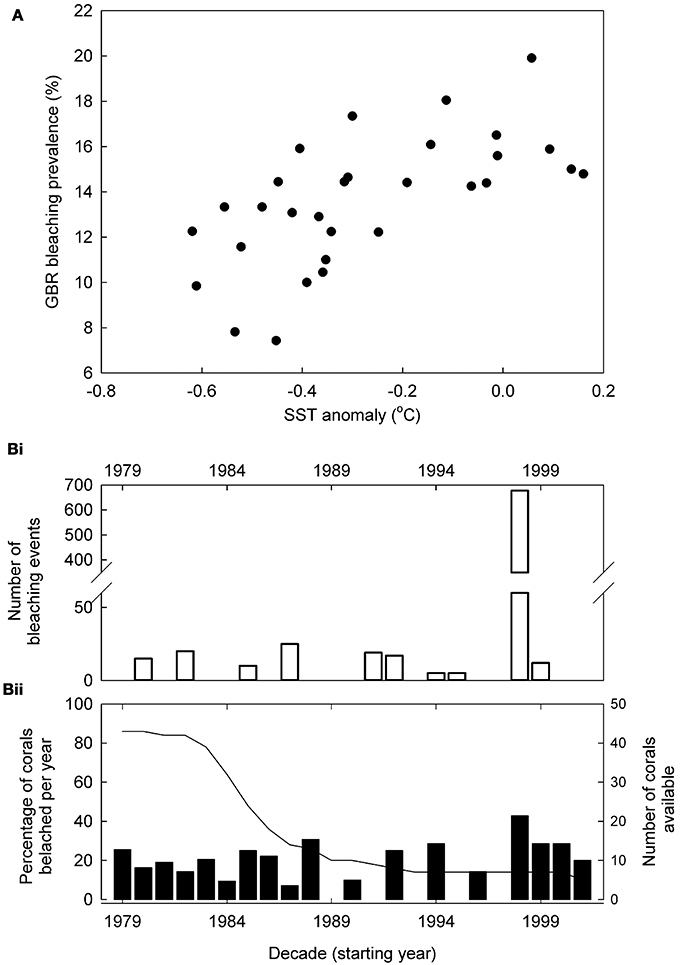
Figure 3. Reconstructed bleaching compared to other records. (A) Bleaching prevalence—temperature responses; decadally binned Great Barrier Reef (GBR) reconstructed bleaching prevalence responses to reconstructed western Pacific (Tierney et al., 2015) sea surface temperature (SST) between 1700 and 1989 (correlation: r = 0.621, p < 0.001). (B) Annual coral bleaching rates on the GBR since 1979; (Bi) Number of observed coral bleaching events (Wilkinson, 2006). These data also include non-Porites sp. so will overestimate Porites spp. bleaching. (Bii) GBR reconstructed Porites spp. bleaching prevalence (%, bars) and number of coral cores used (line). Where the growth effects of a single bleaching event lasted several successive years, this was classified as a single bleaching event of individual corals.
GBR-Wide Bleaching Patterns
Reconstructed bleaching was evident through the entire available record from 1575 to 2001. To determine any GBR-wide bleaching patterns, corals were pooled from all regions in the GBR spanning 15.13 to 22.23°S. Quantitative assessments of bleaching were conducted between 1620 and 2001 for frequency and 1640–2001 for prevalence. Outside of these periods, while we have documented historic bleaching, it is possible that the number of cores available drove the patterns observed.
Reconstructed bleaching frequency demonstrated three trends; (1) frequency increased from 1620 to 1753 ± 31 (years) reaching up to 6 years of each decade showing evidence of bleaching in at least 20% of coral cores. (2) Bleaching frequency decreased to 1820 ± 31 when only 1 year of every decade had evidence of bleaching in at least 20% of coral cores. (3) Bleaching frequency increased again from 1820 ± 31 to 2001. The 1890 and 1750 decades were notable for unusually high bleaching frequencies (Figure 4B, Table SM2). In contrast to bleaching frequency, bleaching prevalence only demonstrated two trends; (1) a negative trend from 1640 – 1774 ± 78, with prevalence falling from 41 to 21%. 2) An increase in bleaching prevalence from 21% in 1774 ± 78 to 31 % in 2001 (Figure 4C, Table SM2).
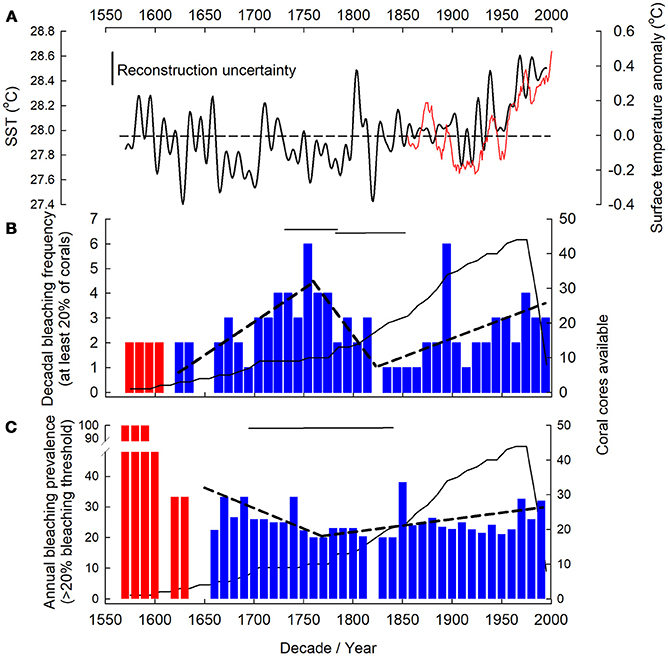
Figure 4. Massive Porites spp. bleaching frequency and prevalence. NOAA extended sea surface temperature (SST) for Great Barrier Reef (GBR) (A) from ship and buoy data (1854–2000, 11 yr moving average, red line) (Smith et al., 2008). Southern hemisphere surface temperature anomaly (ocean and land) reconstruction (Mann et al., 2008) (solid line) with the uncertainty (0.23°C) on the reconstruction indicated (the reconstruction includes coral extension rates so is not fully independent of our reconstruction). Dashed line indicates mean temperature over record length (1570–1995). Bleaching frequency (B) (number of years per decadal bin in which bleaching occurred) and prevalence (C) (% of corals bleached per decade) observed in at least 20% of coral for the GBR denoted by blue bars. Red color bars indicate years in which less than 2 (frequency)/3 (prevalence) coral cores were available; those years were excluded from further analysis (see Supplementary Material). Number of coral cores available in each decade indicated by solid black line. Dashed black line indicates breakpoint determined linear trend in bleaching and horizontal solid black lines represent breakpoint location 95%CI. For (B) decade notation marks the start of the bin for frequency and each coral core (n = 44) contributed up to 10 annual growth extensions per decadal bin.
Site-Specific Bleaching Patterns
There were no consistent annually resolved site-specific differences in reconstructed bleaching in response to large scale climatic patterns (ENSO, PDO, and SOI) between or within different locations on the GBR (Figure 1B, Table SM1) over 138–503 year long periods (depending on core length). Only 12 of 44 coral cores showed evidence of bleaching in synchrony with large scale climatic processes. Bleaching was synchronous with ENSO extremes in six corals (Snapper core 01A, Normanby 01B, Britomart 01B, Pandora 04A, Wheeler 01A, Stanley 01A), PDO extremes in six corals (Agincourt 01B, Britomart 01B, Pandora 04A, Lupton Island 01A, Lupton Island 01C, Abraham 01H) and SOI extremes in five corals (Findlers 01A, Pandora 04A, Magnetic Island 01D, Stanley 01A, Abraham 01H).
Discussion
At higher SSTs, we show increased bleaching prevalence across the GBR; this is the expected response and indicates a consistent reconstructed bleaching pattern of massive Porites spp. corals to warming between 1770 and 1989. Significantly, if SST was not causing bleaching, growth would be higher in warmer years (Lough and Barnes, 2000) as long as heat-stress was not incurred, and no correlation would be present. Both our reconstructed and the observational GBR bleaching records show maximum bleaching during 1998 across the whole GBR (Figure 3) although this is not scaled for observational effort. In addition, at the individual reef scale (Rib and Kelso Reefs) both our and the Cantin and Lough (2014) bleaching reconstruction show no bleaching during 1998 despite overall widespread GBR bleaching. These similarities of our bleaching reconstruction to the observational bleaching record and local reef responses at different spatial scales further validate our approach to bleaching reconstruction.
There are some individual years during which reconstructed bleaching does not match the observational record. These differences may be due to (1) massive Porites spp. being less susceptible to bleaching than other coral species (Marshall and Baird, 2000) causing differences in bleaching presence/intensity between the observed (all coral sp.) and our reconstructed record. (2) The observational bleaching records may be related to lethal beaching, and where not, subjective determination of bleached and non-bleached state may influence reported bleaching prevalence in observational records. Conversely, reconstructed bleaching is quantitative and focuses on non-lethal bleaching likely explaining some annual scale differences. (3) Microclimatic influences and light stress (see below). (4) Local adaptation in individual corals (Kenkel and Matz, 2016). (5) The observational record not being scaled for observational effort.
There is evidence of GBR bleaching since at least 1575 (Figures 4B,C). As all the corals were cored whilst live, this bleaching does not represent lethal bleaching events, but rather sub-lethal bleaching with a measurable decrease in growth before recovery. With reconstructed bleaching occurring in more than 20% of corals this bleaching represents widespread bleaching across the GBR, and since 1774 ± 78, the prevalence of those bleaching events has increased by 10%. This is concomitant with a trend for positive hemispheric temperature anomalies since the late 1700s (Figure 4A), more pronounced GBR SST increases since the late 1800s (Smith et al., 2008), along with wider southern hemisphere marine and terrestrial surface temperatures increases (Mann et al., 2008; IPCC, 2013; Tierney et al., 2015). Here we observed a 10% increase in bleaching prevalence since the mid 1700s, while other studies show more significant increases since the 1980s (Bellwood et al., 2004). We attribute this difference to our bleaching reconstruction being intrinsically conservative, through the use of massive Porites spp. corals that are robust to bleaching and have survived historic bleaching events, using a centennial-length (as opposed to previous decades) bleaching baseline to place recent bleaching into context, and because we impose a 20% minimum bleaching threshold when attributing bleaching years. In addition, this 10% increase is unlikely to be attributed to sea level rise as sea levels were stable on the GBR until the mid-1950s (GBRMPA, 2018).
Bleaching frequency and prevalence did not consistently show the same trends (Figure 4); for example, the reduction in bleaching prevalence but variable bleaching frequency (increase and subsequent decrease) during the period 1620–1750s, when average temperatures were not increasing (Figure 4A), suggests a possible adaptive and/or acclimatory change in the coral holobiont through changes to the dominant symbiont type, potential evolution of the algal symbiont itself over subsequent generations (Lajeunesse, 2005), or through host plasticity (Bellantuono et al., 2012). Acclimatization of the host, through changes in their associated symbionts and microbes, may lead to a period of reduced bleaching prevalence until changes in environmental conditions once again push the host closer to its acclimatory capacity. Although mechanisms for past coral holobiont adaptation remain unknown, they could account for decreased bleaching prevalence observed here, and remain a key area for future research given the importance of this question with regard to present day bleaching events and trajectories. The presence of such adaptation mechanisms will remain important processes for corals to keep pace with projected increased warming (Logan et al., 2014).
While acclimatization may have occurred in the past leading to reduced bleaching frequency, this occurred during a period of relative temperature stability compared to present times. The increase in bleaching frequency and prevalence post 1850, where temperatures were on average increasing, may indicate that corals are coming closer to the uppermost limit of their thermal acclimatization and adaptive capacity. Although the coral holobiont can acclimatize to bleaching stressors over time (Grottoli et al., 2014; Palumbi et al., 2014; Kenkel and Matz, 2016; Bay et al., 2017), multiple or chronic stressors can effect holobiont fitness and bleaching resilience (Carilli et al., 2009; Grottoli et al., 2014). This may be why bleaching prevalence has been exacerbated in recent times due to additional human induced stressors (Carilli et al., 2010b) overlaying the average increase in temperature (Figure 4A) [although widespread stressors can still cause significant bleaching (e.g., temperature and light alone and synchrony)]. Given the combined rise in bleaching frequency and prevalence documented here, the potential for future acclimatization and adaptation of the coral holobiont, through plasticity (Kenkel and Matz, 2016), host acclimatization (Palumbi et al., 2014), changes in symbiont community or symbiont evolution over relatively rapid timescales is uncertain. At slower rates of change there may, however, be scope for heat tolerance via rapid evolution (Bay et al., 2017).
However, within the general trend of increased bleaching prevalence from 1770s to 2001, there are still periods of low prevalence and frequency, e.g., from the 1820 to 1830s. This reduction follows an abrupt drop in 1820s temperature (Figure 4A) suggesting that when stressors are low the reduced stress leads to reduced bleaching or the potential for a temporary increase in coral fitness and bleaching resilience through a break from chronic stress (Carilli et al., 2009), possibly driven by holobiont plasticity (Kenkel and Matz, 2016).
Absence of consistent bleaching patterns in response to large scale climatic processes (ENSO, PDO and SOI) highlights that (1) their role on GBR bleaching may be localized via the specific nature of the stressor they generate (e.g., runoff), (2) other processes, e.g., water runoff impacting particular corals (Hendy et al., 2003; Clark et al., 2014), light stress variability (Scheufen et al., 2017) through water runoff (turbidity) or cloud cover, and local adaptation to environmental variability (Kenkel and Matz, 2016) may also influence bleaching at local scales (West and Salm, 2003) and 3) similarly to 2), SSTs during bleaching can be patchy even if driven by large scale climatic processes. This suggests that while large-scale climatic patterns may drive bleaching at large spatial scales (GBR wide) depending on the stressor they produce, this may be modulated by corals responding to their localized environment or weather (Hughes et al., 2017) with additional potential variability between genetic individuals, and spatial hologenome variation within individuals. In those instances, multiple cores taken from a single colony (top and side of individuals Lough et al., 1999) may have different extension rates driven by differences in light availability. As it is known that the bleaching response is impacted through both light and thermal stress (Hennige et al., 2011; Coelho et al., 2017; Scheufen et al., 2017) it is reasonable to assume that multiple cores from within a single colony, taken from shaded and well-lit regions may not provide the same bleaching response, although single cores are nearly always taken from the top, well-lit, region of the colony so this should not affect our observations.
The response of individual reefs or regions to thermal stress may also differ, as they could have been subjected to varying degrees of light stress. Whilst new advances are being made in combining light and thermal stress into new coral bleaching forecasting (Skirving et al., 2018), we currently cannot reconstruct historic light stress or its synergistic effect with thermal stress. With new advances in forecasting algorithms and further work into discerning the impact of thermal and light stress (at varying degrees) on coral extension rates, future studies may be able to delineate the “temperature-induced coral bleaching” presented here further.
Our reconstruction includes corals from inshore and offshore areas of the GBR. It is possible that inshore corals may have experienced more exposure to terrestrial run-off than offshore corals dependent on their proximity to point runoff sources. Large scale climatic processes (ENSO, PDO, SOI) are important in driving both thermal and precipitation patterns, yet despite this, no consistent spatial patterns (inshore vs. offshore) were present over the timescale of our reconstruction (Figure 1B) as discussed previously.
We have had a limited understanding of centennial scale bleaching dynamics, time scales that are key for determining the future survival of corals reefs in a changing world via adaptive and acclimation processes. Here we show that corals bleached pre-industrially, but subsequently, bleaching has intensified. Importantly, reconstructions here are based on tolerant coral species and conservative threshold values, meaning that frequency and prevalence of bleaching experienced by other species may have been higher. While the processes of acclimatization and adaptation indicate that, given enough time, the coral holobiont may be able to adapt to new, higher thermal levels (Bay et al., 2017), post 1850 increases in bleaching frequency and prevalence suggest corals may struggle to survive in the future as conditions continue to rapidly change. Our results thus place recently observed increases in GBR coral bleaching into a wider temporal context; bleaching has been occurring on the GBR at least during the last 4 centuries, and has increased 10% in prevalence since the 1790s. Over the coming century, rapid environmental change will include multiple-stressors (Hoegh-Guldberg et al., 2007; Hofmann and Schellnhuber, 2009; Bay et al., 2017), and the increase in prevalence and frequency indicate that we may be coming to a tipping point beyond which survival in uncertain.
Author Contributions
NK and SH conceived the study, conducted the analysis, and wrote the paper.
Funding
Independent Research Fellowships were funded by NERC for NK and SH (NE/H010025, NE/K009028/1 and NE/K009028/2) and the Royal Society of Edinburgh/Scottish Government to NK (RSE 48701/1).
Conflict of Interest Statement
The authors declare that the research was conducted in the absence of any commercial or financial relationships that could be construed as a potential conflict of interest.
Acknowledgments
IGBP PAGES/WDCA, NOAA National Climate Data Centre, NASA and ReefBase curated and provided data. Where appropriate, sampling permits are acknowledged in the original submissions and host database (cited in section Methods). We also thank Jessica Carilli, Sandy Tudhope, and Joanie Kleypas for helpful suggestions.
Supplementary Material
The Supplementary Material for this article can be found online at: https://www.frontiersin.org/articles/10.3389/fmars.2018.00283/full#supplementary-material
References
AIMS (2014). Coral Bleaching Events [Online]. Available online at: https://www.aims.gov.au/docs/research/climate-change/coral-bleaching/bleaching-events.html (Accessed June 1, 2014).
Bates, D., Maechler, M., Bolker, B., and Walker, S. (2014). lme4: Linear Mixed-Effects Models Using Eigen and S4. R Package Version 1.1-7. Available online at: http://CRAN.R-project.org/package=lme4
Bay, R. A., Rose, N. H., Logan, C. A., and Palumbi, S. R. (2017). Genomic models predict successful coral adaptation if future ocean warming rates are reduced. Sci. Adv. 3:e1701413. doi: 10.1126/sciadv.1701413
Bellantuono, A. J., Hoegh-Guldberg, O., and Rodriguez-Lanetty, M. (2012). Resistance to thermal stress in corals without changes in symbiont composition. Proc. R. Soc. B Biol. Sci. 279, 1100–1107. doi: 10.1098/rspb.2011.1780
Bellwood, D. R., Hughes, T. P., Folke, C., and Nyström, M. (2004). Confronting the coral reef crisis. Nature 429:827. doi: 10.1038/nature02691
Berkelmans, R., De'ath, G., Kininmonth, S., and Skirving, W. J. (2004). A comparison of the 1998 and 2002 coral bleaching events of the Great Barrier Reef: spatial correlation, patterns and predictions. Coral Reefs 23, 74–83. doi: 10.1007/s00338-003-0353-y
Cantin, N. E., and Lough, J. M. (2014). Surviving coral bleaching events: porites growth anomalies on the Great Barrier Reef. PLoS ONE 9:e88720. doi: 10.1371/journal.pone.0088720
Carilli, J., Donner, S. D., and Hartmann, A. C. (2012). Historical temperature variability affects coral response to heat stress. PLoS ONE 7:e34418. doi: 10.1371/journal.pone.0034418
Carilli, J., Godfrey, J., Norris, R. D., Sandin, S. A., and Smith, J. E. (2010a). Periodic endolithic algal blooms in Montastrea faveolata corals may represent periods of low-level stress. Bull. Mar. Sci. 86, 709–708. Available online at: https://goo.gl/kaHktH
Carilli, J. E., Norris, R. D., Black, B., Walsh, S. M., and Mcfield, M. (2010b). Century-scale records of coral growth rates indicate that local stressors reduce coral thermal tolerance threshold. Glob. Change Biol. 16, 1247–1257. doi: 10.1111/j.1365-2486.2009.02043.x
Carilli, J. E., Norris, R. D., Black, B. A., Walsh, S. M., and Mcfield, M. (2009). Local stressors reduce coral resilience to bleaching. PLoS ONE 4:e6324. doi: 10.1371/journal.pone.0006324
Carricart-Ganivet, J. P., Cabanillas-Terán, N., Cruz-Ortega, I., and Blanchon, P. (2012). Sensitivity of calcification to thermal stress varies among genera of massive reef-building corals. PLoS ONE 7:e32859. doi: 10.1371/journal.pone.0032859
Chalker, B., Barnes, D., and Isdale, P. (1985). Calibration of X-ray densitometry for the measurement of coral skeletal density. Coral Reefs 4, 95–100. doi: 10.1007/BF00300867
Clark, T. R., Roff, G., Zhao, J. X., Feng, Y. X., Done, T. J., Mccook, L. J., et al. (2017). U-Th dating reveals regional-scale decline of branching Acropora corals on the great barrier reef over the past century. Proc. Natl. Acad. Sci. U.S.A. 114, 10350–10355. doi: 10.1073/pnas.1705351114
Clark, T. R., Zhao, J.-X., Roff, G., Feng, Y.-X., Done, T. J., Nothdurft, L. D., et al. (2014). Discerning the timing and cause of historical mortality events in modern Porites from the great barrier reef. Geochim. Cosmochim. Acta 138, 57–80. doi: 10.1016/j.gca.2014.04.022
Coelho, V., Fenner, D., Caruso, C., Bayles, B., Huang, Y., and Birkeland, C. (2017). Shading as a mitigation tool for coral bleaching in three common Indo-Pacific species. J. Exp. Mar. Biol. Ecol. 497, 152–163. doi: 10.1016/j.jembe.2017.09.016
Crueger, T., Zinke, J., and Pfeiffer, M. (2009). Patterns of pacific decadal variability recorded by indian ocean corals. Int. J. Earth Sci. 98, 41–52. doi: 10.1007/s00531-008-0324-1
De'ath, G., Lough, J. M., and Fabricius, K. E. (2009). Declining coral calcification on the Great Barrier Reef. Science 323, 116–119. doi: 10.1126/science.1165283
Dishon, G., Fisch, J., Horn, I., Kaczmarek, K., Bijma, J., Gruber, D. F., et al. (2015). A novel paleo-bleaching proxy using boron isotopes and high-resolution laser ablation to reconstruct coral bleaching events. Biogeosciences 12, 5677–5687. doi: 10.5194/bg-12-5677-2015
D'Olivo, J. P., and Mcculloch, M. T. (2017). Response of coral calcification and calcifying fluid composition to thermally induced bleaching stress. Sci. Rep. 7:2207. doi: 10.1038/s41598-017-02306-x
Donner, S. D., Skirving, W. J., Little, C. M., Oppenheimer, M., and Hoegh-Guldberg, O. V. E. (2005). Global assessment of coral bleaching and required rates of adaptation under climate change. Glob. Change Biol. 11, 2251–2265. doi: 10.1111/j.1365-2486.2005.01073.x
Eakin, C. M., Morgan, J. A., Heron, S. F., Smith, T. B., Liu, G., Alvarez-Filip, L., et al. (2010). Caribbean corals in crisis: record thermal stress, bleaching, and mortality in 2005. PLoS ONE 5:e13969. doi: 10.1371/journal.pone.0013969
Fabricius, K. E. (2005). Effects of terrestrial runoff on the ecology of corals and coral reefs: review and synthesis. Mar. Pollut. Bull. 50, 125–146. doi: 10.1016/j.marpolbul.2004.11.028
GBRMPA (2018). Impacts of Sea Level Rise on the [Great Barrier] Reef (Great Barrier Reef Marine Park Authority. Available online at: http://www.gbrmpa.gov.au/managing-the-reef/threats-to-the-reef/climate-change/how-climate-change-can-affect-the-reef/sea-level-rise (Accessed May 3, 2018).
Glynn, P. W. (1991). Coral reef bleaching in the 1980s and possible connections with global warming. Trends Ecol. Evol. 6, 175–179. doi: 10.1016/0169-5347(91)90208-F
Glynn, P. W., and D'croz, L. (1990). Experimental evidence for high temperature stress as the cause of El Niño coincident coral mortality. Coral Reefs 8, 181–191. doi: 10.1007/BF00265009
Goreau, T. J., and Macfarlane, A. H. (1990). Reduced growth rate of Montastrea annularis following the 1987–1988 coral-bleaching event. Coral Reefs 8, 211–215. doi: 10.1007/BF00265013
Grottoli, A. G., Warner, M. E., Levas, S. J., Aschaffenburg, M. D., Schoepf, V., Mcginley, M., et al. (2014). The cumulative impact of annual coral bleaching can turn some coral species winners into losers. Glob. Change Biol. 20, 3823–3833. doi: 10.1111/gcb.12658
Heikoop, J. M., Dunn, J. J., Risk, M. J., Schwarcz, H. P., Mcconnaughey, T. A., and Sandeman, I. M. (2000). Separation of kinetic and metabolic isotope effects in carbon-13 records preserved in reef coral skeletons. Geochim. Cosmochim. Acta 64, 975–987. doi: 10.1016/S0016-7037(99)00363-4
Hendy, E. J., Lough, J. M., and Gagan, M. K. (2003). Historical mortality in massive Porites from the central Great Barrier Reef, Australia: evidence for past environmental stress?. Coral Reefs 22, 207–215. doi: 10.1007/s00338-003-0304-7
Hennige, S. J., Mcginley, M. P., Grottoli, A. G., and Warner, M. E. (2011). Photoinhibition of Symbiodinium spp. within the reef corals Montastraea faveolata and Porites astreoides: implications for coral bleaching. Mar. Biol. 158:2515. doi: 10.1007/s00227-011-1752-1
Hetzinger, S., Pfeiffer, M., Dullo, W.-C., Zinke, J., and Garbe-Schönberg, D. (2016). A change in coral extension rates and stable isotopes after El Niño-induced coral bleaching and regional stress events. Sci. Rep. 6:32879. doi: 10.1038/srep32879
Hoegh-Guldberg, O. (1999). Climate change, coral bleaching and the future of the world's coral reefs. Mar. Freshw. Res. 50, 839–866. doi: 10.1071/MF99078
Hoegh-Guldberg, O., Mumby, P. J., Hooten, A. J., Steneck, R. S., Greenfield, P., Gomez, E., et al. (2007). Coral reefs under rapid climate change and ocean acidification. Science 318, 1737–1742. doi: 10.1126/science.1152509
Hofmann, M., and Schellnhuber, H. J. (2009). Oceanic acidification affects marine carbon pump and triggers extended marine oxygen holes. Proc. Natl. Acad. Sci. U.S.A. 106, 3017–3022. doi: 10.1073/pnas.0813384106
Hughes, T. P., Kerry, J. T., Álvarez-Noriega, M., Álvarez-Romero, J. G., Anderson, K. D., Baird, A. H., et al. (2017). Global warming and recurrent mass bleaching of corals. Nature 543, 373–377. doi: 10.1038/nature21707
IPCC (2013). Climate Change 2013: The Physical Science Basis. Contribution of Working Group I to the Fifth Assessment Report of the Intergovernmental Panel on Climate Change, Cambridge: Cambridge University Press.
Kenkel, C. D., and Matz, M. V. (2016). Gene expression plasticity as a mechanism of coral adaptation to a variable environment. Nat. Ecol. Evol. 1:0014. doi: 10.1038/s41559-016-0014
Knutson, D. W., Buddemeier, R. W., and Smith, S. V. (1972). Coral chronometers: seasonal growth bands in reef corals. Science 177, 270–272. doi: 10.1126/science.177.4045.270
Können, G. P., Jones, P. D., Kaltofen, M. H., and Allan, R. J. (1998). Pre-1866 extensions of the southern oscillation index using early Indonesian and Tahitian meteorological readings. J. Clim. 11, 2325–2339. doi: 10.1175/1520-0442(1998)011<2325:PEOTSO>2.0.CO;2
Lajeunesse, T. C. (2005). “Species” radiations of symbiotic dinoflagellates in the Atlantic and indo-pacific since the miocene-pliocene transition. Mol. Biol. Evol. 22, 1158–1158. doi: 10.1093/molbev/msi042
Leder, J. J., Szmant, A. M., and Swart, P. K. (1991). The effect of prolonged “bleaching” on skeletal banding and stable isotopic composition in Montastrea annularis. Coral Reefs 10, 19–27.
Li, J., Xie, S.-P., Cook, E. R., Huang, G., D'arrigo, R., Liu, F., et al. (2011). Interdecadal modulation of El Niño amplitude during the past millennium Nat. Clim. Change 1, 114–118. doi: 10.1038/nclimate1086
Liu, G., Strong, A. E., Skirving, W. J., and Arzayus, L. F. (2006). “Overview of NOAA coral reef watch program's near-real-time satellite global coral bleaching monitoring activities,” in Proceedings of the 10th International Coral Reef Symposium (Okinawa), 1783–1793.
Logan, C. A., Dunne, J. P., Eakin, C. M., and Donner, S. D. (2014). Incorporating adaptive responses into future projections of coral bleaching. Glob. Change Biol. 20, 125–139. doi: 10.1111/gcb.12390
Lough, J. (2011). Measured coral luminescence as a freshwater proxy: comparison with visual indices and a potential age artefact. Coral Reefs 30, 169–182. doi: 10.1007/s00338-010-0688-0
Lough, J., Barnes, D., Devereux, M., Tobin, B., and Tobin, S. (1999). Variability in Growth Characteristics of Massive Porites on the Great Barrier Reef. CRC Reef Research Technical Report.
Lough, J. M., and Barnes, D. J. (1997). Several centuries of variation in skeletal extension, density and calcification in massive Porites colonies from the Great Barrier Reef: a proxy for seawater temperature and a background of variability against which to identify unnatural change. J. Exp. Mar. Biol. Ecol. 211, 29–67. doi: 10.1016/S0022-0981(96)02710-4
Lough, J. M., and Barnes, D. J. (2000). Environmental controls on growth of the massive coral Porites. J. Exp. Mar. Biol. Ecol. 245, 225–243. doi: 10.1016/S0022-0981(99)00168-9
Loya, Y., Sakai, K., Yamazato, K., Nakano, Y., Sambali, H., and Van Woesik, R. (2001). Coral bleaching: the winners and the losers. Ecol. Lett. 4, 122–131. doi: 10.1046/j.1461-0248.2001.00203.x
Mann, M. E., Zhang, Z., Hughes, M. K., Bradley, R. S., Miller, S. K., Rutherford, S., et al. (2008). Proxy-based reconstructions of hemispheric and global surface temperature variations over the past two millennia. Proc. Natl. Acad. Sci. U.S.A. 105, 13252–13257. doi: 10.1073/pnas.0805721105
Mann, M. E., Zhang, Z., Rutherford, S., Bradley, R. S., Hughes, M. K., Shindell, D., et al. (2009). Global signatures and dynamical origins of the little ice age and medieval climate anomaly. Science 326, 1256–1260. doi: 10.1126/science.1177303
Marshall, J. F., and Mcculloch, M. T. (2002). An assessment of the Sr/Ca ratio in shallow water hermatypic corals as a proxy for sea surface temperature. Geochim. Cosmochim. Acta 66, 3263–3280. doi: 10.1016/S0016-7037(02)00926-2
Marshall, P. A., and Baird, A. H. (2000). Bleaching of corals on the Great Barrier Reef: differential susceptibilities among taxa. Coral Reefs 19, 155–163. doi: 10.1007/s003380000086
Mcculloch, M., Fallon, S., Wyndham, T., Hendy, E., Lough, J., and Barnes, D. (2003). Coral record of increased sediment flux to the inner Great Barrier Reef since European settlement. Nature 421, 727–730. doi: 10.1038/nature01361
Mieog, J. C., Olsen, J. L., Berkelmans, R., Bleuler-Martinez, S. A., Willis, B. L., and van Oppen, M. J. (2009). The roles and interactions of symbiont, host and environment in defining coral fitness. PLoS ONE 4:e6364. doi: 10.1371/annotation/e06b31ef-6b29-44ae-aec1-1740daa93f4b
Muggeo, V. M. R. (2008). Segmented: an R package to Fit Regression Models With Broken-line Relationships. R News, 8/1, 20-25. Available online at: http://cran.r-project.org/doc/Rnews
NASA Goddard Space Flight Center OEL Ocean Biology Processing Group (2017). Moderate-Resolution Imaging Spectroradiometer (MODIS) Aqua Sea Surface Temperature 11μ Daytime Data. (Accessed May 26, 2017)
NOAA (2018). NOAA Coral Reef Watcht. Available online at: http://www.coralreefwatch.noaa.gov
Oliver, J. K., Berkelmans, R., and Eakin, C. M. (2009). “Coral bleaching in space and time,” in Coral Bleaching Patterns, Processes, Causes and Consequences, eds J. Lough and M. J. H. Van Oppen (Berlin: Springer-Verlag), 21–39.
Pages WDCA. (2015). From the NOAA National Climatic Data Centre. Available online at: https://www.ncdc.noaa.gov/paleo/study/1863
Palumbi, S. R., Barshis, D. J., Traylor-Knowles, N., and Bay, R. A. (2014). Mechanisms of reef coral resistance to future climate change. Science 344, 895–898. doi: 10.1126/science.1251336
Ridd, P. V., Da Silva, E. T., and Stieglitz, T. (2013). Have coral calcification rates slowed in the last twenty years?. Mar. Geol. 346, 392–399. doi: 10.1016/j.margeo.2013.09.002
Rodrigues, L. J., and Grottoli, A. G. (2006). Calcification rate and the stable carbon, oxygen, and nitrogen isotopes in the skeleton, host tissue, and zooxanthellae of bleached and recovering Hawaiian corals. Geochim. Cosmochim. Acta 70, 2781–2789. doi: 10.1016/j.gca.2006.02.014
Rosenberg, E., Koren, O., Reshef, L., Efrony, R., and Zilber-Rosenberg, I. (2007). The role of microorganisms in coral health, disease and evolution. Nat. Rev. Microbiol. 5, 355–362. doi: 10.1038/nrmicro1635
RRCT. (2014). A Language and Environment for Statistical Computing. R Foundation for Statistical Computing, Vienna. Available online at: http://www.R-project.org/
Rstudio (2014). RStudio: Integrated Development Environment for R (Version 0.96.122) [Computer software]. Boston, MA.
Scheufen, T., Krämer, W. E., Iglesias-Prieto, R., and Enríquez, S. (2017). Seasonal variation modulates coral sensibility to heat-stress and explains annual changes in coral productivity. Sci. Rep. 7:4937. doi: 10.1038/s41598-017-04927-8
Skirving, W., Enríquez, S., Hedley, J., Dove, S., Eakin, C., Mason, R., et al. (2018). Remote sensing of coral bleaching using temperature and light: progress towards an operational algorithm. Remote Sens. 10:18. doi: 10.3390/rs10010018
Smith, T. M., Reynolds, R. W., Peterson, T. C., and Lawrimore, J. (2008). Improvements to NOAA's historical merged land-ocean surface temperature analysis (1880-2006). J. Clim. 21, 2283–2296. doi: 10.1175/2007JCLI2100.1
Suggett, D. J., and Smith, D. J. (2011). Interpreting the sign of coral bleaching as friend vs. foe. Glob. Change Biol. 17, 45–55. doi: 10.1111/j.1365-2486.2009.02155.x
Suzuki, A., Gagan, M. K., Fabricius, K., Isdale, P. J., Yukino, I., and Kawahata, H. (2003). Skeletal isotope microprofiles of growth perturbations in Porites corals during the 1997–1998 mass bleaching event. Coral Reefs 22, 357–369. doi: 10.1007/s00338-003-0323-4
Tierney, J. E., Abram, N. J., Anchukaitis, K. J., Evans, M. N., Giry, C., Kilbourne, K. H., et al. (2015). Tropical sea surface temperatures for the past four centuries reconstructed from coral archives. Paleoceanog. Paleoclim. 30, 226–252. doi: 10.1002/2014PA002717
Van Hooidonk, R., Maynard, J. A., and Planes, S. (2013). Temporary refugia for coral reefs in a warming world. Nat. Clim. Change 3, 508–511. doi: 10.1038/nclimate1829
Veron, J. E. N. (2000). Corals of the World. Vol 1-3. Townsville, QLD: Australian Institute of Marine Science.
Weis, V. M. (2008). Cellular mechanisms of Cnidarian bleaching: stress causes the collapse of symbiosis. J. Exp. Biol. 211, 3059–3066. doi: 10.1242/jeb.009597
West, J. M., and Salm, R. V. (2003). Resistance and resilience to coral bleaching: implications for coral reef conservation and management. Conserv. Biol. 17, 956–967. doi: 10.1046/j.1523-1739.2003.02055.x
Wiedenmann, J., D'angelo, C., Smith, E. G., Hunt, A. N., Legiret, F. E., Postle, A. D., et al. (2013). Nutrient enrichment can increase the susceptibility of reef corals to bleaching. Nat. Clim. Change 3, 160–164. doi: 10.1038/nclimate1661
Wilkinson, C. (2006). Status of the Coral Reefs of the World: 2004. (Townsville, QLD: Australian Institute of Marine Science).
Yu, K., Zhao, J.-X., Shi, Q., and Price, G. J. (2012). Recent massive coral mortality events in the South China Sea: Was global warming and ENSO variability responsible?. Chem. Geol. 320–321, 54–65. doi: 10.1016/j.chemgeo.2012.05.028
Yu, K.-F., Zhao, J.-X., Shi, Q., Chen, T.-G., Wang, P.-X., Collerson, K. D., et al. (2006). U-series dating of dead Porites corals in the South China sea: evidence for episodic coral mortality over the past two centuries. Quat. Geochron. 1, 129–141. doi: 10.1016/j.quageo.2006.06.005
Zinke, J., Loveday, B. R., Reason, C. J., Dullo, W. C., and Kroon, D. (2014a). Madagascar corals track sea surface temperature variability in the Agulhas Current core region over the past 334 years. Sci. Rep. 4:4393. doi: 10.1038/srep04393
Keywords: coral, bleaching, adaptation, acclimatization, Symbiodinium, growth
Citation: Kamenos NA and Hennige SJ (2018) Reconstructing Four Centuries of Temperature-Induced Coral Bleaching on the Great Barrier Reef. Front. Mar. Sci. 5:283. doi: 10.3389/fmars.2018.00283
Received: 25 July 2017; Accepted: 25 July 2018;
Published: 15 August 2018.
Edited by:
Stefano Goffredo, Università degli Studi di Bologna, ItalyReviewed by:
Susana Enríquez, Universidad Nacional Autónoma de México, MexicoGabriel Grimsditch, United Nations Environment Programme (Kenya), Kenya
Douglas Fenner, NOAA NMFS Contractor, United States
Colleen A. Burge, University of Maryland, Baltimore County, United States
Daniel J. Thornhill, National Science Foundation (NSF), United States
Copyright © 2018 Kamenos and Hennige. This is an open-access article distributed under the terms of the Creative Commons Attribution License (CC BY). The use, distribution or reproduction in other forums is permitted, provided the original author(s) and the copyright owner(s) are credited and that the original publication in this journal is cited, in accordance with accepted academic practice. No use, distribution or reproduction is permitted which does not comply with these terms.
*Correspondence: Nicholas A. Kamenos, bmljay5rYW1lbm9zQGdsYXNnb3cuYWMudWs=