Phosphorus Contents Re-visited After 40 Years in Muddy and Sandy Sediments of a Temperate Lagoon System
- 1Biological Station Zingst, Institute of Biological Sciences, Department of Mathematics and Natural Sciences, University of Rostock, Germany
- 2Marine Chemistry, Leibniz Institute for Baltic Sea Research, Warnemünde, Germany
Worldwide, coastal water bodies suffer from anthropogenically elevated nutrient inputs, which led to eutrophication. Sediments in eutrophic systems are assumed to be an important internal nutrient source. The total phosphorus (TP) concentration (mg g-1 dry mass) is widely used as a proxy for the sediment nutrient load. 2-D distribution maps of TP concentrations are used for management plans, where areas of high TP values are marked in red. However, the sediment density is lowered at increasing water content, which can lead to different TP stocks per g m-2. The aim of this study was, to do a re-evaluation of TP concentrations and stocks in the model ecosystem of the Darß-Zingst Bodden chain, a typical lagoon system of the southern Baltic Sea. Sediment cores were taken at eight stations along transects from shallow to deeper parts of the lagoon. Samples were analyzed for TP, water and organic content, as well as density. This data set was compared to results from a sediment survey during the time of highest nutrient inputs (40 years ago) at the same sampling stations. TP concentrations from 40 years ago and today were in the same range. The highest TP concentrations (up to 0.6 mg TP g-1 dry mass) were found in the deeper basins and lowest concentrations in the shallow areas of the lagoon (down to 0.05 mg TP g-1 dry mass). However, normalization over dry bulk density (DBD) reversed some results. The highest TP stocks (up to 5 g TP m-2) were then found in the shallow areas and lowest stocks (down to 0.2 g TP m-2) in the deeper parts of the lagoon. Some stations did not exhibit any differences of TP at all, even after including the DBD. These findings suggest that there seems to be no up-, or downward trend in nutrient concentrations of sediments even after 25 years of reduced external nutrient inputs. Furthermore, TP stocks point to possible diffuse P entry pathways that counteract external nutrient reductions. These findings can have an impact on possible countermeasures for ecosystems rehabilitation, like sediment removal or nutrient reductions in the adjacent land.
Introduction
Along with the Green Revolution in agriculture in the last 50–75 years in the developed countries, ecosystems worldwide are strongly influenced by high anthropogenic nutrient inputs especially nitrogen (N) and phosphorus (P) (e.g., Smil, 2000; Liu et al., 2008; Schröder et al., 2010; Childers et al., 2011; Lu and Tian, 2017). Even the most optimistic future scenarios point to an overall increased use of P worldwide (Van Vuuren et al., 2010), i.e., overall use will most likely still lead to increased P inputs into aquatic ecosystems, despite of examples of reverse trends by effective reduction of P entering aquatic systems (e.g., Civan et al., 2018). Moreover, Yan et al. (2016) pointed to the fact that P seems to accumulate faster than N in aquatic systems, which means that even reduced P loads can still increase the P background within systems. This accumulated P has short and long-term effects in aquatic ecosystems. In the short term, the primary production within a system increased on a recurring annual basis. On a longer timeframe, P accumulates in sediments, where it can be remobilized occasionally (e.g., Ruttenberg, 2003). Consequently, P is trapped in the biological cycles until it is precipitated in more or less biologically insoluble forms. Such increases of insoluble forms of P in sediments are well described worldwide (e.g., Heathwaite, 1994; Jensen et al., 1995). Elevated P pools are still a burden for restoration, as they can be released massively during suboxia in near-bottom water layers or at a slower rate by diffusion. Such release situations are well described for, e.g., the Baltic proper and sediments of the Baltic coastal zones (Pitkänen et al., 2001). If P is released, it starts to refuel primary production again and the system will remain in its current state (Vahtera et al., 2007). Furthermore, Duarte et al. (2009) described this elevated P background as a main cause, why restoration will not work and ecosystems fail to return to the reference status upon nutrient reduction.
The Baltic Sea is such a highly anthropogenically impacted brackish water body, where the drainage basin (∼1,739,000 km2, Nilsson, 2006) is around four times larger than the sea (∼412,500 km2) itself. The nutrient loads into the Baltic Sea increased from the 1950s until peak values around 1980 followed by a decrease continuing up to present (Gustafsson et al., 2012). However, the inputs amounted to 1,056,922 tons of N and 41,163 tons of P in 1995 and 825,825 tons N and 30,949 tons P in 2014 (HELCOM, 2018). Nevertheless, P inputs into water bodies by point sources were reduced during the mid-1980s and are permanently low in German coastal water bodies since then. Therefore, this input reduction of up to 80% (Nausch et al., 2011) could meanwhile have impacted P stocks in sediments of the coastal water bodies. Coastal lagoon systems are of special interest, as they cover 5% of the entire Baltic coastal zone, but account for 10% of the P burial and half of the denitrification of all coastal ecosystems in the Baltic Sea (Asmala et al., 2017). Therefore, such lagoon systems can act as nutrient filter for the open Baltic Sea by trapping nutrients within their system. However, these reduced P inflows take only the reduction of direct river-borne P into account, but Germany’s federal state agencies indicated that diffuse P inputs became increasingly important during the last decades (BMUB and BMEL, 2016). In this context, Karstens et al. (2016) showed the impact of the adjacent land use on the wetland sediments in coastal systems, and Berthold et al. (2018a) described the possible direct export of P from adjacent wetlands into coastal water bodies. These diffuse P input could strongly affect the spatial distribution of P accumulation especially in dependency from coastal distance.
A well-described typical shallow lagoon system of the southern Baltic Sea is the DZBC (e.g., Schiewer, 2007), where a synoptic sediment study was conducted 40 years ago (e.g., Schlungbaum, 1982; Schlungbaum et al., 1994). At that time, sediment samples were taken in every lagoon part in a 500 to 1,000 m sampling grid in shallow and deep areas. Certain P hotspots were shown, often in deeper waters (e.g., Nausch and Schlungbaum, 1984). The sampling coincided with the peak of riverine TP inputs of up to 80 t a-1 during 1976 to 1980. Highest TP values were found in the deeper parts between 2 mg P g-1 DM in the inner lagoon, and up to 1 mg P g-1 DM in the outer lagoon. The LOI ranged between <1 and 40% (Nausch, 1981) depending on the sampling depth. Afterward, TP inputs by rivers were steadily reduced by 75% and are now for the last 20 years constant at 20 t a-1 (LUNG, 2013). Interestingly, these P reductions did not reduce turbidity, or phytoplankton biomass within the system, but the light limitation of phytoplankton seems to be so stable that small, light-efficient cyanobacteria (Schiewer, 2007; Albrecht et al., 2017) remain dominant. The original annual periodicity of phytoplankton composition disappeared, leaving the DZBC in a state of permanent high turbidity since the end of the 1980s (Schiewer, 2007). Such results indicate, that other P sources than direct inputs by rivers might explain the permanent high phytoplankton biomass. However, the DZBC is also described to possess a nutrient filter function, as the water exchange rate with the surrounding open Baltic sea is low (0.15 a-1 Schiewer, 2007), and the sediments sorption capacity for P is high (0.05–1.1 mg P g-1 DM, Schlungbaum, 1982). Furthermore, the particulate matter in this lagoon system has a high TN:TP (15-year median: 36–47:1, mol:mol) ratio, compared to other coastal water bodies of this region (Berthold et al., 2018b). This finding means that sedimented particulate material had a relatively low P status. Moreover, the sedimentation rate in this lagoon system is postulated to be between 1 (Lampe et al., 2013) and 5 mm a-1 (Nausch, 1981). Therefore, we hypothesized that the lowered P inputs had already an effect on the first centimeters of the sediment and re-evaluation of sediment P loads might reflect lowered P inputs into the lagoon. Such re-evaluations of sediments are exceptionally important, as the EU-Water Framework Directive and society have to consider the necessary time-spans for reducing anthropogenic impacts in aquatic ecosystems.
As mentioned, P concentrations (in mg g dry mass-1 or % of DM) in sediments are assumed to be important indicators for the total P load in an aquatic system and they are often used to create heat maps where hot spots of P- and other element-concentrations are described (e.g., Leipe et al., 2017). This approach could be questionable because sediment density can vary strongly due to the texture and water and organic matter content (Avnimelech et al., 2001; Köster and Meyer-Reil, 2001; Verstraeten and Poesen, 2001; Faas and Wartel, 2006) strongly affecting P stock in the sediment volume. Moreover, P stocks per m-2 seemed to be ecologically much more important, because the exchange at the sediment/water contact zone happens per area.
Due to the potential spatial and temporal differences in P loads of the sediments in the DZBC, sediments were sampled in transects in a right angle from the coastline, i.e., from shallow to the deeper areas across all lagoon basins. These samples were analyzed for bulk density, water and organic matter content and TP concentrations. From theses analyses, P stocks of sediment were calculated per area. All of these data were compared to the historical sample data 40 years ago. With this approach we want to answer the questions (1) if there is a gradient of increasing TP in the sediment from shore to deeper waters – both on a concentration or on a stock basis and (2) if surface TP changed within 40 years in line with decreasing P inputs into the lagoon system and the Baltic Sea.
Materials and Methods
Sampling Area
The DZBC is a temperate lagoon system with consecutively connected lagoon basins. The innermost lagoon parts Saaler Bodden (SB) and Bodstedter Bodden (BoB) are oligohaline (salinity 1–4) and highly eutrophic (Schiewer, 2007). The two outer lagoon parts Barther Bodden (BB) and Grabow (GB) are less influenced by riverine nutrient inputs (salinity 5–12) and less turbid (secchi depth up to 100 cm). Sampling spots were chosen for a gradient from shallow areas, proximate to the coastline to deeper parts of the lagoon. Two different spots were chosen per lagoon part. Additionally, samples from the outer southern Baltic coast line were taken as comparison. Those stations were located in Kühlungsborn, Zingst, island of Hiddensee and island of Usedom (Figure 1).
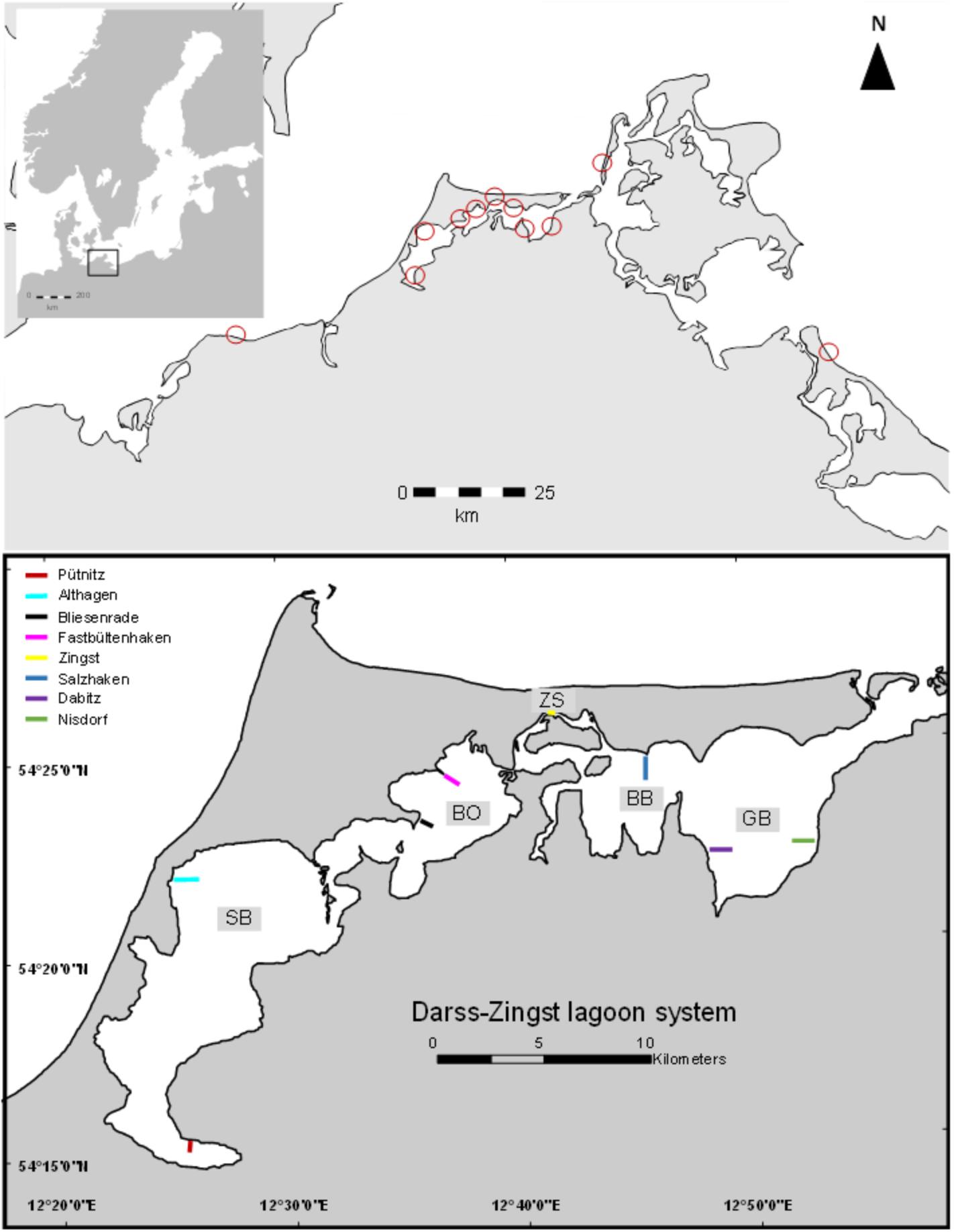
FIGURE 1. Map of the sampling area (drawn with ArcGIS version 10.2). Colored bars indicate samplings spots of conducted transects. GPS coordinates can be found in the Supplementary Table 1.
Sampling
The sedimentation rate in this lagoon system is postulated to be between 1 (Lampe et al., 2013) and 5 mm a-1 (Nausch, 1981). We used the lower sedimentation rate as a conservative approach to test our hypothesis. The time between the first sampling in 1977 and 2017 equals for 4 cm of increased sediment height. P inputs are on a constant low level for more than 27 years (=2.7 cm, LUNG, 2013). We sampled the upper first 1.4 cm (first 5 ml of a syringe), which is half of the sediment increase.
Sediment samples in the DZBC were taken with a sediment corer (10 cm diameter) (Hydrobios) between the water depths of 0.5 and 3.5 m, depending on the sampling site. Geographic coordinates for start and end point of each transect can be found in Supplementary Table 1. Sediment samples from the outer coast line were taken by divers, which used hand-held sediment corer with only 5 cm diameter. Six to eight sediment cores were taken along transects per sampling spot from shore to open water. Samples from the outer coast were taken with hand-held sediment tubes (10 cm diameter) by divers starting at water depths of 20 cm up to 5 m water depth. Three sub-samples of the first 1.4 cm were taken with a cylinder, which had a fixed volume, from the sediment cores. All samples were taken in 2017, except for a transect near Dabitz in the GB, which was already sampled in 2013 and included as an additional station. Additionally, sediment from this transect was separated in the horizons of 0–1, 1–2, and 2–5 cm.
Determination of Dry Bulk Density, Water, and Organic Matter Content
The fixed volume of a cylinder (syringe, 5 ml volume) was used to calculate DBD (g cm-3) after drying. Sub-samples were weighed wet, dried at 105°C for 24 h, and weighed dry again. The difference was used to calculate the WC (%) and DBD. Afterward, the sub-samples were combusted at 550°C for 4 h in a muffle furnace (Heraeus). The sediment ash of the sub-samples were weighed again after cooling down in a desiccator. The difference was used to calculate the organic content as LOI of the sediment. At least, the sub-samples of the sediment ash were pooled again and grinded in a powder mill (5,000 rpm, 2 min, Fritsch). The final grain size was between 1 and 10 μm. The dust was used for subsequent TP analyses.
Determination of Total Phosphorus
Ten to 50 mg of sediment ash were weighed in perfluoroalkoxy-polymer (PFA) tubes (AHF Analysentechnik) and suspended in 15 ml of ultrapure, phosphate-free water (power plant Rostock). Subsequently, 1.5 ml of acid persulfate was added, the samples were tightly closed and oxidized at 90°C for 24 h (Berthold et al., 2015). Afterward, the complete sample was neutralized and filtered (GF/F filter, Whatman). TP was determined with molybdenum blue according to Hansen and Koroleff (1999) with a photometer (Hach DR3900, 5 cm cuvette) at 885 nm. All sediment samples were digested and measured in quadruplicates.
The synoptic sediment study from 40 years ago collected amongst other parameters, WC, LOI, and TP concentrations. DBD was only sampled on a random basis and results were averaged for sediments with an LOI of <5% (1.5 g cm-3) and >5% (1.0 g cm-3). These mean DBD values were used to estimate the total P stock (in g P cm-2) in the first cm of the sediments of the lagoon system (Nausch, 1981). Instead, we took the historic values of WC and TP concentrations (only available as range) to re-calculate DBD and areal TP stocks (Equation 1) as such a relationship was missing in the studies from 40 years ago (see the section “Discussion”). The equation for areal TP stocks (g m-2) was calculated out of the TP concentrations (mg P g-1 DM sediment) and the relationship of WC (%) to sediment density (Figure 2A). The relationship of areal TP stocks to sediment densities are rather widely distributed (Figure 4), which affects this approach.
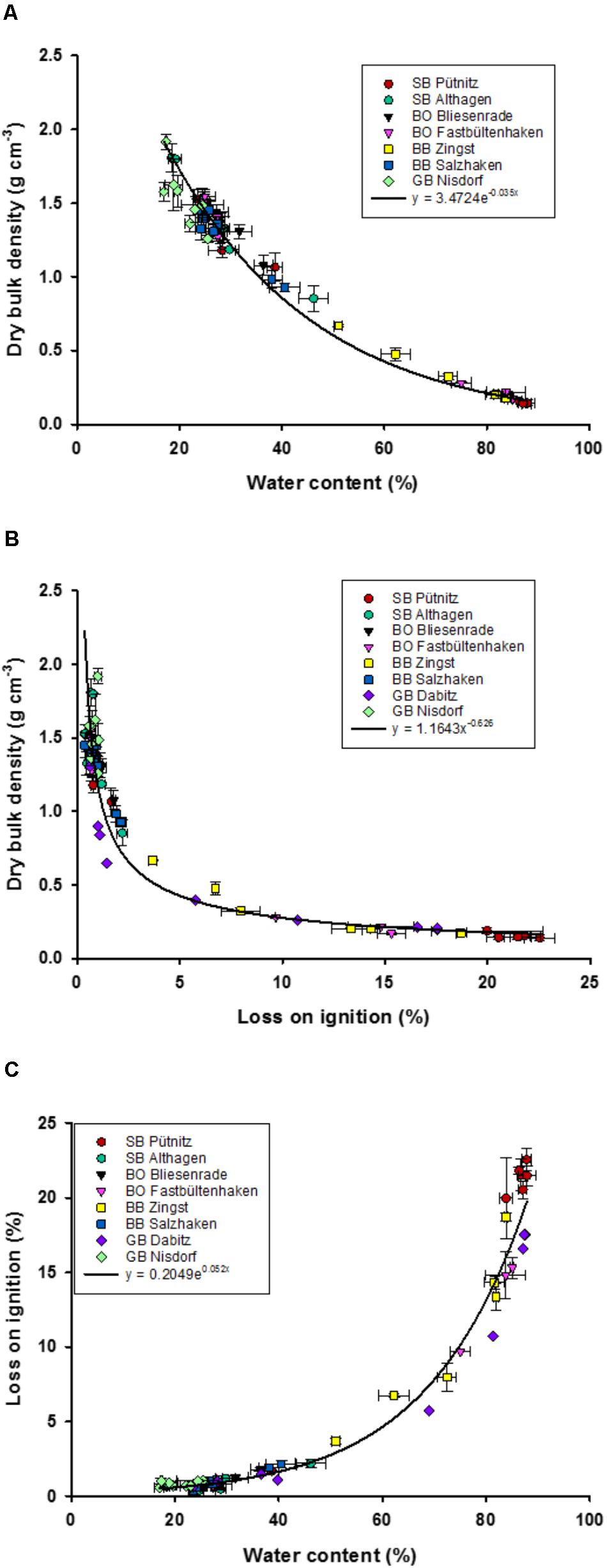
FIGURE 2. Relationships of dry bulk density (g dry mass cm-3) to water content (%) (A), and loss on ignition (%) (B), and water content (%) to loss on ignition (%) (C), in the upper sediment (1.4 cm). Fits were chosen to represent all data points, and not the highest coefficients of determinations (R2). Error bars are standard deviation of three replicate measurements.
Quality Management and Statistics
Determination (quantification) limit for TP was 0.22 μmol l-1 by the blank method in the digestate. Sediment samples were prepared to be above that limit and reach at least 2 μmol l-1 in the extract. The combined standard deviation was 11.8% for TP determination, which combines the random error of replicate samples and all standard values measured over the whole investigation period. This value describes reproducibility of both (samples and standards, i.e., random error of both).
SigmaPlot 13.0 was used for statistical analysis. Shapiro–Wilk tested all data for normal distribution. A Spearman Rank Order test was used for correlations. The principal component analysis (PCA) for the separation of sampling sites was computed for the water depth (in m), the LOI (in %), the DBD (in g cm-3), the total P concentration (in mg TP g-1) and the total P stocks of the first cm (in g TP cm-2) of the sediment samples using STATISTICA 6.0 (StatSoft, Inc., 2001).
Results
Characteristics and P-Content of Sediment Samples
The water depth of the 64 sediment samples of the eight transects ranged from 0.4 to 4 m. The WC ranged between 17 and 88% and the LOI between 0.036 and 23% LOI (Figure 2). Sediment samples from the outer coastline had a WC between 14 and 20% and a DBD of 1.4 to 1.7 g cm-3 (Supplementary Figure 1). At least one out of four samples had an LOI higher than 5%, while one third of those samples had a DBD below 1 g cm-3 (Figure 2). WC and LOI were significantly positively correlated (r = 0.91) and both negatively with DBD (r = –0.84 and – 0.74, respectively). Additionally, the sediment characteristics were different in shallow and deeper waters, since the water depth correlated significantly with WC (r = 0.52), LOI (r = 0.67), and DBD (r = –0.48).
The median of the P concentrations ranged from 0.05 to 1.1 mg TP g dry mass-1 and those of the P stocks from 0.24 to 5.6 g P cm-2 in the first cm of the sediment. The highest TP concentrations were found at higher LOI (Figure 3A). However, there was a huge variability, as some sediments with a high LOI had very low TP concentration per g DM. Low TP concentrations were measured at increasing DBD, i.e., lower WC and OC. In contrast, TP stocks were very variable at low LOI (Figure 3B). Nonetheless, LOI correlated significantly with both, TP concentrations (r = 0.4) and areal TP stocks (r = –0.42). Most of the samples with a low TP concentration and high WC and LOI originated from the station Zingst and were close to a harbor whereas others were from Pütniz, the innermost lagoon basin.
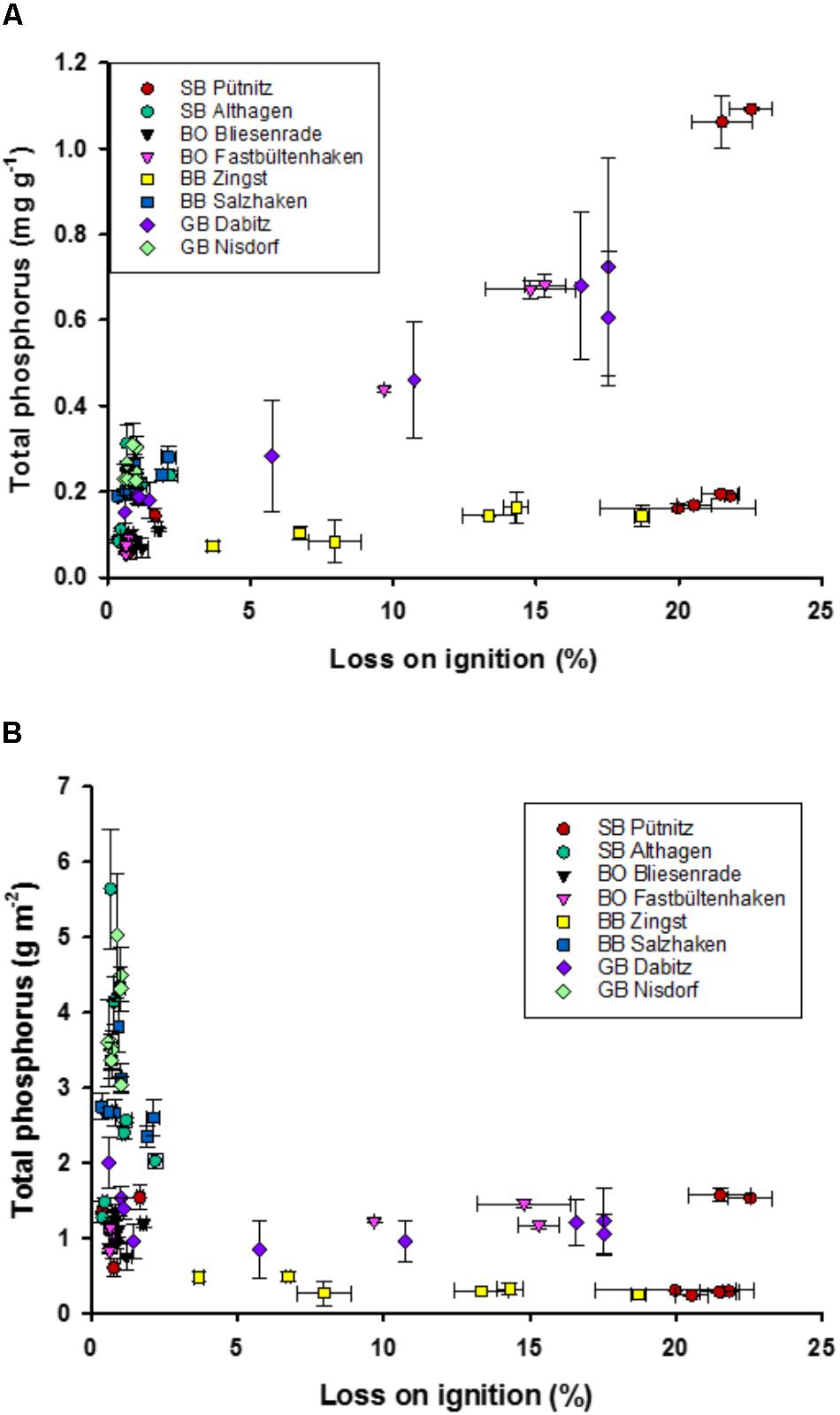
FIGURE 3. Relationship of total phosphorus concentration (TP) in g P dry mass-1 (A) and areal TP stock in g P m-2 (B) to loss on ignition (%). Error bars are standard deviation of three replicate measurements for organic content and four for TP.
There was less variation in TP stocks at lower DBDs compared to sampling sites with higher DBD (Figure 4). For example, samples from the lagoon part BoB had high areal TP stocks, compared to samples of lower DBD from deeper parts. In contrast, there were also samples with a DBD comparable to BoB (i.e., GB and BB), but those had three- to six-times higher areal TP stocks. Comparable TP stocks of 0.6–2.9 g TP m-2 at DBD’s from 1.3 to 1.6 were found at the outer coastline (Supplementary Figure 2). Interestingly, the stations with very high areal TP stocks were at the southern site of the lagoon, which is at the coast of the main land and receives riverine water. It becomes apparent that even stations in the most eutrophic part of the lagoon, i.e., SB and BoB, have comparably low areal TP stocks. The stations Fastbültenhaken and Bliesenrade, which are in the middle of the lagoon system, had the lowest variations, even though all samples originated from different depths. There were also outliers present in the innermost and outermost lagoon, i.e., Altenhagen and Nisdorf. Areal TP stocks of up to 6 g m-2 were found, but at water depths of around 2 m and with low LOI. DBD correlated positively and significantly with the areal TP stocks. Dabitz (GB) was the only transect with low as well as high DBD. At the same depth range the stations at the outer coast only varied in areal TP, but not in DBD (Supplementary Figure 1).
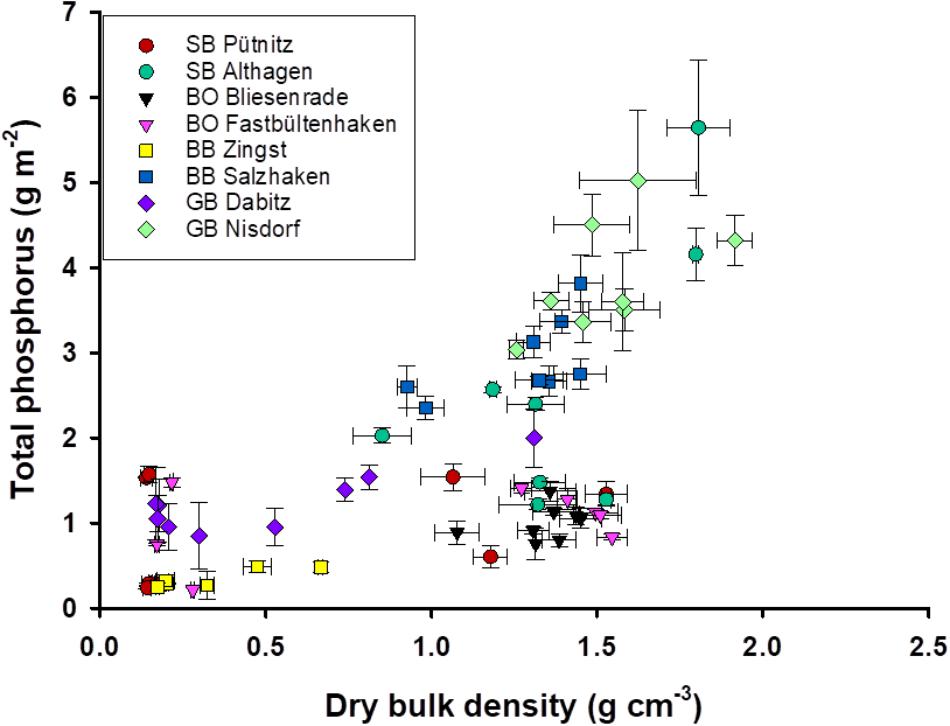
FIGURE 4. Total phosphorus stock (g P m-2) and dry bulk density (g dry mass cm-3). Error bars are standard deviation of three replicate measurements for DBD and four for TP.
Spatial Separation of Sampling Sites
Water depth, LOI, DBD, TP concentrations and TP stocks were included in the PCA to visualize separation of sampling sites in a two-dimensional plot (Figure 5). The first two PCs of the PCA explained together 82% and the first three PCs 95% of the variance between samples (Figure 5). Generally, the stronger the correlation of a factor to a specific PC (Table 1) the more the samples are shifted to the outward. Therefore, especially correlations of about ≥0.7 are highly important for separation of samples whereas correlations <0.5 are of low importance. Additionally, a negative correlation to a PC shifted samples to the left (PC 1) or downward (PC2) and a positive correlation to the right (PC1) or upward (PC2) (Figure 5). According to correlations (>0.9) of factors to PC1 (Table 1): the higher the LOI the more the samples are shifted to the right and the higher the DBD the samples are shifted to the left. The water depth had a correlation of 0.672 and the TP concentration of 0.635 to the PC1. That means that the deeper the water and the higher the TP concentrations the more the samples are shifted to the right but due to the lower correlations compared to LOI and DBD the shift is not so strong. The correlation of the TP stock to PC1 is the lowest of all factors and shifted to the opposite direction compared to the TP concentration (Tab. 1). However, TP concentrations and TP stocks were highest positively correlated to PC2 (Table 1). That means that samples with higher TP concentrations and/or TP stocks are shifted downward.
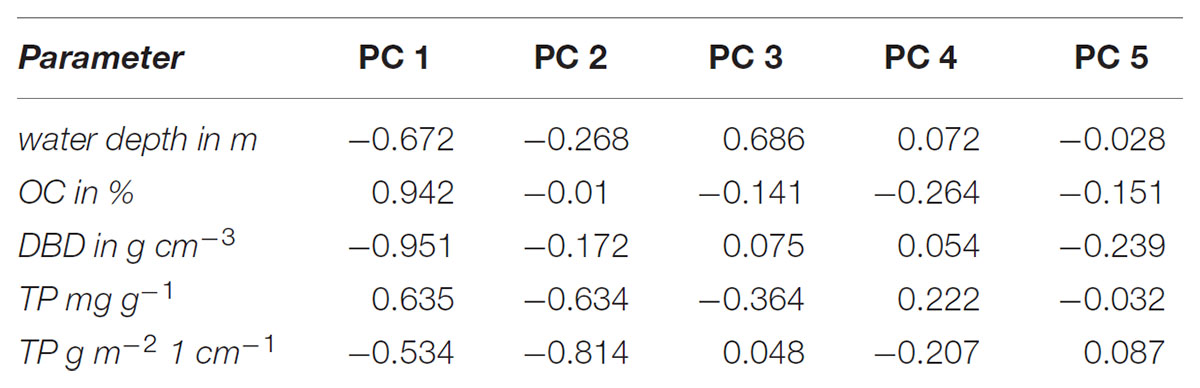
TABLE 1. Correlation of the parameters water depth, organic matter content (OC %), dry bulk density (DBD in g cm-3), total concentration of P (TP in mg g-1) and total P stocks in the first cm (TP in g m-2) with the principle components PC 1 to PC 5.
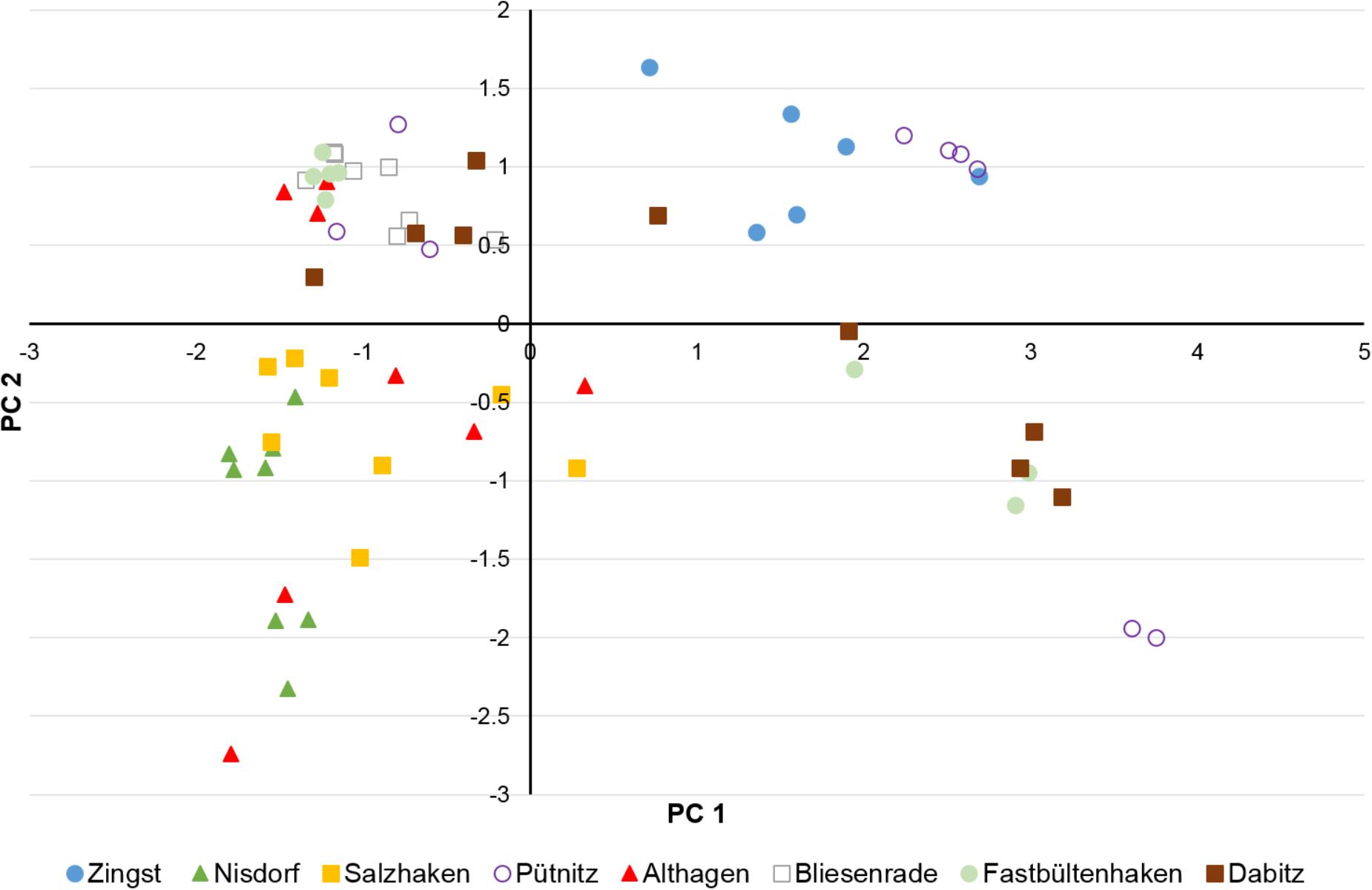
FIGURE 5. Score plot of the principle component analysis (PCA) of water depth, DBD, concentration of LOI and TP and TP stocks in the first cm of the sediment samples of the transects near Zingst, Nisdorf, Salzhaken, Pütnitz, Althagen, Bliesenrade, Fastbültenhaken, and Dabitz.
It is clearly visible that the sediment samples of Nisdorf (green triangles) and Salzhaken (yellow squares) and in parts those of Althagen (red triangles) are separated from the other sampling transects by shifting downward on the left side of the diagram (Figure 5). The shifting of these three transects was mainly caused by highest P stocks (1.2–5.6 g TP m-2) in combination with generally higher DBD (0.85–1.92 g cm-3) and lower LOI (0.36–2.19%) in contrast to the other transects (Figures 2–5). For the other transects the TP stocks ranged from 0.24 to 2.0 g TP cm-2, the DBD from 0.14 to 1.53 g cm-3 and the LOI from 0.36 to 22.5% (Figure 5). The transect Zingst (blue circles) was completely separated from Nisdorf, Salzhaken, and Althagen because the samples of Zingst had low TP stocks (maximum 0.49 g TP cm-2), low DBDs (maximum 0.67 g cm-3) and high LOIs (3.7–18.7%).
Sampling points of the transect Pütnitz (lilac circles) are widely spread at the score plot (Figure 5) because TP stocks (0.24–1.57 g TP cm-2), DBD (0.14–1.53 g cm-3), LOI (0.36–22.5%) and TP concentrations (0.06–1.09 mg TP g-1) varied strongly between samples (compare Figures 2, 4). Additionally, the six samples from Pütnitz transect with the highest LOI (minimum 20%) had the lowest DBD (maximum 0.19 g cm-3) in contrast to the three samples with lowest LOI (maximum 1.7%) and highest DBD (minimum 1.07 g cm-3). The spreading of the sampling points of the transect Dabitz (brown squares) was caused by the combination of high LOI (minimum 10%) with high TP concentration (minimum 0.46 mg TP g-1) and low DBD (maximum 0.26 g cm-3) in four of the samples whereas in the other five samples the low LOI (maximum 5.8%) was combined with low TP concentrations (maximum 0.28 mg TP g-1) and higher DBD (minimum 0.39 g cm-3). The sampling points of the transect Bliesenrade (gray squares) clustered together because the sediment samples varied less and were very similar to each other (LOI: 0.63–1.78%, DBD: 1.08–1.45 g cm-3, TP concentration: 0.06–0.10 mg TP g-1, TP stocks: 0.75–1.37 g TP cm-2).
There was a general trend of increasing LOI and TP concentrations but decreasing DBD and nearly constant TP stocks with increasing water depth for all samples of all transects (Supplementary Figure 3). The nearly constant TP stocks can be attributed to the opposite trends in the different transects because in the transects Zingst, Nisdorf, Althagen, Bliesenrade, and Fastbültenhaken the TP stocks increased but for the transects Salzhaken, Pütnitz, and Dabitz the TP stocks decreased with water depth. Correlations between water depth and the sediment parameters were found for the transect Dabitz. In this transect, the sediment samples were additionally evaluated at three sediments depth at each sampling point. The TP concentrations in Dabitz ranged between 0.13 and 0.76 mg TP g DM-1 with increasing values at deeper waters. Additionally, stations below 1 m water depth had an increasing WC and LOI of up to 87% and 17%, respectively. In contrast, highest TP stocks were found nearshore and were decreasing with distance from land (Figure 6), even though the first centimeter was comparably between all stations. The nearshore cores (water depth 0.5–0.7 m) had a TP stock peak in 1–2 cm, even though DBD was highest from 2 to 5 cm sediment depth. This result indicates a P enrichment. Furthermore, combined TP stocks of all horizons were at those nearshore stations at least two times higher, compared to stations below 1 m water depth. The LOI decreased per depth from around 2 to 0.5% at the shallow stations (except at 0.6 m). Samples below 1 m water depth had no clear depth gradient for TP stocks, DBD or OC.
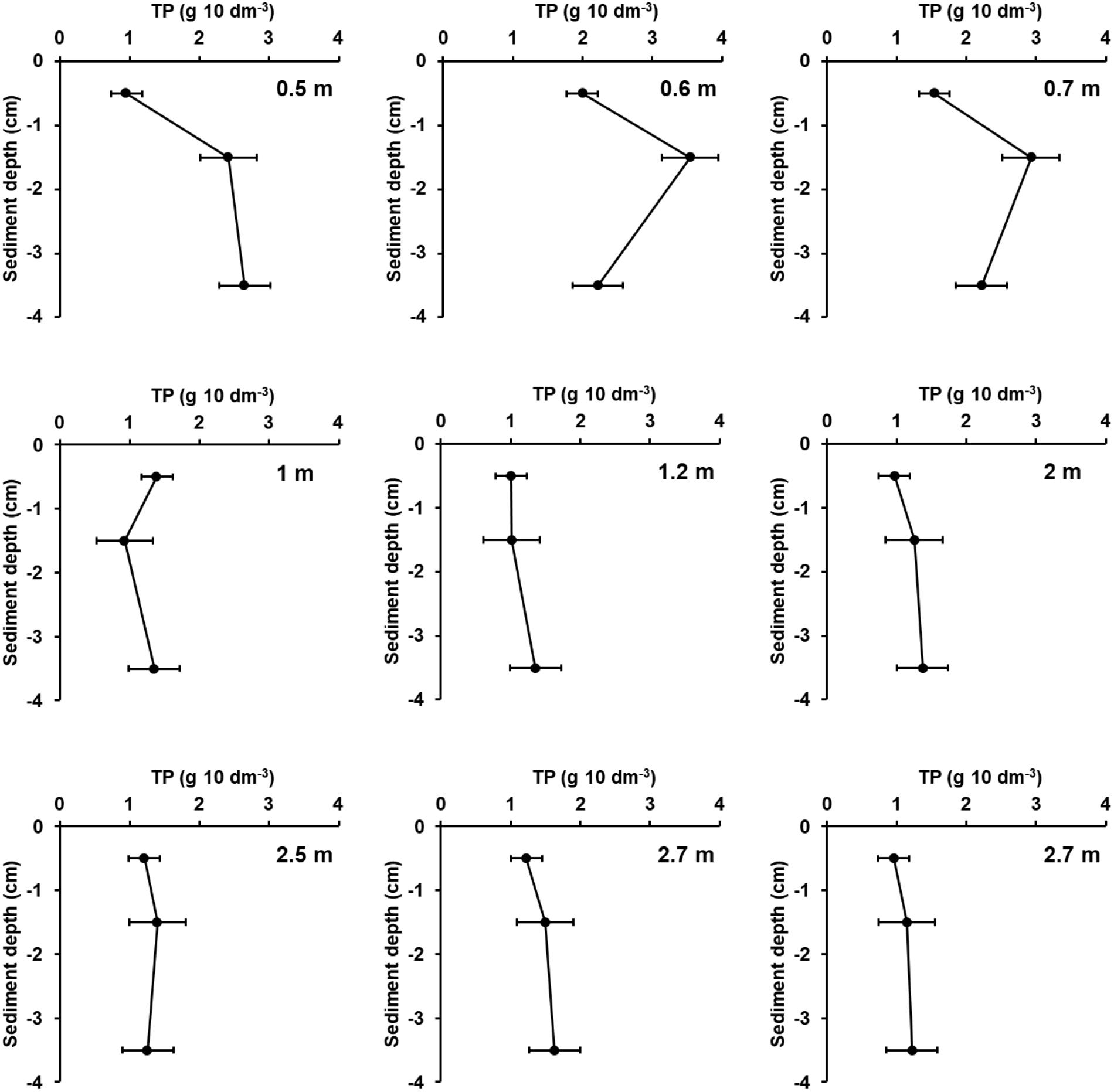
FIGURE 6. Total phosphorus stocks (g TP 10 dm-3, 1 cm slices) within the upper 5 cm of sediment in Dabitz (Grabow). Sediment was sampled in a transect at nine stations and separated in three horizons, 0–1, 1–2, 2–5 cm. The horizon in 3.5 cm depth was a mixed sample of 2–5 cm. Water depth is shown within the diagrams. Station 8 and 9 are spatial separated but in the same water depth. Error bars are standard deviation of four measurement replicates.
Discussion
Evaluation of P-Content in Sediments
The TP concentration (mg g-1) found in the upper sediments of this study were in a comparable range with other sediments (Table 2) of the DZBC (Berghoff et al., 2000), the south-western Baltic Sea (Leipe et al., 2017) and the Gulf of Finland (Lehtoranta and Pitkänen, 2003). However, higher TP concentrations compared to those of our study were detected in sediments of the Archipelago Sea (Conley and Johnstone (1995) and the Landsort Deep (Dijkstra et al., 2016). The LOI (or calculated POC content) found in this study was within the same range as in other studies, but with one of the highest values found in the DZBC. Leipe et al. (2011) found a similar non-linearity between POC and DBD as found in this study. Furthermore, they described that with increasing POC content particles get smaller, whereas mud content <63 μm increases. Simultaneously, WC increases as well, i.e., the amount of water per area increases. Furthermore, the smaller particles have a higher area:volume ratio, compared to bigger particles, which increases P adsorbing capacities (Borggaard, 1983). These results mean, that in areas with fine sediment, like in basins, P concentrations are likely to be higher per g sediment, but decrease per area (Carman and Jonsson, 1991). However, we did not find a high correlation of LOI to TP concentrations, as described by, e.g., McComb et al. (1998) for the Peel-Harvey estuarine system. This result indicate, that there are possibly other factors influencing the TP concentration besides organic content.
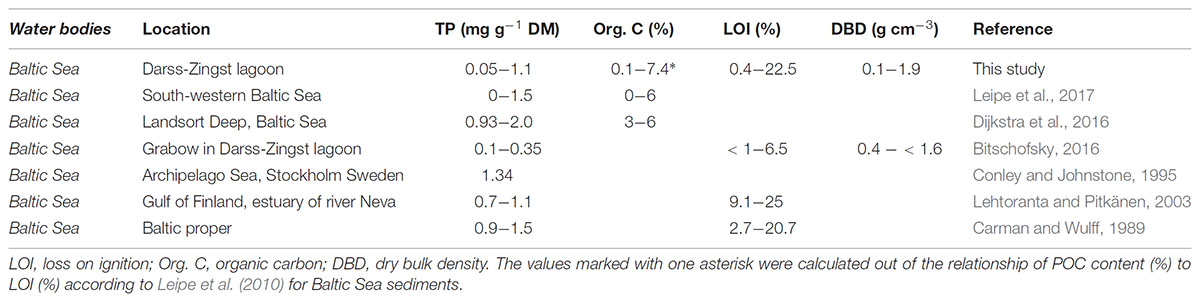
TABLE 2. Overview of commonly found sediment parameter in combination with total phosphorus (TP) concentrations.
The graphical summary in Figure 7 points to this additional problem. The share of the solid phase on the wet mass density strongly decreases with increasing WC. Even though the LOI increases from 0.05 to 17% of the solid phase, it only increases from 0.3 to 2% of the complete sediment volume in the WC rich sediments. It seems unreasonable to assume that this small increase represents completely organic bound P. Furthermore, several authors point to the high pore-water concentrations in such apparently LOI rich sediments (e.g., Søndergaard et al., 1992; Kraal et al., 2013; Bitschofsky, 2016), whereas pore-water concentrations in sediments low on LOI have lower or non-determinable pore-water concentrations (e.g., Bitschofsky, 2016). For example, Bitschofsky (2016) determined 3–5 μmol l-1 dissolved inorganic phosphate in the pore-water of the DZBC at 2 m water depth. A sediment core with 85% WC would have 80–130 ng PO4 cm-3. The water evaporates during drying, but P is adsorbed to the solid phase and is determined as TP. However, TP ranged from 90 to 140 ng cm-3 in this study and is only slightly higher than the assumed pore-water concentration per sediment volume. This assumption brings up the question how P is actually bound in such water rich sediments. Up-to-date analysis methods like synchrotron-based XAS might be necessary to evaluate this question.
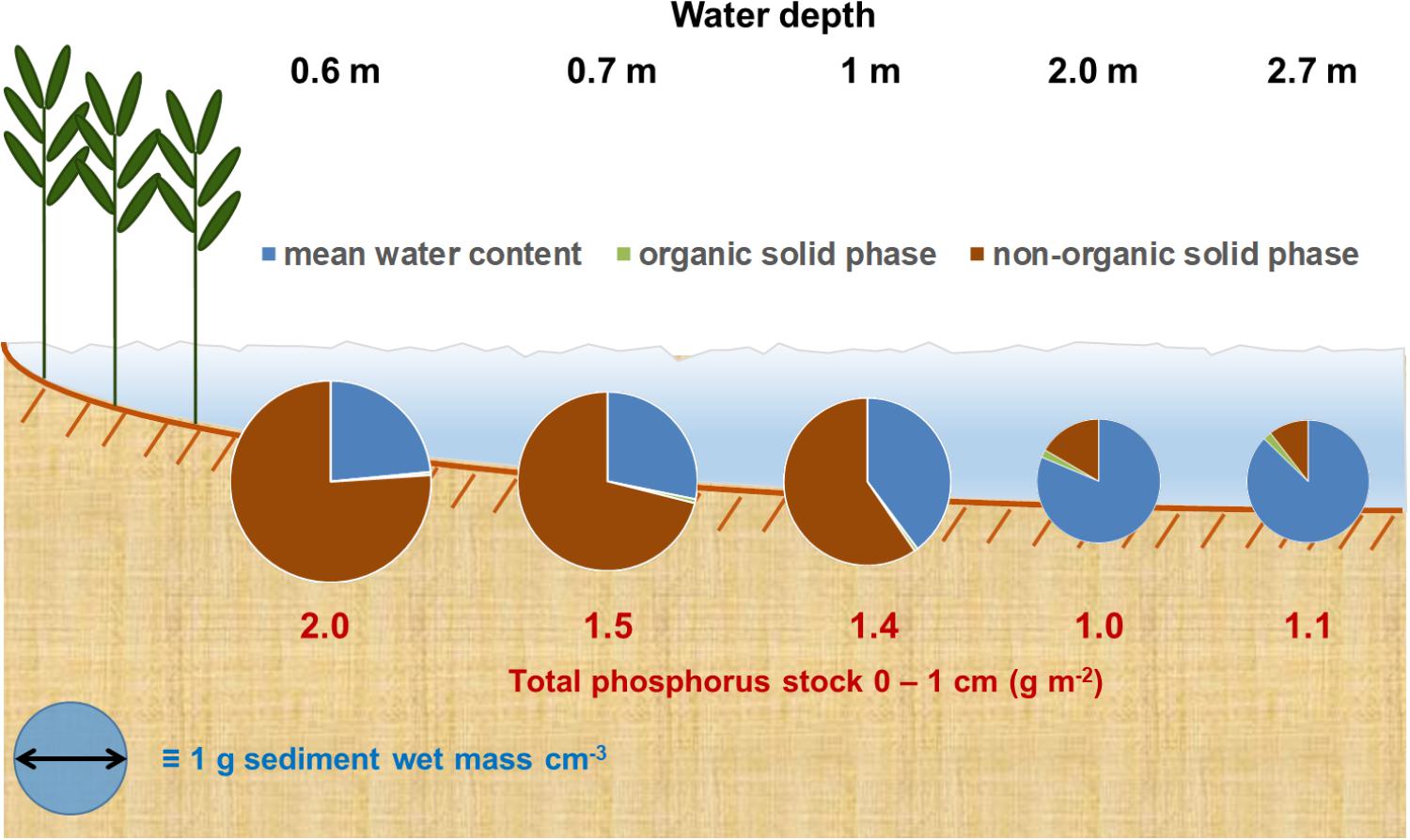
FIGURE 7. Graphical summary of sediment properties development along the exemplary transect in the Grabow. Sediments wet bulk density is direct proportional to the circle’s diameter. Organic solid phase was calculated as share of the whole solid phase per wet sediment volume.
Nonetheless, several locations in the Baltic Sea have areal TP stocks between 1.6 g TP m-2 (Lehtoranta, 2003) and 4.9 g TP m-2 (Puttonen et al., 2014). Those sites showed high TP stocks even at very low DBD, compared to a maximum of 2 g TP m-2 at stations with low DBD in the DZBC. Contrary to these studies, the highest TP stocks were observed in sediments with low LOI and high DBD in this study (up to 6 g m-2, see Figure 3B, station Althagen, Nisdorf). Such findings were also described by Carman and Wulff (1989), who found 9.5 g TP m-2 in highly dense sediments (WC – 29% LOI 2.7%, 0–1 cm), but only 1.15 g TP m-2 in less dense sediments (WC 91%, LOI 20.7%, 0–1 cm) in the Baltic Proper. This discrepancy highlights the importance of the factor sediment density, as small changes in TP concentrations affect TP stocks due to the non-linear relation (Figure 3). Furthermore, these contrary results suggest that knowledge of P loading and cycling within the system is necessary besides the sediment parameter.
Origin of P in Sediments – Location of Sampling Transects
In the PCA Nisdorf, Salzhaken and Althagen clustered together (Figure 5), resulting from similar higher TP stocks and DBDs but lower LOI compared to the other transects. Similarity of these samples is surprising, since these stations are from completely different lagoon parts (Figure 1). However, the hinterland of these transects in Nisdorf and Althagen is intensively used for agriculture whereas that of Salzhaken is mainly used as pasture. This agricultural usage and possibly higher TP input could explain similarity of these transects and enriched of P stocks. Heathwaite (1994) described that runoff, transported suspended matter, N, and P loading increased by a factor of 8–9 from heavily grazed pasture areas intro the adjacent land. This increased nutrient inflow was found in the lake sediments as increased allogenic fraction. Generally, P can be transported into the water by agricultural drainage especially during storm events (e.g., Zimmer et al., 2016), by direct soil erosion with soil particles or by submarine groundwater discharge (Knee and Paytan, 2012). However, it has to be pointed out that phosphorus is strongly adsorbed to soil particles especially amorphous Fe-(hydr)oxides (Cornell and Schwertmann, 2004; Fink et al., 2016; Gypser et al., 2017) and only low percentages of P are extractable by water and/or anion exchange resin (e.g., Koch et al., 2018). That means that transport of P in a dissolved form with drainage water or by groundwater discharge might be restricted especially to extreme storm events, to sandy soils with low P sorption capacity or to soils with high P loads (e.g., Tiemeyer et al., 2009; Knee and Paytan, 2012; Zimmer et al., 2016). Therefore, it is assumed that P loading of the shallow sediments in the DZBC resulted mainly from soil erosion by water and transport of P loaded soil particles from adjacent agricultural land. This assumption is supported by the unchanged positive P accumulation in German agricultural used soils (1.8 kg ha-1 a-1), as described by van Dijk et al. (2016). A change of P stock in the sediments would be less likely with permanent diffuse P loadings, besides the reduced point sources. However, transport proportions of P adsorbed to soil particles and dissolved P into the sediment cannot be estimated at this point. There is no data available on P specific ground water fluxes or soil erosion in this area.
The effects of usage of the adjacent land on P stocks in sediment is further visible in the separation from the above mentioned transects and the clustering in the PCA (Figure 5) of the transects Bliesenrade and Fastbültenhaken. The adjacent land of both transects are without agricultural usage and have comparably low areal TP stock at similar DBDs. The transects Zingst, Pütnitz, and Dabitz are partly separated from the transects of Nisdorf, Salzhaken, and Althagen (Figure 5). At theses transects the land is used as harbor area, former airport with close river connection and agricultural used adjacent land. All these land usages might explain an overall increased P stock, as in the other areas. However, it is interesting, that Zingst, Pütnitz, and Dabitz represent a nutrient gradient, i.e., high TP (4 μmol l-1) concentration in the water column in Pütnitz against low TP (2 μmol l-1) in Dabitz (Berthold et al., 2018b). This result might imply that besides the water column as important P source for the sediment, the adjacent land and subsequent run-off is important as well. The transects in Dabitz and Fastbültenhaken exhibited a wide range in the PCA (Figure 5) pointing to strong differences between sampling points within both transects. At both stations a sharp increase in LOI and TP concentration but decrease in DBD was observed with increasing water depth (Supplementary Figure 3). The transect in Dabitz is located in the western lagoon GB where water exchange with the open Baltic Sea is highest. Fastbültenhaken is in the middle part of the lagoon and is likely subject to changing fresh- and seawater exchange. The clustering of both stations can be a result of different sediment accumulation, due to hydrological conditions. A hydrological model for the DZBC lagoon is missing, therefore no statements are possible regarding underwater currents.
Changes in Sediment P in the Last 40 Years and Ecological Evaluation
The data set from 40 years ago presented a wide range of TP concentrations between 0.05 and 1.5 mg TP g-1 DM (Nausch, 1981) (Table 3). The LOI ranged between 0.6 and >30% in the whole system, where deeper basins (up to 4 m depth) had not exclusively higher LOI values (possible range 1–30%). This distribution was most likely a result of underwater currents and sediment transports. The percentage of mineral sediments (<5% LOI) of the separate lagoon parts ranged between 60% (GB) and 40% (SB). In the present study had a share of 80% of the sediments were mineral sediments. However, these percentage is not representative as straight sampling transects were used, rather than a sampling grid. Furthermore, Nausch (1981) sampled 100 sediment cores per lagoon part, whereas the present study had only a maximum of 17 per lagoon part. Generally, the TP concentrations of Nausch (1981) and the present study are comparable for the range and depth distribution (Table 3). However, the original data set is only available as a range in tables and figures in the cited publication. DBD cannot be attributed to special samples, only to charts and averages. An uncertainty of the TP stock resulted from the missing exact values for the DBD from the old data sets. Sediment density should always be present within publications, as well as GPS coordinates, or deposited at data bases for future comparisons. This data set will be made accessible via PANGAEA1. Methodology of TP extraction was comparable, as the different digestion methods in these studies (HCl) and today (acid persulfate) were equally efficient (Berthold et al., 2015).
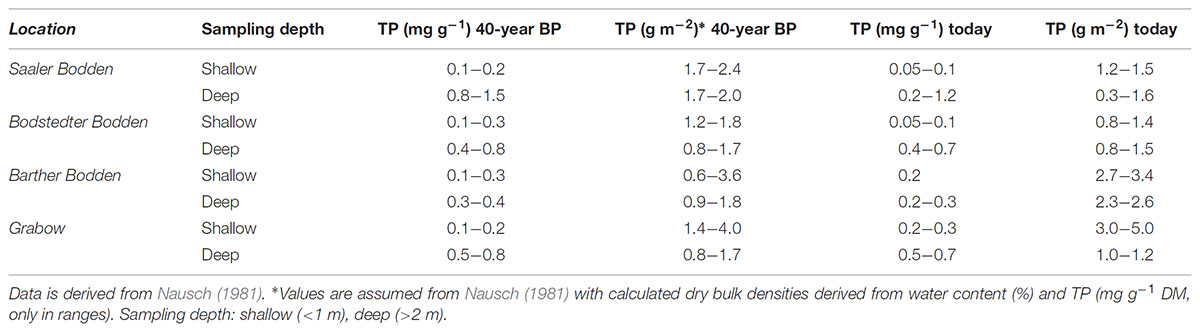
TABLE 3. Total phosphorus (TP) (stock in g P m-2 0–1 cm, and concentration in mg P g dry mass-1) from 40 years ago (40-year BP – before present) compared with today at the same sampling spots.
Generally, the results suggest that a significant P reduction within 40 years was not verifiable. The TP concentration ranges of the upper sediment layers did not change within the last 40 years. For example, sediment samples of the deeper parts (>1 m) were in the same range as already described by Nausch (1981) (Table 3). However, some stations from 2017 had TP concentrations at the lower range compared to 40 years before present. In general, the results found in this study and from 40 years ago are comparable. This similarity of old and new concentrations and stock is somewhat surprising as P inputs were at least reduced by 60 t P a-1 (LUNG, 2013). There were around 290 t P bound in the sediments (0–1 cm), if the mean P stocks of the whole system are re-calculate from 1980. The total P stored in the sediments nowadays is 257 t P, if it is assumed that (1) values of this study were representative for most sediments in the system and (2) that sediment characteristics did not change dramatically, i.e., the percentage of mineral to mud sediments remained constant. Some lowered ranges of TP occurred mainly in BoB and SB, which may reflect lower influxes from the Recknitz River. The reduction in TP influxes was largest in the Recknitz decreasing from 50 to 10 t a-1 during 1980 through 2010 (LUNG, 2013). No matter if the sediment P load decreased or not, the most important proportion of P in the sediment is the portion of P which normally interacts with the water column.
The interactions between the water column and the sediment depend also on the potential of P being released into or exported from the water column. This potential is higher in suboxic waters regardless of the areal TP. In fact, the State Agency for this region (LUNG, 2013) reported that all inner coastal water bodies of the southern German Baltic coast are oxygen-saturated even above the sediments, i.e., >6 mg O2 l-1. One pathway for PO4 in oxic waters is co-precipitation with calcite (Gunduz et al., 2011) or the formation of iron-rich colloids with PO4 (Gunnars et al., 2002). Such PO4-colloids are removed by 40% within ca. 40 h in brackish waters (salinity of around 6) (Gunnars et al., 2002). These results suggest that the oxic environment is favoring net P export from the water column into the sediment. Furthermore, Lampe et al. (2013) described that a wind speed of >8 m/s (5 Bft), or a ground flow velocity of >0.4 m s-1 is necessary to induce resuspension events in the inner lagoon part SB. This wind speed happened only 7% of the time in, e.g., 2011 and a TP export via particulate matter into the Baltic Sea amounted only to 3 t a-1 (Lampe et al., 2013). However, this export is hard to quantify due to frequent water level changes. A regular occurring resuspension can increase the overall cycling of P between the water column and sediment and might gradually even out the TP concentrations in the growing sediments. Nonetheless, these results indicate that abiotic and biotic particles will be trapped within the lagoon and therefore lead to a TP deposition. Even the deeper basins of other lagoon parts (e.g., station Dabitz, GB) were probably permanently affected by transport of fine material down the slope (Håkanson, 1977), which would explain the absent TP peaks in the sediment horizons (Figure 6). This would support the low export rates and the sediment sink function for TP.
There is the possibility that especially high areal TP stocks in shallow areas are subject to frequent TP release events, as they are populated with macrozoobenthos. For example, the burrowing polychaetes Hediste diversicolor can reach average population densities of 700 Ind. m-2 in the shallow areas (<2 m, max. 7000 Ind. m-2) of the BB and GB. This species was not found below 2 m or in the less saline lagoon parts, i.e., BoB and SB (Arndt, 1989). The burrows of H. diversicolor can increase the water-sediment surface by a factor of 3 as well as the nutrient exchange to the overlying water through their ventilation activity (Davey and Watson, 1995). Additionally, Marenzelleria spp. established stable populations since the early 1990s (Zettler, 1996) in this lagoon system with 2,000 to 8,000 Ind. m-2 in all lagoon parts (Zettler, 1997). Marenzelleria can simultaneously shift and increase the PO4 porewater concentrations in the upper 5 cm sediment depth to the sediment surface by around 5 μmol PO4 l-1 in sandy sediments (Renz and Forster, 2014). Both polychaetes, Marenzelleria and H. diversicolor have comparable irrigation rates of 12 l m-2 d-1 at population densities of 3,200 and 800 Ind. m-2, respectively (Hedman et al., 2011). Thus, Marenzelleria spp. can replace H. diversicolor and expand the bioirrigation activity into the inner lagoon waters. Chironomidae occur in high abundances in the SB and BoB with 2,000 to 5,000 Ind. m-2 (Arndt, 1994; Zettler, 1997). They appear to be absent in the BB and GB, which means that bioirrigation by these species for deeper lagoon parts can be neglected. Deeper parts of the lagoon may have lower areal TP stocks as well as less P release by animals. The areal P release probably remains lower in deeper parts even if an average diffusive flux of 28.3 ± 15.2 μmol PO4 l-1 m-2 d-1 is considered compared to a non-determinable, perhaps lower flux in shallow, unpopulated sediments (Bitschofsky, 2016).
However, these transport fluxes were not commonly monitored, as PO4 concentrations in the water column were on median 0.1 μmol PO4 l-1, which is close to the determination limit (0.05 μmol l-1) (Berthold et al., 2018b). One cause can be the luxury consumption of PO4 by phytoplankton (e.g., Taft et al., 1975). A PO4 increase would be missed analytically and phytoplankton may still be permanently fertilized. This low, but permanent fertilization might explain the missing ecosystem improvement regarding a lower phytoplankton biomass and turbidity within the lagoon (Schiewer, 2007; Berthold et al., 2018b). This possible fertilization effect in the shallow areas is even more concerning, as phytoplankton and submerged macrophytes are not subject to light limitation in these areas, i.e., they can use available nutrients directly for growth and biomass increase, instead of storage. On an area base, TP stocks are much higher here and may fertilize phytoplankton and macrophytes considerably. On the other side, the low PO4 concentrations within the system could also be caused by the high macrozoobenthos population. One major shift within the system was the aforementioned Marenzelleria colonization. The increased water-sediment contact surface in worm burrows could also lower water P concentrations through oxic precipitation and increased remineralization as described earlier. Additionally, a present light limitation (Schiewer, 2007) would always cap phytoplankton biomass, regardless of available P. Therefore, it is reasonable to assume that the P reduction caused only a phytoplankton adaptation to the reduced P availability, like postulated in Sas (1989) for lakes. The reduction of P might have triggered the species composition change (Schiewer, 2007) to small cell cyanobacteria with high volume to surface ratios, which are highly competitive in nutrient uptake (e.g., Friebele et al., 1978). This change could also explain the high TN:TP ratios (36–50) throughout the year found in this lagoon system (Berthold et al., 2018b), as cyanobacteria always show high N:P ratios (Finkel et al., 2010). As conclusion, the net P flux to the sediment might have stayed the same, as seen by the constant TP levels in the sediment, but the decreased P inputs were buffered by a changing species composition and inventory.
Such observations are important for management and restoration actions within eutrophic coastal water bodies. Therefore, future restoration measures shall not predominantly focus on apparently high TP concentrations (mg g-1 DM) in the sediment, but rather on diffuse sources in the very shallow areas of coastal water bodies. Those areas can have a greater impact than assumed so far.
Conclusion
Analysis of different characteristics of sediment samples, especially TP content, from different transects in the DZBC pointed to the importance of the land use near the transect, affecting the earlier and present day diffuse P input especially in such an almost closed water system such as the DZBC. However, strong differences between sampling points within a transect seem to call for additional effects of P- and/or sediment distribution such as DZBC internal sediment transport or biological sediment mixing by plants and animals. The sampling of sediment cores with parallel analysis of volume percentage of water, DBD and density specific calculation of P stock underlines the importance of sample preparation and area specific P stocks to evaluate ecological relevant P stocks in sediments. Furthermore, it seems reasonable, that the P stocks did not change in 40 years due to a constant P flux into the sediments and increased bioturbation by invasive species. The phytoplankton composition might have adapted to the lower available P concentrations within the water column and bypassed an ecosystem improvement by, e.g., lower turbidity. Therefore, it can be highlighted that P stock specific evaluation of sediments can draw a different picture of spatial P distribution compared to simple P concentrations in the solid sediment phase. Nevertheless, sediment core analysis have to be extended to separate TP analysis of pore water in the sediment volume and analysis of TP and total concentrations of Al, Ca, Fe, and Mn in the solid phase to get deeper insights into the proportions of dissolved P and adsorbed P as well as in binding partners of P in sediments. This knowledge is urgent necessary to evaluate the potential of P release and its real contribution to eutrophication in shallow lagoon systems and the Baltic Sea. Such enhanced analyses especially of the solid sediment phase from sediment cores from such transects could also contribute to improve the understanding in which diffuse ways P is mainly imported from the adjacent land into the water body. Additionally, a hydrological model seems necessary to determine, where particulate material is transported. This model would help to evaluate, if the lagoon sediment is a permanent P sink, or if particulate matter is transported with a delay into the Baltic Sea.
Author Contributions
MB and VR planned and conducted the sampling. DZ performed the statistics. MB and RS performed the calculations. MB wrote the manuscript with extensive contributions of DZ, VR, and RS. RS conducted a substantial part of the phosphorus analytics.
Funding
This work was done in the context of the BACOSA II project (funded by the German Ministry for Education and Research, funding 03F0737A). This research was performed within the scope of the Leibniz ScienceCampus Phosphorus Research Rostock. We acknowledge financial support by Deutsche Forschungsgemeinschaft and Universität Rostock within the funding programme Open Access Publishing.
Conflict of Interest Statement
The authors declare that the research was conducted in the absence of any commercial or financial relationships that could be construed as a potential conflict of interest.
Acknowledgments
Heike Lippert and Ronny Weigelt supplied us with sediment cores from the outer coast line. Brandt Coleman, Kana Kuriyama, Samira Roshan Khanipour, and Elena Samolov helped with the analytical work. We thank the reviewers with their comments and constructive criticism, which improved the manuscript, as well as the editorial and guest editorial board of this special issue.
Supplementary Material
The Supplementary Material for this article can be found online at: https://www.frontiersin.org/articles/10.3389/fmars.2018.00305/full#supplementary-material
Abbreviations
DBD, dry bulk density; DM, dry mass; DZBC, Darß-Zingst Bodden chain; LOI, loss on ignition; TP, total phosphorus; WC, water content.
Footnotes
References
Albrecht, M., Pröschold, T., and Schumann, R. (2017). Identification of Cyanobacteria in a eutrophic coastal lagoon on the Southern Baltic Coast. Front. Microbiol. 8:923. doi: 10.3389/fmicb.2017.00923
Arndt, E. A. (1989). “Ecological, physiological and historical aspects of brackish water fauna distribution,” in European Marine Biology Symposium, 23rd Edn, eds J. S. Ryland and P. A. Tyler (Denmark: Olsen & Olsen),327–338.
Arndt, E. A. (1994). Struktur und Dynamik des Makrozoobenthos in der Darß-Zingster Boddenkette im Laufe der Letzten 25 Jahre unter besonderer Berücksichtigung der Makrozoobenthos — Ent-wicklung im Saaler Bodden von 1986-1990. Rostocker Meeresbiol. Beit. 2, 93–119.
Asmala, E., Carstensen, J., Conley, D. J., Slomp, C. P., Stadmark, J., and Voss, M. (2017). Efficiency of the coastal filter: nitrogen and phosphorus removal in the Baltic Sea. Limnol. Oceanogr. 62, 222–238. doi: 10.1002/lno.10644
Avnimelech, Y., Ritvo, G., Meijer, L. E., and Kochba, M. (2001). Water content, organic carbon and dry bulk density in flooded sediments. Aquac. Eng. 25, 25–33. doi: 10.1016/S0144-8609(01)00068-1
Berghoff, S., Schlungbaum, G., and Selig, U. (2000). Phosphorus in sediments from coastal waters of Mecklenburg-Vorpommern (southern Baltic sea). Proc. Mar. Sci. 2, 161–173. doi: 10.1016/S1568-2692(00)80013-3
Berthold, M., Karsten, U., von Weber, M., Bachor, A., and Schumann, R. (2018b). Phytoplankton can bypass nutrient reductions in eutrophic coastal water bodies. Ambio 47, 146–158. doi: 10.1007/s13280-017-0980-0
Berthold, M., Karstens, S., Buczko, U., and Schumann, R. (2018a). Potential export of soluble reactive phosphorus from a coastal wetland in a cold-temperate lagoon system: buffer capacities of macrophytes and impact on phytoplankton. Sci. Total Environ. 616–617, 46–54. doi: 10.1016/j.scitotenv.2017.10.244
Berthold, M., Zimmer, D., and Schumann, R. (2015). A simplified method for total phosphorus digestion with potassium persulphate at sub-boiling temperatures in different environmental samples. Rostocker Meeresbiol. Beiträge 25, 7–25.
Bitschofsky, F. (2016). Phosphorus Dynamics in Sediments of Darß-Zingst Bodden Chain, a Eutrophic Estuary in the Southern Baltic Sea. Available at: http://rosdok.uni-rostock.de/resolve/id/rosdok_disshab_0000001782
BMUB, and BMEL (2016). Nitrate Report 2016 - Shared Report of the Federal Ministry for Environment, Nature Conversation, Building and Nuclear Safety and the Federal Ministry of Food and Agriculture. Bonn: BMUB.
Borggaard, O. K. (1983). Effect of surface area and mineralogy of iron oxides on their surface charge and anion adsorption properties. Clays Clay Miner. 31, 230–232. doi: 10.1346/CCMN.1983.0310309
Carman, R., and Jonsson, P. (1991). Distribution patterns of different forms of phosphorus in some surficial sediments of the Baltic Sea. Chem. Geol. 90, 91–106. doi: 10.1016/0009-2541(91)90036-Q
Carman, R., and Wulff, F. (1989). Adsorption capacity of phosphorus in Baltic Sea sediments. Estuar. Coast. Shelf Sci. 29, 447–456. doi: 10.1016/0272-7714(89)90079-6
Childers, D. L., Corman, J., Edwards, M., and Elser, J. J. (2011). Sustainability challenges of phosphorus and food: solutions from closing the human phosphorus cycle. Bioscience 61, 117–124. doi: 10.1525/bio.2011.61.2.6
Civan, A., Worrall, F., Jarvie, H. P., Howden, N. J. K., and Burt, T. P. (2018). Forty-year trends in the flux and concentration of phosphorus in British rivers. J. Hydrol. 558, 314–327. doi: 10.1016/j.jhydrol.2018.01.046
Conley, D. J., and Johnstone, R. W. (1995). Biogeochemistry of N, P and Si in Baltic Sea sediments: response to a simulated deposition of a spring bloom. Mar. Ecol. Prog. Ser. 122, 265–276. doi: 10.3354/meps122265
Cornell, R. M., and Schwertmann, U. (2004). The Iron Oxides: Structure, Properties, Reactions, Occurences and Uses, 2nd Edn. Weinheim: Wiley-VCH, doi: 10.1002/3527602097
Davey, J. T., and Watson, P. G. (1995). The activity of Nereis diversicolor (Polychaeta) and its impact on nutrient fluxes in estuarine waters. Ophelia 41, 57–70. doi: 10.1080/00785236.1995.10422037
Dijkstra, N., Slomp, C. P., and Behrends, T. (2016). Vivianite is a key sink for phosphorus in sediments of the Landsort Deep, an intermittently anoxic deep basin in the Baltic Sea. Chem. Geol. 438, 58–72. doi: 10.1016/j.chemgeo.2016.05.025
Duarte, C. M., Conley, D. J., Carstensen, J., and Sanchez-Camacho, M. (2009). Return to neverland: shifting baselines affect eutrophication restoration targets. Estuaries Coast. 32, 29–36. doi: 10.1007/s12237-008-9111-2
Faas, R., and Wartel, S. (2006). Rheological properties of sediment suspensions from eckernförde and kieler förde bays, western Baltic Sea. Int. J. Sediment. Res. 21, 24–41.
Fink, J. R., Inda, A. V., Tiecher, T., and Barrón, V. (2016). Iron oxides and organic matter on soil phosphorus availability. Ciênc. Agrotecnol. 40, 369–379. doi: 10.1590/1413-70542016404023016
Finkel, Z. V., Beardall, J., Flynn, K. J., Quigg, A., Rees, T. A. V., and Raven, J. A. (2010). Phytoplankton in a changing world: cell size and elemental stoichiometry. J. Plankton Res. 32, 119–137. doi: 10.1093/plankt/fbp098
Friebele, E. S., Correll, D. L., and Faust, M. A. (1978). Relationship between phytoplankton cell size and the rate of orthophosphate uptake: in situ observations of an estuarine population. Mar. Biol. 45, 39–52. doi: 10.1007/BF00388976
Gunduz, B., Aydin, F., Aydin, I., and Hamamci, C. (2011). Study of phosphorus distribution in coastal surface sediment by sequential extraction procedure (NE Mediterranean Sea, Antalya-Turkey). Microchem. J. 98, 72–76. doi: 10.1016/j.microc.2010.11.006
Gunnars, A., Blomqvist, S., Johansson, P., and Andersson, C. (2002). Formation of Fe (III) oxyhydroxide colloids in freshwater and brackish seawater, with incorporation of phosphate and calcium. Geochim. Cosmochim. Acta 66, 745–758. doi: 10.1016/S0016-7037(01)00818-3
Gustafsson, B. G., Schenk, F., Blenckner, T., Eilola, K., Meier, H. E. M., Müller-Karulis, B., et al. (2012). Reconstructing the development of baltic sea eutrophication 1850-2006. Ambio 41, 534–548. doi: 10.1007/s13280-012-0318-x
Gypser, S., Hirsch, F., Schleicher, A. M., and Freese, D. (2017). Impact of crystalline and amorphous iron- and aluminum hydroxides on mechanisms of phosphate adsorption and desorption. J. Environ. Sci. 70, 175–189. doi: 10.1016/j.jes.2017.12.001
Håkanson, L. (1977). The influence of wind, fetch, and water depth on the distribution of sediments in Lake Vänern. Sweden. Can. J. Earth Sci. 14, 397–412. doi: 10.1139/e77-040
Hansen, H. P., and Koroleff, F. (1999). “Determination of nutrients,” in Methods of Seawater analysis, eds K. Grasshoff, K. Kremling, and M. Ehrhardt (Weinheim: Wiley-VCH), 159–251. doi: 10.1002/9783527613984.ch10
Heathwaite, A. L. (1994). Chemical fractionation of lake sediments to determine the effects of land-use change on nutrient loading. J. Hydrol. 159, 395–421. doi: 10.1016/0022-1694(94)90269-0
Hedman, J. E., Gunnarsson, J. S., Samuelsson, G., and Gilbert, F. (2011). Particle reworking and solute transport by the sediment-living polychaetes Marenzelleria neglecta and Hediste diversicolor. J. Exp. Mar. Bio. Ecol. 407, 294–301. doi: 10.1016/j.jembe.2011.06.026
HELCOM. (2018). Sources and pathways of nutrients to the Baltic Sea. Balt. Sea Environ. Proc. 153:48.
Jensen, H. S., Mortensen, P. B., Andersen, F. O., Rasmussen, E., and Jensen, A. (1995). Phosphorus cycling in a coastal marine sediment, Aarhus Bay, Denmark. Limnol. Oceanogr. 40, 908–917. doi: 10.4319/lo.1995.40.5.0908
Karstens, S., Buczko, U., Jurasinski, G., Peticzka, R., and Glatzel, S. (2016). Impact of adjacent land use on coastal wetland sediments. Sci. Total Environ. 550, 337–348. doi: 10.1016/j.scitotenv.2016.01.079
Knee, K. L., and Paytan, A. (2012). Submarine Groundwater Discharge: A Source of Nutrients, Metals, and Pollutants to the Coastal Ocean. New York, NY: Elsevier, doi: 10.1016/B978-0-12-374711-2.00410-1
Koch, M., Kruse, J., Eichler-Löbermann, B., Zimmer, D., Willbold, S., Leinweber, P., et al. (2018). Phosphorus stocks and speciation in soil profiles of a long-term fertilizer experiment: evidence from sequential fractionation, P K-edge XANES, and31P NMR spectroscopy. Geoderma 316, 115–126. doi: 10.1016/j.geoderma.2017.12.003
Köster, M., and Meyer-Reil, L. A. (2001). Characterization of carbon and microbial biomass pools in shallow water coastal sediments of the southern Baltic Sea (Nordrügensche Bodden). Mar. Ecol. Prog. Ser. 214, 25–41. doi: 10.3354/meps214025
Kraal, P., Burton, E. D., Rose, A. L., Cheetham, M. D., Bush, R. T., and Sullivan, L. A. (2013). Decoupling between water column oxygenation and benthic phosphate dynamics in a shallow eutrophic estuary. Environ. Sci. Technol. 47, 3114–3121. doi: 10.1021/es304868t
Lampe, R., Baudler, H., Schumann, R., and Buckmann, K. (2013). Restaurierung der Darß-Zingster Boddenkette (DZBK) – Bilanzierung des Wasser- und Detritusaustausches in der DZBK. Greifswald: University of Greifswald.
Lehtoranta, J. (2003). Dynamics of sediment phosphorus in the brackish Gulf of Finland. Monogr. Boreal Environ. Res. 24, 5–58.
Lehtoranta, J., and Pitkänen, H. (2003). Binding of phosphate in sediment accumulation areas of the eastern Gulf of Finland. Baltic Sea. Hydrobiologia 492, 55–67. doi: 10.1023/A:1024869929510
Leipe, T., Naumann, M., Tauber, F., Radtke, H., Friedland, R., Hiller, A., et al. (2017). Regional distribution patterns of chemical parameters in surface sediments of the south-western Baltic Sea and their possible causes. Geomarine Lett. 37, 593–606. doi: 10.1007/s00367-017-0514-6
Leipe, T., Tauber, F., and Vallius, H. (2011). Particulate organic carbon (POC) in surface sediments of the Baltic Sea. Geomarine Lett. 31, 175–188. doi: 10.1007/s00367-010-0223-x
Leipe, T., Tauber, F., Vallius, H., Virtasalo, J., Uścinowicz, S., Kowalski, N., et al. (2010). Particulate organic carbon (POC) in surface sediments of the Baltic Sea. Geomarine Lett. 31, 175–188.
Liu, Y., Villalba, G., Ayres, R. U., and Schroder, H. (2008). Global phosphorus flows and environmental impacts from a consumption perspective. J. Ind. Ecol. 12, 229–247. doi: 10.1111/j.1530-9290.2008.00025.x
Lu, C., and Tian, H. (2017). Global nitrogen and phosphorus fertilizer use for agriculture production in the past half century: shifted hot spots and nutrient imbalance. Earth Syst. Sci. Data 9, 181–192. doi: 10.5194/essd-9-181-2017
LUNG (2013). Development and Status of Nutrient Loads in the Coastal Water Bodies of Mecklenburg-Vorpommern. Güstrow: Berichte zur Gewässergüte.
McComb, A. J., Qiu, S., Lukatelich, R. J., and McAuliffe, T. F. (1998). Spatial and temporal heterogeinity of sediment phosphorus in the Peel-Harvey estuarine system. Estuar. Coast. Shelf Sci. 47, 561–577. doi: 10.1006/ecss.1998.0389
Nausch, G. (1981). Die Sedimente der Darß-Zingster Boddengewässer - Zustandsanalyse und Stellung im Phosphorkreislauf. DissOnline Universität Rostock.
Nausch, G., Bachor, A., Petenati, T., Voss, J., and Von Weber, M. (2011). Nutrients in the German coastal waters of the Baltic Sea and adjacent areas (in German). Meeresumwelt Aktuell Nord und Ostsee 2011, 1–16.
Nausch, G., and Schlungbaum, G. (1984). Sedimentchemische Untersuchungen in Küstengewässern der Deutschen Demokratischen Republik Teil 17. Spezielle Untersuchungen zur Dynamik der Oberflächensedimente eines flachen Boddengewässers (Barther Bodden). Acta Hydrochim. Hydrobiol. 12, 61–72. doi: 10.1002/aheh.19840120112
Nilsson, S. (2006). International River Basins in the Baltic Sea Region. Report of the BSR INTERREG III B Programme. Available at: http://www.baltex-research.eu/material/downloads/riverbasins.pdf [accessed April 15, 2017].
Pitkänen, H., Lehtoranta, J., and Räike, A. (2001). Internal nutrient fluxes counteract decreases in external load: the case of the estuarial Eastern Gulf of Finland, Baltic Sea. Ambio 30, 195–201. doi: 10.1579/0044-7447-30.4.195
Puttonen, I., Mattila, J., Jonsson, P., Karlsson, O. M., Kohonen, T., Kotilainen, A., et al. (2014). Distribution and estimated release of sediment phosphorus in the northern Baltic Sea archipelagos. Estuar. Coast. Shelf Sci. 145, 9–21. doi: 10.1016/j.ecss.2014.04.010
Renz, J. R., and Forster, S. (2014). Effects of bioirrigation by the three sibling species of Marenzelleria spp. on solute fluxes and porewater nutrient profiles. Mar. Ecol. Prog. Ser. 505, 145–159. doi: 10.3354/meps10756
Ruttenberg, K. C. (2003). “The Global Phosphorus Cycle,” in Treatise on Geochemistry, eds H. D. Holland and K. K. Turekian (Oxford: Pergamon), 585–643. doi: 10.1016/B0-08-043751-6/08153-6
Sas, H. (1989). “The relation between inlake-P concentration and algal biomass indicators (subsystem 2),” in Lake Restoration by Reduction of Nutrient Loading: Expectations, Experiences, Extrapolations, eds H. Sas, J. Ahlgren, H. Bernhardt, B. Boström, J. Clasen, and C. Forsberg (Sankt Augustin: RicharzAcademia Verlag), 102–148.
Schiewer, U. (2007). “Darß-Zingst Boddens, Northern Rügener Boddens and Schlei,” in Ecology of Baltic Coastal Waters, eds U. Schiewer, M. M. Caldwell, G. Heldmaier, R. B. Jackson, O. L. Lange, and H. A. Mooney (Berlin: Springer), 35–86.
Schlungbaum, G. (1982). Sedimentchemische Untersuchungen in den Küstengewässern der DDR. Teil 10: Die Rolle der Stoffaustauschprozesse an der Sediment/Wasser-Kontaktzone eutropher Flachgewässer und Möglichkeiten zur Untersuchung am Beispiel des Phosphatkreislaufes — ein Überblick am Beispiel der Darß-Zingster Boddenkette. 10, 119–134. doi: 10.1002/aheh.19820100204
Schlungbaum, G., Nausch, G., and Baudler, H. (1994). Sedimentstruktur und Sedimentdynamik in den Darß-Zingster Boddengewässern. Rostock. Meeresbolog. Beitr. 2, 27–40.
Schröder, J. J., Cordell, D., Smit, A. L., and Rosemarin, A. (2010). Sustainable use of phosphorus. Wageningen. Available at: http://ec.europa.eu/environment/natres/pdf/phosphorus/sustainable_use_phosphorus.pdf
Smil, V. (2000). Phosphorus in the environment: natural flows and human interferences. Annu. Rev. Energy Environ. 25, 53–88. doi: 10.1146/annurev.energy.25.1.53
Søndergaard, M., Kristensen, P., and Jeppesen, E. (1992). Phosphorus release from resuspended sediment in the shallow and wind-exposed Lake Arresø, Denmark. Hydrobiologia 228, 91–99. doi: 10.1007/BF00006480
Taft, J. L., Taylor, W. R., and McCarthy, J. J. (1975). Uptake and release of phosphorus by phytoplankton in the Chesapeake Bay estuary, USA. Mar. Biol. 33, 21–32. doi: 10.1007/BF00394997
Tiemeyer, B., Kahle, P., and Lennartz, B. (2009). Phosphorus losses from an artificially drained rural lowland catchment in North-Eastern Germany. Agric. Water Manag. 96, 677–690. doi: 10.1016/j.agwat.2008.10.004
Vahtera, E., Conley, D. J., Gustafsson, B. G., Kuosa, H., Pitkänen, H., Savchuk, O. P., et al. (2007). Internal ecosystem feedbacks enhance nitrogen-fixing cyanobacteria blooms and complicate management in the Baltic Sea. Ambio J. Hum. Environ. 36, 186–194. doi: 10.1579/0044-7447(2007)36[186:IEFENC]2.0.CO;2
van Dijk, K. C., Lesschen, J. P., and Oenema, O. (2016). Phosphorus flows and balances of the European Union Member States. Sci. Total Environ. 542, 1078–1093. doi: 10.1016/j.scitotenv.2015.08.048
Van Vuuren, D. P., Bouwman, A. F., and Beusen, A. H. W. (2010). Phosphorus demand for the 1970-2100 period: a scenario analysis of resource depletion. Glob. Environ. Chang. 20, 428–439. doi: 10.1016/j.gloenvcha.2010.04.004
Verstraeten, G., and Poesen, J. (2001). Variability of dry sediment bulk density between and within retention ponds and its impact on the calculation of sediment yields. Earth Surf. Process. Landf. 26, 375–394. doi: 10.1002/esp.186
Yan, Z., Han, W., Peñuelas, J., Sardans, J., Elser, J. J., Du, E., et al. (2016). Phosphorus accumulates faster than nitrogen globally in freshwater ecosystems under anthropogenic impacts. Ecol. Lett. 19, 1237–1246. doi: 10.1111/ele.12658
Zettler, M. L. (1996). Successful establishment of the spionid polychaete. Arch. Fish. Mar. Res. 43, 273–284.
Zettler, M. L. (1997). Population dynamics, growth and production of the neozoon Marenzelleria cf. viridis (Verrill, 1873) (Polychaeta: Spionidae) in a coastal water of the southern Baltic Sea. Aquat. Ecol. 31, 177–186. doi: 10.1023/A:1009903521182
Keywords: phosphorus concentration, sediment, brackish lagoon, dry bulk density, Baltic Sea, organic content
Citation: Berthold M, Zimmer D, Reiff V and Schumann R (2018) Phosphorus Contents Re-visited After 40 Years in Muddy and Sandy Sediments of a Temperate Lagoon System. Front. Mar. Sci. 5:305. doi: 10.3389/fmars.2018.00305
Received: 29 April 2018; Accepted: 10 August 2018;
Published: 05 September 2018.
Edited by:
Karol Kulinski, Institute of Oceanology (PAN), PolandReviewed by:
Dirk De Beer, Max-Planck-Gesellschaft (MPG), GermanySarah Elizabeth Reynolds, University of Portsmouth, United Kingdom
Copyright © 2018 Berthold, Zimmer, Reiff and Schumann. This is an open-access article distributed under the terms of the Creative Commons Attribution License (CC BY). The use, distribution or reproduction in other forums is permitted, provided the original author(s) and the copyright owner(s) are credited and that the original publication in this journal is cited, in accordance with accepted academic practice. No use, distribution or reproduction is permitted which does not comply with these terms.
*Correspondence: Maximilian Berthold, maximilian.berthold@uni-rostock.de